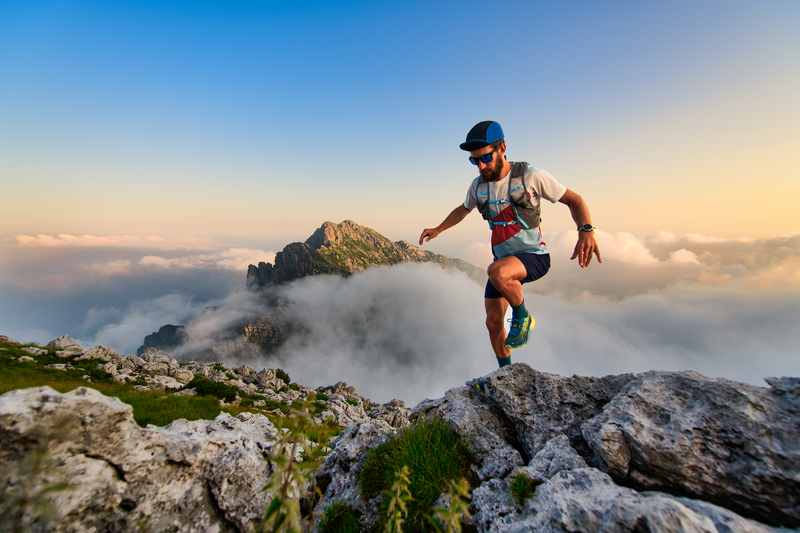
95% of researchers rate our articles as excellent or good
Learn more about the work of our research integrity team to safeguard the quality of each article we publish.
Find out more
ORIGINAL RESEARCH article
Front. Mar. Sci. , 11 October 2019
Sec. Marine Evolutionary Biology, Biogeography and Species Diversity
Volume 6 - 2019 | https://doi.org/10.3389/fmars.2019.00628
This article is part of the Research Topic Exploring the Genetic Diversity of Marine Organisms Based on the Analysis of Chromosome and Genomic DNA Markers View all 10 articles
Karyotype stasis, characterized by the absence of changes in chromosome number, genomic structure, and ploidy, is a recurrent condition in many biological groups, such as plants, amphibians, birds, and fishes. In fishes, the Percomorpha clade (>17,000 species), especially, its largest series, Eupercaria (>6,000 species and 161 families), shows an extensively shared karyotype with 2n = 48 acrocentrics; the phylogenetic extension in this series is still unknown. Haemulidae (grunts) family, which is one of the groups in the Eupercaria series, are effectively distributed across wide geographic areas; these fishes have a huge ecological relevance in reef environments and have been shown to be useful in clarifying the karyotype stasis in marine fish. In the western Atlantic Ocean, the Amazonas/Orinoco biogeographic barrier, which bounds the zoogeographic marine Provinces of the Caribbean and Brazil, has a variable effect on the gene flow in diverse fish groups. Geographically, this barrier is located inside the distribution area of several fish species of Atlantic, allowing the examination of its role on the eventual karyotype differentiations in the populations of grunts. In this sense, cytogenomic analyses were carried out in eight haemulid species by conventional cytogenetic approaches (Giemsa staining, C-banding, and Ag-NORs technique), staining with base-specific fluorochromes, and two-color fluorescent in situ hybridization (FISH) with 5S and 18S rDNA probes. Additionally, we performed an exhaustive survey on the cytogenetic data of species from the Eupercaria series to assess the general context of karyotype divergences. Haemulid species and populations showed a pronounced sharing of karyotypes with 2n = 48a, simple NORs, and reduced centromeric heterochromatin, apart from recurrent patterns of ribosomal 5S and 18S rDNA sites. Karyotype similarities persisted at intra- and congeneric levels in the Haemulidae family, indicating accentuated syntenic conservatism through large divergence periods. Stable karyotype patterns were extensively present in the majority of members from Eupercaria (88% of the clades in this series). These results indicate that karyotype stasis, which is found in a large spectrum of marine fishes, is a multifactorial process in terms of phylogenetic, biological, and biogeographic contexts.
Karyotype stasis refers to the absence of changes in the chromosome number and structure, ploidy levels, as well as the genomic composition during phylogenetic divergence (Kahl, 2015). This process has been described in groups as diverse as plants (Mandáková et al., 2010; Bomfleur et al., 2014; Samad et al., 2016), amphibians (Sessions and Kezer, 1991; Aprea et al., 2004), birds (Ellegren, 2010), and fish (Molina, 2007; Motta-Neto et al., 2011a, b; Molina et al., 2014).
Extensive cytogenetic analyses of at least one species among about 45% of families belonging to Percomorpha (Arai, 2011), a clade with >17,000 species (Hughes et al., 2018), have highlighted notable karyotype similarities among lineages showing very ancient stages of divergence (Molina et al., 2013; Costa et al., 2016).
Meta-analysis approaches have improved the understanding of karyotype stasis in marine fish (Molina, 2007), evidencing cases of slow rate of karyotype evolution (Molina et al., 2014). Previously published data have revealed an astonishing panel of clades sharing karyotypes with 2n = 48 acrocentric chromosomes, in addition to other traits such as reduced amount of heterochromatin, and occurrence of a single pair of chromosome-bearing nucleolar organizer regions (Ag-NORs/rDNA 18S sites) (Galetti et al., 2000; Molina, 2007).
The family Haemulidae, a typical group of the Percomorpha clade, comprises 136 species of fishes that are commonly known as grunts (Fricke et al., 2019) and distributed circumglobally in tropical and subtropical oceanic waters. Grunts are small-to-medium-sized carnivorous fishes that predominantly inhabit rocky substrates at a low depth (Nelson et al., 2016). This group has conservative karyotypes (Motta-Neto et al., 2012), a well-known phylogenetic relationship (Rocha et al., 2008; Tavera et al., 2012), and several examples of sibling-species divergence in the New World (Tavera et al., 2018; Bernal et al., 2019; Tavera and Wainwright, 2019), making it a good model for the evaluation of slow chromosome changes in a space-temporal context.
Remarkable phylogenetic reorganizations have occurred in the Percomorpha clade (Near et al., 2012; Betancur-R et al., 2013, 2017; Hughes et al., 2018). Among the nine series proposed in this group, Eupercaria is the largest, with more than 6000 species arranged in 161 families and at least 17 orders (Hughes et al., 2018). Eupercaria encompasses some of the most diverse orders (Betancur-R et al., 2017), which have received considerable attention with regards to their cytogenetic data.
To estimate karyotype stasis at different scales of diversity, we compiled the karyotype data for fishes from Eupercaria, the largest series in the Percomorpha (=Percomorphaceae) clade, and performed cytogenetic analyses of eight Haemulidae species and Atlantic populations by means of conventional techniques, staining with base-specific fluorochromes, and fluorescent in situ hybridization (FISH) with 18S and 5S rDNA sequences.
The eight species of the family Haemulidae used in the cytogenetic analysis (Figure 1) were collected using fishing nets from the Rio Grande do Norte state coast in northeastern Brazil, the Fernando de Noronha Archipelago in the western Atlantic region, and the Caribbean, along the coast of Key Largo, Florida (United States). Information regarding the species and samples is shown in Table 1.
Figure 1. Map of the collection sites of the analyzed Haemulidae species. The species of the Brazilian Province were obtained from the Archipelago of Fernando de Noronha (FN) and the Rio Grande do Norte State coast (RN), while the species of the Caribbean Province were obtained from Key Largo, Florida (United States).
The samples were collected with permission from the Chico Mendes Institute for Biodiversity Conservation (ICMBio/SISBio) (License# 02001.001902/06-82). All the experimental procedures were approved by the Committee of Ethics for the use of Animals of the Federal University of Rio Grande do Norte (# 044/2015).
The individuals were subjected to mitotic stimulation for 18–24 h (Molina et al., 2010). The chromosome preparations were obtained from the cells of the cephalic kidney, in accordance with the method described by Gold et al. (1990). The nucleolar organizer regions (NORs) and heterochromatin were analyzed by the Ag-NOR technique (Howell and Black, 1980) and C-banding (Sumner, 1972), respectively. Additionally, the chromosome spreads were staining with CMA3/DAPI base-specific fluorochromes (Schweizer, 1980).
The 5S and 18S rDNA probes were isolated from the genome of Rachycentron canadum (Euteleostei, Perciformes) and amplified using primers A 5′-TAC GCC CGA TCT CGT CG ATC-3′ and B 5′-CGA GCT GGT ATG GCC GTA AGC-3′ (Pendás et al., 1994) and NS1 5′-GTA GTC ATA TGC TTG TCTC-3′ and NS8 5′-TCC GCA GGT TCA CCT ACG GA-3′ (White et al., 1990), respectively. The 5S and 18S rDNA probes, which were obtained by the PCR amplification of nuclear DNA, contained 200 bp and 1400 bp of the rRNA genes, respectively. The mapping of 5S and 18S rDNA sequences was performed by in situ hybridization (Pinkel et al., 1986). The 5S rDNA probes were labeled by nick translation with biotin-14-dATP (Roche, Mannheim, Germany), and the 18S rDNA probes were labeled with digoxigenin-11-dUTP (Roche, Mannheim, Germany), according to the manufacturer’s specifications.
About thirty metaphases of each individual were analyzed using optical microscopy under a 1000× magnification, and photographed using an OlympusTM BX51 epifluorescence photomicroscope, coupled with an Olympus DP-72 digital capture system using the cellSens® OlympusTM software. For the preparation of karyotypes, the chromosomes were classified in terms of their arms ratios (Levan et al., 1964) and organized in the descending order of size.
The survey of karyotype data for members from the Eupercaria series (sensu Hughes et al., 2018) was performed by running searches in several scientific web portals.
To ensure adequate phylogenetic representation, karyotype data (2n and NF – chromosome arms) of 671 species (∼10% of total species) distributed into 62 families (38.5% of the families) and 15 orders (88% of the orders) of Eupercaria (data summarized as Supplementary Material) were analyzed. The karyotypes of species within the Eupercaria series were categorized based on 2n and NF values greater than, equal to, or smaller than the basal karyotype of 2n = 48 acrocentric chromosomes.
NF (chromosome arm number) was determined considering metacentric/submetacentric chromosomes having two arms and subtelocentric/acrocentric chromosomes having one single arm. In species with sex chromosome systems, the karyotypes of the homogametic sex were considered as the standard for the species.
All the eight haemulid species analyzed had 2n = 48 acrocentric chromosomes (NF = 48). The karyotypes were remarkably symmetrical, exhibiting a small size variation between the largest and the smallest chromosome pairs. Individuals of A. virginicus, H. squamipinna and H. parra presented chromosome pairs with visible secondary constrictions, co-localized with the NORs (Figures 2, 3).
Figure 2. Karyotypes of Anisotremus surinamensis, A. virginicus, and Paranisotremus moricandi visualized by conventional staining with Giemsa, C-banding, and double-FISH with18S rDNA (red) and 5S rDNA (green) probes. The NOR-bearing chromosomes are presented in the larger boxes, from the top to the bottom, as observed after silver staining, C-banding, and CMA3/DAPI staining. Scale bar = 5 μm.
Figure 3. Karyotypes of Brachygenys chrysargyreum, Haemulon flavolineatum, H. parra, H. squamipinna, and H. melanurum visualized by conventional staining with Giemsa, C-banding, and double-FISH with18S rDNA (red) and 5S rDNA (green) probes. The NOR-bearing chromosomes are presented in the larger boxes, from the top to the bottom, as observed after silver staining, C-banding, and CMA3/DAPI staining. Scale bar = 5 μm.
In general, the species exhibited regions with reduced heterochromatin, which was mainly located in the centromeric position, especially in the NORs, which were the only GC-rich sites in the karyotypes (Figures 2, 3).
The Ag-NOR sites were located in a single chromosomal pair in all species. They were mainly positioned in the short arms of the 24th pair, except in case of the species Brachygenys chrysargyreum (5th pair, subterminal position of the long arm) and Haemulon melanurum (10th pair, pericentromeric position of the long arm) (Figures 3, 4).
Figure 4. Macrostructural aspects of karyotypes and the occurrence of rDNA sites in Western Atlantic haemulid species from the phylogenetic [adapted from Tavera et al. (2018)] and biogeographic perspectives. The distribution ranges of the species were estimated from the databases of the Ocean Biogeographic Information System – OBIS (https://obis.org). The references symbols refer to: ∗ – present study; [1] – Motta-Neto et al. (2011a); [2] – Nirchio et al. (2007); [3] Lima and Molina (2004); [4] – Nirchio and Oliveira (2014); [5] – Motta-Neto et al. (2011b).
Fluorescent in situ hybridization showed a complete congruence among the 18S rDNA and Ag-NOR sites. In most species, the 5S rDNA sites were present in the terminal position on the short arm of the 10th pair (Figures 2, 3), except in case of Anisotremus virginicus (5th pair, terminal region of the long arm), and Paranisotremus moricandi (24th pair, the whole short arm). H. melanurum exhibited an exclusive array of ribosomal sites; the 5S and 18S rDNA sites were co-located on the 10th pair of the chromosomes (Figure 3).
Population analyses of A. virginicus and B. chrysargyreum (Brazil/Caribbean) and Haemulon parra (oceanic islands and coastal areas of Brazil) did not reveal any structural chromosome divergences or changes in the organization of ribosomal genes.
The estimation of karyotype conservatism in members from the Eupercaria series based on the frequencies of 2n and NF values was based on a vast cytogenetic dataset encompassing 88% of the total clades in the Eupercaria series. Samples from the orders Perciformes, Labriformes, Centrarchiformes, Chaetodontiformes, and Spariformes, and the incertae sedis groups Lutjanidae, Haemulidae, and Sciaenidae, for whom more cytogenetic information was available, presented a high frequency of karyotypes with 2n = 48 (74–100%) (Figure 5).
Figure 5. Frequencies of 2n and NF (number of chromosome arms) in the representative groups of Eupercaria.
The most notable degree of diversification was observed in case of the well-sampled order Tetraodontiformes (71 spp.), in which karyotypes with 2n = 48 were observed in only 5.6% of the species, while 87.3% of the species showed karyotypes with 2n < 48, and only 4.3% of the species showed karyotypes with 2n = 48a (Supplementary Tables S1, S2).
The evolutionary conservatism of the karyotype structure was estimated based on the frequency of karyotypes with NF = 48. The groups with the greatest structural conservatism were the incertae sedis families Lutjanidae, Sciaenidae, and Haemulidae (100, 88, and 88% of the total spp., respectively) and the order Chaetodontiformes (75% of the total spp.) (Figure 5).
The cytogenetic data of the diverse order Perciformes mainly showed karyotypes with NF > 48 (74% of the spp.). However, this order also includes groups such as Serranidae, which had a high frequency of karyotypes with 2n = 48 acrocentric chromosomes (∼61% of spp.) (Supplementary Tables S1, S2).
The cytogenetic data set for members from the Haemulidae family reveals a remarkable karyotype conservatism. The karyotype stasis in the grunts was characterized by the extensive sharing of a standard karyotype composed of 2n = 48 acrocentric chromosomes, with a small variation in size between the largest and smallest chromosomes of the karyotype, distribution of heterochromatin in the centromeric regions of chromosomes, and distribution of Ag-NOR sites recurrently on the same chromosome pair.
In a broader context, stasis has been found to be maintained even in case of comparisons of diverse chromosome regions (Motta-Neto et al., 2012), at a wide phylogenetic spectrum, wide periods of divergence (>25 M.a), and varied biogeographic scales (Caribbean and western Atlantic).
Heterogeneous heterochromatins, when are present in variable amounts among chromosomes could suggest diverse evolutionary origins and dynamics in the karyotype complement. In contrast, the heterochromatin content in the haemulid species showed a regular and reduced distribution in the centromeric regions of the chromosomes, with only the NORs showing a high GC content.
Comparative analyses of replication bands in chromosomes from the haemulid species have shown regions of repetitive DNA with synchronous replication patterns, suggesting that it was likely that they shared functional and compositional similarities (Motta-Neto et al., 2012). In fact, cross-amplification tests for microsatellite sequences have shown a high level of success in Haemulon spp. (Quintero et al., 2018), providing support for the evolutionary conservation of repetitive DNA.
Analysis of repetitive DNA classes has served as a very useful approach to assess karyotype changes among groups of fishes. The physical mapping of rDNA in the chromosomes allows the identification of cryptic rearrangements in the chromosomes (e.g., Borges et al., 2019) and cytotaxonomic markers (Nakayama et al., 2012; Gornung, 2013; Almeida et al., 2017). However, the interspecific recurrence of rDNA distribution patterns on the chromosomes from the haemulid species restricts their use for this purpose. In fact, in this family, the Ag-NORs/18S rDNA sites are simple, and in most of the species, they were positioned in the short arm of the smallest chromosome pair (24th pair). In some species, they may also occur in other pairs of chromosomes (pair 10 in H. melanurum, pair 5 in B. chrysargyreum, and pair 18 in C. nobilis), but the repertoire of changes is quite restricted.
The 5S rDNA sites were shown to be more evolutionarily diverse among the haemulid species. They were localized in one or two chromosome pairs; however, they also exhibited a considerable regularity of number and position in homeologous chromosomes, usually in the pairs 5 (long arm) and 10 (short arm). Often, these sites were non-syntenic with 18S rDNA; however, co-localized 5S/18S rDNA arrays were found in H. melanurum (pair 10) and P. moricandi (pair 24) (Figure 4).
The mapping of the 5S rDNA sites revealed five basic patterns of the organization of rDNA regions in Haemulidae (Figure 4). The first, which was the most frequent (35% of spp.), consisted of karyotypes with a single site in the pericentromeric position of chromosomes in pair 10 and was present in Anisotremus surinamensis, Haemulon flavolineatum, H. parra, Haemulon squamipinna, B. chrysargyreum, and H. melanurum, as well as in species of more basal genera like Conodon nobilis. The second (21% of spp.) showed the presence of sites in the terminal arm of chromosomes in pair 5 and was present in Haemulon aurolineatum, Haemulon steindachneri, Haemulopsis corvinaeformis, and A. virginicus. The third consisted of sites on chromosomes in pairs 5 and 10 (28% of the spp.) and was present in Haemulon plumierii, Haemulon bonariense (Nirchio and Oliveira, 2014), possibly in H. aurolineatum (Nirchio et al., 2007), and in C. nobilis.
The fourth pattern involved the presence of a syntenic 5S/18S rDNA array in the chromosomes of pair 10 (7% of the species) and was exclusive to H. melanurum; the fifth pattern, which showed a 5S/18S rDNA array in the chromosomes of pair 24 (7% occurrence), was present in P. moricandi (Figure 4).
Chromosomal stasis mainly extends to the conservation of the order of genes (Ellegren, 2010). This condition in Haemulidae has been ascertained through similarities of the replication band patterns in the chromosomes (Motta-Neto et al., 2012). Additionally, the number and similarity of the localization of 5S and 18S rDNA sites have aided the identification of homeolog chromosomes, which have been widely identified in several fish groups (Vicente et al., 2001; Centofante et al., 2002; Costa et al., 2016).
Changes in the 5S rDNA sites in the grunt karyotypes were restricted to only two chromosome pairs (pairs 5 and 10). Similarly, 18S rDNA sites almost invariably occur in pair 24, which is the smallest chromosome pair, evidencing the occurrence of syntenic groups and the low evolutionary dynamics of these repetitive sequences. Thus, the phylogenetic diversification of rDNA sites mainly reveals “variations on the same theme,” indicating a slow and recurrent “birth-death” process of these sequences, with sporadic co-localized 5S/18S rDNA arrangements resulting from preferential transpositions between rDNA regions.
Environmental factors and the life history of the species likely contribute to the karyotype patterns of marine fish (Molina and Galetti, 2004; Sena and Molina, 2007).
The roles played by ecological or physical barriers on gene flow and the origin of genetic diversity are well known. The action of physical barriers is evidenced by greater karyotype diversity in freshwater fish species, while in case of marine species, a greater degree of conservation is normally observed (Brum and Galetti, 1997).
The species of Haemulidae have large populations distributed over wide geographic areas, where oceanographic variables (temperature, salinity, phytoplankton biomass, zooplankton biomass, and station depth) play an important role in the distribution patterns of ichthyoplankton (Souza and Mafalda-Junior, 2019).
The western Atlantic haemulid species have broad geographic distribution areas, which range from 800 to 7,000 km2 (Figure 4), depicting a geographical context very different from that normally found in case of freshwater fish species. This environmental scenario contributes sufficiently to gene flow, reducing the possibility of the formation of isolated groups, in which, by chance, chromosome rearrangements could be more frequently fixed. Analysis of the extensive geographic distribution, with a marked spatial overlap of sibling species, has revealed that besides vicariant events, ecological speciation also participates in the evolutionary diversification of this family of fishes (Rocha et al., 2008). In fact, in this family, recurrent sympatric speciation processes occurring in short periods of time (∼5 M.a), especially in species of the genus Haemulon, have been observed (Tavera et al., 2012; Tavera and Wainwright, 2019); recent events of genetic introgression have also been observed in some cases (Bernal et al., 2017).
In ecological speciation processes, with the fragmentation of ecological niches, in general, the split of the gene pool occurs without remarkable genetic changes or high selection pressures (Rocha and Bowen, 2008), thereby producing less propitious conditions to fix conspicuous chromosome rearrangements.
An extensive sharing of karyotypes in an evolutionary lineage could be caused from a recent diversification burst, during which genetic or chromosome changes have not yet been achieved. However, although some Haemulon species have been shown to demonstrate recent diversification (Rocha et al., 2008; Tavera et al., 2018), merely this condition seems insufficient to explain the karyotype stasis in members from this family. In fact, shared cytogenetic patterns are maintained among genera with long periods of divergence, which may reach up to 20 M.a (Tavera et al., 2018). Other factors, such as large populations, environmental continuities, and large distribution areas, seem to contribute to the panmixia of haemulid populations.
A test of the role of biogeographic barriers in the population structure of members from Haemulidae is offered by the plume of the Amazon and Orinoco rivers, which divides the Brazilian and Caribbean Provinces and plays an important role in the diversification of the faunas of these regions (Rocha, 2003; Bowen and Karl, 2008).
Cytogenetic analyses of populations of fishes from the genera Haemulon and Anisotremus located in the north and south of the Amazonas/Orinoco barrier have revealed a dichotomous pattern of karyotype divergence. Divergences of the rDNA sites were perceptible when the populations of H. aurolineatum from the Caribbean (Nirchio et al., 2007) and those from the Brazilian coast were compared (Motta-Neto et al., 2011b). On the other hand, populations of A. virginicus from the Caribbean and Brazilian coastal areas and those of B. chrysargyreum from the Fernando de Noronha Archipelago (Western Atlantic) region and the Caribbean did not present a detectable karyotype differentiation.
The interpopulational karyotype patterns underscore the chromosome conservatism in this group, even under distinct biogeographical pressures strengthened by action of the Amazonas/Orinoco barrier.
The dimension of karyotype stasis has been noted in several groups of Percomorpha (=Percomorphaceae) (Galetti et al., 2000; Molina, 2007), the most species-rich clade of modern fishes (Hughes et al., 2018). This clade has been reported to have a controversial taxonomic cohesion; however, the use of comparative genomics databases has promoted a remarkable phylogenetic redefinition, leading to the identification of its major lineages (Near et al., 2012; Betancur-R et al., 2017; Hughes et al., 2018). Its enormous diversity and increasingly robust phylogenetic relationships have made this group an excellent model to investigate karyotype evolution.
Among the 9 Percomorpha series, Eupercaria, the most diverse series, with more than 163 families and 6,000 species (sensu Hughes et al., 2018), encompasses orders with marked karyotype conservatism. In fact, members of the clade Percomorpha have been shown to present karyotypes with 2n = 48 acrocentric chromosomes both in basal and recent groups, clearly indicating that this pattern is symplesiomorphic (Galetti et al., 2000; Motta-Neto et al., 2012; Calado et al., 2014; Molina et al., 2014; Costa et al., 2016). However, thus far, its phylogenetic extent has only been inferred superficially.
Presently, the karyotype conservation estimated in members of the Eupercaria series, allowing a robust view of their karyotype evolution. In ten of the fifteen orders, most species show karyotypes of 2n = 48. Analysis of groups with greater diversity and cytogenetic information representativeness (Figure 5) revealed a high frequency of this diploid number (74–100%). Several groups, such as Chaetodontiformes (80% of spp.), Serranidae, which belongs to the large order Perciformes, or incertae sedis families such as Haemulidae, Sciaenidae, and Lutjanidae (86–100% of spp.), also presented high structural conservatism (NF = 48). The NF maintenance trend has been shown to continue when orders for which lower levels of cytogenetic information are available were considered (Gerreiformes, Ephippiformes, Lobotiformes).
Among the Eupercaria series groups, Labriformes represents one of the most morphologically and ecologically diversified clades in size, shape, and color (Westneat and Alfaro, 2005). Previous cytogenetic studies in this group have showed intermediate rates of chromosomal changes (Molina et al., 2014). Our data reinforce this condition, indicating considerable diversification in the NF (79% of species), despite a more conservative 2n variation (61% of species), with regards to a basal karyotype with 2n = 48a. On the other hand, the order Tetraodontiformes showed the most accentuated diversification of 2n (present only in 5.6% of species) and NF values (4.3% of the species), among the groups. This order, which comprises 438 spp. (Fricke et al., 2019), represents one of the main branches of the teleost diversification; its members present notable morphological and ecological characteristics, and variations in genome size (Brainerd et al., 2001; Santini and Tyler, 2003; Jaillon et al., 2004), which seem to have influenced their rather singular karyotype trends (Sá-Gabriel and Molina, 2005).
Evolutionary stasis is a broad process that involves the absence of conspicuous modifications in different dimensions throughout the evolutionary history of the organisms; its causes have not always been well understood. In some cases, this process may be transient, and has been shown to vary with regards to the occurrence of disruptive factors (Burt, 2001). In some cases, it has been shown to be characterized by the maintenance of a trait that has persisted for millions of years as a possible result of stabilizing selection (Wake et al., 1983). In adaptive terms, evolutionary stasis may reflect developmental homeostasis or static permanence at adaptive peaks (Lerner, 1954; Charlesworth et al., 1982; Kirkpatrick, 1982; Mayr, 1982; Templeton, 1982).
This process could still be related to parameters such as time, inertia, or selection. An explanation of temporal causes would involve the extensive sharing of a karyotype pattern in a group that recently underwent radiation and still did not have enough time to fix karyotype changes (Sola et al., 1981). Another possibility stems from the evolutionary inertia of karyotypes, caused by the absence of environmental destabilizing triggers (extrinsic to chromosomes) or cytogenetic factors (intrinsic to chromosomes). The extrinsic factors favoring gene flow include the absence of geographic (or ecological) barriers, dispersive capacity, population size, and the occurrence of historically stable environments. An additional possibility, but one that is less likely, is that stasis could be caused by the action of stabilizing selection, promoting active restriction to changes in chromosomes. Alternatively, it could be the product of orthoselective processes, where different karyotypes present the tendency to fix certain types of chromosome rearrangements along their evolutionary trajectories, promoting the formation of symmetrical karyotypes, with chromosomes showing approximately the same sizes and morphologies (White, 1973; King, 1981).
Using the available cytogenetic data set from the Eupercaria series, it was possible to analyze the validity of some explanations for the slow karyotype diversification. This clade encompasses evolutionary groups with extremely high diversity (e.g., with regards to natural history, ecological patterns, biogeography, habitat variety) (Nelson et al., 2016) and with very long divergence (70−38 M.a) (Yang et al., 2018); this makes it unlikely that karyotype stasis represents an adaptive condition stricto sensu or is a result of a short divergence time. On the other hand, given the evidence of extensive chromosome synteny, one cannot rule out the possibility that chromosome particularities of a group or related groups, such as low and homogeneous heterochromatin contents, contribute to the promotion and establishment of rearrangements in members from the Eupercaria series.
The vast majority of Eupercaria species are marine organisms (Betancur-R et al., 2017), which are present in large populations, and in general, have extensive distribution limits (Nelson et al., 2016). Under these conditions, a set of species of these groups show remarkable karyotype conservatism (Molina, 2007; Motta-Neto et al., 2011a, b, 2012; Molina et al., 2014) suggesting a role of the environment in the slow process of chromosome diversification.
These conditions presented in members from the Eupercaria series strengthen the hypothesis that environmental and intrinsic factors influencing the chromosomes of a group of fish can act synergistically, underscoring the chromosome conservatism in this group. The period of greatest divergence for members from the Eupercaria series (>70 M.a), which coincides with that for groups that share basal karyotypes, still clarifies that processes of karyotype stasis in fish can be maintained for extremely long periods of time.
Evolutionary karyotype patterns of large vertebrate groups are estimated based on the phylogenetic spectrum, temporal patterns, and cytogenetic approaches. Cytogenetic data from a representative number of haemulid species, which were used here as an evolutionary model, and the meta-analysis of the karyotype patterns of members of the Eupercaria contributed for a better understanding of the process of karyotype stasis in fishes. Haemulid karyotypes are remarkably conserved with regards to the macro- and microstructure of the chromosomes, suggesting the maintenance of extensive synteny throughout their evolutionary history. The ancient periods of evolutionary divergence among its lineages do not support the hypothesis that karyotype stasis is a byproduct of recent processes of speciation. On the other hand, these data suggest that the causes of this evolutionary process are complex, resulting from the synergistic action of the intrinsic characteristics of the chromosomes, together with their biological characteristics and the geographical context in which the species are inserted. The sharing of a generally homogeneous and reduced content of heterochromatin in members of the Haemulidae family seems to minimize the destabilizing role promoted by a high and heterogeneous content of repetitive DNAs and a repository of extensive sequence repertoires (e.g., transposable elements, microsatellites, multigene families) capable of generating evolutionary instability in the chromosomes. In addition, life history and biological characteristics, such as large populations, which are found in members in the Eupercaria series, could restrict the fixation of heterozygous variants. In general, cytogenetic analysis of the members from the Eupercaria revealed a broad scenario of karyotype stasis, except for the members from Tetraodontiformes, an order with diversified karyotype evolutionary trends. In summary, karyotype stasis is not an evolutionary process with a single cause, but is rather, a product of the conjunctural and synergistic effects of different factors. These data create a counterpoint for understanding the extreme diversification observed in other groups that do not meet the criteria for determining karyotype stasis.
All datasets generated for this study are included in the manuscript/Supplementary Files.
The animal study was reviewed and approved by the Committee of Ethics for the use of Animals of the Federal University of Rio Grande do Norte.
CM-N and WM contributed to the conception and design of the study. CM-N, GC, and KA performed the cytogenetic analyses. CM-N, KA, and WM organized the karyotype database. CM-N and WM wrote the first draft of the manuscript. MC, LB, and RA wrote the sections of the manuscript. All authors contributed to the manuscript revision, read, and approved the submitted version.
This work received national funds through the CNPq – Brazilian National Council for Scientific and Technological Development through project Proc. 442664/2015-0. This research was partly supported by the INCT “Marine Sciences” (565054/2010-4).
The authors declare that the research was conducted in the absence of any commercial or financial relationships that could be construed as a potential conflict of interest.
We thank ICMBio SISBIO for permits to collect specimens (licenses 19135-1, 27027-2, and 131360-1). We are also grateful to Jose Garcia Jr. for taxonomic identification of specimens.
The Supplementary Material for this article can be found online at: https://www.frontiersin.org/articles/10.3389/fmars.2019.00628/full#supplementary-material
Almeida, L. A. H., Nunes, L. A., Bitencourt, J. A., Molina, W. F., and Affonso, P. R. (2017). Chromosomal evolution and cytotaxonomy in wrasses (Perciformes; Labridae). J. Hered. 108, 239–253. doi: 10.1093/jhered/esx003
Aprea, G., Andreone, F., Capriglione, T., Odierna, G., and Vences, M. (2004). Evidence for a remarkable stasis of chromosome evolution in Malagasy treefrogs (Boophis, Mantellidae). Ital. J. Zool. 71, 237–244. doi: 10.1080/11250000409356641
Bernal, M., Gaither, M., Simison, W., and Rocha, L. (2017). Introgression and selection shaped the evolutionary history of sympatric sister-species of coral reef fishes (genus: Haemulon). Mol. Ecol. 26, 639–652. doi: 10.1111/mec.13937
Bernal, M. A., Dixon, G. B., Matz, M. V., and Rocha, L. A. (2019). Comparative transcriptomics of sympatric species of coral reef fishes (genus: Haemulon). PeerJ 1:e6541. doi: 10.7717/peerj.6541
Betancur-R, R., Broughton, R. E., Wiley, E. O., Carpenter, K., López, J. A., Li, C., et al. (2013). The tree of life and a new classification of bony fishes. PLoS Curr. 5:ecurrents.tol.53ba26640df0ccaee75bb165c8c26288. doi: 10.1371/currents.tol.53ba26640df0ccaee75bb165c8c26288
Betancur-R, R., Wiley, E. O., Arratia, G., Acero, A., Bailly, N., Miya, M., et al. (2017). Phylogenetic classification of bony fishes. BMC Evol. Biol. 17:162. doi: 10.1186/s12862-017-0958-3
Bomfleur, B., McLoughlin, S., and Vajda, V. (2014). Fossilized nuclei and chromosomes reveal 180 million years of genomic stasis in royal ferns. Science 343, 1376–1377. doi: 10.1126/science.1249884
Borges, A. T., Cioffi, M. B., Bertollo, L. A. C., Soares, R. X., Costa, G. W. W. F., and Molina, W. F. (2019). Paracentric inversions differentiate the conservative karyotypes in two Centropomus species (Teleostei: Centropomidae). Cytogenet. Genome Res. 157, 239–248. doi: 10.1159/000499748
Bowen, B. W., and Karl, S. A. (2008). Population genetics and phylogeography of sea turtles. Mol. Ecol. 16, 4886–4907. doi: 10.1111/j.1365-294x.2007.03542.x
Brainerd, E. L., Slutz, S. S., Hall, E. H., and Phillis, R. W. (2001). Patterns of genome size evolution in Tetraodontiform fishes. Evolution 55, 2363–2368. doi: 10.1111/j.0014-3820.2001.tb00750.x
Brum, M. J. I., and Galetti, P. M. Jr. (1997). Teleostei ground plan karyotype. J. Comp. Biol. 2, 91–102.
Burt, D. B. (2001). Evolutionary stasis, constraint and other terminology describing evolutionary patterns. Biol. J. Linn. Soc. 72, 509–517. doi: 10.1111/j.1095-8312.2001.tb01334.x
Calado, L. L., Bertollo, L. A. C., Cioffi, M. B., Costa, G. W. W. F., Jacobina, U. P., and Molina, W. F. (2014). Evolutionary dynamics of rDNA genes on chromosomes of the Eucinostomus fishes: cytotaxonomic and karyoevolutive implications. Genet. Mol. Res. 13, 9951–9959. doi: 10.4238/2014.November.27.24
Centofante, L., Bertollo, L. A. C., and Moreira-Filho, O. (2002). A ZZ/ZW sex chromosome system in a new species of the genus Parodon (Pisces, Parodontidae). Caryologia 55, 139–150. doi: 10.1080/00087114.2002.10589270
Charlesworth, B., Lande, R., and Slatkin, M. (1982). A neo-darwinian commentary on macroevolution. Evolution 36, 474–498. doi: 10.1111/j.1558-5646.1982.tb05068.x
Costa, G. W. W. F., Cioffi, M. B., Bertollo, L. A. C., and Molina, W. F. (2016). The evolutionary dynamics of ribosomal genes, histone H3, and transposable Rex elements in the genome of Atlantic snappers. J. Hered. 107, 173–180. doi: 10.1093/jhered/esv136
Ellegren, H. (2010). Evolutionary stasis: the stable chromosomes of birds. Trends Ecol. Evol. 25, 283–291. doi: 10.1016/j.tree.2009.12.004
Fricke, R., Eschmeyer, W. N., and Fong, J. D. (2019). Eschmeyer’s Catalog of Fishes: Species by Family/Subfamily. http://researcharchive.calacademy.org/research/ichthyology/catalog/SpeciesByFamily.asp (accessed on May 16 2019).
Galetti, P. M. Jr., Aguilar, C. T., and Molina, W. F. (2000). An overview on marine fish cytogenetics. Hydrobiologia 420, 55–62. doi: 10.1023/A:1003977418900
Gold, J. R., Li, C., Shipley, N. S., and Powers, P. K. (1990). Improved methods for working with fish chromosomes with a review of metaphase chromosome banding. J. Fish Biol. 37, 563–575. doi: 10.1111/j.1095-8649.1990.tb05889.x
Gornung, E. (2013). Twenty years of physical mapping of major ribosomal RNA genes across the Teleosts: a review of research. Cytogenet. Genome Res. 141, 90–102. doi: 10.1159/000354832
Howell, W. M., and Black, D. A. (1980). Controlled silver staining of nucleolus organizer region with protective colloidal developer: a 1st-step method. Experientia 36, 1014–1015. doi: 10.1007/bf01953855
Hughes, L. C., Ortí, G., Huang, Y., Sun, Y., Baldwin, C. C., Thompson, A. W., et al. (2018). Comprehensive phylogeny of ray-finned fishes (Actinopterygii) based on transcriptomic and genomic data. Proc. Natl. Acad. Sci. U.S.A. 115, 6249–6254. doi: 10.1073/pnas.1719358115
Jaillon, O., Aury, J. M., Brunet, F., Petit, J. L., Stange-Thomann, N., Mauceli, E., et al. (2004). Genome duplication in the teleost fish Tetraodon nigroviridis reveals the early vertebrate proto-karyotype. Nature 431, 946–957. doi: 10.1038/nature03025
Kahl, G. (2015). The Dictionary of Genomics, Transcriptomics and Proteomics. Verlag: John Wiley & Sons.
King, M. (1981). “Chromosome change and speciation in lizards,” in Evolution and Speciation. Essays in Honor of M. J. D. White, eds W. R. Atchley, and D. Woodruff, (London: Cambridge University Press), 262–285.
Kirkpatrick, M. (1982). Sexual selection and the evolution of the female choice. Evolution 36, 1–12. doi: 10.1111/j.1558-5646.1982.tb05003.x
Levan, A., Fredga, K., and Sandberg, A. (1964). Nomenclature for centromeric position on chromosomes. Hereditas 52, 201–220. doi: 10.1111/j.1601-5223.1964.tb01953.x
Lima, L. C. B., and Molina, W. F. (2004). Homeostase cariotípica em três espécies do gênero Haemulon (Haemulidae, Perciformes) do litoral Potiguar. Anais X Simpósio de Citogenética e Genética de Peixes. Universidade Federal do Rio Grande do Norte Natal Brasil 1:113.
Mandáková, T., Heenan, P. B., and Lysak, M. A. (2010). Island species radiation and karyotypic stasis in Pachycladon allopolyploids. BMC Evol. Biol. 10:367. doi: 10.1186/1471-2148-10-367
Molina, W. F. (2007). “Chromosomal changes and stasis in marine fish groups,” in Fish Cytogenetics, eds E. Pisano, C. Ozouf-Costaz, F. Foresti, and B. G. Kapoor, (Enfield: Science Publishers), 69–110.
Molina, W. F., Alves, D. E. O., Arauìjo, W. C., Martinez, P. A., Silva, M. F. M., and Costa, G. W. W. F. (2010). Performance of human immunostimulating agents in the improvement of fish cytogenetics. Genet. Mol. Res. 9, 1807–1810. doi: 10.4238/vol9-3gmr840
Molina, W. F., Costa, G. W. W. F., Soares, R. X., and Affonso, P. R. A. M. (2013). Extensive chromosome conservatism in Atlantic butterflyfishes, genus Chaetodon Linnaeus, 1758: implications for the high hybridization success. Zool. Anz. 253, 137–142. doi: 10.1016/j.jcz.2013.10.001
Molina, W. F., and Galetti, P. M. Jr. (2004). Karyotypic changes associated to the dispersive potential on Pomacentridae (Pisces, Perciformes). J. Exp. Mar. Biol. Ecol. 309, 109–119. doi: 10.1016/j.jembe.2004.03.011
Molina, W. F., Martinez, P. A., Bertollo, L. A. C., and Bidau, C. J. (2014). Preferential accumulation of sex and Bs chromosomes in biarmed karyotypes by meiotic drive and rates of chromosomal changes in fishes. An. Acad. Bras. Ciênc. 86, 1801–1812. doi: 10.1590/0001-3765201420130489
Motta-Neto, C. C., Cioffi, M. B., Bertollo, L. A. C., and Molina, W. F. (2011a). Extensive chromosomal homologies and evidence of karyotypic stasis in Atlantic grunts of the genus Haemulon (Perciformes). J. Exp. Mar. Biol. Ecol. 401, 75–79. doi: 10.1016/j.jembe.2011.02.044
Motta-Neto, C. C., Cioffi, M. B., Bertollo, L. A. C., and Molina, W. F. (2011b). Molecular cytogenetic analysis of Haemulidae fish (Perciformes): evidence of evolutionary conservation. J. Exp. Mar. Biol. Ecol. 407, 97–100. doi: 10.1016/j.jembe.2011.07.014
Motta-Neto, C. C., Lima-Filho, P. A., Araújo, W. C., Bertollo, L. A. C., and Molina, W. F. (2012). Differentiated evolutionary pathways in Haemulidae (Perciformes): karyotype stasis versus morphological differentiation. Rev. Fish Biol. Fish. 22, 457–465. doi: 10.1007/s11160-011-9236-4
Nakayama, C. M., Feldberg, E., and Bertollo, L. A. C. (2012). Karyotype differentiation and cytotaxonomic considerations in species of Serrasalmidae (Characiformes) from the Amazon basin. Neotrop. Ichthyol. 10, 53–58. doi: 10.1590/S1679-62252012000100005
Near, T. J., Eytan, R. I., Dornburg, A., Kuhn, K. L., Moore, J. A., Davis, M. P., et al. (2012). Resolution of ray-finned fish phylogeny and timing of diversification. Proc. Natl. Acad. Sci. U.S.A. 109, 13698–13703. doi: 10.1073/pnas.1206625109
Nelson, J. S., Grande, T. C., and Wilson, M. V. H. (2016). Fishes of the World. New York: John Wiley & Sons Inc.
Nirchio, M., Gaviria, J. I., Oliveira, C., Ferreira, I. A., and Martins, C. (2007). Cytogenetic analysis of three species of the genus Haemulon (Teleostei: Haemulinae) from Margarita Island, Venezuela. Genetica 131, 135–140. doi: 10.1007/s10709-006-9123-4
Nirchio, M., and Oliveira, C. (2014). Cytogenetics as a taxonomic tool in fish. Artículo de Revisión Agrobiología 26:11.
Pendás, A. M., Morán, P., and García-Vázquez, E. (1994). Organization and chromosomal location of the major histone cluster in brown trout, Atlantic salmon and rainbow trout. Chromosoma 103, 147–152. doi: 10.1007/BF00352324
Pinkel, D., Straume, T., and Gray, J. W. (1986). Cytogenetic analysis using quantitative, high sensitivity, fluorescence hybridization. Proc. Natl. Acad. Sci. U.S.A. 83, 2934–2938. doi: 10.1073/pnas.83.9.2934
Quintero, P. E. P., Tavera, J. J., Millán-Márquez, A. M., and Acero, P. A. (2018). Amplification of microsatellites in two haemulid species (Haemulon aurolineatum and Haemulon steindachneri). Bol. Invest. Mar. Cost 47, 151–157. doi: 10.25268/bimc.invemar.2018.47.1.743
Rocha, L., Lindeman, K., Rocha, C., and Lessios, H. A. (2008). Historical biogeography and speciation in the reef fish genus Haemulon (Teleostei: Haemulidae). Mol. Phylogenet. Evol. 48, 918–928. doi: 10.1016/j.ympev.2008.05.024
Rocha, L. A. (2003). Patterns of distribution and processes of speciation in Brazilian reef fishes. J. Biogeogr. 30, 1161–1171. doi: 10.1046/j.1365-2699.2003.00900.x
Rocha, L. A., and Bowen, B. W. (2008). Speciation in coral reef fishes. J. Fish Biol. 72, 1101–1121. doi: 10.1111/j.1095-8649.2007.01770.x
Sá-Gabriel, L. G., and Molina, W. F. (2005). Karyotype diversification in fishes of theBalistidae, Diodontidae and Tetraodontidae (Tetraodontiformes). Caryologia 58, 229–237. doi: 10.1080/00087114.2005.10589455
Samad, N. A., Dagher-Kharrat, M. B., Hidalgo, O., El Zein, R., Douaihy, B., and Siljak-Yakovlev, S. (2016). Unlocking the karyological and cytogenetic diversity of iris from lebanon: Oncocyclus section shows a distinctive profile and relative stasis during its continental radiation. PLoS One 11:e0160816. doi: 10.1371/journal.pone.0160816
Santini, F., and Tyler, J. C. (2003). A phylogeny of the families of fossil and extant Tetraodontiform fishes (Acanthomorpha, Tetraodontiformes), upper cretaceous to recent. Zool. J. Linnean Soc. 139, 565–617. doi: 10.1111/j.1096-3642.2003.00088.x
Schweizer, D. (1980). Simultaneous fluorescent staining of R bands and specific heterochromatic regions (DA-DAPI bands) in human chromosomes. Cytogenet. Cell Genet. 27, 190–193. doi: 10.1159/000131482
Sena, D. C. S., and Molina, W. F. (2007). Chromosomal rearrangements associated with pelagic larval duration in Labridae (Perciformes). J. Exp. Mar. Biol. Ecol. 353, 203–210. doi: 10.1016/j.jembe.2007.08.020
Sessions, S. K., and Kezer, J. (1991). “Evolutionary cytogenetics of Bolitoglossine salamanders (family Plethodontidae),” in Amphibian Cytogenetics and Evolution, eds D. M. Green, and S. K. Sessions, (San Diego: Academic Press), 89–130. doi: 10.1016/B978-0-12-297880-7.50009-3
Sola, L., Cataudella, S., and Capanna, E. (1981). New developments in vertebrate cytotaxonomy III. Karyology of bony fishes: a review. Genetica 54, 285–328. doi: 10.1007/BF00135048
Souza, C. S., and Mafalda-Junior, P. O. (2019). Large-scale spatial and temporal variability of larval fish assemblages in the tropical Atlantic Ocean. Acad. Bras. Ciênc. 91:e20170567. doi: 10.1590/0001-3765201820170567
Sumner, A. T. (1972). A simple technique for demonstrating centromeric heterochromatin. Exp. Cell Res. 75, 304–306. doi: 10.1016/0014-4827(72)90558-7
Tavera, J., Acero, P. A., and Wainwright, P. C. (2018). Multilocus phylogeny, divergence times, and a major role for the benthic-topelagic axis in the diversification of grunts (Haemulidae). Mol. Phylogenet. Evol. 121, 212–223. doi: 10.1016/j.ympev.2017.12.032
Tavera, J. J., and Wainwright, P. C. (2019). Geography of speciation affects rate of trait divergence in haemulid fishes. Proc. R. Soc. Lond. 286:20182852. doi: 10.1098/rspb.2018.2852
Tavera, J. T., Acero, A. P., Balart, E. F., and Bernardi, G. (2012). Molecular phylogeny of grunts (Teleostei, Haemulidae), with an emphasis on the ecology, evolution, and speciation history of new world species. BMC Evol. Biol. 12:57. doi: 10.1186/1471-2148-12-57
Templeton, A. R. (1982). “Adaption and the integration of evolutionary forces,” in Perspectives on Evolution, ed. R. Milkman, (Sunderland,MA: Sinauer Associates), 15–31.
Vicente, V. E., Jesus, C. M., and Moreira-Filho, O. (2001). Chromosomal localization of 5S and 18S rRNA genes in three Parodon species (Pisces, Parodontidae). Caryologia 54, 365–369. doi: 10.1080/00087114.2001.10589247
Wake, D. B., Roth, G., and Wake, M. H. (1983). On the problem of stasis in organismal evolution. J. Theor. Biol. 101, 211–224. doi: 10.1016/0022-5193(83)90335-1
Westneat, M. W., and Alfaro, M. E. (2005). Phylogenetic relationships and evolutionary history of the reef fish family Labridae. Mol. Phylogenet. Evol. 36, 370–390. doi: 10.1016/j.ympev.2005.02.001
White, T. J., Bruns, T., Lee, S., and Taylor, J. (1990). “Amplification and direct sequencing of fungal ribosomal RNA genes for phylogenetics,” in PCR Protocols: A Guide to Methods and Applications, eds M. A. Innis, D. H. Gelfand, J. J. Shinsky, and T. J. White, (San Diego: Academic Press Inc), 315–322. doi: 10.1016/b978-0-12-372180-8.50042-1
Keywords: grunts, karyotype evolution, chromosomal divergence, syntenic regions, rDNA
Citation: Motta-Neto CCd, Cioffi MdB, Costa GWWFd, Amorim KDJ, Bertollo LAC, Artoni RF and Molina WF (2019) Overview on Karyotype Stasis in Atlantic Grunts (Eupercaria, Haemulidae) and the Evolutionary Extensions for Other Marine Fish Groups. Front. Mar. Sci. 6:628. doi: 10.3389/fmars.2019.00628
Received: 25 June 2019; Accepted: 24 September 2019;
Published: 11 October 2019.
Edited by:
Dongdong Xu, Marine Fisheries Research Institute of Zhejiang, ChinaReviewed by:
Jose Julian Tavera, Universidad del Valle, ColombiaCopyright © 2019 Motta-Neto, Cioffi, Costa, Amorim, Bertollo, Artoni and Molina. This is an open-access article distributed under the terms of the Creative Commons Attribution License (CC BY). The use, distribution or reproduction in other forums is permitted, provided the original author(s) and the copyright owner(s) are credited and that the original publication in this journal is cited, in accordance with accepted academic practice. No use, distribution or reproduction is permitted which does not comply with these terms.
*Correspondence: Clóvis Coutinho da Motta-Neto, bW90dGFuZXRvLmNjQGdtYWlsLmNvbQ==
Disclaimer: All claims expressed in this article are solely those of the authors and do not necessarily represent those of their affiliated organizations, or those of the publisher, the editors and the reviewers. Any product that may be evaluated in this article or claim that may be made by its manufacturer is not guaranteed or endorsed by the publisher.
Research integrity at Frontiers
Learn more about the work of our research integrity team to safeguard the quality of each article we publish.