- 1Department of Biology and Chemistry, College of Liberal Arts and Sciences, National University of Defense Technology, Changsha, China
- 2Department of Physics, North Carolina State University, Raleigh, NC, United States
- 3Duke University Marine Laboratory, Beaufort, NC, United States
Barnacles are notorious marine fouling organisms, whose life cycle initiates with the planktonic larva, followed by the free-swimming cyprid that voluntarily explores, and searches for an appropriate site to settle and metamorphoses into a sessile adult. Within this life cycle, both the cyprid and the adult barnacle deposit multi-protein adhesives for temporary or permanent underwater adhesion. Here, we present a comprehensive review of the biochemistries behind these different adhesion events in the life cycle of a barnacle. First, we introduce the multiple adhesion events and their corresponding adhesives from two complementary aspects: the in vivo synthesis, storage, and secretion as well as the in vitro morphology and biochemistry. The amino acid compositions, sequences, and structures of adult barnacle adhesive proteins are specifically highlighted. Second, we discuss the molecular mechanisms of adult barnacle underwater attachment in detail by analyzing the possible adhesive and cohesive roles of different adhesive proteins, and based on these analyses, we propose an update to the original barnacle underwater adhesion molecular model. We believe that this review can greatly promote the general understanding of the molecular mechanisms underlying the reversible and irreversible underwater adhesion of barnacles and their larvae. Such an understanding is the basis for the prevention of barnacle fouling on target surfaces as well as designing conceptually new barnacle-inspired artificial underwater adhesives.
Introduction
Many marine creatures inhabiting the wave-swept seashores, such as mussels (Waite, 2017), tubeworms (Stewart et al., 2011b), and barnacles (Kamino, 2006), have evolved the capability of synthesizing, secreting, and curing biological adhesives for temporary or permanent underwater adhesion. These organisms are fascinating, primarily because their adhesives are able to stick a wide range of materials together quickly and firmly in water, whereas, almost all currently available chemical adhesives fail to do so. Conversely, being major fouling organisms, these hard-shell marine animals are frustrating as their adhesion on manmade architectures often causes a severe marine biofouling issue, which refers to the accumulation of marine microbes, plants, and animals on target structures leading to millions of dollars spent annually on antifouling (Callow and Callow, 2002; Schultz et al., 2011). Therefore, understanding their underwater adhesion mechanisms is beneficial for both the design of biomimetic adhesives that can function in aqueous environments (Lee et al., 2011; Zhong et al., 2014; Zhao et al., 2016) and the development of new antifouling technologies (Del Grosso et al., 2016; Amini et al., 2017).
Owing to their wide distribution in the world’s oceans, and robust, gregarious underwater attachment, barnacles are among the most dominant marine fouling organisms. They rely on a multi-protein holdfast with unique chemistry and morphology for surface colonization (Kamino, 2010b). Consequently, barnacles have served as model species for both marine antifouling tests (Holm, 2012) and developing novel bio-inspired wet adhesives (Kamino, 2008). Barnacles belong to the phylum Arthropoda, differing greatly from intensively studied mussels (Mollusca), and tubeworms (Annelida). They can be simply categorized into parasitic and non-parasitic groups; the latter includes the two most studied clades in underwater adhesion research, acorn and stalked barnacles, i.e., orders Sessilia and Pedunculata, respectively (Perez-losada et al., 2009; Power et al., 2010).
The life cycle of barnacles consists of four stages: nauplius, cyprid, juvenile, and adult (Figure 1). After six phases (I–VI), the planktonic nauplius transforms into a pre-settling cyprid, which then actively explores external substrates in search of suitable sites and settles down to metamorphose and develop into benthic juvenile and adult. Thereafter, the adult barnacle is permanently anchored to the substrate, even after its death (Anderson, 1994; Khandeparker and Anil, 2007; Aldred and Clare, 2008). Within the entire life cycle, three different adhesion events, either reversible or irreversible, are involved (Figure 1). First, the cyprid releases a temporary adhesive (footprint) for reversible adhesion during surface exploration. Second, the cyprid produces a permanent adhesive (cyprid cement) for colonization on a suitable site. Third, the adult barnacle secretes barnacle cement to maintain firm attachment. Consequently, the underwater adhesion studies of barnacles usually differentiate into independent studies focusing on one of the three adhesion events and the corresponding adhesive.
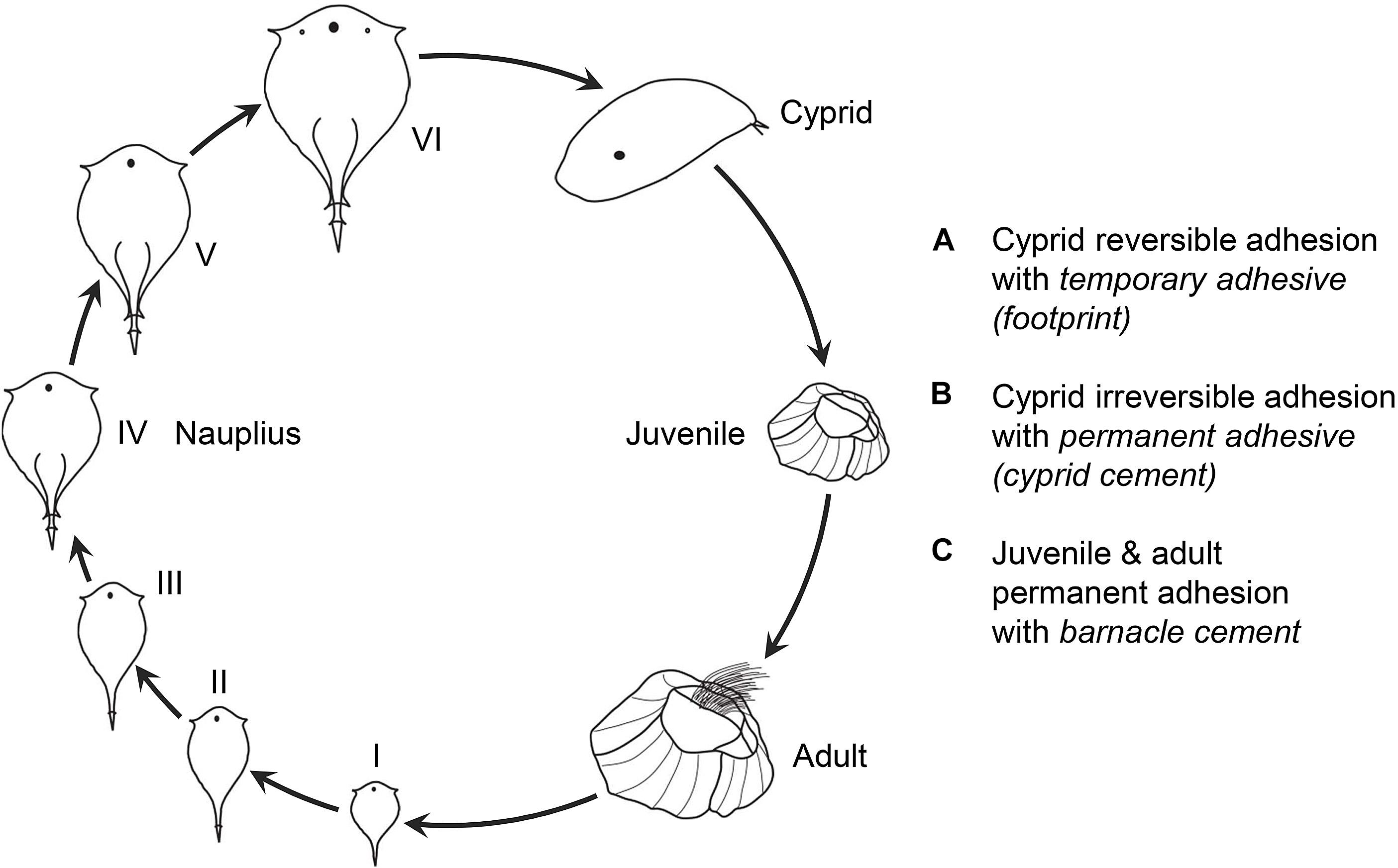
Figure 1. Schematic of the life cycle of a barnacle. The life cycle of a barnacle consists of four stages: nauplius (I–VI), cyprid, juvenile, and adult. Within this life cycle, there are three reversible or irreversible adhesion events as summarized in (A–C). The figure was modified from Khandeparker and Anil (2007). Copyright (2007) Elsevier.
Although research on barnacle underwater adhesion has accelerated in the past decade, likely driven by the urgent need for novel, environmentally benign antifouling technologies (Yebra et al., 2004; Callow and Callow, 2011) and introduction of advanced analytical tools (Hennebert et al., 2015; Aldred and Petrone, 2016), our understanding of the mechanism behind these processes remains incomplete. In marine environments, any submerged objects will be covered instantly by interfacial water layers and later by biofilms comprising microbes and their extracellular secretions (Yebra et al., 2004). These boundary layers pose a big challenge for adhesive bonding as they prevent adhesives from wetting and binding with underlying substrates and greatly compromise the interfacial adhesion strength (Waite, 1987; Lee et al., 2011). In addition, the surrounding bulk seawater, oxidative species, and microbial communities, may also diminish underwater adhesion by diluting, oxidizing, and degrading biological holdfasts. What adaptations have barnacles made to overcome these challenges and achieve tenacious underwater attachment? The question largely remains open.
To update and improve our understanding of the mechanisms behind these reversible and irreversible adhesion events of barnacles and their larvae, a comprehensive review is necessary given the many recent advances in this field. Therefore, this review aims to summarize the past and present studies of barnacle underwater adhesion. Firstly, these three different adhesion events will be sequentially introduced. Their corresponding adhesives will be emphasized from two complementary aspects: the in vivo synthesis, storage, and secretion as well as the in vitro morphology and biochemistry. Subsequently, the adult barnacle underwater attachment mechanism will be elaborated by analyzing the possible adhesive and cohesive interactions of different cement proteins, which have not been discussed in previous reviews (Kamino, 2006, 2013; Power et al., 2010; Zhang et al., 2017).
Surface Exploration: Cyprid Temporary Adhesion
Surface Exploration: Why and How?
Underwater adhesion of the cyprid contains two successive stages, temporary surface exploration, and permanent surface settlement. Prior to permanent settlement, the cyprid must explore the substrate to decide whether to colonize on it; the cyprid settles permanently if the substrate is satisfactory, otherwise it returns to the water column (Aldred and Clare, 2008; Rosenhahn and Sendra, 2012). It was found that cyprids prefer to settle on substrates where the temporary adhesion is strong, and thus it requires a larger force to detach them from these preferable substrates (Aldred et al., 2010). Moreover, cyprids have a strong tendency to settle on surfaces already fouled by adult barnacles, especially conspecific ones, because the benthic life style, and cross fertilization of adult barnacles require that cyprids must settle near conspecific barnacles (Knight-Jones and Crisp, 1953).
The cyprid uses its attachment organ – the two attachment discs (Figure 2A) on the third segment of the paired antennules – to adhere to the substrate (Nott, 1969; Nott and Foster, 1969). Cyprid attachment discs are flat and covered by a carpet of cuticular villi (Figures 2B,C). Among species, they vary markedly in outlines (e.g., circular or elliptical), perimeter structures (e.g., velum or skirt), tilting angles relative to the long axis of the antennule, and microvilli density (Bielecki et al., 2009; Brickner and Hoeg, 2010; Al-Yahya et al., 2016; Yorisue et al., 2016). During surface exploration, the two attachment discs attach and detach from the surface alternatively, allowing the cyprid to “walk” bipedally on the surface. Simultaneously, the cyprid is capable of precisely sensing the biochemical, physicochemical, and topological characteristics of the substrate using an array of antennular setae and chooses to either settle or leave. Consequently, all these factors influence the surface choice of settling cyprids. For instance, simple cyprid settlement assays have confirmed that adult barnacle pheromones present on the substrate can greatly promote surface colonization of conspecific cyprids, even when the clear substratum is not satisfactory (Crisp and Meadows, 1962; Larman and Gabbott, 1975; Matsumura et al., 1998; Dreanno et al., 2006b). Many surface topological and physicochemical properties, e.g., surface textures (Berntsson et al., 2000; Aldred et al., 2010; Chaw et al., 2011), hydrophobicity (Chaw and Birch, 2009; Phang et al., 2009; Guo et al., 2014), surface energy, and charge (Petrone et al., 2011; Di Fino et al., 2014) were also found to influence cyprid surface exploration. Using more complex video-/tracking-based approaches, the dynamic cyprid surface exploration process under different conditions was also monitored, offering a real-time and quantitative method of understanding the settling behaviors of a cyprid by measuring its swimming velocity, step length and duration, body movements, footprint deposition, and so on (Marechal et al., 2004; Andersson et al., 2009; Chaw and Birch, 2009; Maleschlijski et al., 2012; Aldred et al., 2013b, 2018; Maleshlijski et al., 2016).
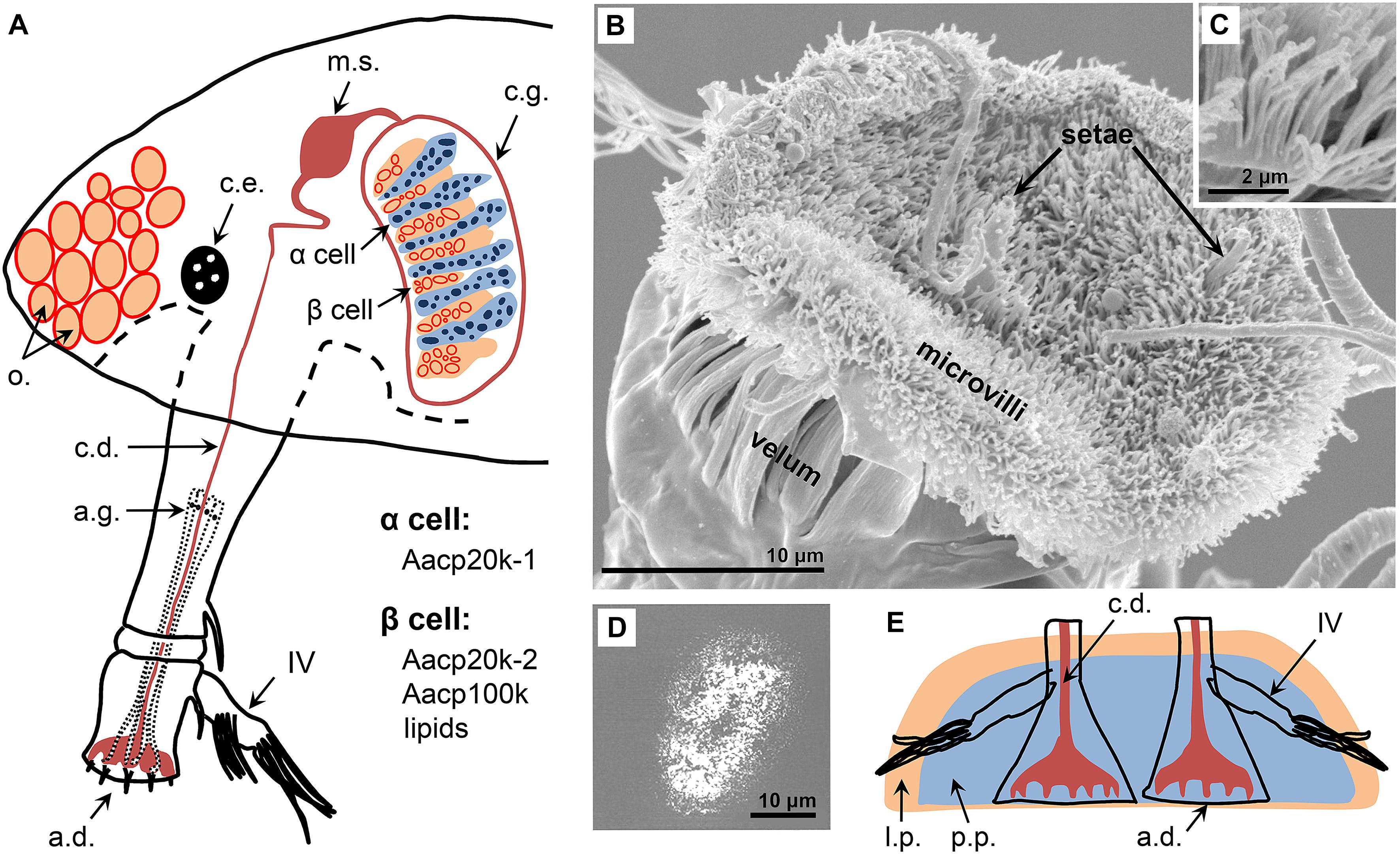
Figure 2. Cyprid underwater adhesion. (A) Schematic of cyprid temporary and permanent attachment organs. The cyprid uses unicellular antennal glands to synthesize the temporary adhesive for surface exploration and kidney-shaped cyprid glands to produce cyprid cement for permanent settlement. The cyprid gland consists of the α and β cells. In the Amphibalanus amphitrite cyprid, Aacp20k-1 is detected in the α cells while Aacp20k-2, Aacp100k, and lipids are present in the β cells. (B,C) Scanning electron microscopy photographs showing the Semibalanus balanoides cyprid attachment disc and the covered microvilli. (D) An atomic force microscopy image showing the remnant elliptical footprint of the A. amphitrite cyprid on the substrate. (E) Schematic of the cyprid permanent cement plaque. a.d., attachment disc; a.g., antennal gland; c.d., cement duct; c.e., compound eye; c.g., cyprid gland; m.s., muscular sac; o., oil; l.p., lipid phase; p.p., protein phase. (A,E) Modified from Walker (1981). Copyright (1981) Gordon and Breach Publishers, Inc. (B,C) Modified from Aldred et al. (2013b) under the Creative Commons CC-BY license. (D) Modified from Guo et al. (2014). Copyright (2014) American Chemical Society.
Cyprid Temporary Adhesion
Cyprid temporary adhesion exhibits three primary properties: tenacity, speed, and reversibility. Regarding tenacity, cyprid adhesion strength varies between approximately 100–300 kPa on different substrates as measured by a microbalance (Yule and Crisp, 1983; Yule and Walker, 1984; Maki et al., 1994). In terms of speed, it is estimated to take only several seconds to form a secure bond between cyprid adhesive disks and a substrate by measuring the time gap between two adjacent paces (Chaw and Birch, 2009). As for reversibility, a cyprid can not only walk on the substrate by alternatively attaching each of its two adhesive disks, but also leave the substrate entirely by detaching both disks. So, how does the cyprid achieve such a tenacious, rapid, and reversible adhesion process employing a pair of attachment discs?
As stated earlier, scanning electron microscopy (SEM) observations revealed that cyprid attachment discs are covered by microvilli and setae. This mat of microvilli and setae are not beneficial to create the temporary seal between attachment discs and the substrate. Moreover, cyprids do not have the antennal musculature required for creating a closed low-pressure environment. These observations collectively deny the assumption that cyprids use the attachment discs as “suction cups” to adhere to surfaces. Currently, it is widely accepted that cyprids secrete bioadhesives to assist in temporary adhesion. In acorn barnacle cyprids, the temporary adhesive was thought to be synthesized in unicellular antennal glands (Figure 2A) located in the second segment of the antennules (Nott, 1969; Nott and Foster, 1969). In the cyprid of the stalked barnacle, Octolasmis angulata, this adhesive is produced in the unicellular glands clustered in the main body (Yap et al., 2017). Synthesized temporary adhesives are then delivered to the attachment discs through the ducts in the antennules. After surface exploration, the residual footprints on the substrate are believed to be cyprid temporary adhesives which were first detected by Walker and Yule using a protein dye (Walker and Yule, 1984). Over two decades later, cyprid footprints deposited on various substrates were directly observed with atomic force microscopy (AFM) (Figure 2D; Phang et al., 2008, 2009, 2010; Guo et al., 2014) and imaging surface plasmon resonance (Andersson et al., 2009), thus adding conclusive evidence to the idea that cyprids secrete adhesives for temporary adhesion. By using AFM-based force spectroscopy, Phang et al. (2008) detected the adhesion strength of cyprid footprints, which was only about 1/3 that of the measured adhesion strength of the cyprid. Accordingly, they speculated that the microvilli on cyprid attachment discs might play a similar physical adhesion role to that of fly pulvilli and gecko spatula to promote temporary cyprid adhesion when boundary water was displaced by temporary adhesives (Phang et al., 2008); but, this requires further confirmation. By employing high-speed photography, Aldred et al. (2013b) discovered that cyprid temporary adhesion was also regulated by its behaviors. For example, during rapid walking, cyprid adhesion is compromised by minimizing contact time and contact area of the attachment discs, while, during careful inspection, cyprid adhesion is enhanced by behaviorally pushing the attachment discs toward the substrate. To achieve reversible adhesion, the cyprid tugs the antennules parallel to the surface and peels the attachment discs from the substrate. To summarize, cyprid temporary adhesion is biochemically, physically, and behaviorally co-mediated.
Settlement-Inducing Protein Complex (SIPC)
The cyprid temporary adhesive mainly comprises proteins as the residual footprints on substrates can be stained by protein dyes (Walker and Yule, 1984; Clare et al., 1994; Matsumura et al., 1998). Guo et al. (2014) discovered that both the size and adhesion strength of cyprid footprints increase on more hydrophobic substrates, probably implying that cyprid footprints are abundant in hydrophobic proteins and they experience conformational changes leading to exposure of hydrophobic domains. Cyprid footprints are also rich in basic proteins that have an average isoelectric point (pI) of 9.6–9.7 (Guo et al., 2016), consistent with the observation that more cyprids choose to settle on negatively charged carboxyl self-assembled monolayers (SAMs) (Petrone et al., 2011).
Through immunoblotting, a glycoprotein called the settlement-inducing protein complex (SIPC) originally isolated from adult barnacles is also identified within cyprid footprints (Matsumura et al., 1998; Dreanno et al., 2006a). The SIPC in Amphibalanus amphitrite (GenBank accession no. AY423545) has three subunits with apparent molecular weights (MW) of approximately 98 kDa, 88 kDa, and 76 kDa. Each of them is glycosylated and can be recognized by lentil lectin. Eliminating glycosylation and signal peptides, A. amphitrite SIPC has a theoretical MW of approximately 169 kDa and a calculated pI of approximately 5.0 (Matsumura et al., 1998; Dreanno et al., 2006b). In a further study, the pI of isolated A. amphitrite SIPC has consistently been determined to be 4.6–4.7 by isoelectric focusing (Petrone et al., 2015). Based on this, the acidic pI of A. amphitrite SIPC is not in agreement with the latest discovery that A. amphitrite cyprid footprints mainly comprise basic proteins, which probably suggests that the SIPC is only one among many unknown footprint proteins. Besides, sequence alignment reveals that A. amphitrite SIPC shares approximately 30% identity with members of the α2-macroglobulin (A2M) family (Dreanno et al., 2006b).
So far, the SIPC is the only characterized protein in cyprid footprints and it plays multiple roles. First, it acts as a conspecific biochemical cue to mediate larvae-adult and larvae-larvae interactions to induce the gregarious settlement of cyprids (Rittschof and Cohen, 2004). Settlement assays found that even a low density of surface-bound SIPC can attract significantly more conspecific cyprids to colonize while the homologous A2M protein fails (Matsumura et al., 1998; Dreanno et al., 2006b, 2007). An SIPC homolog named MULTIFUNCin (GenBank accession no. KC152471) has also been isolated from Balanus glandula. Interestingly, it not only plays the same role as SIPC in promoting gregarious attachment of cyprids, but also functions as a feeding stimulus that induces barnacle predators hunting for barnacles (Ferrier et al., 2016). Another main purpose of the SIPC is functioning as an adhesive protein to facilitate the temporary adhesion of cyprids. By using surface plasmon resonance, Petrone et al. (2015) found that the SIPC of A. amphitrite shows comparable adsorption on various SAMs to that of fibrinogen, a well-known adhesive protein in the extracellular matrix. In contrast, homologous A2M only has weak to no adsorption on the same substrates. It has also been suggested that A. amphitrite SIPC may be able to direct biomineralization, indicating its possible role in the formation of the barnacle calcite basal plate during cyprid metamorphosis (Zhang et al., 2016).
Surface Settlement: Cyprid Permanent Adhesion
Cyprid Cement Apparatus
When the cyprid finds a suitable site during surface exploration, it immediately secretes a permanent adhesive to settle down and initiate metamorphosis. The cyprid permanent adhesive, also called cyprid cement, is synthesized and secreted by the cyprid cement apparatus which includes cement glands and accessory ducts. Notably, the glands used to produce cyprid permanent and temporary adhesives are different. In acorn barnacle cyprids, the permanent cement gland is kidney-shaped, distributed at the posterior of the compound eyes, and contains two types of cells (Figure 2A; Walker, 1971; Gohad et al., 2014), while the unicellular temporary adhesive gland is located in the second segment of the antennules (Nott, 1969; Nott and Foster, 1969). In the cyprid of the stalked barnacle, O. angulata, the permanent adhesive gland is rod-shaped and located at the back of the compound eyes, while the temporary adhesive gland is oval and buried in the mantle of the body (Yap et al., 2017).
In the 1970s, Walker first examined the permanent adhesive gland of the Balanus balanoides cyprid (Walker, 1971). He categorized these gland cells into columnar α and round β cells based on their morphological differences. The two types of cells were located at the apex and the basal area of the gland, respectively (Figure 2A). In addition, he detected phenols and poly phenolase in the α cells but neither of these were found in the β cells. Later, Gohad et al. (2014) discovered that the permanent adhesive of the A. amphitrite cyprid is a dual-phase bioadhesive comprising a protein and a lipid phase (Figure 2E) which are separately stored in the α and β cells before secretion. An in vitro study using isolated permanent adhesive glands of the Megabalanus rosa cyprid found that cyprid cement is secreted via exocytosis under the control of catecholaminergic neurons (Okano et al., 1996). Briefly, when cyprid glands are stimulated by neurotransmitters such as dopamine and noradrenaline, the cytoplasmic vesicles containing cyprid cement migrate to specific regions of the cell to release the glue into the extracellular ducts through exocytosis (Okano et al., 1996). Post secretion, a significant number of densely packed vesicles in gland cells turn into empty vacuoles (Odling et al., 2006). By combining fluorescent labeling and laser scanning confocal microscopy, Gohad et al. (2012) detected adrenergic-like receptors on cyprid gland cells and antennal setae. Moreover, they found that exogenous noradrenaline, an agonist of the adrenergic receptor can induce cyprids to metamorphose without pre-settling on the substrate. After secretion from these glands, cyprid cement is transported via extracellular ducts to the muscular sac for temporary storage. When needed, it is delivered by long cement ducts in the antennules to the attachment discs where it is released through the openings (Figure 2A).
Biochemical Composition of Cyprid Cement
Through early histochemical studies, Hillman and Nace (1970) detected proteins and trace lipids in the permanent adhesive of the Balanus eburneus cyprid and Walker (1971) revealed phenols and poly phenolase, two important components in quinone protein cross-linking in the adhesive plaque of the B. balanoides cyprid. In recent years, with the introduction of advanced microscale and nanoscale analytical techniques, new insights regarding the biochemical compositions of cyprid cement have been obtained (Aldred and Petrone, 2016). For instance, by employing AFM-based force spectroscopy to stretch cyprid cement proteins adhered on the substrate, Phang et al. (2006) observed gradually decreasing stretching events and maximum extension length. This particular result was attributed to the curing of cyprid cement and allowed researchers to estimate curing time to be within several hours (Phang et al., 2006). Via synchrotron radiation based μ-X-ray fluorescence analysis, Senkbeil et al. (2016) detected a large amount of halogen elements as well as low-content metal ions (e.g., Ca2+, Mg2+, and Fe3+) in the permanent adhesive of A. amphitrite and Balanus improvisus cyprids. However, their possible roles have not been discussed. By using laser scanning confocal microscopy to observe cyprid cement deposited on different SAMs, Aldred et al. (2013a) found that a layer of non-protein substance enclosed the proteinaceous bulk glue. Via a combination of chemistry-specific fluorescent probes and Raman microscopy, Gohad et al. (2014) further demonstrated that the non-protein interfacial layer is lipidaceous and the internal bulk glue is phospho proteinaceous (Figure 2E). The protein and lipid phases are separately stored in the α and β cells and the lipid phase is released ahead of the protein phase upon secretion. The interfacial location and prior secretion of the lipid phase probably indicate that it plays the key role in removing boundary water layers and keeping microbes away from the proteinaceous bulk glue (Gohad et al., 2014). This discovery shed light on the important roles of non-protein components in underwater adhesion for the first time. Likewise, He et al. (2018) noted that lipids, as integral components, also assist in mussel underwater adhesion and function similarly as they do in cyprid permanent adhesion. Therefore, secreting water-repelling lipids to prepare the substrate and create an adhesion-friendly local environment ahead of depositing multi-protein holdfasts may be a general strategy for underwater adhesion.
As of date, no glue protein has been isolated from cyprid permanent adhesives, but surprisingly, some barnacle cement proteins (BCPs) originally identified from adult barnacles were found to be also present in cyprid cement glands by immunofluorescence. As shown in Figure 2A, Aacp20k-1 (A. amphitrite cement protein-20 kDa-1) and Aacp20k-2 were separately stored in the α and β cells (He et al., 2013). Aacp100k was detected in the β cells as well (He et al., 2017). Whether other reported BCPs in A. amphitrite, including Aacp19k, Aacp52k, and AaCP43 also exist in cyprid cement glands is unclear at this moment. Nevertheless, these results suggest that adult BCPs may play a role in cyprid permanent adhesion. To confirm this, further studies are required to determine whether they are also present in the cured cyprid adhesive plaque.
Adult Barnacle Underwater Adhesion
On a suitable substrate, the cyprid settles, metamorphoses into a juvenile, and then develops into an adult. During growth, adult barnacles periodically secrete primary cement to achieve firm attachment (Fyhn and Costlow, 1976). Adhered adult barnacles can neither move freely on the surface nor actively detach from the substrate, because in nature, the detachment of a barnacle generally leads to its death. Yet, laboratory studies have revealed that barnacles dislodged from substrates (e.g., silicones) where they have weak adhesion can also secrete secondary cement and attach to a new substrate if their bases are intact. Accordingly, adult barnacle cement is classified into primary and secondary cement. Generally, primary cement refers to the cement used for natural attachment while secondary cement is only employed during reattachment (Saroyan et al., 1970). However, comparative studies confirm that the two types of barnacle cement are identical because they have similar micro- and nano-morphologies, biochemical compositions, and secondary structures (Kamino, 2006; Barlow et al., 2010).
So far, only a few studies have discussed the relationships between adult barnacle cement and cyprid adhesives. Kamino argues that they are different based on the discovery that adult BCP genes of M. rosa are not expressed in the cyprid (Kamino, 2006). While this opinion was well received by most researchers, in A. amphitrite some adult BCPs (Figure 2A) have since been successfully detected in cyprid permanent adhesive glands (He et al., 2013, 2017). Therefore, additional studies are required to elucidate their exact relationships.
Synthesis, Storage, and Secretion of Barnacle Cement
According to histological studies carried out on acorn barnacles in the 1970s (Lacombe and Liguori, 1969; Lacombe, 1970; Walker, 1970; Fyhn and Costlow, 1976) and on stalked barnacles in recent years (Jonker et al., 2012; Zheden et al., 2012; Lobo-da-Cunha et al., 2017), it is generally accepted that barnacle cement is synthesized and stored in unicellular glands, and secreted through a series of extracellular canals. Together, gland cells and drainage canals constitute the entire cement apparatus of adult barnacles.
In most acorn barnacles, cement glands cluster in the mantle chamber next to the basal portion of the body (Figure 3A). They often intermingle with ovarian tissues but can easily be distinguished by Azan staining. A gland cell is globular or oval and its diameter varies with developmental progress. Usually, the cytoplasm of acorn barnacle gland cells is heterogeneous and shows two distinct regions: the synthetic region that is rich in RNA and the storage or secretory region which contains a dense mixture of proteins (Figure 3B; Lacombe and Liguori, 1969; Lacombe, 1970; Walker, 1970; Fyhn and Costlow, 1976). BCPs are synthesized within the synthetic regions and then transported to the storage regions where they are packed in large vacuoles that probably have an acidic internal environment (Power et al., 2010). It is hypothesized that the acidic environment might be beneficial for protein condensation to form high-density secretory granules as well as self-assembled nanofiber structures (Power et al., 2010; Liang et al., 2018). Upon secretion, barnacle cement is delivered via an extracellular canal system comprising collecting canals (CCs), secondary canals (SCs), and principle canals (PCs) to the adhesive joint, where the cement is then released (Figure 3B; Power et al., 2010). The cement apparatus of stalked barnacles is largely similar to that of acorn barnacles with only a few minor differences owing to different life styles and body structures (Jonker et al., 2012; Zheden et al., 2012; Lobo-da-Cunha et al., 2017). First, the unicellular glands of stalked barnacles are gregariously located at the proximal end of the long peduncle adjacent to the capitulum (Figure 3C) rather than the basal portion close to the base. Second, the cytoplasm of gland cells in stalked barnacles does not show the same distinct synthesis and storage regions as seen in acorn barnacles. Instead, stalked barnacle glands contain many uniformly distributed vacuoles. Third, the gland cells of stalked barnacles possess a unique set of intracellular canals (ICCs). Consequently, in stalked barnacles the cement is secreted via the ICCs to the extracellular CCs, and then delivered by the SCs and PCs to the adhesive joint (Figure 3D). More details regarding the cement apparatus of both stalked and acorn barnacles are presented in the review by Power et al. (2010).
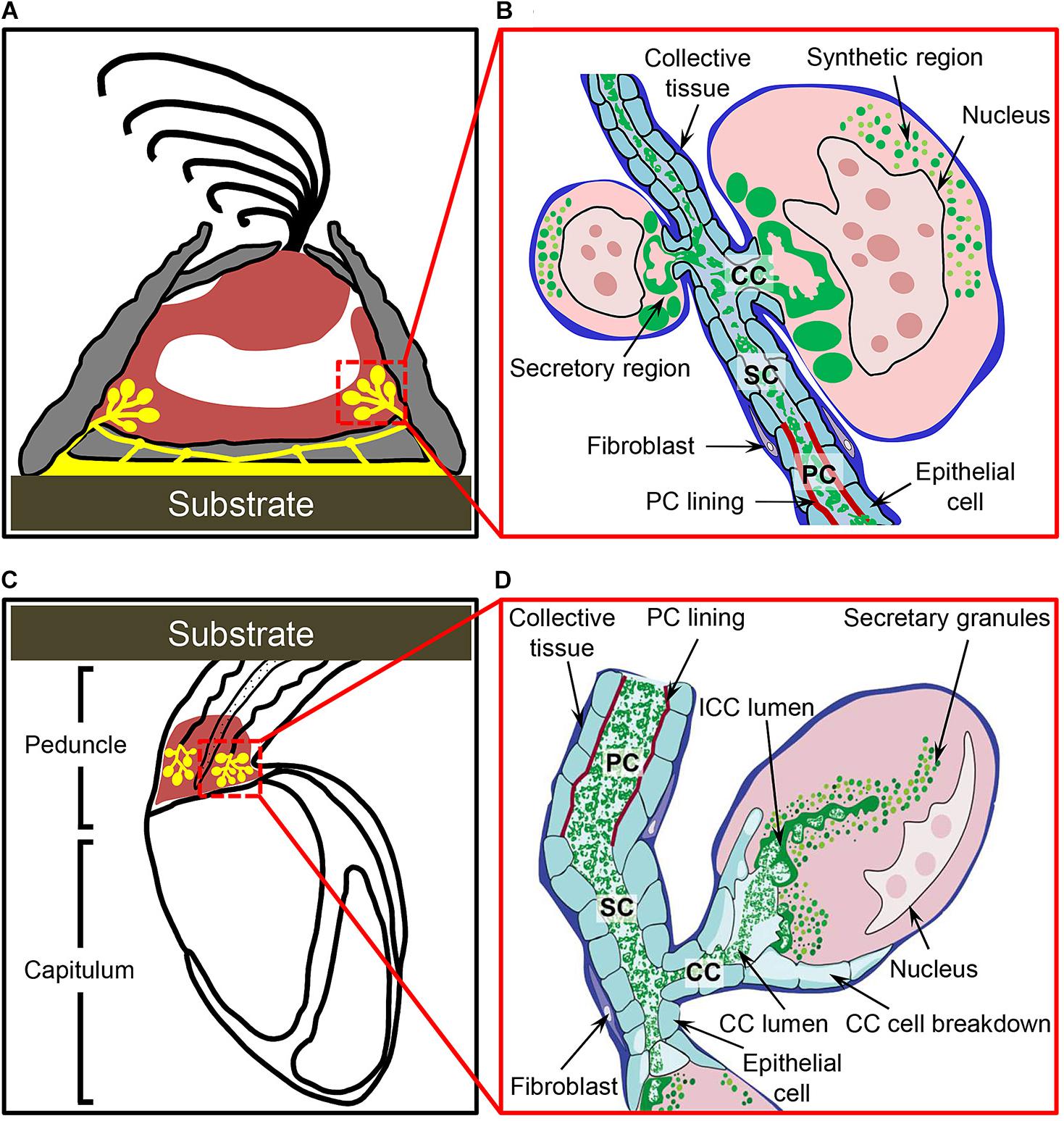
Figure 3. Adult barnacles and their cement apparatuses. (A,B) Schematics of the acorn barnacle and its cement apparatus. In acorn barnacles, cement glands are clustered at the basal region of their bodies and close to the substrate. The cement is synthesized in gland cells, whose cytoplasm is separated into synthetic and secretory regions, and conveyed downward by collecting canals (CCs), secondary canals (SCs), and principle canals (PCs) to the adhesion joint. (C,D) Schematics of the stalked barnacle and its cement apparatus. Cement glands of stalked barnacles are gregariously located at the proximal end of the peduncle and next to the capitulum. In stalked barnacles, the cement is produced in gland cells with homogeneous cytoplasm and transported upward by intracellular canals (ICCs), CCs, SCs, and PCs to the adhesion joint. (C,D) Modified from Jonker et al. (2012). Copyright (2012) Wiley Periodicals, Inc.
Saroyan et al. (1970) noted that the Mallory’s Trichrome stain result of acorn barnacle cement changes when it travels from the SCs to the PCs. Coincidently, a hilum structure was observed at their junction in some acorn barnacles (Lacombe, 1970). In the stalked barnacle, Lepas anatifera, Jonker et al. (2012) failed to detect any histochemical changes in the cement during its transportation in the canals. However, they discovered that the cement changes from a loose, diffused substance to a denser, more clumped substance when it passes through the SCs to the PCs (Jonker et al., 2012). These histochemical and ultrastructural changes possibly imply that barnacle cement has initiated polymerization during transportation in the canals. It is likely that barnacle cement cures slowly in the canals, and therefore, has low-extent cross-linking during secretion so that it does not block the canals entirely. Besides, Burden et al. (2012) proposed that barnacles might employ a step-wise cementing strategy to protect the canals from being blocked. Briefly, a weak adhesive, barnacle cement secretion 1 (BCS1) is first released by barnacles, and subsequently BCS2 is secreted to flush out the canals and cooperate with BCS1 to increase adhesion strength (Burden et al., 2012).
While insightful, these in vivo histological and histochemical studies of barnacle cement apparatuses have yet to be correlated with the in vitro biochemical studies of BCPs that will be discussed in the next section. Thus, the in vivo localizations of different BCPs are not currently understood. Furthermore, this gap has led to an uncertainty whether these isolated BCPs are truly synthesized and secreted via the above discussed pathways. Most notably, a recent study by Fears et al. (2018) noted that in acorn barnacles, the cement deposits at the periphery of barnacle base are transferred to the substrate via the cuticular slip rather than the canal system (Fears et al., 2018). Therefore, the synthesis, storage, and secretion of BCPs are far from being fully understood.
Fibrillar Morphology of Barnacle Cement
Both morphology and adhesion ability of barnacle cement show great variance on different substrates. On stiff substrates that barnacles can easily attach to, such as natural objects, metals, and some polymers, barnacle cement forms a flat, thin, and transparent layer which can duplicate the texture of external substrates and allow for a high adhesion strength (Dougherty, 1990; Raman et al., 2013). Conversely, on soft and elastic substrates like hydrogels and silicones, barnacles deposit thick, opaque, and rubber-like cement which exhibits much lower adhesion strength (Berglin and Gratenholm, 2003; Wiegemann and Watermann, 2003; Holm et al., 2005; Ahmed et al., 2014). This form of barnacle cement occurs probably owing to its high water uptake, as seawater may penetrate into the loosely sealed adhesive joint.
By using microscale and nanoscale imaging tools, the fine structures of barnacle cement on different substrata have been intensively studied. Wiegemann and Watermann (2003) first discovered that the cured cement of B. improvisus mainly comprises of nanoscale globules, which further assemble into different microscale structures, e.g., sponge-like structures or dense sheets on certain metals and fibril meshes on soft surfaces like polydimethylsilane (PDMS). Similarly, the cement of Balanus reticulatus also displays diverse morphologies including fibril meshes, broad bands, and sponge-like structures on different surfaces (Raman and Kumar, 2011). By using AFM imaging, Berglin and Gratenholm (2003) discovered that B. improvisus cement forms dense sheets on polymethylmethacrylate and dispersed nanosized granules on PDMS. Barlow et al. (2010) conducted in vivo AFM observations on the base of A. amphitrite removed from PDMS and detected fibrillar and globular structures in different areas. Likewise, AFM images collected from different regions of A. amphitrite cement deposited on a CaF2 substrate also show different morphologies (Burden et al., 2012). These results probably suggest the spatial and temporal regulation of barnacle cement morphologies. Sullan et al. (2009) observed the same fibrillar structures in A. amphitrite cement remained on glass cover slips, yet, they did not note where the images were captured with respect to the transferred cement.
It was noted that remnants of fibrillar barnacle cement on several substrates could be stained by amyloid fiber dyes – Thioflavin T and Congo Red – indicating that barnacle cement fibers probably contain amyloid fibers (Sullan et al., 2009; Barlow et al., 2010). Amyloid fibers are nanofibers that self-assemble through non-covalent interactions of building blocks rich in β-sheet secondary structures. Both circular dichroism and Fourier transform infrared spectroscopy consistently revealed that around 40% of the total secondary structures of A. amphitrite cement consists of β sheets (Barlow et al., 2009, 2010). Amyloid fibers have exceptional mechanical stability and show resistance to enzymatic degradation (Fukuma et al., 2006; Smith et al., 2006; Fowler et al., 2007; Knowles and Buehler, 2011). Thus, apart from barnacle cement, amyloid fibers have also been detected in some other natural underwater adhesives (Mostaert et al., 2006, 2009). These discoveries have revealed a previously unknown function of amyloid fibers as they were generally only associated with neural degenerative diseases in the past. Therefore, amyloid fibers in bioadhesives are considered as functional amyloid fibers.
Oxidative Chemistry of Barnacle Adhesive Interface
Like other arthropods, the barnacle has an outer cuticle that periodically molts and remolds during growth. In situ observation of the dynamic process of barnacle base expansion and cement secretion throughout the whole molting cycle has found that at the edge of the barnacle base, where newly synthesized cement is released, the outer cuticle of a barnacle is intermixed with the underlying cement layers. Furthermore, the molting of these cuticles coincides with the secretion of barnacle cement (Burden et al., 2014). Consequently, ecdysis-related oxidases (e.g., catechol oxidase and lysyl oxidase) and reactive oxygen species (ROS) build up at the barnacle adhesive interface and lead to a strong oxidative stress (Golden et al., 2016), which is speculated to play a role in catalyzing the cross-linking of barnacle cement fibrils (So et al., 2016, 2017). The detailed cross-linking pathways will be discussed later in this review.
Recently, Fears et al. (2018) found that A. amphitrite releases a lipid- and ROS-abundant oxidative fluid ahead of depositing fibrous barnacle cement. This fluid, whose relationship with ecdysis-related fluid is currently not known, can oxidize surface-adsorbed organic matter, peel off microbial biofilms, and affect the hydration state of the interface (Fears et al., 2018). Similarly, it was observed that the post-settlement A. amphitrite cyprid also secretes a ROS-containing fluid to kill bacteria and clear bacterial biofilms on the substrate during metamorphosis (Essock-Burns et al., 2017). Taken together, the oxidative chemistry of the barnacle adhesive interface can also contribute to remove surface-bound organic layers in order to clear and condition the substrate for strong underwater adhesion.
Isolation and Characterization of BCPs
It is believed that the best way to identify BCPs is to conduct comparative studies on unsolidified liquid cement and cured cement. However, only few studies have examined the biochemical compositions of liquid cement (Essock-Burns et al., 2019), as it can be easily contaminated by other barnacle body liquids leading to controversial conclusions (Kamino, 2010a). On natural substrates, the solidified barnacle cement forms a thin layer with a thickness of only several micrometers underneath the calcified base, making it quite difficult to be collected. Moreover, it is hard to completely dissolve the cured cement. Owing to these challenges, early research on the isolation and characterization of BCPs advanced slowly, although it had already been shown that the protein content of barnacle cement was over 90% (Walker, 1972; Kamino et al., 1996). Later studies noted that barnacles adhering on soft manmade surfaces (e.g., silicones) with thick, opaque, and gummy cement can be dislodged and successfully reattached to specially designed substrates, providing an ideal alternative for the collection of barnacle cement (Rittschof et al., 2008).
Until now, several approaches have been tested to dissolve cured barnacle cement (Barnes and Blackstock, 1976; Naldrett, 1993; Kamino et al., 1996, 2000; Naldrett and Kaplan, 1997; Wiegemann et al., 2006; So et al., 2016), two of which show promising results. The first employs high concentrations of denaturants and reductants that are paired with a heat treatment. This method can render more than 90% of M. rosa cement soluble and release barnacle individuals directly from the substrate (Kamino et al., 2000). With this method, Kamino and his colleagues isolated more than ten protein components from M. rosa cement which were named after their apparent MW estimated by SDS-PAGE. For example, one isolated protein was named Mrcp100k, which denotes a 100-kDa cement protein in M. rosa. The cDNA sequences of Mrcp100k, Mrcp52k, Mrcp20k, and Mrcp19k have been acquired and submitted to the GenBank database (Kamino, 2006, 2008). The second method relies on only one polar solvent, hexafluoroisopropanol, to break down hydrogen bonds important for the stabilization of amyloid fibers. Using this reagent, So et al. (2016) dissolved almost the entire fibrous cement of A. amphitrite and then isolated a new protein named AaCP43, which has a theoretical MW of about 43 kDa but migrates unusually to around 63 kDa during electrophoresis. AaCP43, whose cDNA sequence has also been indexed in the GenBank database, may be the homolog of Mrcp68k owing to their similar amino acid compositions and peptide fragments. However, their sequence identity is not known now as the sequence of Mrcp68k has not been published. So et al. (2016) also identified some proteins homologous to AaCP43 and Aacp19k; yet, no other new types of BCPs were found, probably indicating that barnacles across different taxa share a core set of homologous BCPs.
Many cDNA sequences of homologous BCPs have also been isolated from different barnacle species through rapid amplification of cDNA ends and RNA sequencing. Table 1 summarizes the reported cement proteins with published amino acid sequences from various barnacles. It is worth noting that all the cement proteins in the table are from acorn barnacles except for the recently identified three in the stalked barnacle Pollicipes pollicipes (Rocha et al., 2018). In the next section, the amino acid compositions and the primary and secondary structures of different BCPs will be discussed in detail.
Amino Acid Compositions
The cp100k and cp52k contain a lot of hydrophobic amino acids and their average GRAVY (grand average of hydropathicity) values are 0.089 and −0.081, respectively. In the two proteins, the total content of basic Arg and Lys (11.5% in cp100k and 15.7% in cp52k) as well as that of aromatic Phe and Tyr (9.3% in cp100k and 13.7% in cp52k) is relatively high, while the percentage of Cys is very low (Table 1). In contrast, cp20k lacks hydrophobic amino acids but has an extremely high percentage of Cys. Taking full-length Mrcp20k and Balcp20k as an example, their GRAVY values are −1.082 and −1.188 while their Cys contents are 17.5 and 17.1%, respectively. Moreover, there is an abundance of charged amino acids, such as His (10.4%), Asp (11.5%), and Glu (10.4%) in Mrcp20k as well as His (20.0%) and Lys (9.5%) in Balcp20k (Table 1). The cp19k shows a strong bias to Ser, Thr, Gly, Ala, Lys, and Val, which collectively occupy approximately 70% of the total residues. Notably, cp19k has an extremely low percentage of aromatic amino acids. It can be seen from Table 1 that all these cp19k homologs do not have Tyr or Trp and the average content of Phe is only 1.8%. The amino acid composition of cp68k is quite similar to that of cp19k.
Based on their distinct amino acid compositions, the above five BCPs were classified into three categories by Kamino (2006, 2008) as cement proteins with a high percentage of hydrophobic amino acids (cp100k and cp52k), cement proteins showing a bias to six amino acids (cp68k and cp19k), and cement proteins rich in charged amino acids and Cys (cp20k). Alternatively, So et al. (2016) proposed to divide these BCPs into two groups: the Leu-rich cement protein (LrCP) group which is abundant in Leu, Ile, and Val, and the Gly/Ser-rich cement protein (GSrCP) group that has high proportions of Gly, Ser, Ala, and Thr. Following this classification, cp100k and cp52k belong to the LrCP group, whereas, cp68k and cp19k fall into the GSrCP group. Notably, cp20k falls within neither of these groups.
The post-translationally modified amino acids, 3,4-dihydroxyphenylalanine (DOPA) and phosphorylated serine, play critical roles in mussel and sandcastle worm underwater adhesion (Lee et al., 2011; Stewart et al., 2011a; Waite, 2017). Similarly, could any modified amino acids also function in barnacle underwater attachment systems? Kamino et al. (2012) insist that BCPs do not have any post-translational modifications except for the glycosylation of Mrcp52k. Combining chemistry-specific staining and immunoblotting, Dickinson et al. (2016) detected phosphorylated proteins in the adhesive interface of A. amphitrite, however, these modified proteins have not been isolated. Recently, So et al. (2017) and Fears et al. (2018) confirmed that these phosphorylated proteins are actually cuticular proteins of A. amphitrite rather than its cement proteins.
Primary Structures
The sequence of Mrcp100k is relatively simple. It does not contain any repetitive fragments but instead displays alternating hydrophilic and hydrophobic domains (Kamino et al., 2000), which may be important for its assembly via hydrophobic interactions (Sullan et al., 2009). The primary structure of cp52k, including Mrcp52k and Aacp52k, is made up of four large repeats (Figure 4). Each repeat contains about 150 amino acids and can be further divided into different peptide segments with varying amino acid compositions (Kamino et al., 2012; Nakano and Kamino, 2015). As shown in Figure 4, the primary structure of cp20k can also be divided into multiple repeats based on the regular alignment of Cys (Kamino, 2001; Mori et al., 2007; He et al., 2013). The cp19k and cp68k possess a block copolymer-like sequence property, whose primary structures contain two alternating blocks. One, a STGA-rich segment, showing homology to silk proteins is dominated by Ser, Thr, Gly and Ala, and the other, has an abundance of charged and hydrophobic amino acids (Kamino, 2006; So et al., 2016). The homology of BCPs to silk proteins is attractive but not very surprising given that both barnacles and spiders belong to the phylum Arthropoda (So et al., 2016). Obviously, both animals are capable of fabricating proteinaceous biomaterials with superior mechanical properties. Revealing the sequence characteristics of different BCPs further can enhance our understanding of their structural and functional properties and may inspire the design of novel peptide- or protein-based materials using minimal peptide motifs (Yang et al., 2014).
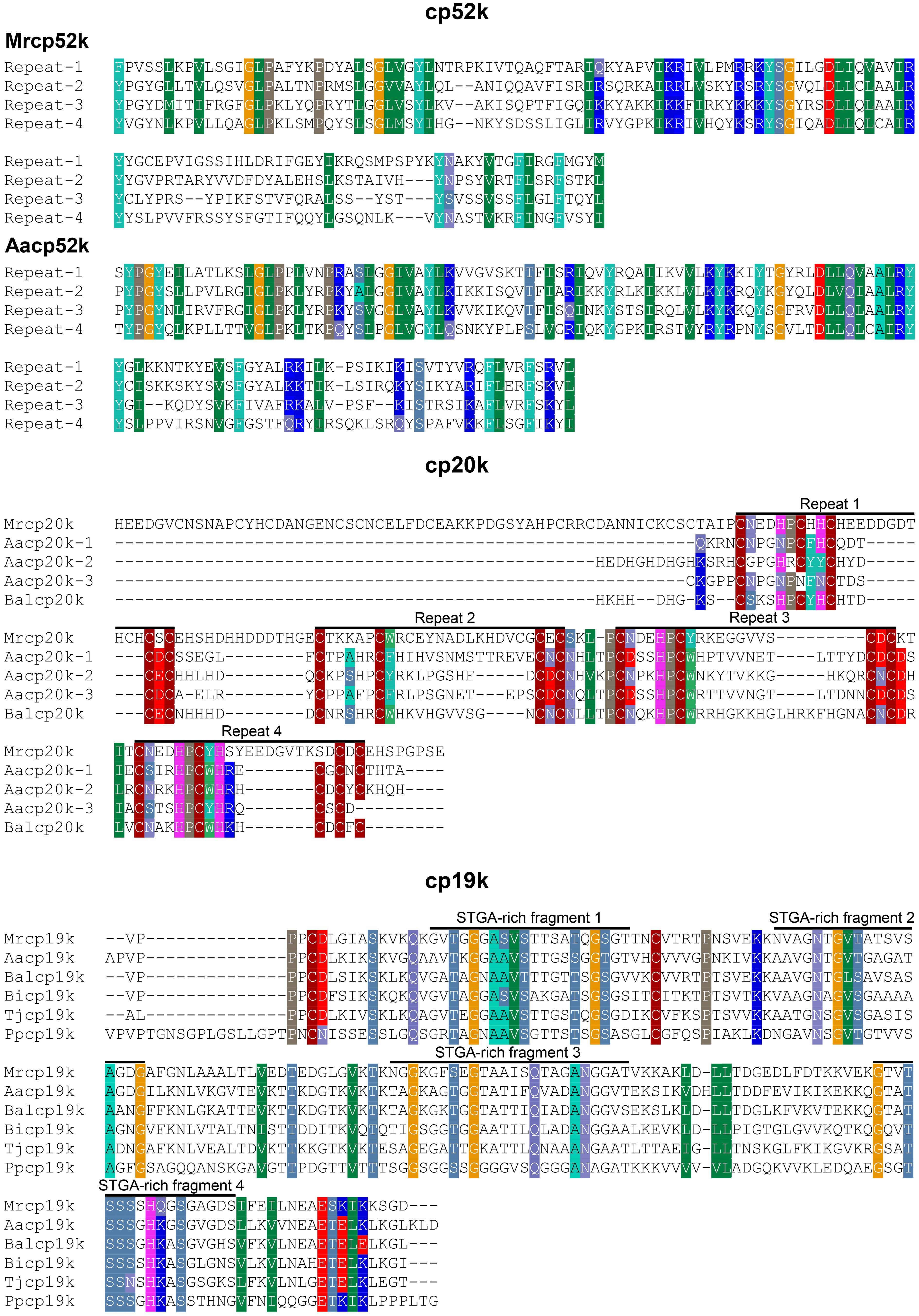
Figure 4. Amino acid sequence alignment of different BCP homologs. The sequence of cp52k (including Mrcp52k and Aacp52k) consists of four large repeats and each of the repeats can be further divided into different fragments based on the distinct amino acid compositions. The primary structure of cp20k also shows repetitive segments according to the regular alignment of Cys. The cp19k exhibits a block copolymer-like sequence characteristic and its primary structure comprises two alternating blocks. One is rich in Ser, Thr, Gly and Ala (STGA-rich fragment), and the other is dominated by hydrophobic and charged amino acids.
Secondary Structures
Owing to the great challenge of isolating natural BCPs, the secondary structures of different BCPs were either theoretically predicted or experimentally examined using peptide analogs or recombinant proteins. It was predicted that approximately 87% of the Mrcp100k sequence forms β sheets (Kamino et al., 2000). The secondary structure of cp52k has not yet been examined, but it was found that Mrcp52k-inspired peptides could form β sheets and self-assemble into amyloid-like fibrils when solution pH and ionic strength increased to a certain threshold (Nakano and Kamino, 2015). Similarly, Nakano et al. (2007) found that Mrcp20k-inspired peptides could also transform to form β sheets and assemble into fibril networks under basic conditions. Although intriguing, these results obtained from peptide studies can not accurately reflect the secondary structures of full-length BCPs because of the significantly different MW, pI, steric hindrance, and so on. Therefore, it is uncertain whether Mrcp20k will change its secondary structures from random coils in a 10 mM sodium phosphate buffer (pH 6.8) to β sheets at higher pH and ionic strength just like Mrcp20k-inspired peptides (Mori et al., 2007). Recently, Wang et al. (2018) and Liang et al. (2018) examined the secondary structures of bacterial recombinant Balcp19k and consistently found that it is dominated by random coils and β sheets. The secondary structure of cp68k is currently not clear.
Molecular Mechanism of Adult Barnacle Underwater Attachment
Underwater adhesion is a multifunctional process that primarily involves four different steps: removing weak boundary layers, wetting, establishing interfacial adhesion, and curing (Waite, 1987). These subfunctions were classified into surface and bulk functions by Kamino (2008), who further claimed that these functions are fulfilled by different components of bioadhesives. In other words, the components of natural adhesives can be functionally classified into their surface and bulk components. Typically, surface components that directly couple with the substrate are located at the interface between the bulk glue and external object. Bulk components, in contrast, are within the glue itself and are responsible for the cross-linking of different glue components. To achieve successful underwater bonding, not only should surface components establish stable interfacial adhesion, but also bulk components must develop strong bulk cohesion. Moreover, surface and bulk components must strongly interact with each other as well. To our knowledge, the extensively studied mussel adhesion system perfectly matches the above assumption. In mussel adhesive plaque, foot protein-3 (fp-3) and fp-5 that bind to the substrate directly are located at the plaque-substrate interface, whereas fp-2 and fp-4 that form foam-like internal structures linking interfacial adhesive proteins and byssus thread structural proteins are distributed within the plaque (Waite, 2017). Based on these principles, the molecular mechanism of adult barnacle underwater adhesion can be understood by addressing the following three critical questions. First, which BCPs are surface proteins and which ones are bulk proteins? Second, how do surface and bulk BCPs achieve interfacial adhesion and bulk cohesion, respectively? Third, how do surface and bulk BCPs interplay?
Based on the pioneering studies on M. rosa cement proteins, Kamino built an original molecular model of adult barnacle underwater attachment (Kamino, 2006). In this model, cp20k, cp19k, and cp68k are speculated to be surface proteins that adhere to substrates via non-covalent interactions, whereas cp100k and cp52k are considered as bulk proteins that play cohesive roles via self-assembling into amyloid fibrils (Kamino, 2006). For the first time, the model discussed the structures and functions of different BCPs and assigned them with surface or bulk subfunctions. In recent years, So et al. (2016, 2017) performed parallel biochemical studies on A. amphitrite cement and discovered that A. amphitrite and M. rosa have different cement protein compositions. In A. amphitrite cement, Aacp19k, AaCP43 (a putative cp68k homolog), and proteins with homology to them are major proteins, whereas Aacp100k and Aacp52k have unexpectedly low percentages. They also noted that underneath the A. amphitrite calcite base there exists a chitinous cuticle layer, and multiple oxidases related to the shedding of this cuticle accumulate in the cement (Burden et al., 2014; So et al., 2017). Based on these findings, So et al. (2017) proposed a different enzyme-catalyzed barnacle cement curing hypothesis, wherein cp19k and cp68k are assumed to play both surface binding and bulk cohesion roles.
In this section, by combining the earlier model and the latest hypothesis, we first discuss the curing mechanism of barnacle cement from three aspects: the interfacial adhesion of surface BCPs, the bulk cohesion of bulk BCPs, and the various interactions between surface and bulk BCPs. Based on these discussions, we then present an updated molecular model of barnacle underwater adhesion.
Interfacial Adhesion of Surface BCPs
Functional characterization of bacterial recombinant BCPs discovered that Mrcp20k specifically adheres to calcite (Mori et al., 2007) while Mrcp19k non-specifically binds to a wide variety of substrates with different surface properties (Urushida et al., 2007). Accordingly, cp20k and cp19k are regarded as surface BCPs playing the key surface coupling role in Kamino’s model. Moreover, based on the empirical principle that the function of a protein is tightly related to its location, it was postulated that cp20k is located at the interface between the barnacle calcite base and bulk cement, while cp19k is distributed at the junction of bulk cement and external substrate (Kamino, 2006; Raman et al., 2016).
Cp20k: Coordination
The selective adhesion of cp20k to specific types of substrates probably implies that chemical bonding rather than physical adhesion is responsible for its interfacial adhesion. It is unlikely that cp20k interacts with the barnacle calcite basal plate via covalent bonds owing to the absence of reactive moieties on this type of inorganic surface. By carefully inspecting the sequence of cp20k, it can be noted that some of its charged amino acids are arranged into small clusters, which may facilitate cp20k to coordinate with metal ions. For example, the EED, EEDDGD, and DHHDDD sequences in Mrcp20k are conducive to its coordination with Ca2+ via side-chain carboxyl groups (Kamino, 2001). Consistently, all the substrates to which Mrcp20k selectively binds normally contain metal ions. Furthermore, the strong molecule-level coordination between cp20k and the barnacle calcite base is also in accord with the macroscopically tenacious adhesion of barnacle cement.
However, the original speculation that cp20k serves as a barnacle calcite base-specific coupling protein is still debated. This is partially because functional studies on bacterial recombinant Mrcp20k reveal its ability to directly mineralize calcite (So et al., 2015). In accordance with this, a transcriptome study of the barnacle Tetraclita japonica formosana which does not have a fully calcified base did not find any cp20k homolog (Lin et al., 2014). Taken together, these results indicate that the exact role of cp20k needs further examination.
Furthermore, in situ observation found a cuticle layer underneath the A. amphitrite calcite base (Burden et al., 2014). Most probably, this structure is also present at the M. rosa adhesive interface, despite it not being mentioned in Kamino’s original model. Its existence greatly complicates our understanding of barnacle underwater adhesion. Briefly, if this organic layer is permeable to barnacle cement, it may not show much influence on Kamino’s model; otherwise, the two interfaces that barnacle cement directly contacts are cuticle and external objects. In this case, the model is quite similar to the adhesion system of barnacles with membranous bases, but unfortunately, such adhesion mechanisms have rarely been studied.
Cp19k and cp68k: Physical Interactions
Barnacles can tenaciously adhere to a wide range of substrates, indicating that proteins assumed to be located at the barnacle cement-substrate boundary, namely cp19k and cp68k, are capable of adaptively and firmly binding to different substrates. That cp19k and cp68k contain numerous amino acids with side-chain amine or hydroxyl groups was believed to be favorable for the removal of surface-bound water layers (Waite, 1987; Maier et al., 2015), a vital step for successful underwater adhesion. Furthermore, it was found that the positively charged Lys, which is abundant in both cp19k and cp68k, can displace the adsorbed cations on mineral substrates to promote the surface binding of interfacial proteins (Maier et al., 2015).
From the amino acid composition of cp19k, it may be inferred that electrostatic interactions, hydrogen bonding, hydrophobic interactions, and van der Waals forces each play a role in its surface binding. It is well known that the neighboring amino acids of active sites always play cooperative roles. For example, in mussel interfacial adhesive proteins, the adjacent Lys of DOPA cooperates with surrounding DOPA to enhance its underwater adhesion ability (Maier et al., 2015; Li et al., 2017a, b). In the mussel foot protein-3s, the proximal hydrophobic amino acids can increase the oxidation potential of DOPA and decrease its self-oxidation tendency (Wei et al., 2013). In cp19k, it is frequent that one side of the Lys region is filled with conformation-flexible amino acids while the other side is made up of hydrophobic amino acids. Based on this, it can be speculated that this alignment of Lys not only endows it with conformational freedom, but also promotes its interfacial adhesion by the synergy between positively charged Lys and hydrophobic amino acids (Ma et al., 2015). It was recently found that recombinant Balcp19k can self-assemble into nanofibers under suitable conditions (Liu et al., 2017; Liang et al., 2018). More importantly, the adhesive ability of self-assembled cp19k nanofibers becomes stronger while also showing a resistance to the adverse influences of basic and high-salinity seawater (Liang et al., 2018). Thus, the unique molecular design of cp19k also enables it to self-assemble into nanofibers, which in turn boosts its overall adhesive ability.
The cp68k has similar amino acid compositions and sequence properties to cp19k, and thus, it is hypothesized to play an interfacial adhesion role too. Yet, its self-assembly property and adhesive ability that have not been examined so far must be somewhat different from cp19k, owing to their different proportions in barnacle cement and distinctive molecular weight, which is known to influence the adhesive ability (Jenkins et al., 2013; Kim et al., 2018).
Internal Cohesion of Bulk BCPs
During dissolution of cured M. rosa cement, it was found that Mrcp100k and Mrcp52k are two major protein components and only after they are dissolved can the surface BCPs be fully released (Kamino et al., 2000). Accordingly, cp100k and cp52k are considered as bulk BCPs. They constitute the insoluble bulk cement of M. rosa and play a cohesive role internally as well as with surface BCPs (Kamino, 2006; Raman et al., 2016). In contrast, So et al. (2016) discovered that cp19k, cp68k, and their homologs, rather than cp100k and cp52k, form the bulk cement of A. amphitrite. Therefore, in the hypothesis proposed by So et al. (2016) these proteins are both surface and bulk proteins.
Self-Assembled Amyloid Fibers
Kamino et al. (2000) first noticed the correlation between barnacle cement and amyloid fibers/plaques, inspired by their similar insolubility, and high β-sheet content. To confirm this correlation, several studies have been conducted to examine the self-assembly properties of different BCPs. Nakano and Kamino (2015) explored the self-assembly property of Mrcp52k-inspired peptides owing to the unavailability of full-length cp52k. They found that these Mrcp52k-inspired peptides can self-assemble into amyloid-like fibrils at increased pH and ionic strengths. Later, our group verified the amyloidogenic self-assembly ability of bacterial recombinant full-length Mrcp52k (Zeng, 2016). Thus, cp52k, and cp100k that has a similar amino acid composition to cp52k, are thought to play cohesive roles via self-assembling into amyloid fibers. It has been proved that hydrogen bonds between protein backbones as well as π-π stacking between aromatic amino acids are important for the assembly and stabilization of amyloid fibers (Gazit, 2002). Consistently, both cp100k and cp52k contain a large number of aromatic amino acids. Besides, hydrophobic interactions may also assist in the self-assembly of cp100k (Sullan et al., 2009).
So et al. (2016) speculate that cp19k and cp68k may also be able to self-assemble into ordered nanofibers, as sequence alignment finds that they contain some randomly distributed silk protein-homologous fragments. Through a series of studies, the self-assembly property of cp19k under different conditions has already been confirmed (Liu et al., 2017; Liang et al., 2018), but whether its self-assembly is enabled by these silk protein-homologous peptide fragments is currently unknown. The self-assembly ability of cp68k has not yet been examined.
Enzyme-Catalyzed Cross-Linking
While investigating the protein components of A. amphitrite cement, So et al. (2017) noted that ecdysis-related oxidative chemistries, including different enzymes and ROS, would accumulate in barnacle cement. Based on this, they hypothesized different enzyme-catalyzed BCP polymerization pathways. As shown in Figure 5A, the peroxidase oxidizes non-peptidyl catechol precursors present in cement layers into an active semi-quinone in a peroxide-dependent way. Subsequently, the semi-quinone reacts with free amine groups in BCPs, forming stable quinone protein cross-linking. This process is similar to the cuticular sclerotization of insects. Compared with the earlier hypothesis that BCPs were cross-linked via quinones oxidized from Tyr under the catalysis of phenol oxidase (Lindner and Dooley, 1973), the current one is more reasonable. This is primarily because the Tyr content in barnacle cement is very low and phenol oxidase inhibitors do not inhibit the curing of barnacle cement. Furthermore, free catechol precursors have also been shown to exist in barnacle cement layers (So et al., 2017). From the perspective of So et al. (2017) the resultant catechol groups in the peroxidase catalyzed cross-linking pathway probably play the important role of interfacial adhesion. In the meanwhile, under the catalysis of lysyl oxidase, barnacle cement fibrils can also be cross-linked via lysine protein cross-linking (Figure 5B), which is commonly observed in the cross-linking of collagen and elastin fibrils (So et al., 2017).
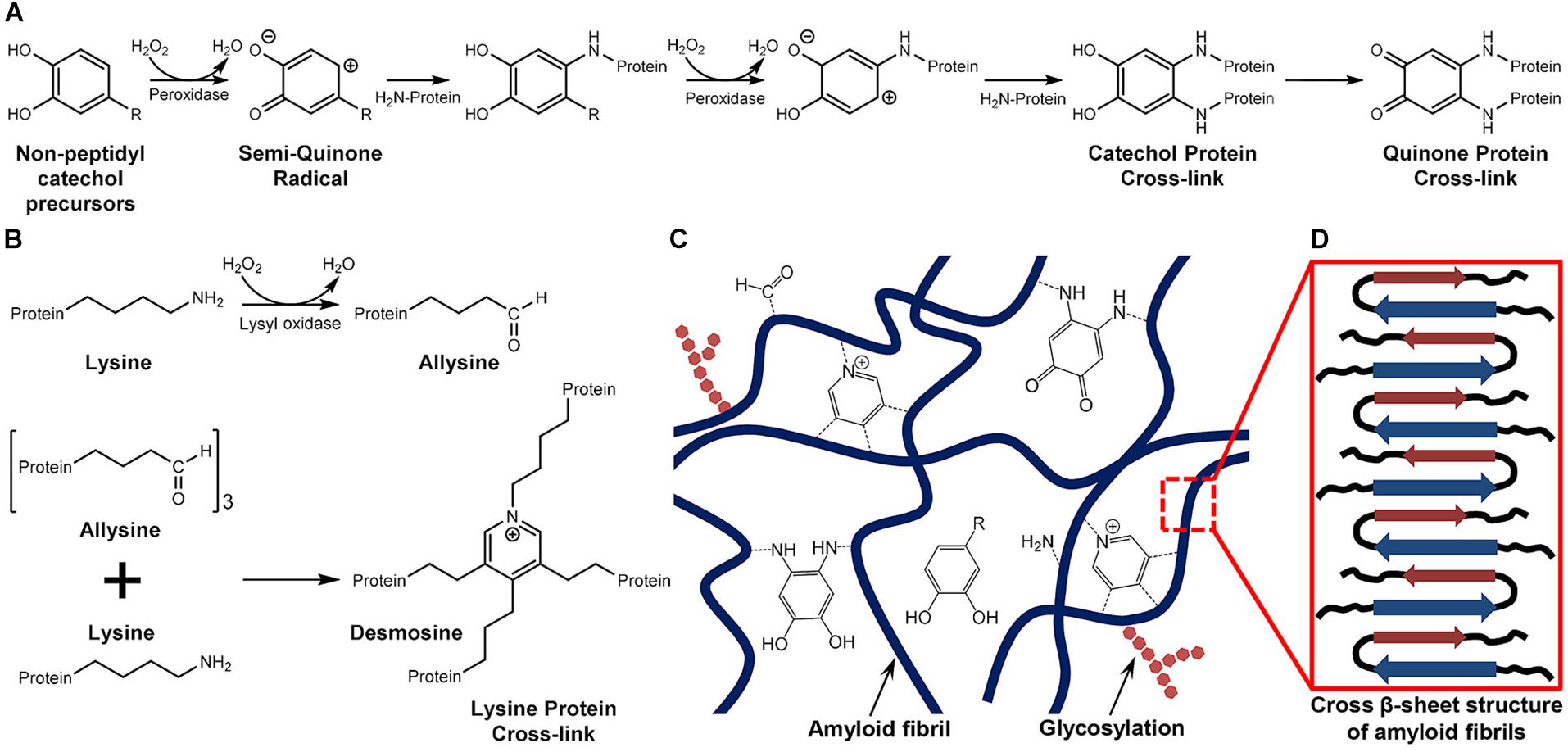
Figure 5. Hypothesized cross-linking mechanisms of bulk BCPs. (A) Quinone protein cross-linking. Non-peptidyl catechol precursors in barnacle cement layers are first oxidized by peroxidase in a peroxide-dependent manner into quinones that then react with amine groups of BCPs to form stable quinone protein cross-links. (B) Lysine (Lys) protein cross-linking. Relying on peroxide, lysyl oxidase converts Lys of BCPs into allysine that further reacts with Lys to form multivalent cross-links. (C) Schematic of the hypothesized cohesion mechanism of bulk BCPs. The bulk BCPs self-assemble into amyloid fibrils, which are then cross-linked through pathways (A,B) to form interwoven fibers. (D) Schematic of typical cross β-sheet structures of amyloid fibers. The figure was modified from So et al. (2017). Copyright (2017) American Chemical Society.
Originally, the above cross-linking reactions were thought to occur on A. amphitrite bulk cement proteins (cp19k and cp68k), but apparently, they could also induce the cross-linking of cp52k and cp100k as long as they were exposed to those oxidative chemistries. In a word, these hypothesized enzyme-catalyzed polymerization reactions shed light on how bulk BCPs are further cross-linked from self-assembled nanofibers to generate fibrous bulk cement (Figures 5C,D). Notably, although the existence of peroxidase and lysyl oxidase has been verified by proteomics (So et al., 2016), the cross-linked products have yet to be identified. It remains inconclusive whether bulk BCPs are cross-linked under their catalysis. Future in vitro studies could substantiate these assumed cross-linking pathways using recombinant BCPs and different enzymes.
Interplays Between Surface and Bulk BCPs
Covalent Cross-Linking
The indispensability of a high concentration of reductant to dissolve cured M. rosa cement, together with the fact of all BCPs containing Cys, may easily lead to the assumption that intermolecular disulfide bonds contribute to the polymerization of barnacle cement. However, in the interfacial Mrcp20k (Kamino, 2001) and the bulk Mrcp52k (Kamino et al., 2012), all Cys residues were believed to form intramolecular disulfide bonds. Raman spectroscopy also failed to detect any intermolecular disulfide bonds in Balanus crenatus cement (Wiegemann et al., 2006). Furthermore, A. amphitrite cement was fully dissolved without supplying any reductant (So et al., 2016). Collectively, it is unlikely that surface and bulk BCPs interact with each other by intermolecular disulfide bonds. As depicted in Figure 5, So et al. (2017) proposed that bulk BCPs could be internally cross-linked under the catalysis of multiple oxidases. Similarly, these enzymes may also be able to catalyze the cross-links between surface and bulk BCPs as all of them can offer lysine to satisfy the requirements of those reactions. It is worth noting that some researchers assume that barnacle cement polymerization is akin to blood coagulation based on the identification of relevant enzymes in unsolidified liquid cement, however, these authors have not discussed how they work on different BCPs yet (Essock-Burns et al., 2019).
Coordination
As bulk BCPs self-assemble into amyloid fibers, the associations between surface and bulk BCPs may be similar to the interactions between amyloid fibers and amyloid fiber binding proteins. It has been reported that a glycoprotein named the serum amyloid P component could bind to the sugar chains of some pathogenic amyloid fibers via coordinate bonds that rely on Ca2+, in order to protect them from being degraded (Pepys et al., 1997). It is a member of the pentraxin family which exists in a wide variety of vertebrates. A pentraxin family member was discovered in the marine arthropod horseshoe crab (Shrive et al., 2009), indicating that homologous pentraxin family members might exist in barnacles too. From current data, it is unlikely that these hypothesized interfacial proteins (cp20k, cp19k, and cp68k) are members of the pentraxin family due to the low sequence homology. However, amino acid composition and sequence analysis suggest that there is still a possibility that cp20k coordinates with sugar chains in barnacle cement amyloid fibers under the mediation of Ca2+. Consistently, amyloidogenic bulk Mrcp52k is glycosylated (Kamino et al., 2012). While the function of glycosylation of Mrcp52k is not clear, the postulation that Mrcp52k uses its sugar chains to associate with cp20k is reasonable. Intermolecular cross-linking based on protein-sugar interactions was also discovered in other marine bioadhesive systems. For example, Apfp-1, a protein that links the byssus and soft foot tissues in the fan shell, Atrina pectinata, employs DOPA-Fe3+ coordination to interact with other foot proteins and uses a lectin binding domain to cross-link with mannose on the cell membrane in a Ca2+ dependent manner (Yoo et al., 2016).
Non-covalent Interactions
When using a surface force apparatus (SFA) to examine the cohesion force of peptide analogs of Mefp-5, Gebbie et al. (2017) discovered that non-covalent cation-π interactions between benzene rings of aromatic amino acids and side-chain amine groups of positively charged amino acids can establish strong intermolecular interactions, especially when no hydroxyl group is on the benzene ring (Phe > Tyr > DOPA). This finding suggests that in barnacle cement, the lysine-rich interfacial cp19k and cp68k may be able to strongly interplay with the internal cp100k and cp52k, which have abundant Phe and Tyr, through cation-π interactions. Importantly, cation-π interactions can also effectively conquer the electrostatic repulsion among likely charged BCPs in seawater (Kim et al., 2016, 2017).
In another study, Raman et al. (2016) designed two cp19k-inspired decapeptides to better understand the adhesion behaviors of full-length cp19k. One (GSGSVPPPCD, Bp1) contains only hydrophobic amino acids while the other (GSKLDLLTDG, Bp2) has both hydrophobic and charged amino acids. Using SFA to characterize their adhesion strength between mica and gold (or SAMs), they found that Bp2 can firmly bridge the two surfaces via electrostatic and hydrophobic interactions, whereas Bp1 fails. Accordingly, they proposed that the heterogeneity of hydrophobic and charged amino acids in cp19k can promote its ability to asymmetrically bridge hydrophobic bulk cement and charged external substrates (Raman et al., 2016).
Updated Molecular Model of Adult Barnacle Underwater Adhesion
According to the above analysis, we now present an updated molecular model of adult barnacle underwater adhesion by highlighting various hypothesized adhesive protein-substrate and cohesive protein-protein interactions.
Acorn barnacles first release an oxidative fluid to clear and condition the substratum, and then deposit fibrous cement for adhesion (Fears et al., 2018). In barnacles with calcified bases, their cement is secreted into the space between the outer cuticle and the external substrate, rather than the calcite base and the external substrate as previously described in Kamino’s model (Kamino, 2006). The cp20k was initially suggested to be a calcite-specific binding protein playing a role in bridging the barnacle basal plate and bulk cement, possibly through coordination. Owing to the presence of this cuticle layer, this speculation now sounds not so reasonable, unless the chitinous cuticle is torn during barnacle growth, and is permeable to barnacle cement. Kamino’s model is based solely on biochemical studies of M. rosa and therefore, cannot be broadly applied to different barnacle species, as the physiological structures and cement protein compositions vary considerably among species. This model postulates that cp19k and cp68k of M. rosa play an interfacial adhesion role via non-covalent interactions. Although physical interactions are relatively weak, their amino acid compositions, sequences, and structures have all been evolutionally optimized to enhance the surface binding ability. In comparison, in A. amphitrite cement, these two types of proteins play both interfacial adhesion and bulk cohesion roles. They rely on pendent catechol groups to establish surface coupling, as well as self-assembled nanofibers and enzyme-catalyzed polymerization to achieve curing (So et al., 2017). As for M. rosa, their bulk cement proteins (cp100k and cp52k) can cure in the same way. In this barnacle, those same enzyme-catalyzed reactions may also promote the interplay between surface and bulk BCPs. Besides, interfacial cp19k and cp68k may be able to use cation-π and hydrophobic interactions to interact with hydrophobic bulk BCPs too.
Given our poor knowledge of the cement protein compositions of stalked barnacles, their underwater adhesion molecular mechanism has not been discussed by any studies so far. Looking forward, conducting more accurate studies regarding these hypothesized molecular interactions is essential and may soon be possible by taking advantage of enhanced nanoscale and microscale surface force characterization tools, such as AFM and SFA, as well as microbial recombinant BCPs and their peptide analogs. Furthermore, barnacle underwater adhesion is a challenging multidisciplinary research field, to fully resolve the underlying molecular mechanism and develop barnacle-inspired technologies also necessitate strengthened collaborations from scientists and engineers with different research backgrounds.
Final Remarks
All the three reversible or irreversible underwater adhesion events involved in a barnacle’s life cycle are accomplished by depositing and curing multi-protein natural adhesives.
During surface exploration, the cyprid uses a pair of attachment discs that are fully covered by cuticular villi to rapidly, tenaciously, and reversibly adhere to the substrate. This adhesion process is chemically, physically, and behaviorally co-mediated. The cyprid footprint is the temporary adhesive, which mainly comprises basic proteins (Guo et al., 2016). The SIPC is the only protein identified so far in cyprid temporary adhesive and plays multiple roles including surface adhesion, biochemical signaling, and biomineralization. Upon finding a suitable site, the cyprid instantly deposits cyprid cement comprising lipids and phosphoproteins for irreversible colonization. The two chemical phases are synthesized and stored in different gland cells. When secreted, lipids are first released to create a niche conducive to protein adhesion. After curing, lipids form the outer shell while phosphoproteins constitute the internal core of the cyprid adhesive plaque (Gohad et al., 2014). A few BCPs have been unexpectedly detected in cyprid permanent adhesive glands, but whether they are cyprid cement components and whether they assist in cyprid irreversible adhesion is currently not clear. The adult barnacle periodically secretes barnacle cement during growth to secure its underwater attachment. It first releases a fluid rich in lipids and oxidants to prepare the substrate and then deposits proteinaceous cement (Fears et al., 2018). Barnacle cement shows fibrillar morphologies and comprises multiple proteins with distinctive amino acid compositions and structural properties. Kamino suggests that different BCPs have different spatial location in the cement layer, but they all rely on non-covalent interactions to achieve adhesion or cohesion (Kamino, 2006). In parallel, the Wahl group revealed the ecdysis-related oxidative stress at the barnacle adhesive interface and proposed thereof an enzyme-catalyzed BCP cross-linking hypothesis (So et al., 2017). Unfortunately, both these hypotheses lack experimental evidences.
The biochemical compositions of cyprid adhesives and their relationships with barnacle cement should be further investigated in detail. To gain a better understanding of adult barnacle underwater adhesion, the secretion sequence of different BCPs and their spatial distribution in the cement layer, which have not yet been examined, also need to be addressed. In addition, further studies can be conducted to substantiate these hypothesized molecular interactions involved in the curing of barnacle cement.
Author Contributions
CL conceived this review and wrote the manuscript with help from all other authors. JS, ZY, WW, BH, and DR provided the helpful suggestions and revised the manuscript.
Funding
This work was supported by an award from the National University of Defense Technology (ZK17-03-43).
Conflict of Interest Statement
The authors declare that the research was conducted in the absence of any commercial or financial relationships that could be construed as a potential conflict of interest.
Acknowledgments
The authors thank the editor and reviewers for their constructive comments on the manuscript.
References
Ahmed, N., Murosaki, T., Kurokawa, T., Kakugo, A., Yashima, S., Nogata, Y., et al. (2014). Prolonged morphometric study of barnacles grown on soft substrata of hydrogels and elastomers. Biofouling 30, 271–279. doi: 10.1080/08927014.2013.863280
Aldred, N., Alsaab, A., and Clare, A. S. (2018). Quantitative analysis of the complete larval settlement process confirms Crisp’s model of surface selectivity by barnacles. Proc. R. Soc. B 285, 20171957. doi: 10.1098/rspb.2017.1957
Aldred, N., and Clare, A. S. (2008). The adhesive strategies of cyprids and development of barnacle-resistant marine coatings. Biofouling 24, 351–363. doi: 10.1080/08927010802256117
Aldred, N., Gohad, N. V., Petrone, L., Orihuela, B., Liedberg, B., Ederth, T., et al. (2013a). Confocal microscopy-based goniometry of barnacle cyprid permanent adhesive. J. Exp. Biol. 216, 1969–1972. doi: 10.1242/jeb.084913
Aldred, N., Hoeg, J. T., Maruzzo, D., and Clare, A. S. (2013b). Analysis of the behaviours mediating barnacle cyprid reversible adhesion. PLoS One 8:e68085. doi: 10.1371/journal.pone.0068085
Aldred, N., and Petrone, L. (2016). “Progress in the study of adhesion by marine invertebrate larvae,” in Biological Adhesives, ed. A. M. Smith (Cham: Springer International Publishing), 87–105. doi: 10.1007/978-3-319-46082-6_4
Aldred, N., Scardino, A., Cavaco, A., de Nys, R., and Clare, A. S. (2010). Attachment strength is a key factor in the selection of surfaces by barnacle cyprids (Balanus amphitrite) during settlement. Biofouling 26, 287–299. doi: 10.1080/08927010903511626
Al-Yahya, H., Chen, H.-N., Chan, B. K. K., Kado, R., and Høeg, J. T. (2016). Morphology of cyprid attachment organs compared across disparate barnacle taxa: does it relate to habitat? Biol. Bull. 231, 120–129. doi: 10.1086/690092
Amini, S., Kolle, S., Petrone, L., Ahanotu, O., Sunny, S., Sutanto, C. N., et al. (2017). Preventing mussel adhesion using lubricant-infused materials. Science 357, 668–673. doi: 10.1126/science.aai8977
Anderson, D. T. (1994). Barnacles: Structure, Function, Development and Evolution. London: Chapman and Hall.
Andersson, O., Ekblad, T., Aldred, N., Clare, A. S., and Liedberg, B. (2009). Novel application of imaging surface plasmon resonance for in situ studies of the surface exploration of marine organisms. Biointerphases 4, 65–68. doi: 10.1116/1.3274060
Barlow, D. E., Dickinson, G. H., Orihuela, B., Kulp, J. L. III, Rittschof, D., and Wahl, K. J. (2010). Characterization of the adhesive plaque of the barnacle Balanus amphitrite: amyloid-like nanofibrils are a major component. Langmuir 26, 6549–6556. doi: 10.1021/la9041309
Barlow, D. E., Dickinson, G. H., Orihuela, B., Rittschof, D., and Wahl, K. J. (2009). In situ ATR-FTIR characterization of primary cement interfaces of the barnacle Balanus amphitrite. Biofouling 25, 359–366. doi: 10.1080/08927010902812009
Barnes, H., and Blackstock, J. (1976). Further observations on the biochemical composition of the cement of Lepas fascicularis Ellis & Solander; electrophoretic examination of the protein moieties under various conditions. J. Exp. Mar. Biol. Ecol. 25, 263–271. doi: 10.1016/0022-0981(76)90128-3
Berglin, M., and Gratenholm, P. (2003). The barnacle adhesive plaque: morphological and chemical differences as a response to substrate properties. Colloids Surf. B 28, 107–117. doi: 10.1016/s0927-7765(02)00149-2
Berntsson, K. M., Jonsson, P. R., Lejhall, M., and Gatenholm, P. (2000). Analysis of behavioural rejection of micro-textured surfaces and implications for recruitment by the barnacle Balanus improvisus. J. Exp. Mar. Biol. Ecol. 251, 59–83. doi: 10.1016/S0022-0981(00)00210-0
Bielecki, J., Chan, B. K. K., Hoeg, J. T., and Sari, A. (2009). Antennular sensory organs in cyprids of balanomorphan cirripedes: standardizing terminology using Megabalanus rosa. Biofouling 25, 203–214. doi: 10.1080/08927010802688087
Brickner, I., and Hoeg, J. T. (2010). Antennular specialization in cyprids of coral-associated barnacles. J. Exp. Mar. Biol. Ecol. 392, 115–124. doi: 10.1016/j.jembe.2010.04.015
Burden, D. K., Barlow, D. E., Spillmann, C. M., Orihuela, B., Rittschof, D., Everett, R. K., et al. (2012). Barnacle Balanus amphitrite adheres by a stepwise cementing process. Langmuir 28, 13364–13372. doi: 10.1021/la301695m
Burden, D. K., Spillmann, C. M., Everett, R. K., Barlow, D. E., Orihuela, B., Deschamps, J. R., et al. (2014). Growth and development of the barnacle Amphibalanus amphitrite: time and spatially resolved structure and chemistry of the base plate. Biofouling 30, 799–812. doi: 10.1080/08927014.2014.930736
Callow, J. A., and Callow, M. E. (2011). Trends in the development of environmentally friendly fouling-resistant marine coatings. Nat. Commun. 2:244. doi: 10.1038/ncomms1251
Chaw, K. C., and Birch, W. R. (2009). Quantifying the exploratory behaviour of Amphibalanus amphitrite cyprids. Biofouling 25, 611–619. doi: 10.1080/08927010903033621
Chaw, K. C., Dickinson, G. H., Ang, K., Deng, J., and Birch, W. R. (2011). Surface exploration of Amphibalanus amphitrite cyprids on microtextured surfaces. Biofouling 27, 413–422. doi: 10.1080/08927014.2011.577210
Chen, Z.-F., Matsumura, K., Wang, H., Arellano, S. M., Yan, X., Alam, I., et al. (2011). Toward an understanding of the molecular mechanisms of barnacle larval settlement: a comparative transcriptomic approach. PLoS One 6:e22913. doi: 10.1371/journal.pone.0022913
Clare, A. S., Freet, R. K., and McClary, M. (1994). On the antennular secretion of the cyprid of Balanus amphitrite amphitrite, and its role as a settlement pheromone. J. Mar. Biol. Assoc. U.K. 74, 243–250. doi: 10.1017/s0025315400035803
Crisp, D. J., and Meadows, P. S. (1962). The chemical basis of gregariousness in cirripedes. Proc. R. Soc. Lond. B 156, 500–520. doi: 10.1098/rspb.1962.0052
Del Grosso, C. A., McCarthy, T. W., Clark, C. L., Cloud, J. L., and Wilker, J. J. (2016). Managing redox chemistry to deter marine biological adhesion. Chem. Matter. 28, 6791–6796. doi: 10.1021/acs.chemmater.6b03390
Di Fino, A., Petrone, L., Aldred, N., Ederth, T., Liedberg, B., and Clare, A. S. (2014). Correlation between surface chemistry and settlement behaviour in barnacle cyprids (Balanus improvisus). Biofouling 30, 143–152. doi: 10.1080/08927014.2013.852541
Dickinson, G. H., Yang, X., Wu, F. H., Orihuela, B., Rittschof, D., and Beniash, E. (2016). Localization of phosphoproteins within the barnacle adhesive interface. Biol. Bull. 230, 233–242. doi: 10.1086/BBLv230n3p233
Dougherty, W. J. (1990). SEM observations on the interfacial surface of the cement of the adult barnacle, attached to natural and synthetic adherends. Tissue Cell 22, 463–470. doi: 10.1016/0040-8166(90)90075-k
Dreanno, C., Kirby, R. R., and Clare, A. S. (2006a). Smelly feet are not always a bad thing: the relationship between cyprid footprint protein and the barnacle settlement pheromone. Biol. Lett. 2, 423–425. doi: 10.1098/rsbl.2006.0503
Dreanno, C., Matsumura, K., Dohmae, N., Takio, K., Hirota, H., Kirby, R. R., et al. (2006b). An α2-macroglobulin-like protein is the cue to gregarious settlement of the barnacle Balanus amphitrite. Proc. Natl. Acad. Sci. U.S.A. 103, 14396–14401. doi: 10.1073/pnas.0602763103
Dreanno, C., Kirby, R. R., and Clare, A. S. (2007). Involvement of the barnacle settlement-inducing protein complex (SIPC) in species recognition at settlement. J. Exp. Mar. Biol. Ecol. 351, 276–282. doi: 10.1016/j.jembe.2007.07.003
Essock-Burns, T., Gohad, N. V., Orihuela, B., Mount, A. S., Spillmann, C. M., Wahl, K. J., et al. (2017). Barnacle biology before, during and after settlement and metamorphosis: a study of the interface. J. Exp. Biol. 220, 194–207. doi: 10.1242/jeb.145094
Essock-Burns, T., Soderblom, E. J., Orihuela, B., Moseley, M. A., and Rittschof, D. (2019). Hypothesis testing with proteomics: a case study using wound healing mechanisms in fluids associated with barnacle glue. Front. Mar. Sci. 6:343. doi: 10.3389/fmars.2019.00343
Fears, K. P., Orihuela, B., Rittschof, D., and Wahl, K. J. (2018). Acorn barnacles secrete phase-separating fluid to clear surfaces ahead of cement deposition. Adv. Sci. 5:1700762. doi: 10.1002/advs.201700762
Ferrier, G. A., Kim, S. J., Kaddis, C. S., Loo, J. A., Ann Zimmer, C., and Zimmer, R. K. (2016). MULTIFUNCin: a multifunctional protein cue induces habitat selection by, and predation on, barnacles. Integr. Comp. Biol. 56, 901–913. doi: 10.1093/icb/icw076
Fowler, D. M., Koulov, A. V., Balch, W. E., and Kelly, J. W. (2007). Functional amyloid - from bacteria to humans. Trends Biochem. Sci. 32, 217–224. doi: 10.1016/j.tibs.2007.03.003
Fukuma, T., Mostaert, A. S., and Jarvis, S. P. (2006). Explanation for the mechanical strength of amyloid fibrils. Tribol. Lett. 22, 233–237. doi: 10.1007/s11249-006-9086-8
Fyhn, U. E. H., and Costlow, J. D. (1976). Histochemical study of cement secretion during intermolt cycle in barnacles. Biol. Bull. 150, 47–56. doi: 10.2307/1540588
Gazit, E. (2002). A possible role for π-stacking in the self-assembly of amyloid fibrils. FASEB J. 16, 77–83. doi: 10.1096/fj.01-0442hyp
Gebbie, M. A., Wei, W., Schrader, A. M., Cristiani, T. R., Dobbs, H. A., Idso, M., et al. (2017). Tuning underwater adhesion with cation–π interactions. Nat. Chem. 9, 473–479. doi: 10.1038/nchem.2720
Gohad, N. V., Aldred, N., Hartshorn, C. M., Lee, Y. J., Cicerone, M. T., Orihuela, B., et al. (2014). Synergistic roles for lipids and proteins in the permanent adhesive of barnacle larvae. Nat. Commun. 5:4414. doi: 10.1038/ncomms5414
Gohad, N. V., Aldred, N., Orihuela, B., Clare, A. S., Rittschof, D., and Mount, A. S. (2012). Observations on the settlement and cementation of barnacle (Balanus amphitrite) cyprid larvae after artificial exposure to noradrenaline and the locations of adrenergic-like receptors. J. Exp. Mar. Biol. Ecol. 416, 153–161. doi: 10.1016/j.jembe.2012.02.013
Golden, J. P., Burden, D. K., Fears, K. P., Barlow, D. E., So, C. R., Burns, J., et al. (2016). Imaging active surface processes in barnacle adhesive interfaces. Langmuir 32, 541–550. doi: 10.1021/acs.langmuir.5b03286
Guo, S., Puniredd, S. R., Janczewski, D., Lee, S. S. C., Teo, S. L. M., He, T., et al. (2014). Barnacle larvae exploring surfaces with variable hydrophilicity: influence of morphology and adhesion of “footprint” proteins by AFM. ACS Appl. Mater. Inter. 6, 13667–13676. doi: 10.1021/am503147m
Guo, S., Zhu, X., Jańczewski, D., Lee, S. S. C., He, T., Teo, S. L. M., et al. (2016). Measuring protein isoelectric points by AFM-based force spectroscopy using trace amounts of sample. Nat. Nanotechnol. 11, 817–823. doi: 10.1038/nnano.2016.118
He, L.-S., Zhang, G., and Qian, P.-Y. (2013). Characterization of two 20kDa-cement protein (cp20k) homologues in Amphibalanus amphitrite. PLoS One 8:e64130. doi: 10.1371/journal.pone.0064130
He, L.-S., Zhang, G., Wang, Y., Yan, G.-Y., and Qian, P.-Y. (2017). Toward understanding barnacle cementing by characterization of one cement protein-100kDa in Amphibalanus amphitrite. Biochem. Biophys. Res. Commun. 495, 969–975. doi: 10.1016/j.bbrc.2017.11.101
He, Y., Sun, C., Jiang, F., Yang, B., Li, J., Zhong, C., et al. (2018). Lipids as integral components in mussel adhesion. Soft Matter. 14, 7145–7154. doi: 10.1039/c8sm00509e
Hennebert, E., Maldonado, B., Ladurner, P., Flammang, P., and Santos, R. (2015). Experimental strategies for the identification and characterization of adhesive proteins in animals: a review. Interface Focus 5:20140064. doi: 10.1098/rsfs.2014.0064
Hillman, R. E., and Nace, P. F. (1970). “Histochemistry of barnacle cyprid adhesive formation,” in Adhesion in Biological Systems, ed. R. S. Manly (New York: Academic Press), 113–121. doi: 10.1016/b978-0-12-469050-9.50011-9
Holm, E. R. (2012). Barnacles and biofouling. Integr. Comp. Biol. 52, 348–355. doi: 10.1093/icb/ics042
Holm, E. R., Orihuela, B., Kavanagh, C. J., and Rittschof, D. (2005). Variation among families for characteristics of the adhesive plaque in the barnacle Balanus amphitrite. Biofouling 21, 121–126. doi: 10.1080/08927010512331344188
Jenkins, C. L., Meredith, H. J., and Wilker, J. J. (2013). Molecular weight effects upon the adhesive bonding of a mussel mimetic polymer. ACS Appl. Mater. Inter. 5, 5091–5096. doi: 10.1021/am4009538
Jonker, J.-L., von Byern, J., Flammang, P., Klepal, W., and Power, A. M. (2012). Unusual adhesive production system in the barnacle Lepas anatifera: an ultrastructural and histochemical investigation. J. Morphol. 273, 1377–1391. doi: 10.1002/jmor.20067
Kamino, K. (2001). Novel barnacle underwater adhesive protein is a charged amino acid-rich protein constituted by a Cys-rich repetitive sequence. Biochem. J. 356, 503–507. doi: 10.1042/0264-6021:3560503
Kamino, K. (2006). “Barnacle underwater attachment,” in Biological Adhesives, eds A. Smith and J. Callow (Berlin: Springer Berlin Heidelberg), 145–166. doi: 10.1007/978-3-540-31049-5_8
Kamino, K. (2008). Underwater adhesive of marine organisms as the vital link between biological science and material science. Mar. Biotechnol. 10, 111–121. doi: 10.1007/s10126-007-9076-3
Kamino, K. (2010a). Absence of cross-linking via trans-glutaminase in barnacle cement and redefinition of the cement. Biofouling 26, 755–760. doi: 10.1080/08927014.2010.514335
Kamino, K. (2010b). Molecular design of barnacle cement in comparison with those of mussel and tubeworm. J. Adhes. 86, 96–110. doi: 10.1080/00218460903418139
Kamino, K. (2013). Mini-review: barnacle adhesives and adhesion. Biofouling 29, 735–749. doi: 10.1080/08927014.2013.800863
Kamino, K., Inoue, K., Maruyama, T., Takamatsu, N., Harayama, S., and Shizuri, Y. (2000). Barnacle cement proteins - importance of disulfide bonds in their insolubility. J. Biol. Chem. 275, 27360–27365. doi: 10.1074/jbc.M910363199
Kamino, K., Nakano, M., and Kanai, S. (2012). Significance of the conformation of building blocks in curing of barnacle underwater adhesive. FEBS J. 279, 1750–1760. doi: 10.1111/j.1742-4658.2012.08552.x
Kamino, K., Odo, S., and Maruyama, T. (1996). Cement proteins of the acorn barnacle. Megabalanus rosa. Biol. Bull. 190, 403–409. doi: 10.2307/1543033
Khandeparker, L., and Anil, A. C. (2007). Underwater adhesion: the barnacle way. Int. J. Adhes. Adhes. 27, 165–172. doi: 10.1016/j.ijadhadh.2006.03.004
Kim, E., Dai, B., Qiao, J. B., Li, W., Fortner, J. D., and Zhang, F. (2018). Microbially synthesized repeats of mussel foot protein display enhanced underwater adhesion. ACS Appl. Mater. Inter. 10, 43003–43012. doi: 10.1021/acsami.8b14890
Kim, S., Huang, J., Lee, Y., Dutta, S., Yoo, H. Y., Jung, Y. M., et al. (2016). Complexation and coacervation of like-charged polyelectrolytes inspired by mussels. Proc. Natl. Acad. Sci. U.S.A. 113, E847–E853. doi: 10.1073/pnas.1521521113
Kim, S., Yoo, H. Y., Huang, J., Lee, Y., Park, S., Park, Y., et al. (2017). Salt triggers the simple coacervation of an underwater adhesive when cations meet aromatic π electrons in seawater. ACS Nano 11, 6764–6772. doi: 10.1021/acsnano.7b01370
Knight-Jones, E. W., and Crisp, D. J. (1953). Gregariousness in barnacles in relation to the fouling of ships and to anti-Fouling research. Nature 171, 1109–1110. doi: 10.1038/1711109a0
Knowles, T. P. J., and Buehler, M. J. (2011). Nanomechanics of functional and pathological amyloid materials. Nat. Nanotechnol. 6, 469–479. doi: 10.1038/nnano.2011.102
Lacombe, D. (1970). A comparative study of the cement glands in some balanid barnacles (Cirripedia, Balanidae). Biol. Bull. 139, 164–179. doi: 10.2307/1540134
Lacombe, D., and Liguori, V. R. (1969). Comparative histological studies of the cement apparatus of Lepas anatifera and Balanus tintinnabulum. Biol. Bull. 137, 170–180. doi: 10.2307/1539940
Larman, V. N., and Gabbott, P. A. (1975). Settlement of cyprid larvae of Balanus balanoides and Elminius modestus induced by extracts of adult barnacles and other marine animals. J. Mar. Biol. Assoc. U.K. 55, 183–190. doi: 10.1017/s0025315400015824
Lee, B. P., Messersmith, P. B., Israelachvili, J. N., and Waite, J. H. (2011). Mussel-inspired adhesives and coatings. Annu. Rev. Mater. Res. 41, 99–132. doi: 10.1146/annurev-matsci-062910-100429
Li, Y., Liang, C., Gao, L., Li, S., Zhang, Y., Zhang, J., et al. (2017a). Hidden complexity of synergistic roles of Dopa and lysine for strong wet adhesion. Mater. Chem. Front. 1, 2664–2668. doi: 10.1039/c7qm00402h
Li, Y., Wang, T., Xia, L., Wang, L., Qin, M., Li, Y., et al. (2017b). Single-molecule study of the synergistic effects of positive charges and Dopa for wet adhesion. J. Mater. Chem. B 5, 4416–4420. doi: 10.1039/c7tb00131b
Liang, C., Ye, Z., Xue, B., Zeng, L., Wu, W., Zhong, C., et al. (2018). Self-assembled nanofibers for strong underwater adhesion: the trick of barnacles. ACS Appl. Mater. Inter. 10, 25017–25025. doi: 10.1021/acsami.8b04752
Lin, H.-C., Wong, Y. H., Tsang, L. M., Chu, K. H., Qian, P.-Y., and Chan, B. K. K. (2014). First study on gene expression of cement proteins and potential adhesion-related genes of a membranous-based barnacle as revealed from next-generation sequencing technology. Biofouling 30, 169–181. doi: 10.1080/08927014.2013.853051
Lindner, E., and Dooley, C. (1973). “Chemical bonding in cirripede adhesive,” in Proceedings of the Third International Congress on Marine Corrosion and Fouling, (Evanston, IL: Northwestern University Press), 653–673.
Liu, X., Liang, C., Zhang, X., Li, J., Huang, J., Zeng, L., et al. (2017). Amyloid fibril aggregation: an insight into the underwater adhesion of barnacle cement. Biochem. Biophys. Res. Commun. 493, 654–659. doi: 10.1016/j.bbrc.2017.08.136
Lobo-da-Cunha, A., Alves, Â, Oliveira, E., and Cunha, I. (2017). The cement apparatus of the stalked barnacle Pollicipes pollicipes. Mar. Biol. 164:11. doi: 10.1007/s00227-016-3047-z
Ma, C. D., Wang, C., Acevedo-Vélez, C., Gellman, S. H., and Abbott, N. L. (2015). Modulation of hydrophobic interactions by proximally immobilized ions. Nature 517, 347–350. doi: 10.1038/nature14018
Maier, G. P., Rapp, M. V., Waite, J. H., Israelachvili, J. N., and Butler, A. (2015). Adaptive synergy between catechol and lysine promotes wet adhesion by surface salt displacement. Science 349, 628–632. doi: 10.1126/science.aab0556
Maki, J. S., Yule, A. B., Rittschof, D., and Mitchell, R. (1994). The effect of bacterial films on the temporary adhesion and permanent fixation of cypris larvae, Balanus amphitrite Darwin. Biofouling 8, 121–131. doi: 10.1080/08927019409378267
Maleschlijski, S., Sendra, G. H., Di Fino, A., Leal-Taixé, L., Thome, I., Terfort, A., et al. (2012). Three dimensional tracking of exploratory behavior of barnacle cyprids using stereoscopy. Biointerphases 7:50. doi: 10.1007/s13758-012-0050-x
Maleshlijski, S., Sendra, G. H., Aldred, N., Clare, A. S., Liedberg, B., Grunze, M., et al. (2016). Imaging SPR combined with stereoscopic 3D tracking to study barnacle cyprid-surface interactions. Surface Sci. 643, 172–177. doi: 10.1016/j.susc.2015.08.027
Marechal, J. P., Hellio, C., Sebire, M., and Clare, A. S. (2004). Settlement behaviour of marine invertebrate larvae measured by EthoVision 3.0. Biofouling 20, 211–217. doi: 10.1080/08927010400011674
Matsumura, K., Nagano, M., and Fusetani, N. (1998). Purification of a larval settlement-inducing protein complex (SIPC) of the barnacle, Balanus amphitrite. J. Exp. Zool. 281, 12–20. doi: 10.1002/(sici)1097-010x(19980501)281:1<12:aid-jez3<3.0.co;2-f
Matsumura, K., Nagano, M., Kato-Yoshinaga, Y., Yamazaki, M., Clare, A. S., and Fusetani, N. (1998). Immunological studies on the settlement–inducing protein complex (SIPC) of the barnacle Balanus amphitrite and its possible involvement in larva–larva interactions. Proc. R. Soc. Lond. B 265, 1825–1830. doi: 10.1098/rspb.1998.0508
Mori, Y., Urushida, Y., Nakano, M., Uchiyama, S., and Kamino, K. (2007). Calcite-specific coupling protein in barnacle underwater cement. FEBS J. 274, 6436–6446. doi: 10.1111/j.1742-4658.2007.06161.x
Mostaert, A. S., Higgins, M. J., Fukuma, T., Rindi, F., and Jarvis, S. P. (2006). Nanoscale mechanical characterisation of amyloid fibrils discovered in a natural adhesive. J. Biol. Phys. 32, 393–401. doi: 10.1007/s10867-006-9023-y
Mostaert, S. A., Crockett, R., Kearn, G., Cherny, I., Gazit, E., Serpell, C. L., et al. (2009). Mechanically functional amyloid fibrils in the adhesive of a marine invertebrate as revealed by Raman spectroscopy and atomic force microscopy. Arch. Histol. Cytol. 72, 199–207. doi: 10.1679/aohc.72.199
Nakano, M., and Kamino, K. (2015). Amyloid-like conformation and interaction for the self-assembly in barnacle underwater cement. Biochemistry 54, 826–835. doi: 10.1021/bi500965f
Nakano, M., Shen, J.-R., and Kamino, K. (2007). Self-assembling peptide inspired by a barnacle underwater adhesive protein. Biomacromolecules 8, 1830–1835. doi: 10.1021/bm0612236
Naldrett, M. J. (1993). The importance of sulphur cross-links and hydrophobic interactions in the polymerization of barnacle cement. J. Mar. Biol. Assoc. U.K. 73, 689–702. doi: 10.1017/S0025315400033221
Naldrett, M. J., and Kaplan, D. L. (1997). Characterization of barnacle (Balanus eburneus and B. cenatus) adhesive proteins. Mar. Biol. 127, 629–635. doi: 10.1007/s002270050053
Nott, J. A. (1969). Settlement of barnacle larvae: surface structure of the antennular attachment disc by scanning electron microscopy. Mar. Biol. 2, 248–251. doi: 10.1007/bf00351147
Nott, J. A., and Foster, B. A. (1969). On the structure of the antennular attachment organ of the cypris larva of Balanus balanoides (L.). Philos. Trans. R. Soc. Lond. B 256, 115–134. doi: 10.1098/rstb.1969.0038
Odling, K., Albertsson, C., Russell, J. T., and Martensson, L. G. E. (2006). An in vivo study of exocytosis of cement proteins from barnacle Balanus improvisus (D.) cyprid larva. J. Exp. Biol. 209, 956–964. doi: 10.1242/jeb.02031
Okano, K., Shimizu, K., Satuito, C. G., and Fusetani, N. (1996). Visualization of cement exocytosis in the cypris cement gland of the barnacle Megabalanus rosa. J. Exp. Biol. 199, 2131–2137.
Pepys, M. B., Booth, D. R., Hutchinson, W. L., Gallimore, J. R., Collins, I. M., and Hohenester, E. (1997). Amyloid P component. A critical review. Amyloid 4, 274–295. doi: 10.3109/13506129709003838
Perez-losada, M., Hoeg, J. T., and Crandall, K. A. (2009). “Stalked and acorn barnacles (Thoracica),” in The Timetree of Life, eds S. Blair Hedges and S. Kumar (Oxford: Oxford University Press), 298–301.
Petrone, L., Aldred, N., Emami, K., Enander, K., Ederth, T., and Clare, A. S. (2015). Chemistry-specific surface adsorption of the barnacle settlement-inducing protein complex. Interface Focus 5:20140047. doi: 10.1098/rsfs.2014.0047
Petrone, L., Di Fino, A., Aldred, N., Sukkaew, P., Ederth, T., Clare, A. S., et al. (2011). Effects of surface charge and Gibbs surface energy on the settlement behaviour of barnacle cyprids (Balanus amphitrite). Biofouling 27, 1043–1055. doi: 10.1080/08927014.2011.625474
Phang, I. Y., Aldred, N., Clare, A. S., Callow, J. A., and Vancso, G. J. (2006). An in situ study of the nanomechanical properties of barnacle (Balanus amphitrite) cyprid cement using atomic force microscopy (AFM). Biofouling 22, 245–250. doi: 10.1080/08927010600857686
Phang, I. Y., Aldred, N., Clare, A. S., and Vancso, G. J. (2008). Towards a nanomechanical basis for temporary adhesion in barnacle cyprids (Semibalanus balanoides). J. R. Soc. Interface 5, 397–401. doi: 10.1098/rsif.2007.1209
Phang, I. Y., Aldred, N., Ling, X. Y., Huskens, J., Clare, A. S., and Vancso, G. J. (2010). Atomic force microscopy of the morphology and mechanical behaviour of barnacle cyprid footprint proteins at the nanoscale. J. R. Soc. Interface 7, 285–296. doi: 10.1098/rsif.2009.0127
Phang, I. Y., Chaw, K. C., Choo, S. S. H., Kang, R. K. C., Lee, S. S. C., Birch, W. R., et al. (2009). Marine biofouling field tests, settlement assay and footprint micromorphology of cyprid larvae of Balanus amphitrite on model surfaces. Biofouling 25, 139–147. doi: 10.1080/08927010802592925
Power, A., Klepal, W., Zheden, V., Jonker, J., McEvilly, P., and von Byern, J. (2010). “Mechanisms of adhesion in adult barnacles,” in Biological Adhesive Systems, eds J. von Byern and I. Grunwald (Vienna: Springer), 153–168. doi: 10.1007/978-3-7091-0286-2_9
Raman, S., Karunamoorthy, L., Doble, M., Kumar, R., and Venkatesan, R. (2013). Barnacle adhesion on natural and synthetic substrates: adhesive structure and composition. Int. J. Adhes. Adhes. 41, 140–143. doi: 10.1016/j.ijadhadh.2012.11.003
Raman, S., and Kumar, R. (2011). Interfacial morphology and nanomechanics of cement of the barnacle, Amphibalanus reticulatus on metallic and non-metallic substrata. Biofouling 27, 569–577. doi: 10.1080/08927014.2011.589027
Raman, S., Malms, L., Utzig, T., Shrestha, B. R., Stock, P., Krishnan, S., et al. (2016). Adhesive barnacle peptides exhibit a steric-driven design rule to enhance adhesion between asymmetric surfaces. Colloids Surf. B 152, 42–48. doi: 10.1016/j.colsurfb.2016.12.038
Rittschof, D., and Cohen, J. H. (2004). Crustacean peptide and peptide-like pheromones and kairomones. Peptides 25, 1503–1516. doi: 10.1016/j.peptides.2003.10.024
Rittschof, D., Orihuela, B., Stafslien, S., Daniels, J., Christianson, D., Chisholm, B., et al. (2008). Barnacle reattachment: a tool for studying barnacle adhesion. Biofouling 24, 1–9. doi: 10.1080/08927010701784920
Rocha, M., Antas, P., Castro, L. F. C., Campos, A., Vasconcelos, V., Pereira, F., et al. (2018). Comparative analysis of the adhesive proteins of the adult stalked goose barnacle Pollicipes pollicipes (Cirripedia: Pedunculata). Mar. Biotechnol. 21, 38–51. doi: 10.1007/s10126-018-9856-y
Rosenhahn, A., and Sendra, G. H. (2012). Surface sensing and settlement strategies of marine biofouling organisms. Biointerphases 7:63. doi: 10.1007/s13758-012-0063-5
Saroyan, J. R., Lindner, E., and Dooley, C. A. (1970). Repair and reattchment in the balanidae as related to their cementing mechanism. Biol. Bull. 139, 333–350. doi: 10.2307/1540088
Schultz, M. P., Bendick, J. A., Holm, E. R., and Hertel, W. M. (2011). Economic impact of biofouling on a naval surface ship. Biofouling 27, 87–98. doi: 10.1080/08927014.2010.542809
Senkbeil, T., Mohamed, T., Simon, R., Batchelor, D., Di Fino, A., Aldred, N., et al. (2016). In vivo and in situ synchrotron radiation-based μ-XRF reveals elemental distributions during the early attachment phase of barnacle larvae and juvenile barnacles. Anal. Bioanal. Chem. 408, 1487–1496. doi: 10.1007/s00216-015-9253-6
Shrive, A. K., Burns, I., Chou, H.-T., Stahlberg, H., Armstrong, P. B., and Greenhough, T. J. (2009). Crystal structures of limulus SAP-like pentraxin reveal two molecular aggregations. J. Mol. Biol. 386, 1240–1254. doi: 10.1016/j.jmb.2009.01.008
Smith, J. F., Knowles, T. P. J., Dobson, C. M., MacPhee, C. E., and Welland, M. E. (2006). Characterization of the nanoscale properties of individual amyloid fibrils. Proc. Natl. Acad. Sci. U.S.A. 103, 15806–15811. doi: 10.1073/pnas.0604035103
So, C. R., Fears, K. P., Leary, D. H., Scancella, J. M., Wang, Z., Liu, J. L., et al. (2016). Sequence basis of barnacle cement nanostructure is defined by proteins with silk homology. Sci. Rep. 6:36219. doi: 10.1038/srep36219
So, C. R., Liu, J., Fears, K. P., Leary, D. H., Golden, J. P., and Wahl, K. J. (2015). Self-assembly of protein nanofibrils orchestrates calcite step movement through selective nonchiral interactions. ACS Nano 9, 5782–5791. doi: 10.1021/acsnano.5b01870
So, C. R., Scancella, J. M., Fears, K. P., Essock-Burns, T., Haynes, S. E., Leary, D. H., et al. (2017). Oxidase activity of the barnacle adhesive interface involves peroxide-dependent catechol oxidase and lysyl oxidase enzymes. ACS Appl. Mater. Inter. 9, 11493–11505. doi: 10.1021/acsami.7b01185
Stewart, R. J., Ransom, T. C., and Hlady, V. (2011a). Natural underwater adhesives. J. Polym. Sci. Pol. Phys. 49, 757–771. doi: 10.1002/polb.22256
Stewart, R. J., Wang, C. S., and Shao, H. (2011b). Complex coacervates as a foundation for synthetic underwater adhesives. Adv. Colloid Interface Sci. 167, 85–93. doi: 10.1016/j.cis.2010.10.009
Sullan, R. M. A., Gunari, N., Tanur, A. E., Yuri, C., Dickinson, G. H., Orihuela, B., et al. (2009). Nanoscale structures and mechanics of barnacle cement. Biofouling 25, 263–275. doi: 10.1080/08927010802688095
Urushida, Y., Nakano, M., Matsuda, S., Inoue, N., Kanai, S., Kitamura, N., et al. (2007). Identification and functional characterization of a novel barnacle cement protein. FEBS J. 274, 4336–4346. doi: 10.1111/j.1742-4658.2007.05965.x
Waite, J. H. (1987). Nature’s underwater adhesive specialist. Int. J. Adhes. Adhes. 7, 9–14. doi: 10.1016/0143-7496(87)90048-0
Waite, J. H. (2017). Mussel adhesion - essential footwork. J. Exp. Biol. 220, 517–530. doi: 10.1242/jeb.134056
Walker, G. (1970). The histology, histochemistry and ultrastructure of the cement apparatus of three adult sessile barnacles, Elminius modestus, Balanus balanoides and Balanus hameri. Mar. Biol. 7, 239–248. doi: 10.1007/bf00367494
Walker, G. (1971). A study of the cement apparatus of the cypris larva of the barnacle Balanus balanoides. Mar. Biol. 9, 205–212. doi: 10.1007/bf00351380
Walker, G. (1972). The Biochemical composition of the cement of two barnacle species, Balanus Hameri and Balanus Crenatus. J. Mar. Biol. Assoc. U.K. 52, 429–435. doi: 10.1017/S0025315400018786
Walker, G., and Yule, A. B. (1984). Temporary adhesion of the barnacle cyprid - the existence of an antennular adhesive secretion. J. Mar. Biol. Assoc. U.K. 64, 679–686. doi: 10.1017/S0025315400030344
Wang, X., Wang, C., Xu, B., Wei, J., Xiao, Y., and Huang, F. (2018). Adsorption of intrinsically disordered barnacle adhesive proteins on silica surface. Appl. Surf. Sci. 427, 942–949. doi: 10.1016/j.apsusc.2017.08.108
Wang, Z., Leary, D. H., Liu, J., Settlage, R. E., Fears, K. P., North, S. H., et al. (2015). Molt-dependent transcriptomic analysis of cement proteins in the barnacle Amphibalanus amphitrite. BMC Genomics 16:859. doi: 10.1186/s12864-015-2076-1
Wei, W., Yu, J., Broomell, C., Israelachvili, J. N., and Waite, J. H. (2013). Hydrophobic enhancement of Dopa-mediated adhesion in a mussel foot protein. J. Am. Chem. Soc. 135, 377–383. doi: 10.1021/ja309590f
Wiegemann, M., Kowalik, T., and Hartwig, A. (2006). Noncovalent bonds are key mechanisms for the cohesion of barnacle (Balanus crenatus) adhesive proteins. Mar. Biol. 149, 241–246. doi: 10.1007/s00227-005-0219-7
Wiegemann, M., and Watermann, B. (2003). Peculiarities of barnacle adhesive cured on non-stick surfaces. J. Adhes. Sci. Technol. 17, 1957–1977. doi: 10.1163/156856103770572070
Yang, Y. J., Jung, D., Yang, B., Hwang, B. H., and Cha, H. J. (2014). Aquatic proteins with repetitive motifs provide insights to bioengineering of novel biomaterials. Biotechnol. J. 9, 1493–1502. doi: 10.1002/biot.201400070
Yap, F. C., Wong, W.-L., Maule, A. G., Brennan, G. P., Chong, V. C., and Lim, L. H. S. (2017). First evidence for temporary and permanent adhesive systems in the stalked barnacle cyprid, Octolasmis angulata. Sci. Rep. 7:44980. doi: 10.1038/srep44980
Yebra, D. M., Kiil, S., and Dam-Johansen, K. (2004). Antifouling technology - past, present and future steps towards efficient and environmentally friendly antifouling coatings. Prog. Org. Coat. 50, 75–104. doi: 10.1016/j.porgcoat.2003.06.001
Yoo, H. Y., Iordachescu, M., Huang, J., Hennebert, E., Kim, S., Rho, S., et al. (2016). Sugary interfaces mitigate contact damage where stiff meets soft. Nat. Commun. 7:11923. doi: 10.1038/ncomms11923
Yorisue, T., Chan, B. K. K., Kado, R., Watanabe, H., Inoue, K., Kojima, S., et al. (2016). On the morphology of antennular sensory and attachment organs in cypris larvae of the deep-sea vent/seep barnacles, Ashinkailepas and Neoverruca. J. Morphol. 277, 594–602. doi: 10.1002/jmor.20522
Yule, A. B., and Crisp, D. J. (1983). Adhesion of cypris larvae of the barnacle, Balanus Balanoides, to clean and arthropodin treated surfaces. J. Mar. Biol. Assoc. U.K. 63, 261–271. doi: 10.1017/S002531540007065X
Yule, A. B., and Walker, G. (1984). The temporary adhesion of barnacle cyprids - effects of some differing surface characteristics. J. Mar. Biol. Assoc. U.K. 64, 429–439. doi: 10.1017/S0025315400030101
Zeng, L. (2016). Expression and Functional Characterization of the 52kDa Cement Protein in Megabalanus rosa. master’s thesis, National University of Defense Technology: Changsha.
Zhang, G., Yang, X. X., Leung, P. M., He, L. S., Chan, T. Y., Yan, G. Y., et al. (2016). Secretory locations of SIPC in Amphibalanus amphitrite cyprids and a novel function of SIPC in biomineralization. Sci. Rep. 6:29376. doi: 10.1038/srep29376
Zhang, X. K., Liu, X. P., Zeng, L., Ye, Z. H., Hu, B. R., and Wu, W. J. (2017). Barnacle adhesion: from substrate detection to cement curing. Prog. Biochem. Biophys. 44, 204–214. doi: 10.16476/j.pibb.2016.0390
Zhao, Q., Lee, D. W., Ahn, B. K., Seo, S., Kaufman, Y., Israelachvili, J. N., et al. (2016). Underwater contact adhesion and microarchitecture in polyelectrolyte complexes actuated by solvent exchange. Nat. Mater. 15, 407–412. doi: 10.1038/nmat4539
Zheden, V., Von Byern, J., Kerbl, A., Leisch, N., Staedler, Y., Grunwald, I., et al. (2012). Morphology of the cement apparatus and the cement of the buoy barnacle Dosima fascicularis (Crustacea, Cirripedia, Thoracica, Lepadidae). Biol. Bull. 223, 192–204. doi: 10.1086/BBLv223n2p192
Keywords: underwater adhesion, barnacles, cyprid, surface exploration and settlement, cement proteins, self-assembly, curing mechanism
Citation: Liang C, Strickland J, Ye Z, Wu W, Hu B and Rittschof D (2019) Biochemistry of Barnacle Adhesion: An Updated Review. Front. Mar. Sci. 6:565. doi: 10.3389/fmars.2019.00565
Received: 27 April 2019; Accepted: 27 August 2019;
Published: 10 September 2019.
Edited by:
Andrew Stanley Mount, Clemson University, United StatesReviewed by:
Nick Aldred, Newcastle University, United KingdomWong Yue Him, Shenzhen University, China
Copyright © 2019 Liang, Strickland, Ye, Wu, Hu and Rittschof. This is an open-access article distributed under the terms of the Creative Commons Attribution License (CC BY). The use, distribution or reproduction in other forums is permitted, provided the original author(s) and the copyright owner(s) are credited and that the original publication in this journal is cited, in accordance with accepted academic practice. No use, distribution or reproduction is permitted which does not comply with these terms.
*Correspondence: Biru Hu, aHViaXJ1MDhAbnVkdC5lZHUuY24=