- 1Centre for Sustainable Tropical Fisheries and Aquaculture, College of Science and Engineering, James Cook University, Townsville, QLD, Australia
- 2Australian Institute of Marine Science, Cape Cleveland, QLD, Australia
- 3AIMS@JCU, James Cook University, Townsville, QLD, Australia
- 4Cawthron Institute, Nelson, New Zealand
- 5Independent Researcher, Houston, TX, United States
- 6Department of Zoology, University of Cambridge, Cambridge, United Kingdom
- 7Wellcome Sanger Institute, Hinxton, United Kingdom
- 8Marine Biological Laboratory, Woods Hole, MA, United States
As coral aquaculture is increasing around the world for reef restoration and trade, mitigating the impact of coral predators, pathogens and parasites is necessary for optimal growth. The Acropora coral-eating flatworm (AEFW), Prosthiostomum acroporae (Platyhelminthes: Polycladida: Prosthiostomidae) feeds on wild and cultivated Acropora species and its inadvertent introduction into reef tanks can lead to the rapid death of coral colonies. To guide the treatment of infested corals we investigated the flatworm’s life cycle parameters at a range of temperatures that represent those found in reef tanks, coral aquaculture facilities and seasonal fluctuations in the wild. We utilized P. acroporae from a long-term in vivo culture on Acropora species to examine the effects of temperature (3°C increments from 21 to 30°C) on flatworm embryonation period, hatching success, hatchling longevity, and time to sexual maturity. Our findings show that warmer seawater shortened generation times; at 27°C it took, on average, 11 days for eggs to hatch, and 35 days for flatworms to reach sexual maturity, giving a minimum generation time of 38 days, whereas at 24°C the generation time was 64 days. Warmer seawater (24–30°C) also increased egg hatching success compared to cooler conditions (21°C). These results indicate that warmer temperatures lead to higher population densities of P. acroporae. Temperature significantly increased the growth rate of P. acroporae, with individuals reaching a larger size at sexual maturity in warmer temperatures, but it did not influence hatchling longevity. Hatchlings, which can swim as well as crawl, can survive between 0.25 and 9 days in the absence of Acropora, and could therefore disperse between coral colonies and inter-connected aquaria. We used our data to predict embryonation duration and time to sexual maturity at 21–30°C, and discuss how to optimize current treatments to disrupt the flatworm’s life cycle in captivity.
Introduction
Trade in live coral has increased by 10–50% annually since 1987 (Rhyne et al., 2009) and is valued at between $US 200–330 million each year (Wabnitz, 2003). Stony corals (Order: Scleractinia) in the genus Acropora are one of the most popular corals collected for the global marine aquarium trade because of their vibrant colors and diversity of growth forms (Rhyne et al., 2014; Barton et al., 2017). Acropora spp. are suitable candidates for aquaculture in situ or ex situ in land-based facilities and are propagated in a variety of geographic and socioeconomic regions including the Indo-Pacific, Caribbean, and the Great Barrier Reef. Rearing acroporid corals for a sustainable marine aquarium trade could relieve the pressure that conventional collection strategies have placed on wild stocks (Tlusty et al., 2013; Rhyne et al., 2014). For example, recent studies show that the majority of acroporids exported by Indonesia are now cultured (Rhyne et al., 2012, 2014). Coral aquaculture endeavors also form the basis for active restoration programs to restore denuded reefs and mitigate the cumulative pressures on reef ecosystems (e.g., sedimentation, climate change; Fabricius, 2005; Hoegh-Guldberg et al., 2007; Doney et al., 2009; De’ath et al., 2012; Hughes et al., 2017). Acropora spp. are common target species for reef restoration because of their fast growth-rates relative to other scleractinian corals and their contribution to structural complexity (Craggs et al., 2017; Pollock et al., 2017).
Coral propagation efforts can be threatened by the introduction or natural occurrence of coral predators, pathogens and parasites. The Acropora-eating flatworm, Amakusaplana acroporae (Rawlinson et al., 2011) [now known as Prosthiostomum acroporae (Litvaitis et al., 2019)] has been a problematic pest for the coral hobbyist community globally for over a decade (Nosratpour, 2008). Their inadvertent introduction into coral aquaria can lead to irreversible tissue damage and ultimately to the death of entire Acropora colonies (Delbeek and Sprung, 2005; Carl, 2008; Nosratpour, 2008; Rawlinson et al., 2011; Hume et al., 2014). Prosthiostomum acroporae is a polyclad flatworm (Platyhelminthes: Polycladida) belonging to the sub-order Cotylea and family Prosthiostomidae. It lays its egg clusters on bare coral skeleton, and each cluster contains multiple egg capsules within which multiple embryos develop (Rawlinson et al., 2011). Like other cotylean polyclads, P. acroporae development proceeds via a larval form with lobes and ciliary bands for swimming and feeding in the water column (Rawlinson, 2014), but unlike other cotyleans, these larval features develop and are then reduced and lost while still inside the egg capsule, i.e., it is an intracapsular larva that undergoes metamorphosis before hatching as a juvenile (Rawlinson et al., 2011). A consequence of this life history strategy could be increased retention of hatchlings on the natal coral and limited dispersal potential, both of which contribute to its rapid proliferation in captive systems. A further factor aiding its success in captive systems is its camouflage and cryptic habit (Rawlinson et al., 2011; Hume et al., 2014). The long supply chain within the ornamental trade (Wabnitz, 2003; Rubec and Cruz, 2005; Cohen et al., 2013; Fujita et al., 2014) not only presents the opportunity for flatworms to spread between corals at each holding location, but the stress from transportation may increase the susceptibility of Acropora colonies to infestation. Despite a long-standing infamy among coral hobbyists, P. acroporae was only recently reported in the wild from Lizard Island on the Great Barrier Reef, Australia, and the biogeographic range and impact on wild acroporids is not known (Rawlinson and Stella, 2012).
There have been no empirical studies on the management of P. acroporae in captivity or in the wild. Effective management of this species requires a comprehensive understanding of its life cycle so that vulnerable life stages can be identified and eradicated through appropriate treatments. The developmental rate of poikilothermic animals, including polyclad flatworms, is greatly influenced by temperature (Gammoudi et al., 2012), therefore it is important to consider P. acroporae development at a range of temperatures relevant to seasonal fluctuations in the wild and within the temperature ranges of corals maintained in aquaria. The aim of this study was to provide the knowledge that will help formulate approaches that disrupt the life cycle of P. acroporae by investigating the effects of temperature on the embryonation period and hatching success, the longevity of hatchlings and the time to, and size at, sexual maturity (Figure 1). Additionally, there was morphological variation observed for hatchlings, with some emerging with lobes and others without, which may affect their dispersal potential (i.e., those with lobes may have greater dispersal ability). Although difficult to accurately quantify the variation in hatchling morphology, the survivorship was determined across these morphologies as a measure of their dispersal potential.
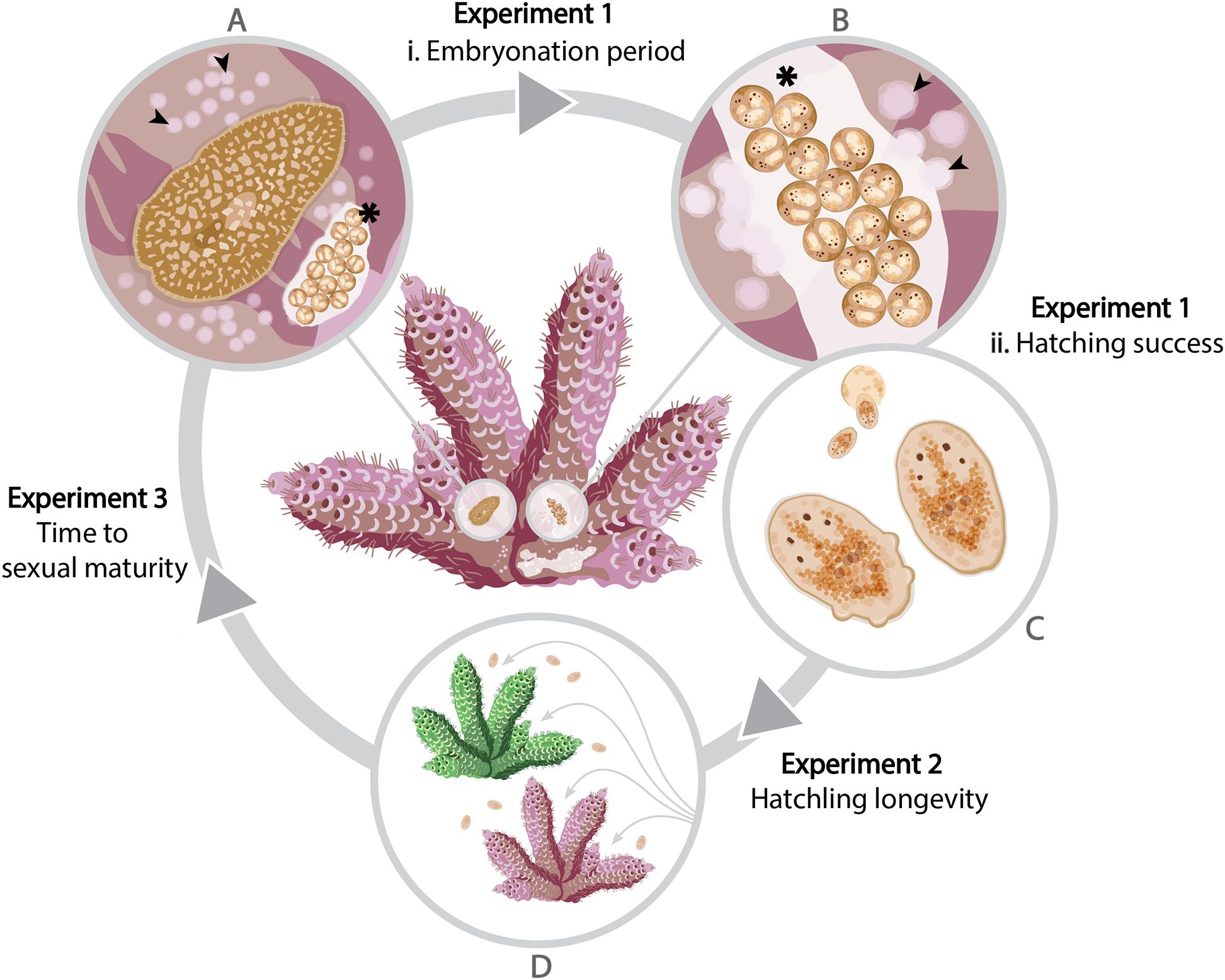
Figure 1. A schematic of the life cycle of Prosthiostomum acroporae showing three experiments to assess the effect of temperature on development. Adult flatworms (A) leave circular feeding scars (arrowheads) on the coral tissue and deposit egg clusters (asterisk) on the coral skeleton. The time from (A) oviposition of the egg cluster to (B,C) hatching is referred to as the embryonation period. Hatchlings (D) may settle on their natal coral (pink), or find a new Acropora host (green), where they feed and develop to sexual maturity, as evidenced by the appearance of the next generation of eggs.
Materials and Methods
Prosthiostomum acroporae Culture
Prosthiostomum acroporae were collected from Acropora spp. colonies (A. millepora, A. spathulata, A. loripes, A. tenuis, A. microclados, A. nasuta, A. microphthalma, A. rosaria) harvested between May 2016 and January 2018 from various inshore (Esk Reef 18° 46.420′ S 146° 31.372′ E) and mid-shelf reefs (Trunk Reef 18°23′20.4′′ S 146°48′25.8′′ E, Davies Reef 18°49′21.6′′ S 147°39′12.5′′ E and Rib Reef 18°28′47.1′′ S 146°52′00.9′′ E) that form part of the central Great Barrier Reef of Australia (GBRMPA Permit No. G12/3236.1). Flatworms were removed from the corals using a jet of filtered seawater (see Supplementary Methods for details on screening corals for flatworms). A continuous culture of P. acroporae was established on a mixture of captive Acropora species (primarily A. millepora, A. spathulata, A. tenuis, A. loripes, and A. nasuta) housed at the National Sea Simulator (SeaSim) at the Australian Institute of Marine Science (AIMS), Queensland. The culture was maintained at 27°C in two 250 L flow-through aquaria supplied with fresh filtered seawater at approximately 2 L min–1. Colony fragments were added to replace dead corals as needed and density adjustments of flatworm infestation were conducted regularly (see Supplementary Material for details on co-culturing flatworms and corals).
Prosthiostomum acroporae lay their eggs on bare coral skeleton (Figure 1) which renders microscopic observations of embryonic development difficult. Subsequently, in vitro laid egg clusters used in experiments were collected using two methods. The first approach involved removing P. acroporae adults from host Acropora colonies using a jet of water and individual worms being placed in plastic bags (Sandvik® plastic bags [127 mm × 200 mm]) containing filtered seawater (1 μm). Visual inspection for egg clusters and 75% water changes were performed daily, and egg clusters were collected within 24 h of oviposition. This method was used for experiment 1 to determine the effect of temperature on embryonation period and hatching success. A second approach was later developed to bolster P. acroporae cultures and provide more egg clusters for experimentation, and was subsequently used for experiments 2 (hatchling longevity and morphology) and 3 (time to sexual maturity and size at sexual maturity). This method used rectangles of clear plastic (2 cm × 5 cm) cut from clean plastic bags, pegged with metal-free clothes pegs onto infested Acropora colonies proximal to feeding scars (see Supplementary Material). The plastic substrates were monitored daily so that egg clusters could be collected within 24 h of oviposition. Egg clusters collected using the second method were only used if they were encased in a continuous layer of ‘cement’ (Figure 2A), indicating that all eggs in the cluster were the product of one laying event from a single parent. Extraneous egg capsules (not covered by a continuous cement layer) were removed.
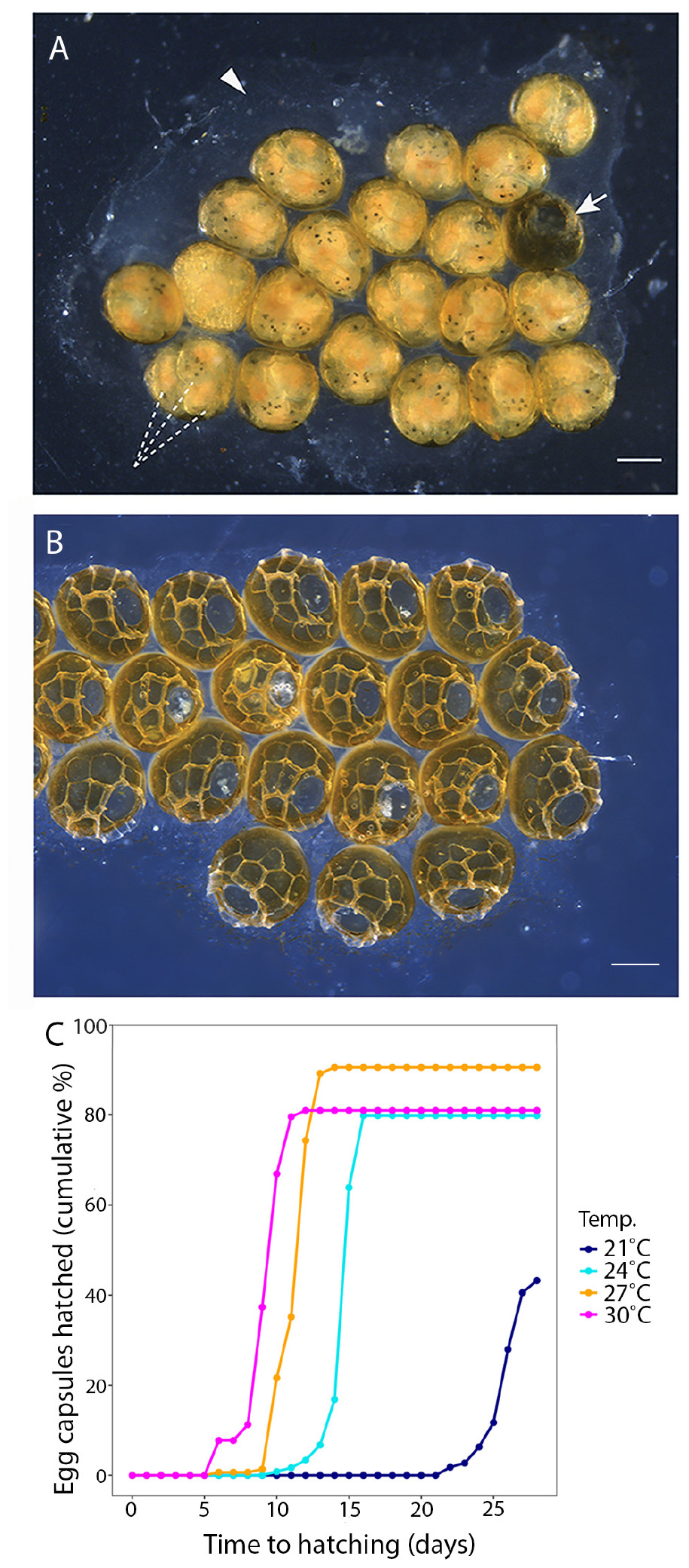
Figure 2. (A) A P. acroporae egg cluster showing multiple embryos (dashed lines) inside each egg capsule (arrow points to a hatched capsule), cemented to the substrate (arrowhead) (scale = 200 μm). (B) An egg cluster showing capsules after the hatchlings have emerged. (C) A cumulative hatching plot showing the effect of temperature on embryonation period and hatching success (n = 125 egg capsules at 21°C, n = 124 egg capsules at 24°C, n = 144 egg capsules at 27°C, n = 129 egg capsules at 30°C; nine replicate egg clusters per temperature).
Experiment 1 – Embryonation Period and Hatching Success
To examine the influence of temperature on embryonation period and hatching success, P. acroporae egg clusters were placed in four separate temperature treatments [21, 24, 27, or 30°C (±0.2°C)]. These temperatures represent the range at which Acropora spp. grow along the Great Barrier Reef, Australia, according to the average monthly water temperature in the northern (Lizard Island), middle (Davies Reef), and southern (Heron Island) portion of the reef1. The chosen temperature range also includes the range of temperatures at which Acropora spp. are commonly kept in captive aquaria.
Thirty-six P. acroporae egg clusters were placed in individual 500 mL flow-through hatching chambers and distributed equally among the four temperature treatments (during September to November 2016). For each temperature treatment, nine hatching chambers were split between three incubation tanks (i.e., three replicate hatching chambers per tank; three tanks total) that were randomly positioned within the experimental room. Hatching chambers were provided with individual water supply and housed in triplicate to ensure temperature stability within each chamber. A 12:12 (light:dark) cycle was used with the first and last hour ramping to the desired intensity of 24 ± 2 μmole m–2 s–1 provided by AquaIllumination Hydra 52 LED modules. This light intensity was selected based on measurement of the light intensity (LI-COR LI-250A light meter and LI-190R Quantum Sensor) reaching egg capsules that were laid on the underside of A. millepora colonies.
Egg clusters were examined daily under a dissection microscope (Leica MZ16; 10–40x) to monitor the development of the embryos in each egg capsule. Observation of each egg cluster continued until the last individual was observed to hatch, or when it was determined that no further embryos were viable. The embryonation period of each egg capsule within a given cluster (Figures 2A,B) was defined as the number of days post-oviposition until the emergence of all viable individuals from the egg capsule through the operculum. Hatching success was expressed as the proportion of capsules within a cluster that gave rise to hatchlings from the total number of capsules in a cluster.
Experiment 2 – Hatchling Longevity and Morphological Variation
We observed variation in the morphology of P. acroporae hatchlings, so to investigate if there was variation within and between egg clusters, we collected all the hatchlings from three clusters reared at 27°C, made live observations of their swimming/crawling movements and subsequently fixed them in 4% paraformaldehyde (PFA) for sorting by morphological variation. To assess dispersal potential, we measured how long P. acroporae hatchlings could survive in the absence of coral (hatchling longevity) and whether temperature impacted this. Freshly laid egg clusters (using the egg collection method 2 described above) were reared in individual Petri dishes of filtered seawater (1 μm) incubated in tanks at 24, 27, or 30°C (±0.2°C), with five replicates per treatment. The 21°C treatment was excluded because of the poor hatching success rates in experiment 1. Hatchlings were collected as they emerged from their egg capsules and incubated in Petri dishes of filtered seawater at the temperature in which they were reared. Each Petri dish contained 10 hatchlings, which were collected at the same time and then monitored every 6 h to assess survival. Hatchlings were considered dead once they showed no signs of motion and failed to respond to a gentle stream of water from a plastic pipette, or when they failed to regulate their position in the water column following gentle centrifugal motion of their Petri dish. Once pronounced dead, they were examined in the subsequent monitoring period for confirmation. Longevity of each hatchling was expressed as the time elapsed from emergence from the egg capsules to death.
Experiment 3 – Time to Sexual Maturity and Size at Sexual Maturity
The time for P. acroporae to reach sexual maturity was assessed by determining the time between coral infestation with hatchlings to the first appearance of eggs on the coral skeleton (Figure 1). Uninfested coral fragments for this experiment were prepared from an A. millepora colony collected from Davies Reef (18°49′21.6′′ S 147°39′12.5′′ E; GBRMPA Permit No. G12/3236.1) in November 2017 and the experiment was conducted between February and April 2018. Egg clusters (27 clusters; nine per treatment) were collected from the culture on plastic strips and incubated and monitored using three treatment temperatures 24 ± 0.2, 27 ± 0.2, or 30 ± 0.2°C. A constant supply (0.2 L/min) of filtered seawater provided stable temperature and water quality within the 27, 1.5 L PVC infestation chambers. Each chamber housed one A. millepora fragment and one P. acroporae egg cluster. Aeration was provided to each infestation chamber to maintain water flow and was only reduced during hatching to facilitate P. acroporae recruitment. Each infestation chamber was placed in a group of three within temperature-controlled water baths, with replication to account for potential tank effects (i.e., three incubation aquaria per temperature; three infestation chambers per water baths). Before the addition of egg clusters, A. millepora fragments were acclimated to their assigned treatment within the experimental system with temperature change no greater than 0.8°C per week. Daily monitoring of egg clusters informed when hatching would occur; in the embryo pigmentation of the gut and development of five or more eye spots indicated imminent hatching. A section of coral tissue was removed with pressurized air (∼4 mm) to expose the coral skeleton and provide substrate for P. acroporae to deposit eggs. Each infestation chamber was fitted with 60 μm mesh ‘banjo filters’ on each chamber outlet to prevent loss of hatchlings when hatching was imminent. These filters were cleaned three to four times per day to remove biofouling. Egg capsules were checked twice daily (morning and evening) for hatching. The first day of hatching was considered day zero of time to reach sexual maturity. The exact numbers of fresh hatchlings were not examined, because this process would disrupt recruitment of P. acroporae to the host Acropora millepora fragment. Daily checks of the coral using a magnifying lens (SubSee +10 Diopter) were made to assess the progression of P. acroporae infestation (e.g., feeding scars) and look for the next generation of egg capsules. Egg deposition on the host coral was used as a proxy for the first attainment of sexual maturity in each cohort. Once eggs were observed, adult worms were collected by holding the infested coral over a 2 L Pyrex® bowl and removing them with streams of water from a ‘turkey baster.’ Each flatworm was measured using a ruler (to the nearest mm) and Olympus Tough camera to determine the mean size at sexual maturity at each temperature.
Statistical Analysis
Data were analyzed using RStudio (Version 1.0.143) for the influence of temperature on the embryonation period, hatching success, time to hatchling death, time to sexual maturity, and size at sexual maturity of P. acroporae. Normality was assessed using QQplot and Shapiro–Wilk tests. A linear mixed effects model [LME; R package “nmle” (Pinheiro et al., 2019)] was used to examine the influence of temperature on the time to sexual maturity (Shapiro–Wilk; p > 0.05). Because data from embryonation period and hatchling longevity experiments did not meet the assumption of normality and are time–to–event experiments, a time–to–event semi-parametric mixed effects Cox proportional hazards model [COXME; R package “survival” (Therneau, 2015)] was performed instead. Each model considered temperature fixed effect, and the cluster each egg capsule belonged to as a random effect, with significance level defined at p < 0.05. Adult length was also considered a fixed effect for embryonation period and hatching success data. Post hoc pairwise comparisons with Bonferroni correction were also performed for both LME [R package “emmeans” (Lenth, 2019)] and COXME (R package “survival”) analyses, to examine differences between temperature treatments.
Because this study aimed to provide predictions of how long flatworms take to hatch (embryonate) and reach sexual maturity at different temperatures, models were used to estimate these parameters from temperatures 21–30°C. Kaplan–Meier survival estimates of the Cox model (R package “survival” survfit function) were used to estimate the duration of the embryonation period (95% CI) at temperatures 21–30°C. Similarly, the linear mixed effects model was used to estimate time to sexual maturity at temperatures 21–30°C based on the relationship between temperature and rate of attaining sexual maturity.
A Chi-squared test was performed to investigate the influence of temperature on hatching success (temperature treatment vs. number of eggs hatched and unhatched), followed by independent pairwise comparisons between each treatment with subsequent Bonferroni adjustment to assess significant differences (p < 0.008) in hatching success between temperature treatments. Because of the non-normal distribution of size at sexual maturity data (Shapiro–Wilk; p < 0.05), a Kruskal–Wallis test was used to examine the influence of temperature on size at sexual maturity, and a Dunn test with Bonferroni adjustment examined differences between temperature treatment.
The life cycle generation time, or minimum time to re-infestation by sexually mature worms, was calculated as the sum of time taken for eggs to begin hatching and minimum time to sexual maturity. Hatchlings were considered to be able to infest coral immediately following hatching.
Results
Experiment 1 – Embryonation Period and Hatching Success
Temperature had a significant effect on the duration of the P. acroporae embryonation period [p < 0.0001; 1.76 ± 0.16 (coefficient ± SE); COXME; Figure 2C]. Pairwise comparison reaveals significant differences between all temperature treatments (p < 0.0001). At 21, 24, 27, and 30°C mean embryonation period for P. acroporae egg capsules was 26, 15, 11, and 9 days, respectively (Figure 2C and Table 1). The first and last day of capsule hatching in all egg clusters at a given temperature is shown in Figure 2C and Table 1. Predicted hatching probability curves using experimental embryonation data (Kaplan–Meier survival estimates) suggest that the embryonation period should range from 26 days at 21°C to only 9 days at 30°C (Table 2).
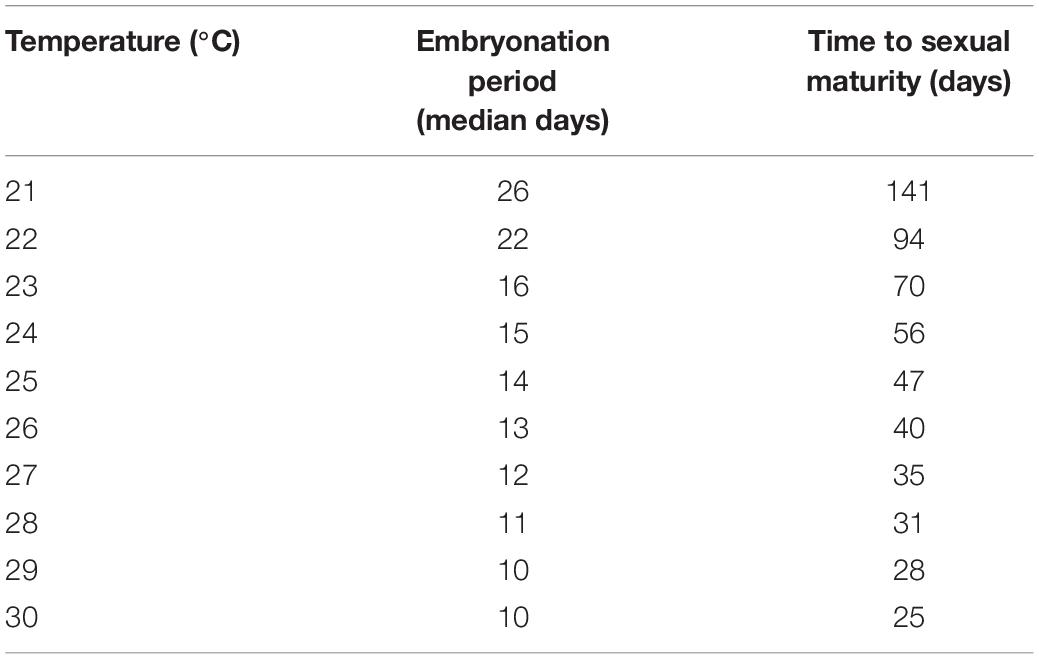
Table 2. Predicted time of embryonation from hatching probability curves using experimental embryonation data (Kaplan–Meier survival estimates), and time to sexual maturity (linear mixed effects model) in days.
Temperature also had a significant influence on the hatching success of P. acroporae from their egg capsules (p < 0.05, Chi-squared test; Figure 2C). Pairwise comparison demonstrated that the hatching success of worms from egg capsules in the 21°C treatment [43 ± 4% (mean ± SE)] was significantly lower (p < 0.008, Chi-squared test) than the other treatments (24, 27, 30°C). There were however no significant differences (p > 0.008) between the hatching success of temperature treatments of 24°C (80 ± 4%), 27°C (91 ± 2%), and 30°C (81 ± 3%) in any combination.
Across the total of 36 egg clusters examined in this experiment, the number of egg capsules that made up a cluster was variable with a mean of 14 ± 1.3 capsules (mean ± SE) and a range of 3 to 36. The number of embryos per capsule ranged from 1 to 5. Not all embryos within each egg cluster successfully hatched at all measured temperatures. We observed cases of incomplete embryonic development, oversized embryos (potentially too large to exit through the capsule operculum), or a general inability of the embryos to exit a given capsule. We considered an egg capsule to be successfully hatched when all viable individuals exited the egg capsule through the operculum.
Experiment 2 – Hatchling Longevity and Morphology
During embryogenesis, P. acroporae developed larval characters typical of other cotylean polyclads (i.e., lobes with ciliary tufts for swimming during a pelagic phase; Figure 3A). There was variation in hatchling morphology, which ranged from having reduced lobes to no lobes (Figure 3B), but all individuals were able to swim and crawl. This morphological variation was subtle and difficult to observe in live specimens due to the plasticity of their body shape. Fixation of the hatchlings enabled examination of the morphological variation within and between clusters and revealed that specimens that had reabsorbed their lobes still retained the ciliary tufts (Figure 3C). It also appeared that there may be variation between egg clusters laid by different parents as all hatchlings from one cluster had reduced lobes, whereas hatchlings from another had completely reabsorbed their lobes (Supplementary Figure 1). Even in fixed hatchlings, the degree of lobe reabsorption was a subtle and a continuous character that was difficult to score. Therefore, due to the lack of clear dimorphism, there was no attempt to quantify the variation in hatchling morphology. Instead, because all hatchlings could swim, we determined the longevity (or survivorship) of hatchlings as a measure of their dispersal potential. Hatchling survival ranged from 0.25 to 9 days in the absence of coral; the mean number of days (± SD) to death was 2 (± 2.12), 1 (± 0.52), and 2 (± 2.27) at 24, 27, and 30°C respectively (Table 1). Temperature did not have a significant influence on hatchling longevity (p > 0.05; COXME; Figure 3A). Pairwise comparison demonstrated significant differences between temperature treatments 24 and 27°C (p < 0.001), 27 and 30°C (p < 0.001), but none between 24 and 30°C (p < 0.001). Hatchling longevity was variable between individuals sourced from within a cluster and also between clusters (Figure 3D).
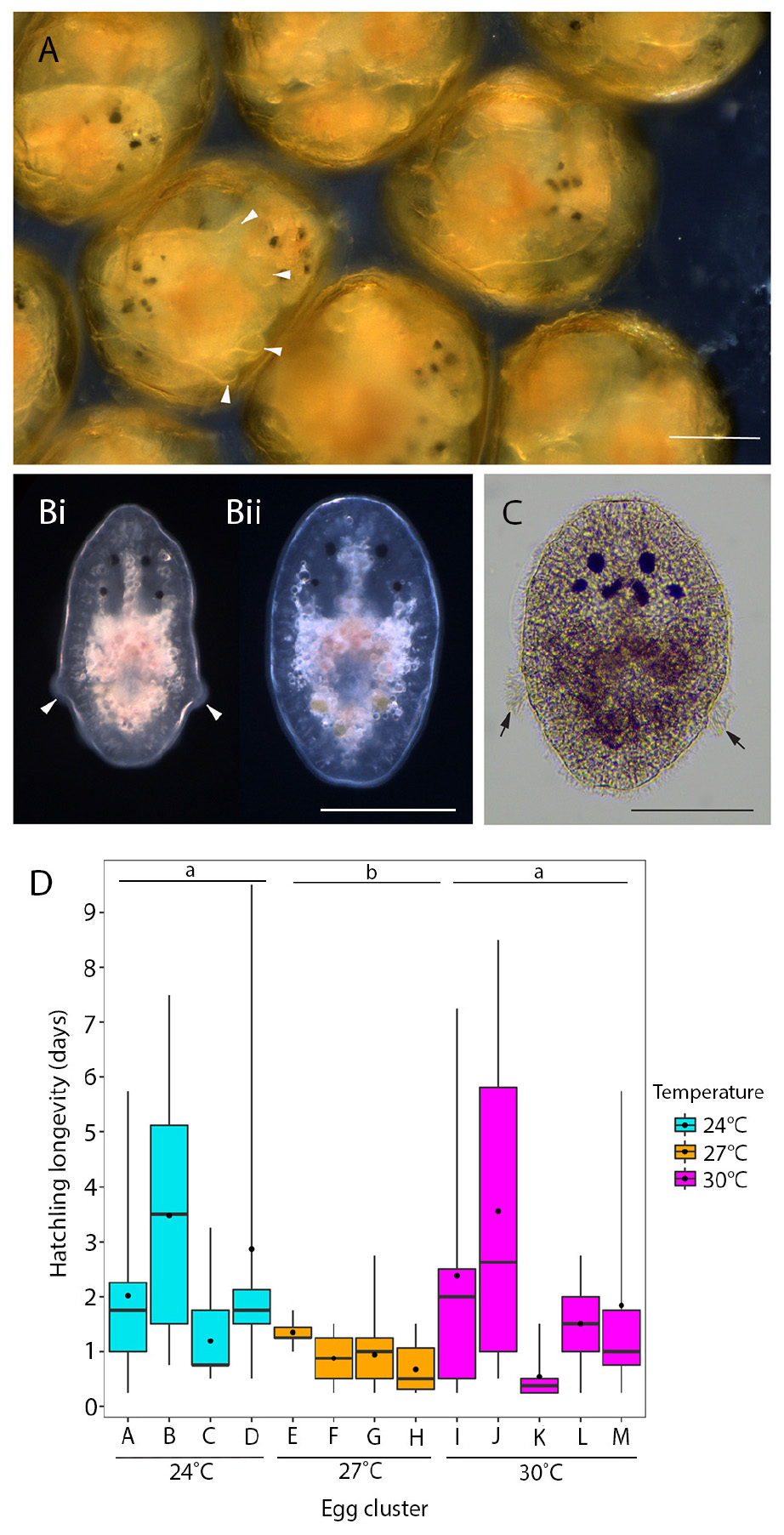
Figure 3. Hatchling morphology and longevity of P. acroporae. (A) Live embryos developing inside the egg capsule showing pronounced larval lobes (arrowheads). (B) At hatching these lobes are either much reduced (arrowheads) (Bi) or lost entirely (Bii) (live hatchlings). (C) Hatchlings that have lost their lobes retain the ciliary tufts (arrow; fixed specimen). Scale = 100 μm. (D) Hatchling longevity (the number of days a hatchling survives in the absence of coral) at 24°C (n = 64, 4 egg clusters), 27°C (n = 81 individuals, 4 egg clusters), and 30°C (n = 62, 5 egg clusters) (error bars = min. and max. values, boxes = lower and upper quartiles, line = median, and dot = mean). Overall temperature did not influence hatchling longevity (p > 0.05), but pairwise comparisons show significant differences between 24 and 27°C (p < 0.001) and 27 and 30°C (p < 0.001). Treatments with the same letter are not significantly different from each other (p > 0.05).
Experiment 3 – Time to Sexual Maturity and Size at Sexual Maturity
Temperature had a significant influence on the time for newly hatched P. acroporae to reach sexual maturity (p < 0.005, R2 = 0.7754, SE = 0.0126, LME; Figure 4A and Table 1). Pairwise comparison (emmeans) of rate of sexual maturity between temperature treatments revealed significant differences between 24 and 27°C (p < 0.005), 24 and 30°C (p < 0.005), but no significant difference between 27 and 30°C (p > 0.005). Predicted time to sexual maturity is up to 141 days at 21°C and as short as 26 days at 30°C (Table 2).
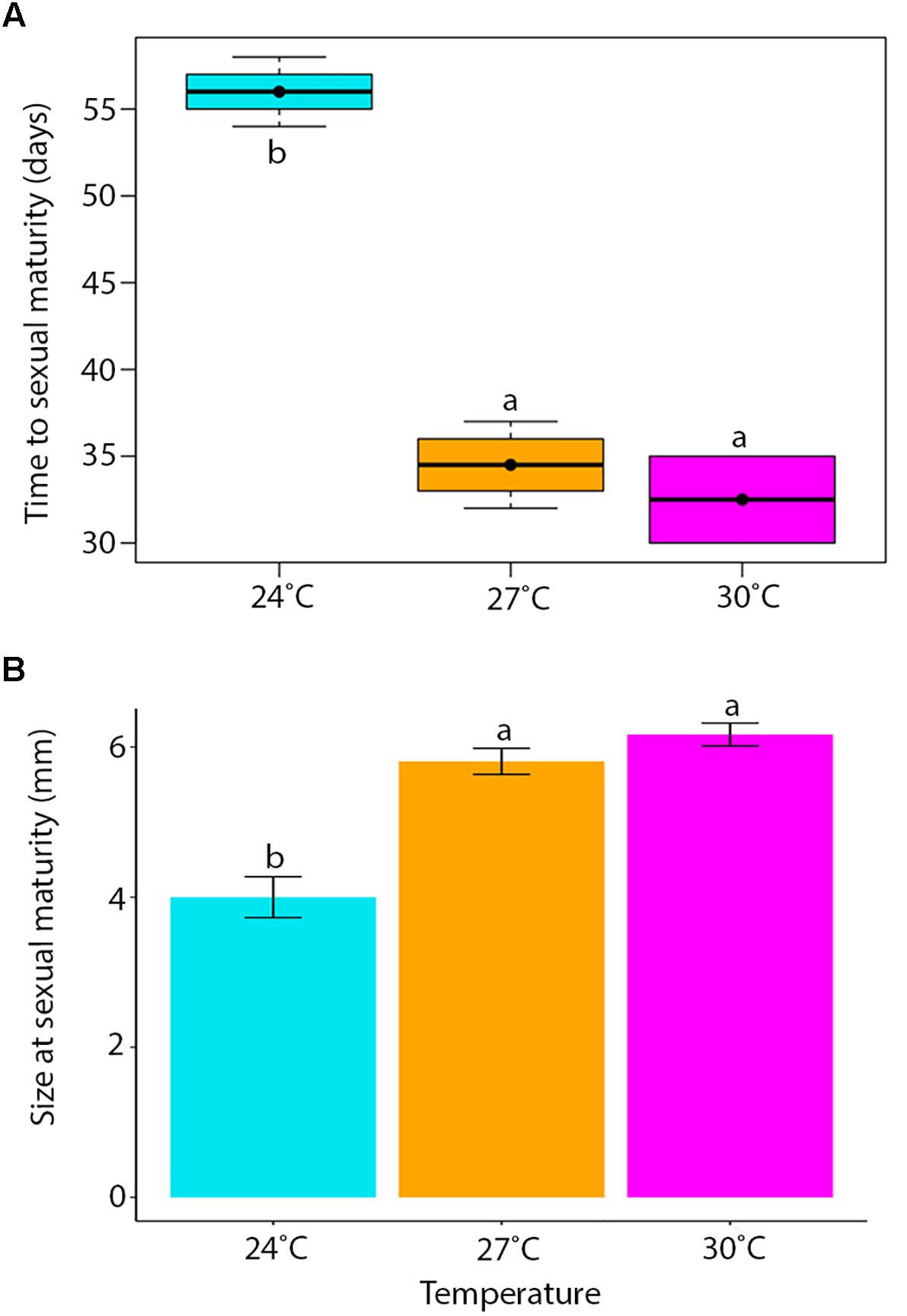
Figure 4. (A) The time for hatchlings to reach sexual maturity (error bars = min. and max. values, boxes = lower and upper quartiles, line = median, and dot = mean). (B) Mean body length (± SE) of individuals at sexual maturity. Treatment boxes and bars with the same letter are not significantly different from each other (p > 0.05).
One 27°C replicate flatworm resided on an unhealthy coral (bleached) and was considered an outlier in the data set, reaching sexual maturity at 58 days compared to 39.4 ± 4.97 days (mean ± SE) and was removed from the analysis. Results were limited by the number of replicates that housed sexually mature flatworms, specifically in the 30°C treatment where only two replicates had individuals that reached sexual maturity. One of these 30°C replicates had five flatworms at the time of oviposition of the first egg cluster (30 egg capsules), while the other fragment had only one flatworm when the first egg cluster was recorded (nine egg capsules).
Although successful initial settlement of hatchlings is not verifiable within our experimental design, it appears that low settlement occurred in the 30°C treatment, at least that which yielded symptoms of infestation. Eight of the nine replicate clusters (one cluster failed to hatch after appearing to complete development) successfully hatched at 30°C, but of these, six failed to infest their associated Acropora millepora fragments. At 25 days post-oviposition, no flatworms were associated with the remaining six replicates.
Temperature had a significant effect on total body length of P. acroporae, where individuals were larger at sexual maturity in warmer temperatures (Kruskal–Wallis, p < 0.001, F = 20.29; Figure 4B). Pairwise comparison revealed significant differences between temperature treatments 24 and 27°C (Dunn’s Test, p < 0.001), 24 and 30°C (p < 0.001), but not between 27 and 30°C (p > 0.001). The consequence was that flatworms reaching sexual maturity at warmer temperatures had total body lengths of 5.81 ± 0.82 and 6.12 ± 0.37 mm (mean ± SE) (27 and 30°C respectively) and were larger than flatworms in cooler conditions (24°C) with a body length of 4.00 ± 0.27 mm.
Discussion
Advances in Our Understanding of the Life Cycle of Prosthiostomum acroporae
The polyclad flatworm P. acroporae presents a serious problem to captive held Acropora corals, impacting their health in both hobbyist aquariums and large-scale coral aquaculture facilities. Effective management of this worm requires detailed understanding of its life cycle, and in this study we determined its embryonation period, hatching success, hatchling longevity, and time to sexual maturity across a range of biologically relevant temperatures (Berkelmans and Willis, 1999; Berkelmans and Van Oppen, 2006; Howells et al., 2013). To our knowledge, this is the first study to determine the timeline of a complete life cycle in a polyclad flatworm. The empirical data on life cycle parameters of Prosthiostomum montiporae allows us to calculate the generation time from oviposition of the parental generation to oviposition of the first generation. In comparison to other flatworms, a life cycle of 38 days for P. acroporae (at 27°C) is long compared to 10–13 days for Neobenedenia girellae (at 26 and 28°C) (Brazenor and Hutson, 2015), and 2–3 weeks for Macrostomum lignano at 20°C (Morris et al., 2004; Wudarski et al., 2019), but short compared to 80 days for the trematode flatworm Schistosoma mansoni at 28°C (in snail host and freshwater) and 37°C (in mammalian host) (Rawlinson, personal observation). This information has important implications for population numbers of P. acroporae and these these findings can be used to identify the timing of treatments to disrupt the life cycle of this coralivorous flatworm.
Interestingly, variation in the morphology of the hatchlings was observed with some hatching with lobes and ciliary tufts, and others hatching without lobes but with ciliary tufts. As this variation was subtle and continuous it may be due to variation in the timing of hatching, with hatchlings emerging at different timepoints during metamorphosis, during which lobes are reabsorbed and ciliary tufts are eventually shed (Kato, 1940; Ruppert, 1978). It does not appear to be a case of clear developmental dimorphism (or poecilogony) with distinct types of embryos within a cluster developing into either long-lived larvae with an obligate feeding period or short-lived larvae that can settle without feeding in the plankton (Krug, 2009). Our observations on fixed hatchlings also suggest that there may be variation between egg clusters, indicating either a parental effect or, more remotely, a case of cryptic species. However, as we only assessed variation in hatchling morphology in three clusters and at one temperature (27°C), a more extensive examination of variation within and between clusters is necessary to draw conclusions on the significance of this finding. Variation in hatchling morphology has interesting ecological, developmental and evolutionary consequences, with those hatchlings retaining lobes potentially able to swim further and for longer durations than those that have already reabsorbed their lobes. Many benthic marine invertebrates, that have a dispersive larval stage, develop tufts of long cilia for swimming during the pelagic phase; and their placement on lobes increases the volume of water moved per ciliary stroke relative to placement of cilia on a flat surface (Emlet, 1991). P. acroporae has retained the dominant life history strategy found in other cotylean polycads, i.e., indirect development via a larval form, but delays hatching until metamorphosis is almost complete. Our results suggest that as hatchlings are able to swim and have ciliary tufts (and some have lobes), there is potential to disperse between coral colonies. However since they are also able to crawl and can survive for up to 9 days (a relatively short time compared to hatchlings of other cotylean species, reviewed in Rawlinson, 2014) in the absence of coral, they could also be competent to settle given the right cues. Our development of life cycle rearing techniques and measurements of life cycle parameters provide a foundation for investigating if any genetic and/or epigenetic factors may influence time of hatching, and hatchling survivorship and dispersal.
Temperature Effects on the Life Cycle and the Timing of Treatments
It is common for poikilothermic animals to exhibit elevated developmental rates with increased temperatures (Howe, 1967; Hoegh-Guldberg and Pearse, 1995; Golizadeh et al., 2007; Wudarski et al., 2019). This phenomenon has historically been studied by entomologists to inform pest control methodology in agriculture and more recently for parasitic disease management in aquaculture (Tubbs et al., 2005; Brazenor and Hutson, 2015). Knowledge of timing of key life cycle stages at different temperatures for P. acroporae will increase the efficacy of treatment regimens to disrupt its life cycle and help advance coral husbandry practices. Currently, a variety of ‘dips’ (such as Levamisole HCl solutions (see Carl, 2008) and other commercial products) are used to treat infestations of P. acroporae in captivity. Here we suggest, that after an initial treatment for P. acroporae, the host colony must be treated again, to target new hatchlings after they emerge from their protective egg capsules. The second treatment should take place before offspring reach sexual maturity (Figure 5 and Table 2). For example, P. acroporae eggs in an aquarium operating at approximately 28°C would complete embryonation in approximately 11 days (Table 2). Assuming these hatchlings find a susceptible Acropora host, they would reach sexual maturity in approximately 31 days at 28°C (Table 2). Therefore, this Acropora colony should receive a second treatment between 13 (11 days embryonation period plus 1 or 2 days for settlement) and 31 days (approximate time to sexual maturity) following the first treatment (Figure 5). In this case (28°C) and at all temperatures, we recommend applying the second treatment at a time interval greater than the duration of embryonation, but less than the estimated time to sexual maturity. Importantly however, the present study did not evaluate the efficiency of any given chemical treatment to remove P. acroporae individuals, and therefore the suggested treatment strategy needs to be rigourously evaluated (see Carl, 2008). The effectiveness of these treatments can be enhanced by the mechanical removal of egg capsules using a scalpel or razor blade. If more than one coral colony in the aquarium system is infested, reinfestation is likely if all infested corals are not treated simultaneously. Although most P. acroporae hatchlings will starve within 2 days without any host material, the most resilient survive up to 9 days. Therefore it is recommended that treated corals be housed in an isolated quarantine tank between treatments, if possible, as this practice will give sufficient time for any P. acroporae hatchlings left in the infested system to starve. P. acroporae can lay their eggs on most hard substrates, which could foster reinfestation if Acropora tissue is still present.
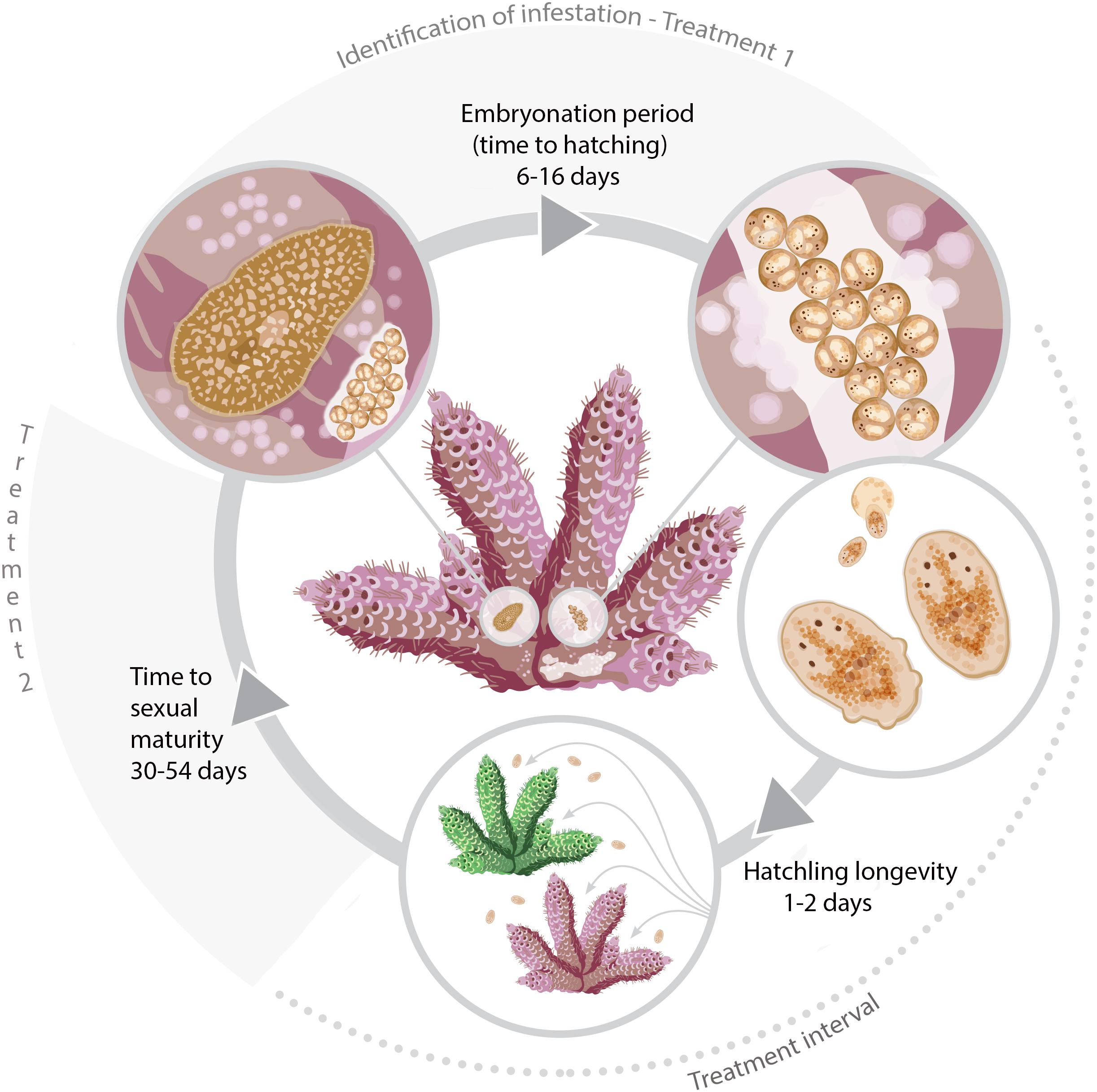
Figure 5. The life cycle of P. acroporae measured at 3°C increments from 24 to 30°C and the recommended timings of treatments (see section “Discussion”).
In addition to optimizing coral dipping treatments to disrupt the flatworm’s life cycle, our data could also be used to minimize P. acroporae numbers along the supply chain and to inform the duration of fallow “Acropora-free’ periods within a system. The relatively slow development and poor hatching success of P. acroporae in the 21°C treatment suggests that captive population numbers will be lower in cooler waters. Although lower temperatures could potentially be used to limit P. acroporae numbers (e.g., during transport of coral), temperature adjustments should primarily be based on the thermal tolerance of the Acropora colonies. Combining knowledge on the lower thermal tolerance of the Acropora species with our generation time predictions at different temperatures (Table 2) could allow a compromise temperature to be found that would minimize flatworm-related, and thermal, stress to the coral. Our data can also guide ‘fallow’ periods in a captive system; for example, by increasing the temperature of a system to 30°C and providing no live Acropora tissue, we would predict that any remaining egg capsules would hatch within 12 days and all subsequent hatchlings would be starved if held a further 9 days, equating to a 21 day fallow period. By contrast, at 24 degrees the same process would take 25 days (i.e., egg capsules hatch within 16 days and a 9 day hatchling starvation period).
Implications for Wild Population Numbers of Prosthiostomum acroporae
Investigating the life cycle of P. acroporae in captivity provides a foundation to understand how populations may fluctuate seasonally in the wild. Based on our findings, we would predict that shorter life cycles during the warmer months could lead to higher population densities of P. acroporae. In contrast, it appears that P. acroporae development slows considerably in cooler temperatures with reduced hatching success, indicating that wild population numbers may fluctuate considerably throughout the year. On Davies Reef, for example, the yearly average water temperature ranges from 29°C in December to 23°C in April2.
Increased sea surface temperatures (SSTs), attributed to climate change, already lead to thermal stress on Acropora populations (De’ath et al., 2012; Hughes et al., 2017). Thermal stress can predispose corals to higher rates of mortality from the feeding activity of corallivores [e.g., Drupella gastropod (Shaver et al., 2018)]. An increase in P. acroporae population density during prolonged thermal stress could exacerbate consequences to host Acropora colonies in a similar fashion, if flatworm populations aren’t controlled by their natural predators. Identifying (and conserving) the natural predators of P. acroporae could help limit numbers in captivity and the wild. In addition to any potential climate change related threats P. acroporae could pose, our data highlights a trade-off between growth and reproduction at different temperatures. In cooler temperatures, P. acroporae appears to sacrifice size to reach sexual maturity, possibly investing energy into reproduction over body size. While the ecological ramifications of reduced size are unknown, this phenotypic plasticity could be advantageous to survival in the temperature gradient along the GBR (21–30°C2; Berkelmans and Willis, 1999; Berkelmans and Van Oppen, 2006; Howells et al., 2013).
Conclusion
Warmer water temperatures lead to faster rates of development (pre- and post-hatching), shorter generation times and increased hatching success of the coralivorus flatworm P. acroporae. The data and models provided in this study detail the timelines for life cycle parameters at a range of biologically relevant temperatures, information critical to aquarists looking to disrupt the coralivorous flatworms’ life cycle. As the coral aquaculture trade grows, more effective management tools are required to control P. acroporae numbers in captive settings and this not only includes better targeted chemical treatments but also identifying natural predators of P. acroporae that are also suitable to captive conditions.
Data Availability
The datasets generated for this study are available on request to the corresponding author (Barton, 2019).
Author Contributions
JB, KH, DB, CH, CD, and KR conceived the research and designed the experiments. JB, KR, and CD performed the experiments. JB analyzed the data. JB and KR drafted the manuscript. All authors read, commented on, and agreed upon the content of the manuscript.
Conflict of Interest Statement
The authors declare that the research was conducted in the absence of any commercial or financial relationships that could be construed as a potential conflict of interest.
Funding
This study was funded by a James Cook University Development Grant, “Parasite cultivation techniques: in vitro and in vivo culture methods for ecological and applied aquatic parasitology research” awarded to KH. Additional funding to KR and CD was raised through crowdfunding on Experiment.com (https://doi.org/10.18258/1621) and a donation from the Atlanta Reef Club, Duluth, GA, United States.
Acknowledgments
The team at the National Sea Simulator (Australian Institute of Marine Science) and the Marine Parasitology Laboratory, James Cook University provided logistical support pivotal to the success of these experiments. Prof. Rhondda Jones and Sally Lau provided statistical support. Brett Bolte, Philip Jones, and Rachel Neil helped to maintain flatworm cultures. Jonathan Armitage built the first flatworm screening system. We thank Eden Cartwright (Bird Circus) for graphic art.
Supplementary Material
The Supplementary Material for this article can be found online at: https://www.frontiersin.org/articles/10.3389/fmars.2019.00524/full#supplementary-material
FIGURE S1 | Variation in Prosthiostomum acroporae hatchling morphology between two egg clusters at 27°C. (A) Hatchlings have reduced lobes (arrowheads); (B) hatchlings have re-absorbed their lobes (scale = 100 μm).
FIGURE S2 | Prosthiostomum acroporae screening method. (A) Photo of the entire screening system [Nally bin, 300-micron PVC screening chamber (arrow), and 20 L bucket]. (B) Inside the Nally bin, a jet of filtered seawater is used to physically remove Prosthiostomum acroporae from the surface of an Acropora colony. (C) 300 μm PVC screening chamber full of P. acroporae; with representative specimens circled. (D) >100 P. acroporae transferred into a plastic beaker for experimentation.
FIGURE S3 | To maintain Prosthiostomum acroporae culture numbers plastic was attached to coral using plastic pegs to provide susbtrate for egg-laying.
Footnotes
References
Barton, J. (2019). Data From: The Life Cycle of the Acropora Coral-Eating Flatworm (AEFW), Prosthiostomum acroporae. James Cook University (dataset). doi: 10.25903/5d5f8271c679c
Barton, J. A., Willis, B. L., and Hutson, K. S. (2017). Coral propagation: a review of techniques for ornamental trade and reef restoration. Rev. Aquacult. 9, 238–256. doi: 10.1111/raq.12135
Berkelmans, R., and Van Oppen, M. J. (2006). The role of zooxanthellae in the thermal tolerance of corals: a ‘nugget of hope’for coral reefs in an era of climate change. Proc. R. Soc. B Biol. Sci. 273, 2305–2312. doi: 10.1098/rspb.2006.3567
Berkelmans, R., and Willis, B. L. (1999). Seasonal and local spatial patterns in the upper thermal limits of corals on the inshore Central Great Barrier Reef. Coral Reefs 18, 219–228. doi: 10.1007/s003380050186
Brazenor, A. K., and Hutson, K. S. (2015). Effects of temperature and salinity on the life cycle of Neobenedenia sp.(Monogenea: Capsalidae) infecting farmed barramundi (Lates calcarifer). Parasitol. Res. 114, 1875–1886. doi: 10.1007/s00436-015-4375-5
Carl, M. (2008). “Predators and pests of captive corals,” in Advances In Coral Husbandry In Public Aquariums Public Aquarium Husbandry, Vol. 2, eds R. J. Leewis and M. Janse (Arnhem: Burgers’ Zoo), 31–36.
Cohen, F. P., Valenti, W. C., and Calado, R. (2013). Traceability issues in the trade of marine ornamental species. Rev. Fish. Sci. 21, 98–111. doi: 10.1080/10641262.2012.760522
Craggs, J., Guest, J. R., Davis, M., Simmons, J., Dashti, E., and Sweet, M. (2017). Inducing broadcast coral spawning ex situ: closed system mesocosm design and husbandry protocol. Ecol. Evol. 7, 11066–11078. doi: 10.1002/ece3.3538
De’ath, G., Fabricius, K. E., Sweatman, H., and Puotinen, M. (2012). The 27–year decline of coral cover on the Great Barrier Reef and its causes. Proc. Natl. Acad. Sci. 109, 17995–17999. doi: 10.1073/pnas.1208909109
Delbeek, J. C., and Sprung, J. (2005). The Reef Aquarium: Science, Art, and Technology. Miami Gardens, FL: Ricordea Publishing.
Doney, S. C., Fabry, V. J., Feely, R. A., and Kleypas, J. A. (2009). Ocean acidification: the other CO2 problem. Annu. Rev. Mar. Sci. 1, 169–192. doi: 10.1146/annurev.marine.010908.163834
Emlet, R. B. (1991). Functional constraints on the evolution of larval forms of marine invertebrates: experimental and comparative evidence. Am. Zool. 31, 707–725. doi: 10.1093/icb/31.4.707
Fabricius, K. E. (2005). Effects of terrestrial runoff on the ecology of corals and coral reefs: review and synthesis. Mar. Pollut. Bull. 50, 125–146. doi: 10.1016/j.marpolbul.2004.11.028
Fujita, R., Thornhill, D. J., Karr, K., Cooper, C. H., and Dee, L. E. (2014). Assessing and managing data-limited ornamental fisheries in coral reefs. Fish Fish. 15, 661–675. doi: 10.1111/faf.12040
Gammoudi, M., Noreña, C., Tekaya, S., Prantl, V., and Egger, B. (2012). Insemination and embryonic development of some Mediterranean polyclad flatworms. Invertebr. Reprod. Dev. 56, 272–286. doi: 10.1080/07924259.2011.611825
Golizadeh, A., Kamali, K., Fathipour, Y., and Abbasipour, H. (2007). Temperature-dependent development of diamondback moth, Plutella xylostella (Lepidoptera: Plutellidae) on two brassicaceous host plants. Insect Sci. 14, 309–316. doi: 10.1111/j.1744-7917.2007.00157.x
Hoegh-Guldberg, O., Mumby, P. J., Hooten, A. J., Steneck, R. S., Greenfield, P., Gomez, E., et al. (2007). Coral reefs under rapid climate change and ocean acidification. Science 318, 1737–1742. doi: 10.1126/science.1152509
Hoegh-Guldberg, O., and Pearse, J. S. (1995). Temperature, food availability, and the development of marine invertebrate larvae. Am. Zool. 35, 415–425. doi: 10.1093/icb/35.4.415
Howe, R. (1967). Temperature effects on embryonic development in insects. Annu. Rev. Entomol. 12, 15–42. doi: 10.1146/annurev.en.12.010167.000311
Howells, E. J., Berkelmans, R., van Oppen, M. J., Willis, B. L., and Bay, L. K. (2013). Historical thermal regimes define limits to coral acclimatization. Ecology 94, 1078–1088. doi: 10.1890/12-1257.1
Hughes, T. P., Kerry, J. T., Álvarez-Noriega, M., Álvarez-Romero, J. G., Anderson, K. D., Baird, A. H., et al. (2017). Global warming and recurrent mass bleaching of corals. Nature 543:373. doi: 10.1038/nature21707
Hume, B. C., D’Angelo, C., Cunnington, A., Smith, E. G., and Wiedenmann, J. (2014). The corallivorous flatworm Amakusaplana acroporae: an invasive species threat to coral reefs? Coral Reefs 33, 267–272. doi: 10.1007/s00338-013-1101-6
Krug, P. (2009). Not My “Type”: larval dispersal dimorphisms and bet-hedging in opisthobranch life histories. Biol. Bull. 216, 355–372. doi: 10.1086/bblv216n3p355
Lenth, R. (2019). Emmeans package: Estimated Marginal Means, aka Least-Squares Means. R package version 1.3.5.1. Available at: https://cran.r-project.org/web/packages/emmeans/emmeans.pdf (accessed July 1, 2019).
Litvaitis, M. K., Bolanos, D. M., and Quiroga, S. Y. (2019). Systematic congruence in Polycladida (Platyhelminthes, Rhabditophora): are DNA and morphology telling the same story? Zool. J. Linn. Soc. 20, 1–27. doi: 10.1093/zoolinnean/zlz007
Morris, J., Nallur, R., Ladurner, P., Egger, B., Rieger, R., and Hartenstein, V. (2004). The embryonic development of the flatworm Macrostomum sp. Dev. Genes Evol. 214, 220–239. doi: 10.1007/s00427-004-0406-4
Nosratpour, F. (2008). Observations of a polyclad flatworm affecting acroporid corals in captivity. Adv. Coral Husb. Public 2, 37–46.
Pinheiro, J., Bates, D., DebRoy, S., and Sarkar, D., and R Core Team (2019). nlme Linear and Nonlinear Mixed Effects Models. R package version 3.1-140. Available at: https://cran.r-project.org/web/packages/nlme/nlme.pdf (accessed April 14, 2019).
Pollock, F. J., Katz, S. M., van de Water, J. A., Davies, S. W., Hein, M., Torda, G., et al. (2017). Coral larvae for restoration and research: a large-scale method for rearing Acropora millepora larvae, inducing settlement, and establishing symbiosis. PeerJ 5:e3732. doi: 10.7717/peerj.3732
Rawlinson, K. A. (2014). The diversity, development and evolution of polyclad flatworm larvae. Evodevo 5:9. doi: 10.1186/2041-9139-5-9
Rawlinson, K. A., Gillis, J. A., Billings, R. E. Jr., and Borneman, E. H. (2011). Taxonomy and life history of the Acropora-eating flatworm Amakusaplana acroporae nov. sp. (Polycladida: Prosthiostomidae). Coral Reefs 30, 693–705. doi: 10.1007/s00338-011-0745-3
Rawlinson, K. A., and Stella, J. S. (2012). Discovery of the corallivorous polyclad flatworm, amakusaplana acroporae, on the great barrier reef, Australia - the first report from the wild. PLoS One 7:e42240. doi: 10.1371/journal.pone.0042240
Rhyne, A., Rotjan, R., Bruckner, A., and Tlusty, M. (2009). Crawling to collapse: ecologically unsound ornamental invertebrate fisheries. PLoS One 4:e8413. doi: 10.1371/journal.pone.0008413
Rhyne, A. L., Tlusty, M. F., and Kaufman, L. (2012). Long-term trends of coral imports into the United States indicate future opportunities for ecosystem and societal benefits. Conserv. Lett. 5, 478–485. doi: 10.1111/j.1755-263X.2012.00265.x
Rhyne, A. L., Tlusty, M. F., and Kaufman, L. (2014). Is sustainable exploitation of coral reefs possible? A view from the standpoint of the marine aquarium trade. Curr. Opin. Environ. Sustain. 7, 101–107. doi: 10.1016/j.cosust.2013.12.001
Rubec, P. J., and Cruz, F. P. (2005). Monitoring the chain of custody to reduce delayed mortality of net-caught fish in the aquarium trade. SPC Live Reef Fish Inform. Bull. 13, 13–23.
Ruppert, E. E. (1978). “A review of metamorphosis of turbellarian larvae,” in Settlement and Metamorphosis of Marine Invertebrate Larvae, F.-S. Chia, and M. E. Rice (New York, NY: Elsevier) 65, 82
Shaver, E. C., Burkepile, D. E., and Silliman, B. R. (2018). Local management actions can increase coral resilience to thermally-induced bleaching. Nat. Ecol. Evol. 2:1075. doi: 10.7924/G8348HFP
Therneau, T. (2015). A Package for Survival Analysis in S. version 2.38. Available at: https://cran.r-project.org/web/packages/survival/survival.pdf (accessed July 15, 2019).
Tlusty, M. F., Rhyne, A. L., Kaufman, L., Hutchins, M., Reid, G. M., Andrews, C., et al. (2013). Opportunities for public aquariums to increase the sustainability of the aquatic animal trade. Zoo Biol. 32, 1–12. doi: 10.1002/zoo.21019
Tubbs, L., Poortenaar, C., Sewell, M., and Diggles, B. (2005). Effects of temperature on fecundity in vitro, egg hatching and reproductive development of Benedenia seriolae and Zeuxapta seriolae (Monogenea) parasitic on yellowtail kingfish Seriola lalandi. Int. J. Parasitol. 35, 315–327. doi: 10.1016/j.ijpara.2004.11.008
Wabnitz, C. (2003). From Ocean to Aquarium: The Global Trade in Marine Ornamental Species. Nairobi: UNEP.
Keywords: AEFW, Acropora, flatworms, reef restoration, coral aquaculture, pest management
Citation: Barton JA, Hutson KS, Bourne DG, Humphrey C, Dybala C and Rawlinson KA (2019) The Life Cycle of the Acropora Coral-Eating Flatworm (AEFW), Prosthiostomum acroporae; The Influence of Temperature and Management Guidelines. Front. Mar. Sci. 6:524. doi: 10.3389/fmars.2019.00524
Received: 11 June 2019; Accepted: 12 August 2019;
Published: 04 September 2019.
Edited by:
António V. Sykes, University of Algarve, PortugalReviewed by:
Ricardo Calado, University of Aveiro, PortugalRonald Osinga, Wageningen University & Research, Netherlands
Evelyn Cox, University of Hawai‘i at Mānoa, United States
Copyright © 2019 Barton, Hutson, Bourne, Humphrey, Dybala and Rawlinson. This is an open-access article distributed under the terms of the Creative Commons Attribution License (CC BY). The use, distribution or reproduction in other forums is permitted, provided the original author(s) and the copyright owner(s) are credited and that the original publication in this journal is cited, in accordance with accepted academic practice. No use, distribution or reproduction is permitted which does not comply with these terms.
*Correspondence: Jonathan A. Barton, am9uYXRoYW4uYmFydG9uMUBteS5qY3UuZWR1LmF1; Kate A. Rawlinson, a3IxNkBzYW5nZXIuYWMudWs=