- International Fund for Animal Welfare, London, United Kingdom
Reducing speeds across shipping fleets has been shown to make a substantial contribution to effective short term measures for reducing greenhouse gas (GHG) emissions, working toward the goal adopted by the International Maritime Organization (IMO) in April 2018 to reduce the total annual GHG emission by at least 50% by 2050 compared to 2008. I review modeling work on GHG emissions and also on the relationships between underwater noise, whale collision risk and speed. I examine different speed reduction scenarios that would contribute to GHG reduction targets, and the other environmental benefits of reduced underwater noise and risk of collisions with marine life. A modest 10% speed reduction across the global fleet has been estimated to reduce overall GHG emissions by around 13% (Faber et al., 2017) and improve the probability of meeting GHG targets by 23% (Comer et al., 2018). I conclude that such a 10% speed reduction, could reduce the total sound energy from shipping by around 40%. The associated reduction in overall ship strike risk has higher uncertainty but could be around 50%. This would benefit whale populations globally and complement current efforts to reduce collision risk in identified high risk areas through small changes in routing.
Introduction
In April 2018, the International Maritime Organization (IMO) adopted an initial strategy on the reduction of greenhouse gas (GHG) emissions from ships. The target is to reduce the total annual GHG emissions by at least 50% by 2050 compared to 2008 values, while at the same time pursuing efforts toward phasing them out entirely. The strategy lists candidate short term measures to reduce GHG emissions in order to meet the agreed targets. IMO has developed the Energy Efficiency Design Index (EEDI) which requires that new ships become increasingly more energy efficient according to the year in which they were built. This is the only legally binding energy efficiency regulation for international shipping and only applies to new builds. This means that in order to reach the IMO 2050 target, measures for improving operational efficiency of existing ships will also be required. One element of the strategy is to “consider and analyze the use of speed optimization and speed reduction as a measure, taking into account safety issues, distance traveled, distortion of the market or to trade and that such measure does not impact on shipping’s capability to serve remote geographic areas” (IMO, 2018). It has been suggested that speed reduction is perhaps the only short-term regulatory option capable of achieving the necessary reductions in GHG emissions to meet IMO targets (CSC, 2017).
Here, I consider the implications of speed optimization and reduction not only for GHG emissions but for two further environmental impacts of shipping: ship strike risk to whales and underwater noise. Collisions between cetaceans and ships occur worldwide where vessel activities overlap with cetacean habitat. Collisions can cause damage to vessels and lead to injury and/or death of cetaceans. In response to this threat, the IMO issued guidance on minimizing the risk of ship strikes to cetaceans (IMO, 2009) in 2009. The impacts of underwater noise from shipping have also become increasingly recognized. IMO agreed to guidelines for the reduction of underwater noise from commercial shipping to address adverse impacts on marine life in 2014 (IMO, 2014).
I examine the implications of changes in vessel speeds at a global level, which is the scale most relevant to GHG emissions; however, ship strike risk and the impacts of underwater noise will depend on the spatial overlap between shipping and the distribution of sensitive species. The International Whaling Commission (IWC) has concluded that the only proven, effective mitigation measures to reduce ship strikes to whales are to avoid areas with known concentrations of whales, or reduce speed while transiting those areas (IWC, 2016). The IMO has recognized that small changes in routing are the most effective way to reduce ship strikes in identified high density whale areas (IMO, 2016). IWC (2016) lists the priority “high risk” areas identified by the IWC, and ongoing research will likely identify more of such areas. In some cases, speed restrictions have been put in place in specific areas where routing options are not possible (e.g., Seasonal Management Areas (SMAs)) on the east coast of United States for North Atlantic right whales; Hauraki Gulf in New Zealand for Bryde’s whales; approaches to the Panama Canal in conjunction with routing for humpback whales (IWC, 2016). However, most whale populations are widely dispersed, and distribution patterns are not predictable enough to allow routing measures. These situations would benefit from more general risk-reduction measures. The greatest impacts from underwater noise will also occur where there is most overlap between shipping and marine species that are particularly sensitive to underwater noise, but shipping has raised ambient noise levels across ocean basins (McDonald et al., 2006; Andrew et al., 2011; Miksis-Olds et al., 2013). Hence, most marine life will be affected to some extent and hence benefit from global measures that reduce noise output.
From a ship strike and noise perspective, speed optimization to minimize impacts could take into account the distribution of species known to be vulnerable to ship strikes or to be particularly sensitive to shipping noise, and be adjusted accordingly. However, this relies on data that are frequently not available, is operationally complex and also may conflict with optimization for other purposes including minimizing GHG emissions. For example, Doudnikoff and Lacoste (2014) showed that CO2 emissions will increase if ships slow down in certain areas and then increase speeds to compensate for the longer sailing time. Hence, the focus in this paper is on a simple assessment of global speed reductions where speeds are optimized with respect to total GHG emissions which will undoubtedly be the overriding concern from a legal and policy perspective. In contrast to mandatory energy efficiency requirements, guidelines related to ship strikes and underwater noise are entirely voluntary. Hence, I have taken proposals to reduce GHGs as a starting point and then examined the impacts of these for ship strike risk and underwater noise.
The 73rd meeting of the IMO Marine Environment Protection Committee (MEPC) in October 2018 considered speed reductions as a proportion of “business as usual” (BAU) speeds. This allows for the requirements of different shipping sectors with different operating requirements and vessel speeds. Vessel speeds will vary with ship type, with container ships and vehicle carriers generally having the highest speeds compared to oil tankers, bulkers, and general cargo (e.g., Bassett et al., 2012).
Faber et al. (2017) considered a range of speed reductions of 10, 20, and 30% compared to “business as usual” as well as the spare capacity within sectors to allow for the same volume of goods if more ships were required because of slower speeds. They note that in 2017, 3.5% of container vessels were idle or laid up and estimated that bringing these vessels back into service would allow the container fleet to reduce speeds by up to 8%. The equivalent figures for bulk carriers and tankers are 3 and 22%, respectively. Lee et al. (2015) developed an economic model which indicated that the savings in total fuel consumption associated with slower speeds were usually higher than the cost of operating the extra vessels required to transport equivalent goods. The growth in global fleet tonnage in 2017 was around 3.3%, and for the first time in recent years the expansion in ship supply capacity was surpassed by faster growth in demand and seaborne trade volumes (United Nations Conference on Trade and Development [UNCTAD], 2018). Thus, total excess capacity reduced slightly in 2017 across the global fleet. Speed reductions of greater than 10% would likely require a further increase in fleet capacity to meet current demand, but historic delivery rates of new vessels suggest that increases in fleet capacity could allow speed reductions as high as 20% or 30% for most ship types (Faber et al., 2017).
Materials and Methods
The first stage of analysis was to examine data on the current distribution of observed vessel speeds. These speed distributions were adjusted according to a number of possible future scenarios aimed at GHG reductions. The observed and adjusted speed distributions were then used to estimate the expected change in ship strike risk and underwater noise output associated with each scenario.
Assessment of Current Distribution of Vessel Speeds
Current vessel speeds were examined from Automatic Identification System (AIS) data. I examined a year of data from two areas, the main shipping lane across the Indian Ocean south of Sri Lanka as representative of long distance oceanic traffic, and a coastal area west of Greece in the Mediterranean as more representative of coastal traffic. These areas were chosen because of the availability and previous analyses of AIS data (Frantzis et al., 2014; Priyadarshana et al., 2016). For the Indian Ocean shipping lane, data were available from 2013/2014 to 2017/2018 for comparison. Vessel speeds were assessed within a period of 1 year for all vessels which crossed a line perpendicular to the shipping route between two waypoints at either side of the main route.
GHG Reduction Scenarios
Slow steaming practices introduced after a slowdown in global trade in 2008 prompted a number of studies of the economic implications and potential for GHG reductions (Cariou, 2011; Lindstad et al., 2011; Lee et al., 2015). Lindstad et al. (2011) found that emissions could be reduced by 19% if speeds were reduced to minimize costs and by 28% if speeds were further reduced but with no increase in costs. Lee et al. (2015) developed a model to quantify the relationship among shipping time, bunker cost and delivery reliability noting that delivery time reliability was an additional advantage of slow steaming. More recently, Mander (2017) found that additional policy measures might be required to ensure slow steaming persisted in the longer term. Between 2013 and 2015 there has been an increase in speed for some of the largest ships (ICCT, 2017).
Faber et al. (2017) estimated reductions in CO2 emissions for speed reductions of 10, 20, and 30% across the global fleet based on the assumptions that a ship’s main engine energy consumption per unit of time has a cubic relationship with its speed and that the efficiency of the auxiliary engines is not affected by speed reduction. They also allowed for the increase in the number of vessels in order to transport the same amount of cargo. However, their estimates did not include the CO2 emissions associated with an increase in ship construction due to demand for more vessels as a result of slower speeds. Previous work (Faber et al., 2012) found the emissions associated with such ship building to be sufficiently small (in the range from 4 to 6% of the emission reductions achieved by slow steaming) that they would not make an appreciable contribution to the estimates. Reduced port call times associated with increases in cargo handling efficiency can also allow for slower speeds for the same amount of cargo transported.
Comer et al. (2018) used the same proportional values of speed reduction (10, 20, 30%) as Faber et al. (2017) but combined these with estimates assuming different scenarios for timescales of new build, technical efficiency improvements and low-carbon fuel introduction. They then used a Monte-Carlo simulation to estimate the probability of meeting the IMO targets.
Ship Strike Risk
Speed reduction has been used as a measure to address ship strike risk in a number of locations (Silber et al., 2012). Speed restrictions to reduce ship strike risks to North Atlantic right whales were first introduced in SMAs off the east coast of the United States in 2008. In the 5 years after the enactment of mandatory 10 knot speed restrictions in several SMAs there were no right whale mortalities attributed to ship strikes either in, or close to these areas. These results indicate a statistically significant reduction in right whale ship strikes in the SMAs suggesting that the speed limits have been effective (Laist et al., 2014). A number of recent studies have also confirmed an increased ship strike risk with increased speed, supporting the use of speed restrictions as a way of reducing risk. Some studies have attempted to quantify the speed-risk relationship for specific whale species (Conn and Silber, 2013) or the hydrodynamic and impact forces in relation to speed (Silber et al., 2010). Others (e.g., Wiley et al., 2011; Chion et al., 2018) have evaluated the relative risk reduction that might be achieved by speed restrictions based on these speed-risk relationships. In addition to studies based on collisions, studies based on observations of whales close to vessels have inferred greater collision risks with increases in speed (Gende et al., 2011; Harris et al., 2012). However, there are still limited data to quantify the relationship between strike rates and vessel speed.
The probability of a fatal ship strike can be expressed as the probability that a strike will occur multiplied by the probability that it will ultimately be fatal (i.e., death or serious injury) given that it has occurred.
The relationship between these probabilities and vessel speed has been studied in most detail for North Atlantic right whales. Vanderlaan and Taggart (2007) estimated the probability of lethal injury with vessel speed at the time of impact (Mv), which was later updated by Conn and Silber (2013) with additional data. In that case Mv for speed v (in knots) was expressed as:
where β0 was estimated as −1.905 (SE = 0.821) and β1 as 0.217 (SE = 0.058).
Conn and Silber (2013) also estimated the relative instantaneous strike rate with speed. They expressed this in the form:
where λ is the instantaneous rate at which whales are struck. It was not possible to estimate α0 (which would have allowed an absolute estimate of strike rate), but α1 was estimated as 0.49 (SE = 0.09), giving a relative estimate of strike rate with speed. There was insufficient evidence to support a more complex quadratic effect with an additional parameter. Thus the formulation generates an exponential increase in strike rate with speed which becomes unrealistic at high speeds. In the analysis, 99% of observed ship speeds were 20.5 knots or below (P. Conn, personal communication). For the purposes of this study, and to avoid the estimates of risk being dominated by a small number of very fast vessels, I assume λ to be constant for speeds greater than 20 knots.
Conn and Silber (2013) then derived an expression for an index of the total mortality hazard based on the sum of the independent relative hazards associated with each transit through an area. The relative hazard for each individual transit is expressed as λvMvDv where Dv is the duration of the transit for vessel speed v. Thus Dv is proportion to 1/v.
In this case for a fixed number of vessel transits globally (i.e., a fixed amount of cargo transported) the equivalent global relative hazard (Hv) can be written as:
The dominant factor affecting the variance of estimates of Hv is uncertainty in λ. At 15 knots, the difference in λ between α1 ± one standard error (i.e., 0.40 or 0.58) is a factor of over 200. The 95% credibility interval for Mv is relatively narrow in comparison (see Conn and Silber, 2013, figure 3).
Thus, any estimates based on Hv need to be treated with caution. In addition these estimates were only made on the basis of strikes to North Atlantic right whales and may not be directly applicable to other species or populations. There is no reason to expect large differences between species in the severity of injury with speed in the event that a strike occurs, but the relationship between speed and strike rate is more likely to vary between species due to different responses to vessels, swimming speeds and ability to maneuver. However, these differences are difficult to predict. For the purposes of this study I use the estimates of Conn and Silber (2013) for North Atlantic right whales as indicative for all large whales, but note that the IWC Scientific Committee has identified the need for a better understanding of the relationship between vessel speed, the risk of death or injury to the whale and damage to the vessel (IWC, 2016).
Underwater Noise
Leaper et al. (2014) reviewed known data on the relationship between vessel speed and broadband source level and concluded that the power relationship suggested by Ross (1976), which was based on vessel noise measurements and cavitation experiments, was the most widely applicable. However, considerably more data have become available since that review.
Some studies have fitted a power relationship to empirical data to estimate the relationship between broadband source level and vessel speed. The difference in source level (ΔSL) can be expressed in terms of original speed v0, final speed v1, and estimated power exponent z by:
which just depends on the ratio v1/v0 and not on the original speed.
A recent study resulting in a large number of suitable measurements was associated with the voluntary slow down program initiated by the Vancouver Fraser Port Authority as part of the Enhancing Cetacean Habitat and Observation (ECHO) program. As part of this program MacGillivray and Li (2018) obtained estimates of z by ship type from a total of 2765 source level measurements including before and after the slow down trial. For broadband monopole source levels, estimates of z varied from 5.1 (containerships and vehicle carriers) to 8.1 (bulkers). Other estimates of z are 4.5 from data in Allen et al. (2012) from fishing vessels and a model from Wittekind (2014) which suggests z = 8 for low frequency propeller noise above cavitation inception speed.
Others (McKenna et al., 2013; Simard et al., 2016; Veirs et al., 2016; Gassmann et al., 2017) have estimated the slope m of a linear regression where:
In this case ΔSL will depend on the actual value of the speeds as well as the ratio. Estimated values of m have ranged from 0.93 (Veirs et al., 2016) to 2.38 Gassmann et al. (2017). Veirs et al. (2016) used a linear regression on a large data set to obtain a slope of 0.93 dB/knot for broadband source level with speed, but they note that most of the variation in SL is likely driven by ship class (which was not controlled for in the regression), with little change in speed within ship class.
All these relationships of noise with speed only apply to vessels with fixed pitch propellers. Substantial cavitation can occur on controllable pitch propellers when operating at slower speeds resulting in higher noise levels. However, vessels with controllable pitch propellers are only a very small proportion of the global fleet (e.g., tugs, ferries).
Leaper et al. (2014) define the acoustic footprint of a vessel as the area of sea for which the source level will be above a given value (which can be defined in terms of energy or pressure). For situations of spherical spreading (20 logR) propagation loss, the ratio A1/A0 of acoustic footprint associated with a change in source level of ΔSL dB is given by:
where A0 is the original acoustic footprint for SL0 and A1 is the footprint associated with SL1 where SL1 = SL0 + Δ SL.
The ratio of acoustic footprints in this case is also the same as the ratio of total sound energy. For slower vessels and longer passage times there will need to be more vessels at sea to carry the equivalent amount of cargo. If all vessels travel at a fraction k of their former speed (i.e., k = v1/v0) then the number of vessels, and the associated acoustic footprints, need to be multiplied by 1/k for the equivalent cargo carried.
For the purposes of this analysis, I summarize the effects of changes in vessel speed in terms of the ratio of sound energy for equivalent cargo carried. The assumption of spherical spreading loss also seems the most appropriate general approximation to apply at a global scale. In different situations propagation loss may be more or less than 20 logR (see Ainslie et al., 2014). Thus, the ratios given can also be visualized in terms of acoustic footprint which will also be roughly proportional to the number of animals affected.
Results
Vessel Speeds
Vessel speeds vary between areas and routes depending on the nature of the traffic. The Indian Ocean route south of Sri Lanka is typical of long distance routes, whereas coastal traffic is more variable. In most cases the distribution is bimodal. This is more pronounced in the coastal traffic example (Figure 1), but the offshore traffic can also be summarized as the sum of two symmetrical approximately normal distributions of “slow” and “fast” vessels (Figure 2). In this case the fast vessel category is dominated by container ships. Median speed for slow vessels was around 13 knots and for the fast vessel category, 18 knots. Median speed for large container ships of the major carriers was 18.4 knots. There were only small changes in median speeds between 2013 (13.7 knots) and 2017 (13.3 knots), with a smaller proportion of vessels in the 16–20 knot range in 2017 and an increase in the 12–14 knot proportion. There were 2308 transits where the same vessel (based on MMSI number) transited at least once in both 2013 and 2017. No significant difference was detected in the speeds of those vessels between years (ANOVA, p = 0.61).
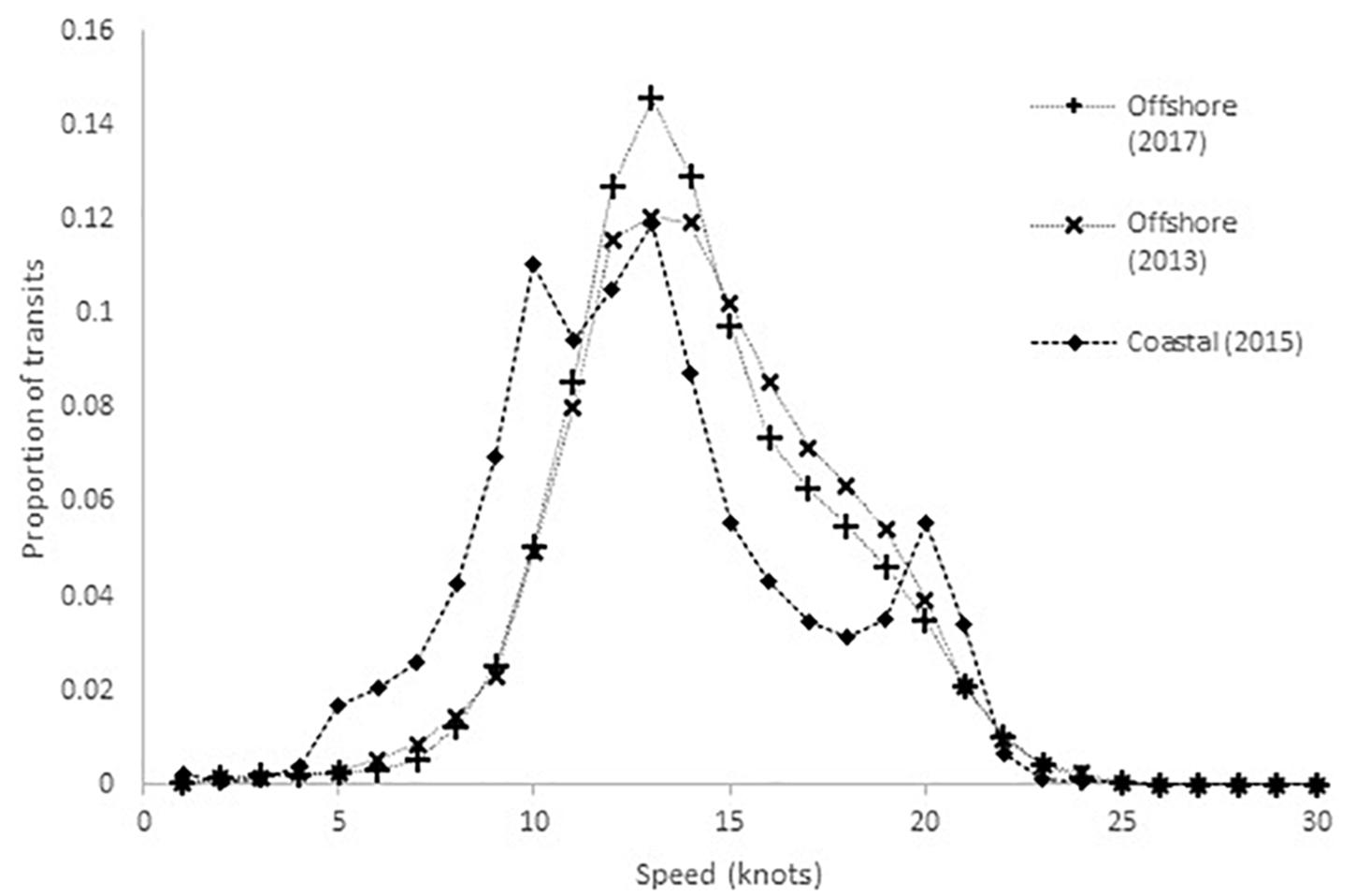
Figure 1. Proportion of transits by speed for offshore traffic (crossing Indian Ocean south of Sri Lanka) and coastal traffic in the eastern Ionian, Mediterranean.
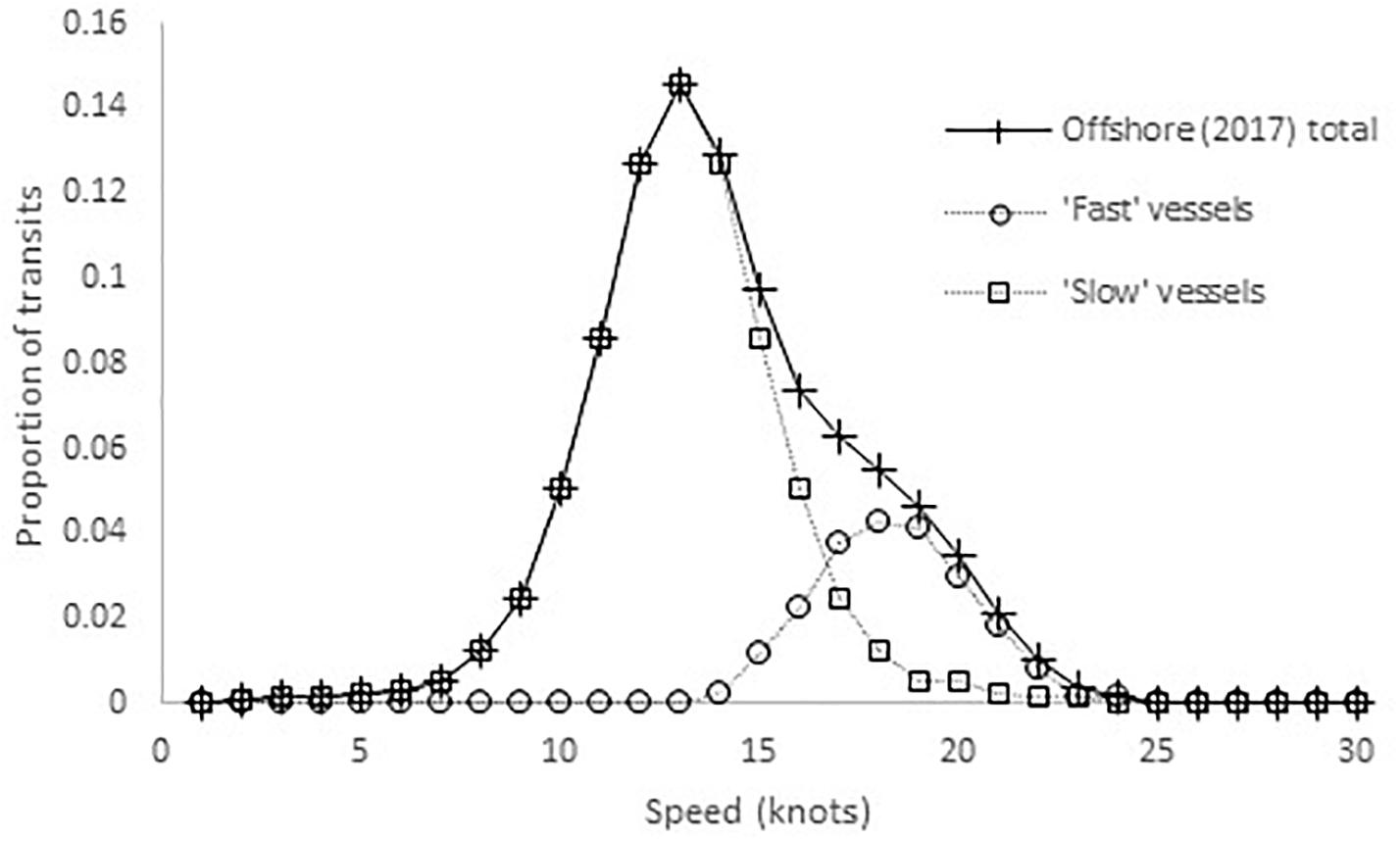
Figure 2. Distribution of offshore vessel speeds in 2017 shown as the sum of two approximately symmetrical distributions representing “fast” and “slow” vessels.
The peak in slower vessels in the Mediterranean is dominated by recreational craft, many of which now voluntarily transmit AIS signals.
Ship Strike Risk
Ten knots has been considered as the speed at which strike risk has dropped to low levels, supported by the reduction in ship strike cases following the introduction of SMAs on the east coast of the US (Laist et al., 2014). Currently, only a very small proportion (<10%) of transits occur at speeds of less than 10 knots for the oceanic traffic. This would increase to around 60% if all vessels slowed by 30% (Table 1).
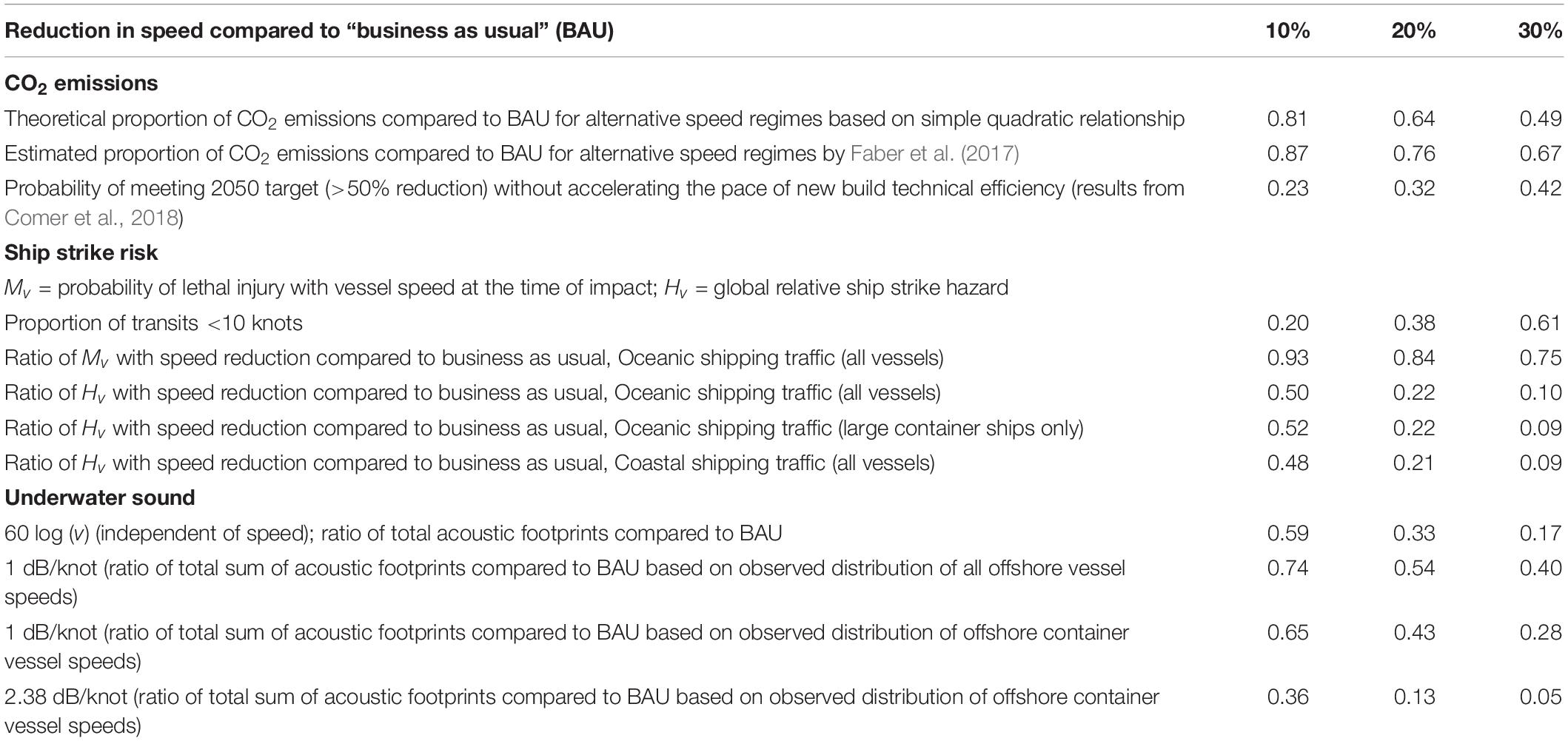
Table 1. Changes in parameters for scenarios of 10–30% speed reductions across the global fleet adjusted for the same total cargo carried.
The estimates of risk reduction associated with speed reductions of 10, 20, and 30% are shown in Table 1. These results are most sensitive to the assumptions about the relationship between strike rate and speed, for which there is the most uncertainty and also likely considerable variation between species. The estimates were all relatively insensitive to the overall distribution of speeds for the sector of the fleet being considered. For example, values of Hv for a speed reduction of 10% were between 0.48 and 0.52.
Underwater Noise
Studies that estimated relationships between source level and speed were divided into two categories: those of the form in Eq. 4 (a simple power relationship) and those of the form in Eq. 5 (a linear regression of source level expressed in dB on speed). These are shown in Figure 3 for reference speeds of 15 and 20 knots for the relationships dependent on the original speed. For the case of a simple power relationship in Eq. 4 between source level and vessel speed, the ratio of sound energy associated with a proportional reduction in speed will be the same for all speeds and so can be estimated across the global fleet regardless of the original speed distribution.
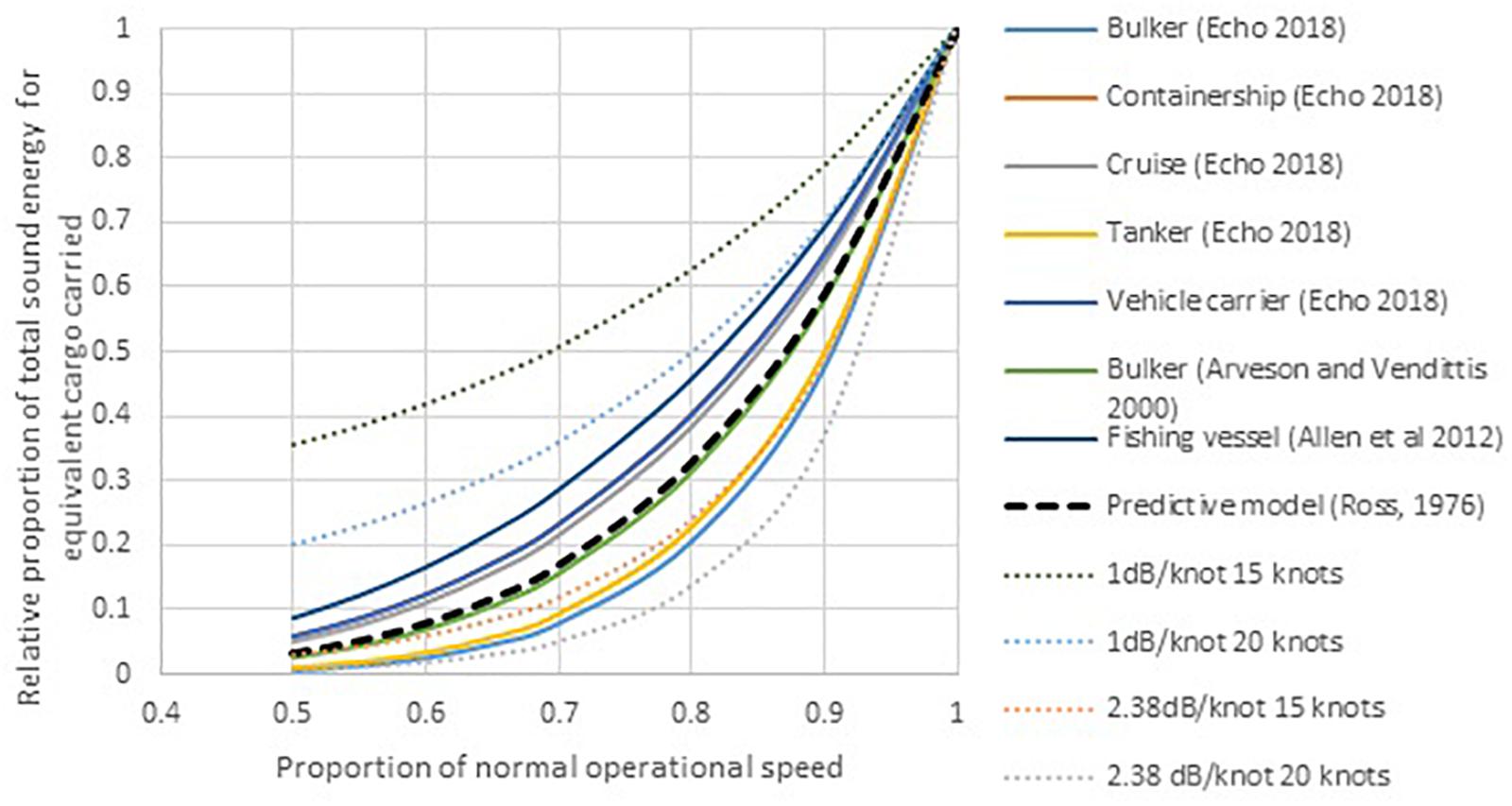
Figure 3. Relative proportion of total sound energy [also equivalent to the relative total acoustic footprint as defined by Leaper et al. (2014)] from a number of studies. Dotted lines indicate relationships that are dependent on the initial speed. The dashed line indicates z = 6 in Eq. 4.
It can be seen that the model of Ross (1976) with z = 6 in Eq. 4 falls in the middle of the more recent empirical studies. The estimates of global proportion of acoustic footprint associated with speed reductions are shown in Table 1 for this model. A 1 dB/knot relationship would suggest a substantially lower reduction across the global fleet than the Ross model, but a closer level to the Ross model for just container vessels because of their higher speeds. Table 1 also shows the reduction associated with the 2.38 dB/knot relationship found by Gassmann et al. (2017) for a specific class of container vessel. This shows the greatest reduction in acoustic footprint, down to 5% of the initial value for a speed reduction of 30%.
Discussion
Several studies have shown that some form of speed reduction will be essential in the short-term if IMO targets on GHGs are to be met, and the IMO has identified speed reduction as a candidate short-term measure. I have attempted a simple quantification of the additional environmental benefits associated with slower speeds motivated by a reduction in GHGs of reduced ship strike risk to whales and underwater noise. These additional benefits further support the calls for effective measures to reduce speeds.
For ship strikes, there are many studies indicating a qualitative risk reduction with slower speeds but limited data are available to quantify the relationship. Only one study has attempted to do this and only for one species. There is therefore considerable uncertainty with these estimates. Nevertheless, the results indicate the potential for a 50% reduction in risk for a modest 10% reduction in global shipping speeds. The uncertainty in the risk reduction achieved means that, where it is possible to separate ships and whales by small changes in routing, this would still be the option most likely to be effective, as noted by IMO (2016).
In recent years, there has been a substantial increase in concern over the impacts of underwater noise and increased research effort including source characteristics of individual vessels (IWC, 2018). These recent studies have given a much more comprehensive assessment of the relationships between source levels and speed. Many of these studies have shown that for individual vessels, the relationship varies considerably with the characteristics of the vessel (e.g., Kellett et al., 2013; Putland et al., 2017), though consistently, slower speeds produce less noise in fixed pitch propellers. Estimates that could apply to the fleet as a whole also show a wide variation (as shown in Figure 3) but support the continued use of the model of Ross (1976) which has been used for some decades and falls in the middle of the more recent observations. This model indicates a 10% reduction in speed would cut global underwater sound energy from shipping by around 40%.
Author Contributions
RL conceived the study, performed the analysis, and wrote the manuscript.
Funding
This study was supported by the International Fund for Animal Welfare (IFAW).
Conflict of Interest Statement
The authors declare that the research was conducted in the absence of any commercial or financial relationships that could be construed as a potential conflict of interest.
Acknowledgments
The author would like to thank Marine Traffic for making the AIS data for this study available and also thank Susannah Calderan, John Maggs, Lindy Weilgart and the reviewers for their comments which greatly improved an earlier draft.
References
Ainslie, M. A., Dahl, P. H., de Jong, C. A. F., and Laws, R. M. (2014). “Practical spreading laws: the snakes and ladders of shallow water acoustics,” in Proceedings of UA2014-2nd International Conference and Exhibition on Underwater Acoustics, 879–886. Available at: http://www.marinecontech.com/content/uploads/2015/01/UA2014_133.pdf (accessed August 6, 2019).
Allen, J. K., Peterson, M. L., Sharrard, G. V., and Wright, D. L. (2012). Radiated noise from commercial ships in the Gulf of Maine: implications for whale/vessel collisions. J. Acoust. Soc. Am 132, EL229–EL235. doi: 10.1121/1.4739251
Andrew, R. K., Howe, B. M., and Mercer, J. A. (2011). Long-time trends in ship traffic noise for four sites off the North American West Coast. J. Acoust. Soc. Am 129, 642–651. doi: 10.1121/1.3518770
Bassett, C., Polagye, B., Holt, M., and Thomson, J. (2012). A vessel noise budget for Admiralty Inlet, Puget Sound, Washington (USA). J. Acoust. Soc. Am. 132, 3706–3719. doi: 10.1121/1.4763548
Cariou, P. (2011). Is slow steaming a sustainable means of reducing CO2 emissions from container shipping? Transp. Res. Part D 16, 260–264. doi: 10.1016/j.trd.2010.12.005
Chion, C., Turgeon, S., Cantin, G., Michaud, R., Ménard, N., Lesage, V., et al. (2018). A voluntary conservation agreement reduces the risks of lethal collisions between ships and whales in the St. Lawrence Estuary (Québec, Canada): from co-construction to monitoring compliance and assessing effectiveness. PLoS One 13:e0202560. doi: 10.1371/journal.pone.0202560
Comer, B., Chen, C., and Rutherford, D. (2018). “Relating short-term measures to IMO’s minimum 2050 emissions reduction target,” in Appendix to paper MEPC 73/INF.27 presented to IMO Marine Environment Protection Committee. 73rd session, (London).
Conn, P. B., and Silber, G. K. (2013). Vessel speed restrictions reduce risk of collision-related mortality for North Atlantic right whales. Ecosphere 4:art43. doi: 10.1890/ES13-00004.1
CSC. (2017). “Global speed management as an important component of an ambitious initial IMO GHG Strategy,” in Paper ISWG-GHG 2/2/16 presented to IMO Working Group on Reduction of GHG Emissions from Ships. 2nd session, (London).
Doudnikoff, M., and Lacoste, R. (2014). Effect of a speed reduction of containerships in response to higher energy costs in Sulphur Emission Control Areas. Transp. Res. Part D Transp. Environ. 28, 51–61. doi: 10.1016/j.trd.2014.03.002
Faber, J., Huigen, T., and Nelissen, D. (2017). Regulating speed: a Short-term Measure to Reduce Maritime GHG Emissions. Netherlands: CE Delft publication.
Faber, J., Nelissen, D., Hon, G., Wang, H., and Tsimplis, M. (2012). Regulated Slow Steaming in Maritime Transport: An Assessment of Options, Costs and Benefits. Delft: CE Delft.
Frantzis, A., Leaper, R., Paraskevi, A., and Lekkas, D. (2014). “Distribution patterns of sperm whales in relation to shipping density in the Hellenic Trench, Greece,” in 11pp. Paper SC/65b/HIM07 presented to IWC Scientific Committee, (Bled Slovenia).
Gassmann, M., Kindberg, L. B., Wiggins, S. M., and Hildebrand, J. A. (2017). Underwater Noise Comparison of Pre- and Post-retrofitted MAERSK G-class Container Vessels CA MPL TM-616. La Jolla: Scripps Institution of Oceanography.
Gende, S. M., Hendrix, A. N., Harris, K. R., Eichenlaub, B., Nielsen, J., and Pyare, S. (2011). A Bayesian approach for understanding the role of ship speed in whale-ship encounters. Ecol. Appl. 21, 232–240.
Harris, K. R., Gende, S. M., Logsdon, M. G., and Klinger, T. (2012). Spatial pattern analysis of cruise ship humpback whale interactions in and near Glacier Bay National Park. Env. Manag. 49, 44–54. doi: 10.1007/s00267-011-9754-9
ICCT (2017). Greenhouse Gas Emissions from Global Shipping, 2013-2015. Washington, D.C: International Council on Clean Transportation.
IMO (2014). “IMO MEPC.1/Circ.833 Guidelines for the reduction of underwater noise from commercial shipping to address adverse impacts on marine life,” in Proceedings of the 21st ASCOBANS Advisory Committee Meeting, (Gothenburg).
IMO (2018). “Resolution MEPC.304(72),” in Initial IMO Strategy on Reduction of GHG Emissions from Ships, (London: IMO),
IWC (2016). “Information on recent outcomes regarding minimizing ship strikes to cetaceans,” in Paper MEPC 69-10-3 Submitted to IMO MEPC, 69th Session, (London).
IWC (2018). “Further information related to impacts of underwater noise on marine life,” in Paper MEPC 72-inf. Submitted to IMO MEPC, 72nd Session, (London).
Kellett, P., Turan, O., and Incecik, A. (2013). A study of numerical ship underwater noise prediction. Ocean Eng. 66, 113–120. doi: 10.1016/j.envpol.2019.06.049
Laist, D., Knowlton, A., and Pendleton, D. (2014). Effectiveness of mandatory vessel speed limits for protecting North Atlantic right whales. Endanger. Species Res. 23, 133–147. doi: 10.3354/esr00586
Leaper, R., Renilson, M. R., and Ryan, C. (2014). Reducing underwater noise from large commercial ships: current status and future directions. J. Ocean Technol. 9, 50–69.
Lee, C.-Y., Lee, H. L., and Zhang, J. (2015). The impact of slow ocean steaming on delivery reliability and fuel consumption. Transp. Res. Part E Logist. Transp. Rev. 76, 176–190. doi: 10.1016/j.tre.2015.02.004
Lindstad, H., Asbjørnslett, B., and Strømman, A. (2011). Reductions in green house gas emissions and cost by shipping at lower speeds. Energy Policy 39, 3456–3464. doi: 10.1016/j.enpol.2011.03.044
MacGillivray, A., and Li, Z. (2018). Vessel Noise Measurements from the ECHO Slowdown Trial: Final Report. Document 01518, Version 3.0. Technical Report by JASCO Applied Sciences for Vancouver Fraser Port Authority ECHO Program. Victoria, BC: JASCO Applied Sciences.
Mander, S. (2017). Slow steaming and a new dawn for wind propulsion: a multi-level analysis of two low carbon shipping transitions. Mar. Policy 75, 210–216. doi: 10.1016/j.marpol.2016.03.018
McDonald, M. A., Hildebrand, J., and Wiggins, S. M. (2006). Increases in deep ocean ambient noise in the northeast Pacific west of San Nicolas Island. California. J. Acoust. Soc. Am. 120, 711–718. doi: 10.1121/1.2216565
McKenna, M. F., Wiggins, S. M., and Hildebrand, J. A. (2013). Relationship between container ship underwater noise levels and ship design, operational and oceanographic conditions. Sci. Rep. 3, 1760.
Miksis-Olds, J. L., Bradley, D. L., and Maggie Niu, X. (2013). Decadal trends in Indian Ocean ambient sound. J. Acoust. Soc. Am. 134, 3464–3475. doi: 10.1121/1.4821537
Priyadarshana, T., Randage, S. M., Alling, A., Calderan, S., Gordon, J., Leaper, R., et al. (2016). Distribution patterns of blue whale (Balaenoptera musculus) and shipping off southern Sri Lanka. Reg. Stud. Mar. Sci. 3, 181–188. doi: 10.1016/j.rsma.2015.08.002
Putland, R. L., Merchant, N. D., Farcas, A., and Radford, C. A. (2017). Vessel noise cuts down communication space for vocalizing fish and marine mammals. Global. Chang. Biol. 7, 5713. doi: 10.111/gcb.13966
Silber, G. K., Slutsky, J., and Bettridge, S. (2010). Hydrodynamics of a ship/whale collision. J. Exp. Mar. Biol. Ecol. 391, 10–19. doi: 10.1016/j.jembe.2010.05.013
Silber, G. K., Vanderlaan, A. S. M., Arceredillo, A. T., Johnson, L., Taggart, C. T., Brown, M. W., et al. (2012). The role of the International maritime organization in reducing vessel threat to whales: process, options, action and effectiveness. Mar. Policy 36, 1221–1233. doi: 10.1016/j.marpol.2012.03.008
Simard, Y., Roy, N., Gervaise, C., and Giard, S. (2016). Analysis and modeling of 255 source levels of merchant ships from an acoustic observatory along St. Lawrence Seaway. J Acoust Soc Am 140:2002. doi: 10.1121/1.4962557
United Nations Conference on Trade and Development [UNCTAD] (2018). Review of Maritime Transport. Available at: http://unctad.org/en/PublicationsLibrary/rmt2018_en.pdf (accessed August 6, 2019).
Vanderlaan, A. S. M., and Taggart, C. T. (2007). Vessel collisions with whales: the probability of lethal injury based on vessel speed. Mar. Mammal. Sci. 23, 144–156. doi: 10.1111/j.1748-7692.2006.00098.x
Veirs, S., Veirs, V., and Wood, J. D. (2016). Ship noise extends to frequencies used for echolocation by endangered killer whales. Peer J 4:e1657. doi: 10.7717/peerj.1657
Wiley, D. N., Thompson, M., Pace, R. M., and Levenson, J. (2011). Modelling speed restrictions to mitigate lethal collisions between ships and whales in the Stellwagen Bank National Marine Sanctuary. U.S.A. Biological. Conservation 144, 2377–2381. doi: 10.1016/j.biocon.2011.05.007
Keywords: speed, GHG, emissions, underwater noise, ship strike
Citation: Leaper R (2019) The Role of Slower Vessel Speeds in Reducing Greenhouse Gas Emissions, Underwater Noise and Collision Risk to Whales. Front. Mar. Sci. 6:505. doi: 10.3389/fmars.2019.00505
Received: 24 January 2019; Accepted: 29 July 2019;
Published: 16 August 2019.
Edited by:
David Peel, Commonwealth Scientific and Industrial Research Organisation (CSIRO), AustraliaReviewed by:
Mark Peter Simmonds, University of Bristol, United KingdomPiers Dunstan, CSIRO Oceans and Atmosphere, Australia
Copyright © 2019 Leaper. This is an open-access article distributed under the terms of the Creative Commons Attribution License (CC BY). The use, distribution or reproduction in other forums is permitted, provided the original author(s) and the copyright owner(s) are credited and that the original publication in this journal is cited, in accordance with accepted academic practice. No use, distribution or reproduction is permitted which does not comply with these terms.
*Correspondence: Russell Leaper, cnVzc2VsbEBpdnl0LmRlbW9uLmNvLnVr