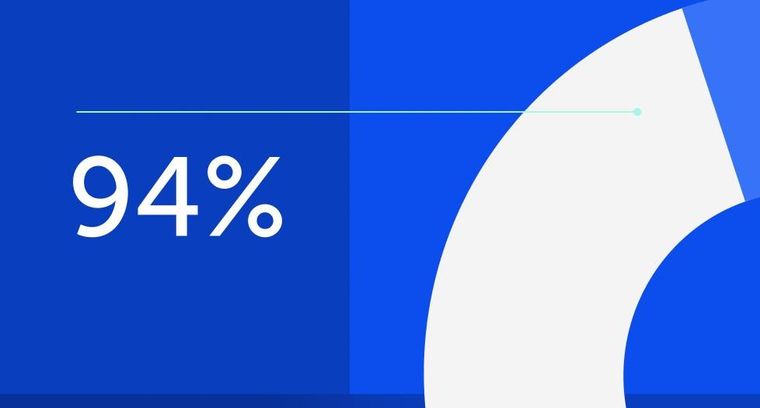
94% of researchers rate our articles as excellent or good
Learn more about the work of our research integrity team to safeguard the quality of each article we publish.
Find out more
ORIGINAL RESEARCH article
Front. Mar. Sci., 25 July 2019
Sec. Global Change and the Future Ocean
Volume 6 - 2019 | https://doi.org/10.3389/fmars.2019.00453
A correction has been applied to this article in:
Corrigendum: Night-Time Temperature Reprieves Enhance the Thermal Tolerance of a Symbiotic Cnidarian
Responses of cnidarian-Symbiodiniaceae associations to warming are determined, in part, by high-frequency temperature variability. Yet, the role of such variability in determining specific maximum temperature thresholds of cnidarian holobionts (the ecological units comprised of cnidarian hosts and associated microorganisms, including Symbiodiniaceae) remains untested. Here we contrasted the thermal resilience (that is the ability to resist stress) of a model symbiotic cnidarian from the Red Sea (jellyfish of the genus Cassiopea) under stable and diel oscillating temperature conditions that provide night-time reprieves from daily maximum temperatures. Holobionts were subjected to two thermal trajectories; one that increased but plateaued at 2°C below identified bleaching thresholds and another that increased incrementally until holobionts bleached. We used behavior, growth, photochemical efficiency, Symbiodiniaceae (symbiont) cell density, and total chlorophyll cell content to characterize thermal resilience and examined Symbiodiniaceae community composition responses at 1 and 13 days of exposure, and post-bleaching. Lower night-time temperatures, resulting in lower daily mean temperatures, allowed holobionts to withstand daily maximum temperatures close to their bleaching thresholds for two extra days than those under stable maximum temperature conditions. Lower night-time temperatures increased the bleaching threshold of the holobionts, whereby holobionts exposed to night-time thermal reprieves tolerated a more extreme daily mean temperature of 40.6°C and reached a daily thermal maxima 4°C higher than those under stable temperature conditions. However, post-bleaching observations indicate that night-time temperature reprieves did not prevent symbiont cell or pigment loss. Symbiodiniaceae communities were unaffected by lower night-time temperatures and no directional changes indicative of symbiont shuffling/selection of thermally tolerant lineages were observed. We show that stable experimental treatments may fail to accurately identify maximum thermal thresholds of non-calcifying cnidarians and limit their relevance to in situ environments that are often characterized by high levels of temperature fluctuations.
Ongoing anthropogenic climate change has heightened the need to understand potential drivers of resilience (defined here as the ability to resist stress, sensu Palumbi et al., 2008) of marine biota. This is especially urgent for the endosymbiotic relationship between cnidarian hosts and dinoflagellates of the family Symbiodiniaceae that fundamentally drives the productivity of tropical and temperate reefs worldwide (Muscatine and Porter, 1977). Indeed, the breakdown of these endosymbiotic relationships in response to warming ocean temperatures (commonly known as bleaching) has caused unprecedented degradation of coral reef ecosystems in recent decades (Hughes et al., 2018), yet our knowledge of factors driving cnidarian thermal resilience is incomplete.
Many experimental assessments of cnidarian responses to thermal stress apply static, carefully controlled elevated temperature scenarios (typically within ±1°C of mean treatment levels, e.g., Bahr et al., 2016; Horvath et al., 2016) to calcifying cnidarians. Such experimental data have been critical to our current understanding of mechanisms underlying bleaching (Lesser, 1997; Warner et al., 1999) and facilitated uniform comparisons of thermal tolerance among calcifying cnidarian taxa (Ulstrup et al., 2006; Negri et al., 2007). However, subjecting biota to stable (static) conditions can be inconsistent with the naturally dynamic temperature conditions inherent to the coastal zone and other shallow ecosystems (Putnam and Edmunds, 2011; Lima and Wethey, 2012) that cnidarians typically inhabit. Some studies of cnidarian bleaching under thermal stress incorporate temperature fluctuations into experimental thermal regimes (e.g., Grottoli et al., 2014; Krueger et al., 2015), providing realistic assessments of thermal tolerance. Although cnidarian bleaching predominately occurs in response to elevated temperature (Glynn, 1993; Hughes et al., 2017), physiological responses to thermal stress depend on multiple factors (Fitt et al., 2001), including absolute temperature, the duration of exposure to elevated temperature, and the rate at which temperatures rise (Hoegh-Guldberg and Smith, 1989; Jokiel and Coles, 1990; Middlebrook et al., 2010).
The extent of temperature variability within coastal ecosystems is often site-specific. Temperatures may vary by up to 10°C within diel cycles (e.g., Safaie et al., 2018), and be moderated by numerous factors, including depth, tidal-flushing (Leichter and Miller, 1999), and waves (Davis et al., 2011). Field observations of coral reef ecosystems suggest that historical temperature variability (over annual to century timescales) may determine the physiological tolerance (Thompson and Van Woesik, 2009; Oliver and Palumbi, 2011) and performance (Castillo et al., 2012) of coral holobionts under thermal stress. Cnidarian resilience to particular thermal regimes may result from host adaptation or acclimatization (Barshis et al., 2013), selection of thermally tolerant Symbiodiniaceae lineages (Hume et al., 2015), and altered bacterial microbiomes (Ziegler et al., 2017). On a global scale, “high-frequency” temperature variability (defined here as within diel cycles) can reduce the risk of coral bleaching (Safaie et al., 2018). These observations are consistent with a recent review, which reports that coral reef biota exposed to varying temperatures are more tolerant to climate change (Rivest et al., 2017). Although experimental studies are limited, cnidarian responses to thermal variation are inconsistent since oscillations in temperature can either alleviate the impacts of warming (Mayfield et al., 2012; Jiang et al., 2017) or induce more severe effects (Putnam and Edmunds, 2008, 2011). Such assessments, however, have seldom considered non-calcifying symbiotic cnidarians, which are widespread in shallow subtropical and tropical coastal waters (Muller-Parker and Davy, 2001; Ohdera et al., 2018).
Experimental assessments of temperature variability often compare cnidarian responses under stable versus variable treatments with the same mean temperature. Such comparisons, however, expose holobionts in variable treatments to daily temperatures that are alternatively higher and lower than those in stable treatments (e.g., Putnam and Edmunds, 2008; Mayfield et al., 2012). Inconsistent findings among such assessments may, in part, depend on the amplitude and nature of temperature fluctuations tested. For instance, constant exposure to 31°C inhibited settlement of Pocillopora damicornis larvae by ∼50%, whereas larvae exposed to temperatures varying between 30 and 33°C exhibited no thermal stress (Jiang et al., 2017). Physiological responses of Pocillopora meandrina and Porites rus exposed to temperatures varying between 26 and 30°C were similar to those continuously exposed to 30°C, despite the mean temperature in the variable treatment being ∼2°C lower (Putnam and Edmunds, 2011). The selection of maximum temperatures in variable and stable treatments is an important consideration because Jensen’s inequality predicts that cooler and warmer periods during thermal regimes will have disproportionate effects on the physiological responses of organisms (Ruel and Ayres, 1999; Denny, 2017). Indeed, cnidarians often exist close to their upper thermal limit and relatively small temperature excursions above the mean summer maximum, even for short periods (i.e., hours), can facilitate the breakdown of cnidarian-algal symbioses (Baker et al., 2008). These observations pose an interesting question as to whether night-time thermal reprieves that provide lower daily mean temperatures enhance resilience of cnidarians to daily maximum temperatures. We are not aware, however, of any experimental tests that quantify the potential role of night-time temperature reprieves from daytime temperature maxima in influencing the thermal resilience of cnidarians or algal symbiont assemblages.
To understand potential mechanisms underlying cnidarian thermal tolerance, recent studies have investigated cnidarians that inhabit naturally high and variable temperature environments (e.g., Oliver and Palumbi, 2011; Schoepf et al., 2015). Jellyfishes of the genus Cassiopea are emerging as highly versatile cnidarian models to investigate the relationship between cnidarian hosts and Symbiodiniaceae spp. in response to environmental change (Klein et al., 2017; Ohdera et al., 2018). Like their anthozoan counterparts (anemones and corals), Cassiopea spp. harbor dense intracellular populations of Symbiodiniaceae spp. and inhabit shallow tropical and sub-tropical waters (Lampert, 2016). Cassiopea spp. are considered robust cnidarians because they can persist under a broad range of temperature and salinity conditions (Klein et al., 2016a; Morandini et al., 2017) and are prevalent in shallow lagoon systems (Lampert, 2016). These habitats are often characterized by intense light and variable yet elevated temperatures in summer relative to other well-flushed coastal waters. Nevertheless, elevated temperatures can disrupt the endosymbiotic relationship between Symbiodiniaceae spp. and Cassiopea hosts, leading to thermally induced bleaching similar to that experienced by corals and anemones (e.g., Fitt and Costley, 1998; McGill and Pomory, 2008).
Here, we characterize the thermal resilience of Cassiopea sp. holobionts from the Red Sea and report our findings in three main parts (henceforth referred to as Part I, II, and III). In Part I, we report the first observations of a natural bleaching event of Cassiopea sp. medusae in a shallow lagoon ecosystem in the central Red Sea, characterized by extreme but highly variable temperature conditions. In Part II, we tested the thermal resilience of Cassiopea sp. and in hospite Symbiodiniaceae communities under “stable” versus oscillating increasing temperature regimes with uniform maximum temperatures, where the regimes increased incrementally but “plateaued” at 2°C below their identified bleaching threshold. Specifically, we hypothesized that exposure to lower night-time temperatures would extend the period over which holobionts could withstand daily maximum temperatures close to their upper thermal threshold. As a corollary, Part III contrasted the thermal resilience of Cassiopea sp. and in hospite Symbiodiniaceae communities under “stable” versus oscillating increasing temperature regimes with the same maximum temperatures, where the regimes increased incrementally until medusae bleached. We hypothesized that holobionts exposed to lower night-time temperatures, and lower daily mean temperatures, would bleach at higher daily maximum temperatures than those exposed to “stable” increments in temperature.
Bleaching of Cassiopea sp. medusae (Supplementary Figure S1) was observed in a semi-enclosed shallow lagoon ecosystem (0.2–1.0 m depth, 22.39°N, 39.13°E) near Thuwal, Saudi Arabia, in the central Red Sea on the 12th September 2017. In situ seawater temperature data were unavailable for the shallow lagoon system at the time of bleaching so historical atmospheric temperature data were retrospectively obtained from AccuWeather©1 (sensu, Mueller et al., 2018) for Thuwal, Saudi Arabia. These data included atmospheric temperatures for the time of bleaching (1st June–28th September 2017) and 15-year average temperatures for the same period. Near-real-time (NRT) sea level anomaly (0.25° × 0.25° grid layer resolution) data for the same period and location were generated using E.U Copernicus Marine Service Information2 (sensu, Kürten et al., 2019).
Cassiopea sp. medusae (bell diameter range: 42–95 mm) were collected from depths of 0.3–0.6 m in the King Abdullah Economic City Lagoon (22°23′46.44′′N, 39° 7′25.05′′E) in the Central Red Sea in November 2017. Medusae were immediately transported to the laboratory at the ambient temperature of 28°C and transferred to four 100 L aquaria filled with ambient (unfiltered) seawater. Medusae were maintained at 28°C (±0.7°C) with a salinity of 41 ppt, under a 14:10 h (light: dark) cycle of ∼450 μmol photons m–2 s–1 (photosynthetic active radiation [PAR]) for 5 days, consistent with the conditions they experienced in the King Abdullah Economic City Lagoon at the time of collection. Ambient seawater flowed continuously through the aquaria at a rate of ∼120 L h–1. Medusae were fed daily using a combination of newly hatched Artemia nauplii and commercial cnidarian food; Zoo⋅blast (Continuum AquaticsTM) and Medusa-G (100–350 μm particle size, Exotic Aquaculture – Sanderia Group Ltd.).
The control treatment was maintained at 28°C and used to ensure incubator conditions were optimal for medusae health during both experiments (Parts II and III, Figures 1A,B, respectively). The “Plateau” experiment (Part II) consisted of two thermal treatments (Figure 1A). Temperature in the Stable Plateau treatment [SPlat] was raised by 1°C d–1 from the ambient temperature of 28°C until the temperature reached 35°C (Figure 1A) where it was maintained for 5 days to acclimate medusae to typical summer temperature conditions. Temperature in the SPlat treatment was then raised to 37°C, equivalent to 2°C below the identified bleaching threshold (B1-2 = 37°C, see Figure 1), and held at 37°C until medusae bleached (Figure 1A). The Oscillating Plateau [OPlat] treatment followed the same thermal trajectory as the SPlat treatment but temperature conditions oscillated; whereby the daily maximum and minimum temperatures increased by 1°C d–1 until a 5°C difference in the minimum and maximum temperatures was attained (Figure 1A). This approach ensured that the magnitude of thermal fluctuation (daily max – min) increased incrementally from the ambient (constant) temperature of 28°C at a rate of 1°C day–1 until the magnitude of fluctuation reached 5°C d–1 after 6 days of exposure (i.e., Figure 1A). The OPlat treatment was then maintained under 5°C d–1 temperature fluctuations and held at a maximum temperature of 35°C for 5 days. After summer acclimation, maximum and minimum temperatures were raised by 2°C in the OPlat treatment and held at a maximum temperature of 37°C (Figure 1) until medusae bleached.
Figure 1. Recorded temperature profiles of (A) the Stable Plateau [SPlat] and Oscillating Plateau [OPlat] treatments used in Part II of this study (the “Plateau” experiment) and (B) the Stable Increasing [SIncr] and Oscillating Increasing [OIncr] treatments employed in Part III of this study (the “Increasing” experiment). The recorded temperature profile of the control treatment is reported for Part II and Part III in panel (A) and (B), respectively. Exposure days 8–12 mark the period of summer acclimation for the “Plateau” and “Increasing” experiments. The solid and dashed arrows represent when the “stable” and “oscillating” treatments bleached, respectively, in the “Plateau” experiment (blue arrows, Part II) and “Increasing” experiment (green arrows, Part III). B1 and B2 represent the temperatures (°C) at which treatments SIncr and OIncr bleached, respectively. B1-2 represents 2°C below the minimum bleaching temperature observed in the “increasing” treatments at which the “Plateau” treatments were held. Note; the “Plateau” experiment commenced 3 days later than the “Increasing” experiment to allow for the minimum bleaching temperature to be identified.
The “Increasing” experiment (Part III) comprised two thermal treatments (Figure 1B) and commenced 3 days prior to the “Plateau” experiment so that the bleaching threshold could be identified (B1, Figure 1B). Bleaching end-points in the SIncr and OIncr treatments were identified as Day 14 (at constant exposure to 39°C), and Day 16 (at maximum exposure to 43°C), respectively (Figure 1B). Temperature conditions in the Stable Increasing (SIncr) and Oscillating increasing treatments (OIncr) followed the same thermal trajectories as those in the SPlat and OPlat but after summer acclimation were raised 2°C d–1 until they bleached (Figure 1B). Medusae were considered “bleached” when parts of a medusa appeared distinctly pale and / or obvious decreases in pigmentation were observed overall relative to photographs taken at Day 1 of the experiment (sensu, DeMartini et al., 2010; Putnam and Edmunds, 2011).
Individual medusae were transferred into 2 L glass aquaria and 11 medusae were allocated to each treatment employed in Part II (SPlat and OPlat) and Part III (SIncr and OIncr), including the control. Consistent size ranges of medusae were allocated to each treatment (see Supplementary Table S1). Temperature and light regimes were manipulated using biological incubators (Percival Scientific©). Replicates from each treatment were allocated to the same incubator but to minimize artifacts associated with individual incubators, treatments were randomly reallocated to a different incubator every second day. Each aquarium was aerated at the surface to ensure adequate flow and normoxic conditions, and a loose-fitting plastic lid was placed over each aquarium to minimize evaporation. All holobionts were acclimated to incubator conditions for 48 h before their respective treatments commenced and exposed to identical diel light conditions throughout the experiment, which consisted of a 15:9 h light: dark cycle where maximum PAR reached 400–450 μmol photons m–2 s–1 between 9:00 and 16:00 local time (Supplementary Figure S2). Individual PAR (Odyssey©) and temperature (HOBO®) sensors were submersed in separate aquaria (without medusae) within each incubator and were cycled among incubators to record PAR and water temperatures for each treatment (Supplementary Figures S2, S3). Medusae were fed a combination of newly hatched Artemia nauplii and Medusa-G daily. Medusae were fed for 2 h, after which any debris was removed from the aquarium using a pipette and ∼80% of the seawater was exchanged with fresh seawater of the temperature appropriate for each treatment. Salinity was maintained at ∼41ppt.
For Parts II and III, holobiont size, behavior (bell contractions min–1), and several photophysiological parameters (maximum photochemical efficiency (Fv/Fm), Symbiodiniaceae cell density and total chlorophyll (chl) cell content) were measured. Medusae growth, behavior and Fv/Fm were measured at Day 1, 4, 7, 10, 13, and daily thereafter. Subsets of medusae from each treatment (n = 3–4, see Figures 2, 3) were destructively sampled to measure Symbiodiniaceae cell density and total chl cell content on Day 1 (start of experiment) and Day 13 (after summer acclimation). Remaining medusae in each treatment were used to measure Symbiodiniaceae cell density and total chl cell content after bleaching. The endpoints of the treatments differed because they were determined by when the medusae bleached. Although it would have been ideal to compare a fixed endpoint among treatments, sampling immediately after bleaching allowed for a separate assessment of the impact of bleaching on the holobiont in response to the various thermal treatments tested.
Figure 2. Mean (±1SE) (A) bell contractions (number min–1), (B) change in size of medusae (%), and (C) maximum photochemical efficiency (Fv/Fm), recorded throughout the 16-day exposure period for each treatment (SPlat, OPlat, and Control) employed in “Plateau” experiment (Part II). Letters next to data points indicate similarities (e.g., AA) or differences (e.g., AB) between the three treatments at each time point, as determined by estimated marginal means post hoc analyses, resulting from analyses reported in Supplementary Tables S2, S3. Note; sample sizes vary through time [Day 1 (n = 11), Days 4–13 (n = 7), Day 14 (n = 4), Days 15–16 (n = 3–4) (n represents number of replicates per treatment)].
Figure 3. Mean (±1SE) rates of (A) total chl content (pg chl Symbiodiniaceae cell–1) and (B) Symbiodiniaceae cell density (μg–1 total protein) for each treatment (SPlat, OPlat, and Control) in the “Plateau” experiment (Part II) recorded at Day 1, 13, and post-bleaching. Letters next to data points indicate similarities (e.g., AA) or differences (e.g., AB) between the three treatments within each time point, as determined by estimated marginal means post hoc analyses, resulting from analyses reported in Supplementary Tables S4, S5. Note; sample sizes vary through time [Day 1 (n = 5), Day 13 (n = 3), Post-bleaching (n = 4) (n represents number of replicates per treatment)]. Days (and temperatures) at which medusae were sampled post-bleaching (shaded blue) differed, where medusae in the SPlat treatment bleached after 14 days of exposure and medusae in the OPlat treatment bleached after 16 days of exposure (see Figure 1A).
Medusae size was measured as the bell diameter (at full extension) to the nearest mm. Measurements of bell diameter were made through the bottom of the glass aquarium using a ruler. Medusae growth (% change in size) was calculated relative to the size of medusae at the start of the experiment (i.e., Day 1). Medusae behavior was characterized by counting the number of bell contractions of individual medusae for 2 min and recorded as bell contractions min–1. To minimize the impact of these measurements on responses, medusae remained inside their respective incubators when measurements of bell diameter and behavior were taken. Fv/Fm was measured at midday using a Mini-pulse amplitude modulator (Mini-PAM, Walz GmbH, Germany). Animals were dark acclimated for 30 min inside their incubators and individual aquaria were removed one at a time for Fv/Fm measurements. Repeated chla fluorescence inductions were made to return values of the minimum (F0) and maximum (Fm), and hence also; Fv/Fm(Fv/Fm=[Fm−F0]/Fm).
Individual medusae were removed from their aquaria (and respective treatments) and frozen at −25°C for measurements of Symbiodiniaceae cell density, total chl cell content, total protein, and MiSeq analyses of Symbiodiniaceae ITS2 type communities. Frozen medusae were macerated using a Phillips© Viva (600W) blender for 30 s and triplicate 1500 μL aliquots were extracted for measurements of Symbiodiniaceae cell densities, chl content, and protein content. 200 μL aliquots were sub-sampled from the 1500 μL aliquots, homogenized in 500 μL of 0.1% sodium dodecyl sulfate (SDS) in deionized water using a Wheaton® tissue grinder and repetitively passed through a 25-gauge needle affixed to a 3 mL syringe. Numbers of Symbiodiniaceae cells in each aliquot were counted using a Guava® easyCyte cell flow cytometer. One 500 μL aliquot was subsampled from the 1500 μL aliquots and used to estimate chl content. Chl samples were centrifuged at 3000 × g at 4°C for 10 min and the supernatant discarded. The pelleted Symbiodiniaceae were re-suspended in 100% ethanol and chl was extracted overnight in darkness at 4°C and then centrifuged at 13000 × g for 5 min. The supernatant was transferred to 10 mm cuvettes and the absorption of the supernatant was determined at 629 and 665 nm using a Thermo Scientific NanoDropTM spectrophotometer. Blanks (100% ethanol) were used to calibrate the spectrophotometer. Total chl (chla+ chlc2) concentrations were determined using coefficients from spectrophotometric equations for chla (−2.6094 × A629 + 12.4380 × A665) and chlc2 (29.8208 × A629 − 5.6461 × A665) for dinoflagellates in ethanol (Ritchie, 2006). Symbiodiniaceae cell densities were standardized to total protein content of the holobiont (PierceTM BCA Protein Assay Kit, [units] μg/mL) and total chl was normalized to pg Symbiodiniaceae cell–1 (units).
We applied a recently revised taxonomic classification, which re-assigns the micro-algal genus Symbiodinium into multiple genera (sensu Symbiodiniaceae, LaJeunesse et al., 2018). The internal transcribed spacer region 2 (ITS2) of Symbiodiniaceae ribosomal DNA was amplified from DNA extracts (using a DNeasy Plant Mini Kit, Qiagen©, following protocols detailed in Hume et al. (2018) of holobionts sacrificed at Day 1, 13, and post-bleaching to determine if the Symbiodiniaceae community composition contributed to changes in holobiont fitness in response to the treatments tested. High-throughput sequencing using the Illumina MiSeq platform was used to analyze the ITS2 amplicons followed by bioinformatical analysis using the SymPortal pipeline3 to resolve Symbiodiniaceae spp. (Hume et al., 2019). The SymPortal pipeline assumes that a host can only host one main taxon per genus (Hume et al., 2019) and categorizes intra-genomic diversity based on this assumption. However, we investigated the possibility of more than one taxon per genus within the holobionts by comparing sequence data obtained from SymPortal data to those from isolates cultured from the experimental samples (unpublished data).
Biological response variables (bell contractions, change in medusae size and Fv/Fm, Symbiodiniaceae cell densities and total chl cell content) were analyzed using Linear Mixed Models (LMMs, see Table 1). Prior to analyses, all data were checked for normality and homoscedasticity using standardized residuals and Q-Q plots and if required, data were either Ln, Ln(x+1), Ln(x×−1) or Ln(x+a+1) transformed, where a = the absolute minimum value of a given dependent variable to convert negative values to positive values.
Table 1. Summary of Linear Mixed Models (LMMs) conducted to analyze biological response variables in (A) the “Plateau experiment” (Part II) and (B) the “Increasing” experiment (Part III).
For Part II of this study, two separate LMMs were used to analyse bell contractions, change in medusae size, and Fv/Fm (Table 1A). First, repeated measures LMMs were used to analyze the dependent variables to compare responses among treatments during the first 14 days of the experiment, where treatment (i.e., SPlat, OPlat, and control) was the fixed factor and time (Days 1, 4, 7, 10, 13, and 14) was the repeated measure (Supplementary Table S2). Second, one-way LMMs analyses were used to contrast responses between the control and the OPlat treatment that remained when the experiment ended on Day 16 (Supplementary Table S3). We compared Symbiodiniaceae cell densities and chl content using two separate LMMs (Table 1A). First, two-way LMMs were conducted to compare responses among treatments at Day 1 and Day 13. The fixed factors were treatment (SPlat, OPlat and control) and exposure time (Days 1 and 13, Supplementary Table S4). Second, one-way LMMs were used to contrast responses among treatments (SPlat, OPlat, and control) post-bleaching (Supplementary Table S5).
For Part III, two separate LMMs were used to compare bell contractions, change in medusae size, and Fv/Fm among treatments (SIncr, OIncr, and control, Table 1B). First, repeated measures LMMs were used to compare responses among treatments during the first 14 days of the experiment (Days 1, 4, 7, 10, 13, and 14, Supplementary Table S6). Second, one-way LMMs analyses were used to contrast responses of the control and the OIncr treatment, which remained at the end of the experiment (Day 16, Supplementary Table S7). To compare Symbiodiniaceae cell densities and chl content among treatments in the “Increasing” experiment we used two separate LMMs (Table 1B). First, two-way LMMs were conducted to compare responses among treatments at Day 1 and Day 13 of the experiment, where, the fixed factors were treatment (SIncr, OIncr and control) and exposure time (Days 1 and 13, see Supplementary Table S8). Second, one-way LMMs were used to compare responses among treatments (SIncr, OIncr, and control) post-bleaching (Supplementary Table S9).
We included aquarium ID as a random factor (or block) into all LMMs analyses to test for potential aquarium (or tank) bias. However, preliminary analyses for all dependent variables revealed this random factor as redundant and so the factor was removed and all analyses re-run. For all repeated measure LMM analyses, a range of repeated covariance structures (e.g., AR [1], AR [1]: heterogeneous, Compound Symmetry [CS]) were investigated and the best model was obtained by comparing various goodness-of-fit statistics (−2 log likelihood, Akaike information criterion [AIC] and Bayesian information criterion [BIC]). Estimated marginal means (post hoc comparisons of least-squares means) were used to identify which means differed for the significant, highest-order term.
Permutational multivariate analysis of variance (PERMANOVA) analyses were used to analyze percent (%) composition of Symbiodiniaceae ITS2 types within holobionts using PRIMER6+. For Part II of this study, we analyzed the % composition of Symbiodiniaceae genetic types in treatments at Day 1 and 13 using a two-way PERMANOVA, where the fixed factors were treatment (SPlat, OPlat, and control) and exposure time (days 1 and 13, Supplementary Table S10). Second, a one-way PERMANOVA analysis was used to contrast the % composition of of Symbiodiniaceae genetic types among treatments in the “Plateau” experiment (SPlat, OPlat, and control) post-bleaching (Supplementary Table S10). For Part III, we contrasted the % composition of Symbiodiniaceae genetic types among treatments in the “Increasing” experiment using a two-way PERMANOVA. The fixed factors were treatment (SIncr, OIncr, and control) and exposure time (days 1 and 13, Supplementary Table S11). Second, a one-way PERMANOVA analysis was used to compare % composition of Symbiodiniaceae ITS2 types within holobionts in the SIncr, OIncr, and control treatments post-bleaching (Supplementary Table S9). Details of all statistical analyses (including transformations, goodness-of-fit statistics, and repeated covariance structures) are provided in the electronic Supplementary Material (Supplementary Tables S2–S11).
Bleaching of Cassiopea sp. medusae coincided with a heat wave (atmospheric temperatures exceeded 44°C) and unusually low sea levels (−0.2 to−0.5 m, relative to mean sea water level). Specifically, maximum daily atmospheric temperature reached 49°C on 24th August 2017 but medusae did not bleach until daily temperatures >44°C persisted for 3 days (9–11th September 2017, see Supplementary Figure S3), equating to 8°C higher than the average historical maximum temperature for the month of September (15-year average). Mass mortality of Cassiopea sp. medusae occurred 5–7 days after bleaching of the holobionts was first observed on the 12th September 2017 (pers. obs. Klein, Hung, and Schmidt-Roach).
Medusae in the control treatment exhibited no change in behavior (Figure 2A), grew by 6.2% ± 2.14 (mean ± 1SE, Figure 2B), and maintained high maximum photochemical efficiency (Fv/Fm, Figure 2C), indicating that incubator conditions provided optimal conditions for the holobionts. Medusae in the Stable Plateau (SPlat) treatment persisted at 37°C for only 2 days before bleaching, whereas medusae exposed to lower night-time temperatures in the Oscillating Plateau treatment (OPlat) bleached after 4 days (Figure 1). Specifically, medusae in the SPlat treatment experienced a temperature range of 36.85–37.44°C in the 2 days before bleaching (Figure 1A), whereas the inclusion of lower night-time temperatures yielded lower daily mean temperatures of 35.1°C in the OPlat treatment but temperatures reached the same daily maximum of 37°C (four day range: 32.01–37.42°C, Figure 1A, see Supplementary Table S11). Even though summer acclimation ended on Day 12, bell contractions and medusae size were similar in the SPlat and OPlat treatments for the first 13 days of the experiment (Figures 2A,B and Supplementary Table S2). Although Fv/Fm values of medusae were similar in the SPlat and OPlat treatment during summer acclimation, Fv/Fm values in the SPlat treatment were ∼29% lower than OPlat treatment when the temperature reached 37°C at Day 13 (Figure 2C and Supplementary Table S2). After 2 days at 37°C (Day 14), bell contraction rates in the SPlat treatment were ∼76% lower than those under oscillating temperature conditions at the same maximum temperature (OPlat) (Figure 2A). Medusae in the SPlat treatment shrunk by 32% (±2.83) after 2 days at 37°C (Day 14), whereas those under oscillating temperature conditions held at the same maximum temperature (OPlat) did not shrink (−1% ± 1.01) (Figure 2B). Although Fv/Fm values for both plateau treatments were reduced at Day 14 relative to those for the previous day, Fv/Fm values were ∼32% lower in the SPlat treatment than the OPlat treatment (Figure 2C).
After bleaching, pigment content of Symbiodiniaceae cells was unaffected by the SPlat and OPlat thermal regimes (Figure 3A and Supplementary Table S5). Even though medusae in the OPlat treatment persisted for 2 days longer than those in the SPlat treatment, albeit at lower daily mean temperatures (SPlat = 37.05°C versus OPlat = 35.09°C), Symbiodiniaceae cell densities remained similar between the plateauing treatments after bleaching (0.695 ± 0.24 cells × 102 μg–1 protein) (Figure 3B and Supplementary Table S5). ITS2 type compositions were generally homogenous among all individuals within the “Plateau” experiment (Supplementary Table S10 and Supplementary Figure S4A) at Day 1, Day 13 and post-bleaching and no directional changes indicative of adaptive symbiont shuffling/selection were observed in response to the SPlat and OPlat treatments. Therefore, thermal-induced mortality conducive to bleaching affected all Symbiodiniaceae variants equally. Consequently, any change in fitness of the holobionts in response to the treatments tested reflects a change of the physiology (and/or abundance) of the existing Symbiodiniaceae types and/or host, rather than a shift toward alternative types with differing physiologies. The most abundant Symbiodiniaceae genera were Symbiodinium (Clade A) and Breviolum (Clade B). However, other genera that included Cladocopium, Durusdinium, and Fugacium were identified in low abundances. SymPortal assigned an average of 64.03% of our ITS2 sequences to “defining intragenomic variants” representative of putative Symbiodiniaceae taxa (see, Hume et al., 2019). The most abundant Symbiodinium defining intragenomic variants, A1 and A4–A4 m, are associated to the species S. microadriaticum and S. linucheae (Supplementary Figures S4A,B). Breviolum spp. were also present across samples (Supplementary Figures S4A,B), with the defining intragenomic variants of B1, B1-980-B1a and B1-B1a, assigned to the three species; B. antillogorgium, B. minutum, and B. pseudominutum, respectively (LaJeunesse et al., 2018).
Although we aimed to euthanize holobionts when bleaching occurred, one medusa from each of the SIncr and OIncr treatments died before bleaching at the final time point for each of their respective treatments [SIncr = Day 14 (39°C) and OIncr = Day 16 (max 43°C)]. All other medusae were alive when they bleached and after response variables were measured, were euthanized. Medusae exposed to temperature oscillations (OIncr) tolerated a daily mean temperature of 40.6°C (daily range: 37.92–43.11°C) and reached a maximum temperature 4°C higher than those under stable temperature conditions (SIncr) that experienced a daily mean temperature of 38.9°C before bleaching (daily range: 38.6–39.2°C, Figure 1B). Regardless of the presence of night-time temperature reprieves, holobionts in the increasing treatments (SIncr and OIncr) responded similarly, for all response variables measured during the first 13 days of the experiment (Figures 4, 5, and Supplementary Table S6). When temperatures reached 39°C on Day 14, bell contraction rates were similar among the SIncr and OIncr treatments and 68% higher than those in the control treatment (Figure 4A). Lower night-time temperatures, however, ameliorated the negative effects on holobiont size and Fv/Fm when temperatures reached 39°C. Specifically, medusae in the OIncr treatment had not grown (mean size change: 3% ± 5.54) but those in the SIncr treatment had shrunk by 18% (±5.81, Figure 4B). These observations were consistent with those of their symbionts; whereby Fv/Fm values were ∼51% lower in the SIncr treatment at Day 14 (when temperatures reached 39°C) than those in the OIncr treatment (Figure 4C).
Figure 4. Mean (±1SE) (A) bell contractions (number min–1), (B) change in size of medusae (%), and (C) maximum photochemical efficiency (Fv/Fm), recorded throughout the 16-day exposure period for each treatment (SIncr, OIncr, and Control) employed in “Increasing” experiment (Part III). Letters next to data points indicate similarities (e.g., AA) or differences (e.g., AB) between the three treatments at each time point, as determined by estimated marginal means post hoc analyses, resulting from analyses reported in Supplementary Tables S6, S7. Note; sample sizes vary through time [Day 1 (n = 11), Days 4–13 (n = 7), Day 14 (n = 4), Days 15–16 (n = 3) (n represents number of replicates per treatment)].
Figure 5. Mean (±1SE) rates of (A) total chl content (pg chl Symbiodiniaceae cell–1) and (B) Symbiodiniaceae cell density (μg–1 total protein) for each treatment (SIncr, OIncr, and Control) in the “Increasing” experiment (Part III) recorded at Day 1, 13, and post-bleaching. Letters next to data points indicate similarities (e.g., AA) or differences (e.g., AB) between the three treatments within each time point, as determined by estimated marginal means post hoc analyses, resulting from analyses reported in Supplementary Tables S8, S9. Note; sample sizes vary through time [Day 1 (n = 5), Day 13 (n = 3), Post-bleaching (n = 3) (n represents number of replicates per treatment)]. Days (and temperatures) at which medusae were sampled post-bleaching (shaded blue) differed, where medusae in the SIncr treatment bleached after 14 days of exposure and medusae in the OIncr treatment bleached after 16 days of exposure (see Figure 1B).
Even though medusae in the OIncr treatment persisted until Day 16 and reached a maximum daily temperature of 43°C, pigment content of Symbiodiniaceae cells were similar in the SIncr and OIncr thermal regimes after bleaching (Figures 5A,B and Supplementary Table S9). Medusae in the SIncr and OIncr treatments exhibited similar reductions in Symbiodiniaceae cell density (82% loss) relative to the control treatment (Figures 5A,B and Supplementary Table S9). ITS2 type compositions were similar among all medusae (Supplementary Table S11 and Supplementary Figure S4B) in the “Increasing” experiment (i.e., SIncr, OIncr, and control) at Day 1, 13, and post-bleaching. Symbiodiniaceae communities were thus unaffected by lower night-time temperatures and no directional changes indicative of selection of thermally tolerant lineages were observed. Symbiodinium (Clade A) and Breviolum (Clade B) dominated Symbiodiniaceae communities and compositions were consistent with those observed in the “Plateau” experiment, including the frequencies of other, less abundant genera (Supplementary Figures S4A,B). Sequences retrieved by this study were deposited in NCBI GenBank under BioProject ID: PRJNA494341.
Here, we present evidence that lower night-time temperatures provide a reprieve from daytime temperature extremes and increase the resilience of the Cassiopea sp. holobiont to bleaching. Specifically, lower night-time temperatures that yielded lower daily mean temperatures extended the duration at which the holobionts could withstand daily thermal maxima close to their bleaching threshold by 2 days and increased the daily maximum and mean temperatures medusae could withstand before bleaching by 4 and 1.7°C, respectively. Although we cannot compare our findings with any other experimental test of thermal resilience under steady versus oscillating regimes, a growing body of field (Oliver and Palumbi, 2011; Safaie et al., 2018) and experimental (Mayfield et al., 2012; Jiang et al., 2017) evidence highlights a fundamental role of temperature variability, particularly within diel timescales, in determining cnidarian responses to elevated temperatures. One of the few studies to contrast cnidarian holobiont responses under stable versus ephemeral exposure to the same maximum temperature reported that daily temperature fluctuations (between 26 and 30°C) elicited similar deleterious responses on two scleractinian corals (Pocillopora meandrina and Porites rus) to those exposed to constant temperature conditions (held at 30°C, Putnam and Edmunds, 2011). In the current study, however, observations of Cassiopea sp. holobionts at temperatures preceding bleaching thresholds are inconsistent with the findings reported for P. meandrina and P. rus (Putnam and Edmunds, 2011). For instance, when temperatures reached 39°C, holobionts exposed to the SIncr temperature regime had higher rates of bell contractions, reduced body size and inhibited photochemical efficiency of Symbiodiniaceae relative to holobionts under oscillating temperature conditions (OIncr) at the equivalent maximum temperature. Together, these observations highlight the need to further investigate potential non-linear responses over diel cycles (Ruel and Ayres, 1999; Denny, 2017) and indicate that non-variable temperature manipulations alone may misrepresent cnidarian physiological responses that inhabit thermally variable ecosystems.
Temperature levels (Negri et al., 2007), duration of exposure (Dunn et al., 2004), and rates of increase (Middlebrook et al., 2010) in seawater temperature influence cnidarian physiological responses to thermal stress. It remains uncertain, however, how these metrics interact over spatial and temporal scales to drive cnidarian resilience or susceptibility to heating events (Putnam and Edmunds, 2011). Hypothesis II, and hence the “Plateau” experiment in the current study, was inspired by observations of a natural Cassiopea sp. bleaching event in a semi-enclosed lagoon in the Central Red Sea, where the breakdown of Cassiopea-Symbiodiniaceae associations occurred after extreme temperatures persisted for 3 days even though one day of exposure to more extreme temperature conditions occurred 16 days prior. Although it would have been ideal to obtain in situ data for the exact thermal regime that triggered the natural bleaching event reported here, our experimental data are highly consistent with these observations. Indeed, Cassiopea sp. holobionts held at 37°C in the Stable Plateau (SPlat) treatment bleached 2 days prior to those exposed to the night-time thermal reprieves held at the same maximum temperature (OPlat). Consistent with the “Increasing” experiment, night-time thermal reprieves alleviated the negative impacts on holobiont size and photochemical efficiency of medusae in the “plateau” treatments. Intriguingly, however, observations of medusae behavior contrasted with those of the “Increasing” experiment since rates of bell contractions increased rather than decreased, relative to the controls. These findings are also contrary to observations of Cassiopea sp. medusae acclimated to thermal conditions of the northern Red Sea, where acute (1–2 h) and chronic exposure (2 weeks) to +7°C warming increased rates of bell contractions (Aljbour et al., 2017), similar to our observations of Cassiopea in the “Increasing” experiment. Even so, these data demonstrate that bleaching of Cassiopea sp. is not driven exclusively by absolute temperature values but instead, can be induced by several metrics of seawater temperature change.
Inhibition of photophysiological metrics and reduced body mass can coincide with natural thermal bleaching of coral holobionts (Fitt et al., 2000; Wooldridge, 2013). Even though the extent to which biological responses of Cassiopea were affected depended on the presence of night-time temperature reprieves, inhibition of Fv/Fm preceded obvious signs of holobiont bleaching and reductions in body size coincided with holobiont bleaching, regardless of their thermal treatment. Although night-time thermal reprieves extended the duration at which the holobionts could persist at daily maximum temperatures in the “Plateau” experiment and enhanced thermal thresholds of Cassiopea under the “Increasing” regimes, oscillations did not prevent expulsion of Symbiodiniaceae cells or pigment loss. These findings are particularly interesting given the substantial differences among thermal conditions under which Cassiopea sp. bleached. Observations of Symbiodiniaceae and pigment loss in Cassiopea sp. are greater than those reported for coral holobiont responses under steady versus oscillating temperature regimes (Jones et al., 1998; Ulstrup et al., 2006). For instance, Symbiodiniaceae cell densities in P. meandrina and P. rus declined by between 17 and 45% after 24 h of ephemeral exposure to 30°C (26–30°C), matching Symbiodiniaceae cell density losses observed under stable exposure to 30°C (Putnam and Edmunds, 2011). In the current study, all Cassiopea sp. exposed to thermal treatments had reduced Symbiodiniaceae densities and chl content that ranged between 71–93% and 51–64%, respectively. These data are consistent with those of the only other published observation of natural Cassiopea sp. bleaching where medusae exhibited ∼80% Symbiodiniaceae cell loss (Fitt and Costley, 1998).
Susceptibility of phototrophic cnidarians to environmental perturbation corresponds, at least partially, to distinct in hospite Symbiodiniaceae species compositions (Berkelmans and Van Oppen, 2006). Differences in species-specific performance under stress can result in an increased proportion of tolerant algal symbionts, a process called symbiont shuffling (Cunning et al., 2015). Consequently, even minor changes in symbiont compositions can be adaptively beneficial (Cunning et al., 2015). However, in Parts II and III of this study, the dominant Symbiodiniaceae species were Symbiodinium microadriaticum and S. linucheae (assigned by their ITS2 types, A1 and A4–A4m, respectively). As we detected no difference is Symbiodiniaceae communities among holobionts (even post-bleaching) within either experiment, there was no evidence of major symbiont shuffling/selection of the dominant symbiont populations. Nevertheless, it is possible that the short duration of our experiments/thermal regimes could have precluded the potential selection of thermally tolerant Symbiodiniaceae lineages (but see, Lewis and Coffroth, 2004). Our findings that the genus Symbiodinium (clade A) dominates Cassiopea sp. aligns with previous observations of Cassiopea sp. holobionts from the Red Sea (e.g., Lampert et al., 2012) but, until now, the level of cryptic diversity of the marginally represented clades within Cassiopea holobionts has been seldom considered, likely due to the lack of resolution of previous methods like DGGE gel analysis. In holobionts studied here, two taxa of the same genus (A1 and A4–A4m) dominated the community at similar frequencies. These findings suggest that previous observations of apparent specificity of adult Cassiopea for single Symbiodiniaceae sp. may have failed to detect the cryptic diversity of marginally represented sp. and co-occurrence of intra-genus strains (Lampert et al., 2012). For this, future experiments of Cassiopea holobionts may consider establishing isolate cultures of Symbiodiniaceae spp. from experimental samples to determine potential co-occurrence of intra-genus taxa.
Generally, the degree of phenotypic plasticity in organisms is positively related with environmental variability (Kenkel and Matz, 2016). The influence of high-frequency temperature oscillations on holobiont fitness is not uniform across all host-Symbiodiniaceae associations. In fact, some studies of coral-cnidarians report no effect of temperature heterogeneity (e.g., Putnam and Edmunds, 2011) or even more severe effects (e.g., Putnam and Edmunds, 2008) in response to thermal stress. Indeed, inconsistent findings among such assessments may depend on the nature and magnitude of temperature fluctuations tested but may also be contingent on the thermal history of particular cnidarian associations. For example, on small spatial scales, corals that live in more variable temperature environments are more tolerant to thermal extremes than conspecifics that inhabit less thermally variable sites (Thompson and Van Woesik, 2009; Barshis et al., 2013; Schoepf et al., 2015). Cassiopea spp., including those studied here from the central Red Sea, often inhabit shallow waters, characterized by high levels of UV radiation and temperature variability (McGill and Pomory, 2008; Lampert, 2016). Thus, it is reasonable to hypothesize that extreme and variable thermal conditions within such habitats contribute to the acquired thermal resilience of Cassiopea-Symbiodiniaceae associations (Ohdera et al., 2018). Although temperature conditions are less severe in the northern Red Sea than those of the central Red Sea (Chaidez et al., 2017), aquarium-reared Cassiopea sp. acclimated to typical summer temperatures in the Gulf of Aqaba were resilient to a +7°C increase in temperature (Aljbour et al., 2017). Indeed, medusae exposed to 32°C for 2 weeks exhibited increased body mass, similar bell sizes and mitochondrial electron transport (ETS) rates relative to their conspecifics in the control treatment (25°C, Aljbour et al., 2017). Thermal resilience of Cassiopea sp. could be acquired via genetic adaption and/or acclimatization processes in the host (Kingsolver and Huey, 1998) or Symbiodiniaceae spp. (Chakravarti et al., 2017), however, without further research such mechanisms remain speculative but warrant investigation.
Projections of coral holobiont responses to rising ocean temperatures rely mostly on field observations of bleaching events (e.g., Logan et al., 2014) and empirical data typically resulting from experiments (e.g., Logan et al., 2014) that employ static temperature conditions (Jokiel and Coles, 1990). Evidence presented here suggests that neglecting to incorporate natural thermal regimes in experimental designs may result in inaccurate thermal threshold estimations and thus, cnidarian resilience to warming conditions. Indeed, recent research highlights the importance of incorporating high-frequency temperature variability into projection models to improve predictions and foresee complex patterns in bleaching prevalence (see, Safaie et al., 2018). For this, greater spatiotemporal resolution of surface sea temperature data from satellite remote sensing (Safaie et al., 2018) and model predictions are required, along with further taxa-specific empirical data of thermal resilience and bleaching thresholds under high-frequency temperature variability, such as those obtained here.
Night-time thermal reprieves that provided lower daily mean temperatures permitted Cassiopea holobionts to persist at higher daily mean and maximum temperatures before bleaching, thereby enhancing thermal resilience of Cassiopea. Non-calcifying holobionts, such as the benthic jellyfish tested here, may thus have a greater competitive advantage in more thermally variable ecosystems but this effect may ultimately depend on the nature of fluctuations tested and be restricted to particular associations that exhibit existing plasticity to temperature heterogeneity. Combined, these findings highlight the need to consider the selection of maximum temperatures in studies assessing the influence of temperature variation and highlight the need to investigate potential non-linear responses over diel cycles to fully unravel the role of night-time thermal reprieves. Whilst temperature oscillations may alleviate the impacts of heating on particular associations, cnidarians can be sensitive to other, highly variable drivers of biological responses inherent to coastal and other shallow ecosystems (e.g., pCO2: Price et al., 2012, e.g., UV: Lesser and Farrell, 2004; Klein et al., 2016b). Thus, to understand whether this potentially important role of night-time temperature reprieves holds when other, highly variable drivers coincide, future experimental tests should consider the effects of additional drivers in combination with temperature heterogeneity innate to coastal ecosystems.
Statistical analyses and raw data supporting this article have been uploaded as the electronic Supplementary Material. Sequences retrieved by this study were deposited in NCBI short read archive sequence data under BioProject ID: PRJNA494341.
SK, KP, CL, and CD developed the concept and design of the experiment. SK, CL, and KP collected the data. SK and S-HH processed and analyzed the samples. SK analyzed biological response of data and wrote the manuscript with contributions from KP and SS-R. SK, KP, CL, and CD contributed to interpretation of the biological response of data. SS-R, S-HH, and MA contributed analysis and interpretation of sequence data to the manuscript. All authors edited the manuscript and gave final approval of the manuscript.
This work was supported by King Abdullah University of Science and Technology (KAUST) through baseline funding to CD and MA.
The authors declare that the research was conducted in the absence of any commercial or financial relationships that could be construed as a potential conflict of interest.
We thank Zenon Batang, Paul Müller, and Nabeel Alikunhi for allocation of working space and technical support at the Coastal and Marine Resources Core Lab (CMOR) and Katherine Rowe for laboratory assistance. We also thank Benjamin Hume and Christian Voolstra for guidance with the SymPortal pipeline and Arun Nagarajan for assistance with bioinformatical analysis.
The Supplementary Material for this article can be found online at: https://www.frontiersin.org/articles/10.3389/fmars.2019.00453/full#supplementary-material
DATA SHEET S1 | Raw data for chla content and symbiont counts.
DATA SHEET S2 | Raw data for behavior, medusae size, and photochemical efficiency.
DATA SHEET S3 | Supporting Statistical Analyses and Figures.
Aljbour, S. M., Zimmer, M., and Kunzmann, A. (2017). Cellular respiration, oxygen consumption, and trade-offs of the jellyfish Cassiopea sp. in response to temperature change. J. Sea Res. 128, 92–97. doi: 10.1016/j.seares.2017.08.006
Bahr, K. D., Jokiel, P. L., and Ku’ulei, S. R. (2016). Relative sensitivity of five Hawaiian coral species to high temperature under high-pCO2 conditions. Coral Reefs 35, 729–738. doi: 10.1007/s00338-016-1405-4
Baker, A. C., Glynn, P. W., and Riegl, B. (2008). Climate change and coral reef bleaching: an ecological assessment of long-term impacts, recovery trends and future outlook. Estuar. Coast. Shelf Sci. 80, 435–471. doi: 10.1016/j.ecss.2008.09.003
Barshis, D. J., Ladner, J. T., Oliver, T. A., Seneca, F. O., Traylor-Knowles, N., and Palumbi, S. R. (2013). Genomic basis for coral resilience to climate change. Proc. Natl. Acad. Sci. U.S.A. 110, 1387–1392. doi: 10.1073/pnas.1210224110
Berkelmans, R., and Van Oppen, M. J. (2006). The role of zooxanthellae in the thermal tolerance of corals: a ‘nugget of hope’for coral reefs in an era of climate change. Proc. R. Soc. Lond. B Biol. Sci. 273, 2305–2312. doi: 10.1098/rspb.2006.3567
Castillo, K. D., Ries, J. B., Weiss, J. M., and Lima, F. P. (2012). Decline of forereef corals in response to recent warming linked to history of thermal exposure. Nat. Clim. Change 2:756. doi: 10.1038/nclimate1577
Chaidez, V., Dreano, D., Agusti, S., Duarte, C. M., and Hoteit, I. (2017). Decadal trends in red sea maximum surface temperature. Sci. Rep. 7:8144. doi: 10.1038/s41598-017-08146-z
Chakravarti, L. J., Beltran, V. H., and Oppen, M. J. (2017). Rapid thermal adaptation in photosymbionts of reef-building corals. Glob. Change Biol. 23, 4675–4688. doi: 10.1111/gcb.13702
Cunning, R., Silverstein, R. N., and Baker, A. C. (2015). Investigating the causes and consequences of symbiont shuffling in a multi-partner reef coral symbiosis under environmental change. Proc. R. Soc. Lond. B Biol. Sci. 282:20141725. doi: 10.1098/rspb.2014.1725
Davis, K., Lentz, S., Pineda, J., Farrar, J., Starczak, V., and Churchill, J. (2011). Observations of the thermal environment on Red Sea platform reefs: a heat budget analysis. Coral Reefs 30, 25–36. doi: 10.1007/s00338-011-0740-8
DeMartini, E. E., Anderson, T. W., Kenyon, J. C., Beets, J. P., and Friedlander, A. M. (2010). Management implications of juvenile reef fish habitat preferences and coral susceptibility to stressors. Mar. Freshw. Res. 61, 532–540.
Denny, M. (2017). The fallacy of the average: on the ubiquity, utility and continuing novelty of Jensen’s inequality. J. Exp. Biol. 220, 139–146. doi: 10.1242/jeb.140368
Dunn, S., Thomason, J., Le Tissier, M., and Bythell, J. (2004). Heat stress induces different forms of cell death in sea anemones and their endosymbiotic algae depending on temperature and duration. Cell Death Diff. 11:1213. doi: 10.1038/sj.cdd.4401484
Fitt, W. K., Brown, B. E., Warner, M. E., and Dunne, R. P. (2001). Coral bleaching: interpretation of thermal tolerance limits and thermal thresholds in tropical corals. Coral Reefs 20, 51–65. doi: 10.1007/s003380100146
Fitt, W. K., and Costley, K. (1998). The role of temperature in survival of the polyp stage of the tropical rhizostome jellyfish Cassiopea xamachana. J. Exp. Mar. Biol. Ecol. 222, 79–91. doi: 10.1016/s0022-0981(97)00139-1
Fitt, W. K., Mcfarland, F., Warner, M. E., and Chilcoat, G. C. (2000). Seasonal patterns of tissue biomass and densities of symbiotic dinoflagellates in reef corals and relation to coral bleaching. Limnol. Oceanogr. 45, 677–685. doi: 10.4319/lo.2000.45.3.0677
Glynn, P. W. (1993). Coral reef bleaching: ecological perspectives. Coral Reefs 12, 1–17. doi: 10.1007/bf00303779
Grottoli, A. G., Warner, M. E., Levas, S. J., Aschaffenburg, M. D., Schoepf, V., McGinley, M., et al. (2014). The cumulative impact of annual coral bleaching can turn some coral species winners into losers. Glob. Change Biol. 20, 3823–3833. doi: 10.1111/gcb.12658
Hoegh-Guldberg, O., and Smith, G. J. (1989). The effect of sudden changes in temperature, light and salinity on the population density and export of zooxanthellae from the reef corals Stylophora pistillata Esper and Seriatopora hystrix Dana. J. Exp. Mar. Biol. Ecol. 129, 279–303. doi: 10.1016/0022-0981(89)90109-3
Horvath, K. M., Castillo, K. D., Armstrong, P., Westfield, I. T., Courtney, T., and Ries, J. B. (2016). Next-century ocean acidification and warming both reduce calcification rate, but only acidification alters skeletal morphology of reef-building coral Siderastrea siderea. Sci. Rep. 6:29613. doi: 10.1038/srep29613
Hughes, T. P., Anderson, K. D., Connolly, S. R., Heron, S. F., Kerry, J. T., Lough, J. M., et al. (2018). Spatial and temporal patterns of mass bleaching of corals in the Anthropocene. Science 359, 80–83. doi: 10.1126/science.aan8048
Hughes, T. P., Kerry, J. T., Álvarez-Noriega, M., Álvarez-Romero, J. G., Anderson, K. D., Baird, A. H., et al. (2017). Global warming and recurrent mass bleaching of corals. Nature. 543:373. doi: 10.1038/nature21707
Hume, B. C., D’angelo, C., Smith, E. G., Stevens, J. R., Burt, J., and Wiedenmann, J. (2015). Symbiodinium thermophilum sp. nov., a thermotolerant symbiotic alga prevalent in corals of the world’s hottest sea, the Persian/Arabian Gulf. Sci. Rep. 5:8562. doi: 10.1038/srep08562
Hume, B. C., Ziegler, M., Poulain, J., Pochon, X., Romac, S., Boissin, E., et al. (2018). An improved primer set and amplification protocol with increased specificity and sensitivity targeting the Symbiodinium ITS2 region. PeerJ. 6:e4816. doi: 10.7717/peerj.4816
Hume, B. C. C., Smith, E. D., Ziegler, M., Warrington, H. J. M., Burt, J. A., Lajeunesse, T. C., et al. (2019). ). SymPortal: a novel analytical framework and platform for coral algal symbiont next-generation sequencing ITS2 profiling. Mol. Ecol. Resour. 19, 1063–1080. doi: 10.1111/1755-0998.13004
Jiang, L., You-Fang, S., Yu-Yang, Z., Guo-Wei, Z., Li, X.-B., Mccook, L. J., et al. (2017). Impact of diurnal temperature fluctuations on larval settlement and growth of the reef coral Pocillopora damicornis. Biogeosciences 14:5741. doi: 10.5194/bg-14-5741-2017
Jokiel, P., and Coles, S. (1990). Response of Hawaiian and other Indo-Pacific reef corals to elevated temperature. Coral Reefs 8, 155–162. doi: 10.1007/bf00265006
Jones, R. J., Hoegh-Guldberg, O., Larkum, A. W., and Schreiber, U. (1998). Temperature-induced bleaching of corals begins with impairment of the CO2 fixation mechanism in zooxanthellae. Plant Cell Environ. 21, 1219–1230. doi: 10.1046/j.1365-3040.1998.00345.x
Kenkel, C. D., and Matz, M. V. (2016). Gene expression plasticity as a mechanism of coral adaptation to a variable environment. Nat. Ecol. Evol. 1:0014. doi: 10.1038/s41559-016-0014
Kingsolver, J. G., and Huey, R. B. (1998). Evolutionary analyses of morphological and physiological plasticity in thermally variable environments. Am. Zoologist 38, 545–560. doi: 10.1093/icb/38.3.545
Klein, S. G., Pitt, K. A., and Carroll, A. R. (2016a). Reduced salinity increases susceptibility of zooxanthellate jellyfish to herbicide toxicity during a simulated rainfall event. Environ. Pollut. 209, 79–86. doi: 10.1016/j.envpol.2015.11.012
Klein, S. G., Pitt, K. A., and Carroll, A. R. (2016b). Surviving but not thriving: inconsistent responses of zooxanthellate jellyfish polyps to ocean warming and future UV-B scenarios. Sci. Rep. 6:28859.
Klein, S. G., Pitt, K. A., Nitschke, M. R., Goyen, S., Welsh, D. T., Suggett, D. J., et al. (2017). Symbiodinium mitigate the combined effects of hypoxia and acidification on a non-calcifying cnidarian. Glob. Change Biol. 23, 3690–3703. doi: 10.1111/gcb.13718
Krueger, T., Hawkins, T. D., Becker, S., Pontasch, S., Dove, S., Hoegh-Guldberg, O., et al. (2015). Differential coral bleaching—contrasting the activity and response of enzymatic antioxidants in symbiotic partners under thermal stress. Comp. Biochem. Physiol. A Mol. Integr. Physiol. 190, 15–25. doi: 10.1016/j.cbpa.2015.08.012
Kürten, B., Zarokanellos, N. D., Devassy, R. P., El-Sherbiny, M. M., Struck, U., Capone, D. G., et al. (2019). Seasonal modulation of mesoscale processes alters nutrient availability and plankton communities in the Red Sea. Prog. Oceanogr. 173, 238–255. doi: 10.1016/j.pocean.2019.02.007
LaJeunesse, T. C., Parkinson, J. E., Gabrielson, P. W., Jeong, H. J., Reimer, J. D., Voolstra, C. R., et al. (2018). Systematic revision of symbiodiniaceae highlights the antiquity and diversity of coral endosymbionts. Curr. Biol. 28, 2570–2580. doi: 10.1016/j.cub.2018.07.008
Lampert, K. P. (2016). “Cassiopea and its zooxanthellae,” in The Cnidaria, Past, Present and Future eds S. Goffredo and Z. Dubinsky (Berlin: Springer), 415–423. doi: 10.1007/978-3-319-31305-4_26
Lampert, K. P., Bürger, P., Striewski, S., and Tollrian, R. (2012). Lack of association between color morphs of the Jellyfish Cassiopea andromeda and zooxanthella clade. Mar. Ecol. 33, 364–369. doi: 10.1111/j.1439-0485.2011.00488.x
Leichter, J. J., and Miller, S. L. (1999). Predicting high-frequency upwelling: spatial and temporal patterns of temperature anomalies on a Florida coral reef. Cont. Shelf Res. 19, 911–928. doi: 10.1016/s0278-4343(99)00004-7
Lesser, M. P. (1997). Oxidative stress causes coral bleaching during exposure to elevated temperatures. Coral Reefs 16, 187–192. doi: 10.1007/s003380050073
Lesser, M. P., and Farrell, J. H. (2004). Exposure to solar radiation increases damage to both host tissues and algal symbionts of corals during thermal stress. Coral Reefs 23, 367–377. doi: 10.1007/s00338-004-0392-z
Lewis, C. L., and Coffroth, M. A. (2004). The acquisition of exogenous algal symbionts by an octocoral after bleaching. Science 304, 1490–1492. doi: 10.1126/science.1097323
Lima, F. P., and Wethey, D. S. (2012). Three decades of high-resolution coastal sea surface temperatures reveal more than warming. Nat. Commun. 3:704. doi: 10.1038/ncomms1713
Logan, C. A., Dunne, J. P., Eakin, C. M., and Donner, S. D. (2014). Incorporating adaptive responses into future projections of coral bleaching. Glob. Change Biol. 20, 125–139. doi: 10.1111/gcb.12390
Mayfield, A. B., Chan, P.-H., Putnam, H. M., Chen, C.-S., and Fan, T.-Y. (2012). The effects of a variable temperature regime on the physiology of the reef-building coral Seriatopora hystrix: results from a laboratory-based reciprocal transplant. J. Exp. Biol. 215, 4183–4195. doi: 10.1242/jeb.071688
McGill, C. J., and Pomory, C. M. (2008). Effects of bleaching and nutrient supplementation on wet weight in the jellyfish Cassiopea xamachana (Bigelow) (Cnidaria: Scyphozoa). Mar. Freshw. Behav. Physiol. 41, 179–189. doi: 10.1080/10236240802369899
Middlebrook, R., Anthony, K. R., Hoegh-Guldberg, O., and Dove, S. (2010). Heating rate and symbiont productivity are key factors determining thermal stress in the reef-building coral Acropora formosa. J Exp. Biol. 213, 1026–1034. doi: 10.1242/jeb.031633
Morandini, A. C., Stampar, S. N., Maronna, M. M., and Da Silveira, F. L. (2017). All non-indigenous species were introduced recently? The case study of Cassiopea (Cnidaria: Scyphozoa) in Brazilian waters. J. Mar. Biol. Association U.K. 97, 321–328. doi: 10.1017/s0025315416000400
Mueller, P., Schile-Beers, L. M., Mozdzer, T. J., Chmura, G. L., Dinter, T., Kuzyakov, Y., et al. (2018). Global-change effects on early-stage decomposition processes in tidal wetlands–implications from a global survey using standardized litter. Biogeosciences. 15, 3189–3202. doi: 10.5194/bg-15-3189-2018
Muller-Parker, G., and Davy, S. K. (2001). Temperate and tropical algal-sea anemone symbioses. Invertebr. Biol. 120, 104–123. doi: 10.1111/j.1744-7410.2001.tb00115.x
Muscatine, L., and Porter, J. W. (1977). Reef corals: mutualistic symbioses adapted to nutrient-poor environments. Bioscience 27, 454–460. doi: 10.2307/1297526
Negri, A., Marshall, P., and Heyward, A. (2007). Differing effects of thermal stress on coral fertilization and early embryogenesis in four Indo Pacific species. Coral Reefs 26, 759–763. doi: 10.1007/s00338-007-0258-2
Ohdera, A. H., Abrams, M. J., Ames, C. L., Baker, D. M., Suescún-Bolívar, L. P., Collins, A. G., et al. (2018). Upside down but headed in the right direction: review of the highly versatile Cassiopea xamachana system. Front. Ecol. Evol. 6:35. doi: 10.3389/fevo.2018.00035
Oliver, T., and Palumbi, S. (2011). Do fluctuating temperature environments elevate coral thermal tolerance? Coral Reefs 30, 429–440. doi: 10.1371/journal.pone.0179753
Palumbi, S. R., Mcleod, K. L., and Grünbaum, D. (2008). Ecosystems in action: lessons from marine ecology about recovery, resistance, and reversibility. AIBS Bull. 58, 33–42. doi: 10.1641/b580108
Price, N. N., Martz, T. R., Brainard, R. E., and Smith, J. E. (2012). Diel variability in seawater ph relates to calcification and benthic community structure on coral reefs. Plos One 7:e43843. doi: 10.1371/journal.pone.0043843
Putnam, H., and Edmunds, P. (2008). “Responses of coral hosts and their algal symbionts to thermal heterogeneity,” in Proceedings of the 11th International Coral Reef Symposium, Fort Lauderdale.
Putnam, H. M., and Edmunds, P. J. (2011). The physiological response of reef corals to diel fluctuations in seawater temperature. J. Exp. Mar. Biol. Ecol. 396, 216–223. doi: 10.1016/j.jembe.2010.10.026
Ritchie, R. J. (2006). Consistent sets of spectrophotometric chlorophyll equations for acetone, methanol and ethanol solvents. Photosynth. Res. 89, 27–41. doi: 10.1007/s11120-006-9065-9
Rivest, E. B., Comeau, S., and Cornwall, C. E. (2017). The role of natural variability in shaping the response of coral reef organisms to climate change. Curr. Clim. Change Rep. 3, 271–281. doi: 10.1007/s40641-017-0082-x
Ruel, J. J., and Ayres, M. P. (1999). Jensen’s inequality predicts effects of environmental variation. Trends Ecol. Evol. 14, 361–366. doi: 10.1016/s0169-5347(99)01664-x
Safaie, A., Silbiger, N. J., Mcclanahan, T. R., Pawlak, G., Barshis, D. J., Hench, J. L., et al. (2018). High frequency temperature variability reduces the risk of coral bleaching. Nat. Commun. 9:1671. doi: 10.1038/s41467-018-04074-2
Schoepf, V., Stat, M., Falter, J. L., and Mcculloch, M. T. (2015). Limits to the thermal tolerance of corals adapted to a highly fluctuating, naturally extreme temperature environment. Sci. Rep. 5:17639. doi: 10.1038/srep17639
Thompson, D. M., and Van Woesik, R. (2009). Corals escape bleaching in regions that recently and historically experienced frequent thermal stress. Proc. R. Soc. Lond. B Biol. Sci. 276, 2893–2901. doi: 10.1098/rspb.2009.0591
Ulstrup, K. E., Berkelmans, R., Ralph, P. J., and Van Oppen, M. J. (2006). Variation in bleaching sensitivity of two coral species across a latitudinal gradient on the Great Barrier Reef: the role of zooxanthellae. Mar. Ecol. Progr. Ser. 314, 135–148. doi: 10.3354/meps314135
Warner, M. E., Fitt, W. K., and Schmidt, G. W. (1999). Damage to photosystem II in symbiotic dinoflagellates: a determinant of coral bleaching. Proc. Natl. Acad. Sci. 96, 8007–8012. doi: 10.1073/pnas.96.14.8007
Wooldridge, S. A. (2013). Breakdown of the coral-algae symbiosis: towards formalising a linkage between warm-water bleaching thresholds and the growth rate of the intracellular zooxanthellae. Biogeosciences 10, 1647–1658. doi: 10.5194/bg-10-1647-2013
Keywords: Cassiopea sp., holobiont, bleaching, warming, temperature oscillations, diel variability, temperature variability, climate change
Citation: Klein SG, Pitt KA, Lucas CH, Hung S-H, Schmidt-Roach S, Aranda M and Duarte CM (2019) Night-Time Temperature Reprieves Enhance the Thermal Tolerance of a Symbiotic Cnidarian. Front. Mar. Sci. 6:453. doi: 10.3389/fmars.2019.00453
Received: 17 March 2019; Accepted: 08 July 2019;
Published: 25 July 2019.
Edited by:
Christopher Edward Cornwall, Victoria University of Wellington, New ZealandReviewed by:
Sarah Whitney Davies, The University of North Carolina at Chapel Hill, United StatesCopyright © 2019 Klein, Pitt, Lucas, Hung, Schmidt-Roach, Aranda and Duarte. This is an open-access article distributed under the terms of the Creative Commons Attribution License (CC BY). The use, distribution or reproduction in other forums is permitted, provided the original author(s) and the copyright owner(s) are credited and that the original publication in this journal is cited, in accordance with accepted academic practice. No use, distribution or reproduction is permitted which does not comply with these terms.
*Correspondence: Shannon G. Klein, c2hhbm5vbi5rbGVpbkBrYXVzdC5lZHUuc2E=
Disclaimer: All claims expressed in this article are solely those of the authors and do not necessarily represent those of their affiliated organizations, or those of the publisher, the editors and the reviewers. Any product that may be evaluated in this article or claim that may be made by its manufacturer is not guaranteed or endorsed by the publisher.
Research integrity at Frontiers
Learn more about the work of our research integrity team to safeguard the quality of each article we publish.