- 1Department of Marine Ecology, Faculty of Biology and Chemistry, University of Bremen, Bremen, Germany
- 2Red Sea Research Center, Division of Biological and Environmental Science and Engineering, King Abdullah University of Science and Technology, Thuwal, Saudi Arabia
- 3Department of Animal Ecology and Systematics, Justus Liebig University Giessen, Giessen, Germany
- 4Department of Biology, University of Konstanz, Konstanz, Germany
Microbial dinitrogen (N2) fixation (diazotrophy) is a trait critical for coral holobiont functioning. The contribution of N2 fixation to holobiont nitrogen (N) supply likely depends on the ecological niche of the coral holobiont. Consequently, coral-associated diazotroph communities may exhibit distinct activity patterns across a water depth gradient. We thus compared relative abundances of diazotrophs in the tissues of two common hard coral species, Podabacia sp. and Pachyseris speciosa, along their water depth distribution (10–30 m and 30–50 m, respectively) in the Central Red Sea. The relative gene copy numbers of the nifH gene (i.e., referenced against the eubacterial 16S rRNA gene), as a proxy for N2 fixation potential, were assessed via quantitative PCR. We hypothesized that relative nifH gene copy numbers would decrease with water depth, assuming a related shift from autotrophy to heterotrophy. Findings confirmed this hypothesis and revealed that nifH gene abundances for both corals decreased by ∼97% and ∼90% from the shallowest to the deepest collection site. However, this result was not significant for Pachyseris speciosa due to high biological variability. The observed decrease in nifH gene abundances may be explained by the relative increase in heterotrophy of the coral animal at increasing water depths. Our results underline the importance of interpreting microbial functions and associated nutrient cycling processes within the holobiont in relation to water depth range reflecting steep environmental gradients.
Introduction
Scleractinian corals are associated with a diverse microbial community. This microbiome is comprised of dinoflagellate algae of the family Symbiodiniaceae (LaJeunesse et al., 2018) and a multitude of other eukaryotic and prokaryotic microbes that together form a meta-organism, the coral holobiont (Rohwer et al., 2002). Coral-associated microbiomes are host-specific, and provide key functions to the holobiont, such as provision and cycling of essential nutrients (among others) (Falkowski et al., 1984; Rohwer et al., 2002; Wegley et al., 2007; Rädecker et al., 2015; Hernandez-Agreda et al., 2018). Among these, diazotrophy or biological fixation of dinitrogen (N2), a functional trait associated with diverse Bacteria and Archaea, i.e., diazotrophs, is associated with a broad range of scleractinian corals (Shashar et al., 1994; Lesser et al., 2007; Lema et al., 2012, 2014; Rädecker et al., 2014; Pogoreutz et al., 2017a,b). Diazotrophy, along with other nitrogen (N) cycling pathways, was proposed central to the exceptional adaptation of scleractinian corals to oligotrophic environments (Shashar et al., 1994; Lesser, 2004; Lesser et al., 2007; Rädecker et al., 2015). Indeed, coral-associated N2 fixation provides an important source of N for Symbiodiniaceae (Lesser et al., 2007; Cardini et al., 2015; Benavides et al., 2016; Bednarz et al., 2017), thereby helping to sustain holobiont productivity in shallow water corals when nutrients are scarce (Lesser et al., 2007; Cardini et al., 2015; Bednarz et al., 2017).
Microbial dinitrogen fixation is catalyzed by the nitrogenase enzyme complex, the activity of which is affected by its abiotic environment. Elevated levels of dissolved oxygen (O2) for instance can irreversibly deactivate dinitrogenase reductase, one of the two subunits of the enzyme complex (Compaoré and Stal, 2010), thereby hampering N2 fixation activity. Further, the enzymatic activity of the nitrogenase complex increases with temperature (Compaoré and Stal, 2010) and is hypothesized to decrease with increased environmental availability of bioavailable N [commonly referred to as “ammonia switch-off”; (Kessler et al., 2001)]. As O2 availability within the coral tissues can exhibit considerable diurnal variation due to high rates of photosynthesis by Symbiodiniaceae during the day (Sorek et al., 2013), irradiance may be a critical driver of coral-associated N2 fixation (Bednarz et al., 2017). These environmental parameters are subject to temporal and spatial change on a coral reef. Thus, to meet the metabolic carbon (C) and N requirements of the holobiont, scleractinian corals exhibit shifts in resource partitioning and may display variable levels of heterotrophy (Muscatine and Kaplan, 1994; Bednarz et al., 2017; Williams et al., 2018).
Acquisition of N through N2 fixation in the coral holobiont is an energetically costly process that is likely fueled by photosynthetically fixed C (photosynthate) of the Symbiodiniaceae (Cardini et al., 2015). Given the attenuation of solar irradiance with depth in the water column (Lesser et al., 2009; Mass et al., 2010; Bednarz et al., 2018), autotrophic potential of the coral holobiont potentially decreases with depth as well, making the holobiont rely more on heterotrophy to fulfill its energy requirements (Ferrier-Pagès et al., 2003; Lesser et al., 2010). As heterotrophic food sources have a higher N content than photosynthate provided by Symbiodiniaceae, the N demand of the coral holobiont may decrease with increasing heterotrophy (Dubinsky and Jokiel, 1994). In this light, Pogoreutz et al. (2017b) showed a negative correlation of N2 fixation activity with heterotrophic potential of scleractinian corals by using relative nifH gene copy numbers as a proxy for N2 fixation potential. Thus, we hypothesized that corals at the shallower end compared to their deeper vertical distribution ranges will exhibit differential relative N2 fixation activity, i.e., lower relative N2 fixation activity with increasing depths. In order to test this hypothesis, we (i) assessed diazotroph abundances in two Red Sea corals, the mushroom coral Podabacia sp. (Fungiidae) and Pachyseris speciosa (Agariciidae), along a depth gradient spanning 10–50 m, and (ii) assessed the correlation of environmental parameters along the corals’ depth gradient with the observed depth-dependent patterns of diazotroph abundance. To assess the relative abundance of diazotrophs in the coral microbiome, relative nifH gene copy numbers were obtained via quantitative PCR of the nifH gene normalized to the 16S rRNA gene (Pogoreutz et al., 2017a,b).
Materials and Methods
Coral Collection
Two species of scleractinian coral, Podabacia sp. and Pachyseris speciosa, were sampled using SCUBA and technical diving at the midshore reef Al Fahal (N22°18′19.98′′, E38°57′46.08′′) in the Saudi Arabian central Red Sea in November 2012 (Ziegler et al., 2015). The Saudi Arabian Coastguard Authority issued sailing permits to the site that include coral collection. Both corals are listed as “least concern” according to the IUCN Red List of Threatened Species (Hoeksema et al., 2014a,b). One fragment from the central upward-facing surface per colony (n = 3–5) of both species were sampled in 10 m intervals from 10 to 50 m water depth. The depths at which the corals were sampled reflects the main distribution of each coral species at our sampling site (see sample distribution in Table 1).

Table 1. Distribution and number of samples used to assess diazotroph abundances associated with the tissues of two species of reef-building corals along a water depth gradient in the central Red Sea.
Quantification of Diazotroph Communities
Coral tissue was removed from snap-frozen fragments with ice cold 4% NaCl solution using a standard airgun and an airbrush (Airbrush-starter-set, Conrad Electronic SE, Germany). Tissue slurries were homogenized and stored at -20°C until further processing. DNA was extracted from coral tissue slurries using the Qiagen DNeasy Plant Mini Kit (Qiagen, Germany) according to manufacturer’s instructions. For DNA extraction, 100 μl aliquots of each tissue slurry sample were used in 300 μl AP-1 buffer. Extracted DNA was quantified using a Qubit fluorometer (Thermo Fisher Scientific, United States) and adjusted to a DNA concentration of 5 ng μl-1.
To determine coral tissue-associated diazotroph abundances [as a proxy for N2 fixation potential (Pogoreutz et al., 2017b)], relative gene copy numbers were quantified for the prokaryotic nifH gene (as the target gene) and the bacterial 16S rRNA (as a reference gene and a proxy for the total bacterial community) as reported previously (Pogoreutz et al., 2017a). The 16S rRNA gene was selected as a reference gene in the present study as it exhibited stable relative gene copy numbers in coral tissues across the entire water depth distribution range (see Supplementary Figure S1). Relative quantification was achieved with quantitative PCR (qPCR) in triplicate reactions with the Platinum SYBR Green qPCR SuperMix-UDG (Invitrogen, United States) according to manufacturer’s instructions. Amplifications were performed with 5 μl SuperMix, 0.2 μL ROX reference dye, 0.2 μL of each 10 μM primer, 1 μL of input DNA template, and RNAse-free water to adjust the reaction volume to 10 μL. To amplify the bacterial 16S rRNA gene, the primers 781F (5′- TCGTCGGCAGCGTCAGATGTGTATAAGAGACAG -3′) and 1061R (5′- GTCTCGTGGGCTCGGAGATGTGTATAAGAGACA -3′) were used (Andersson et al., 2008). This primer pair includes the region V6 and covers the position 781 to 1,060. For amplification of the nifH gene, the degenerate primer pair F2 (5′-TGYGAYCCIAAIGCIGA -3′) and R6 (5′- TCIGGIGARATGATGGC -3′) was used (Gaby and Buckley, 2012). This primer pair covers the position 115 to 473. The qPCR was run under the following thermal profile: 2 min at 50°C, 1 min at 94°C, followed by 50 cycles of 30 s at 94°C, 1 min at 51°C, 1 min at 72°C, and an extension cycle of 1 min at 72°C (Pogoreutz et al., 2017b). The specificity of the amplifications was confirmed by melting curve analysis. Standard calibration curves were run simultaneously covering 6 orders of magnitude (104–109 copies of template per assay for the 16S rRNA and nifH gene). The qPCR efficiency (E) was >85% for both primers, calculated according to the equation E = [10(-1/slope)-1]. Relative fold change of nifH gene copy numbers was calculated for all depths and both species as 2(-ΔΔCt) using samples from the deepest sampling location of the respective species as a reference. Different depths at which both corals were collected (overlap only at 30 m) precluded direct comparisons of relative nifH gene abundances between both species.
Environmental Parameters
Environmental parameters at the collection site were collected on 3 days (within 10 days of each other) around noon in September 2012. All measuring procedures and environmental data were previously described and published in Ziegler et al. (2015). Briefly, a conductivity-temperature-depth recorder (CTD; SBE 16plusV2, Seabird Electronics, United States) was deployed to 50 m depth in close proximity to the sampling location measuring water temperature, PAR, O2 saturation, salinity, turbidity, and chlorophyll concentration approximately every 10 m. Measurements at each depth lasted approximately 1 min.
Statistical Analyses
Data were analyzed using non-parametric permutational multivariate analysis of variance (PERMANOVA) using PRIMER-E version 6 software (Clarke and Gorley, 2006) with the PERMANOVA+ add on (Anderson, 2001). To test for differences in relative nifH gene copy numbers per species and individual environmental parameters across depths, 1-factorial PERMANOVAs were performed, based on Euclidean distances of normalized and/or square-root transformed data. Type I (sequential) sum of squares was used with unrestricted permutation of raw data (999 permutations) and PERMANOVA pairwise tests with parallel Monte Carlo tests were carried out when significant differences occurred.
To identify the environmental parameter that “best explains” the multivariate physiological pattern of the coral samples, a biota-environmental matching (BIOENV) routine was computed with 999 permutations based on Euclidean distances. This analysis maximizes Spearman rank correlations between the resemblance matrices through permutation of all trial variables. Salinity and turbidity were omitted from the analysis due to their small-scale differences along the depth gradient. Input data were normalized prior to analysis by subtracting the variable’s mean from each value and dividing it by the standard deviation using PRIMER normalization option. Finally, a correlation with depth for Symbiodiniaceae cell density of the investigated coral species [data obtained from Ziegler et al. (2015)] and relative nifH gene copy numbers was determined via linear regression using SigmaPlot 12 (Systat software).
Results
Coral Diazotroph Abundances Along a Depth Gradient
The qPCR results confirmed the presence of the nifH gene in the microbial communities associated with both investigated Red Sea coral species and across the entire distribution range of sampling depths (Figure 1). Despite belonging to two different coral families (Fungiidae and Agariciidae) and exhibiting differences in water depth distribution range, the two coral species exhibited a similar pattern: relative nifH gene copy numbers for each coral species were highest at the two shallowest water depths and decreased at the greatest water depth (Figure 1). Significant differences with water depth were found for the mushroom coral Podabacia sp. (PERMANOVA, pseudo-F = 53.2, p < 0.001; Table 2), which exhibited significantly higher numbers of relative nifH gene copies at 10 and 20 m water depth (38-fold and 23-fold, respectively), compared to their conspecifics sampled at 30 m (pair-wise PERMANOVA, 10–30 m: t = 8.61, p = 0.004; 20–30 m: t = 10.82, p < 0.001; Figure 1A). These fold-changes correspond to a decrease in relative nifH gene copy numbers of 97% with water depth. Pachyseris speciosa exhibited 9-fold and 11-fold higher numbers of relative nifH gene copies at 30 and 40 m, respectively, compared to 50 m, but these differences were statistically not significant (PERMANOVA, pseudo-F = 1.69, p = 0.230; Figure 1B and Table 2). These fold-changes correspond to a decrease in relative nifH gene copy numbers of 90% with water depth. Overall, variation of biological replicates was greater in Pachyseris speciosa than in Podabacia sp. (Figure 1).
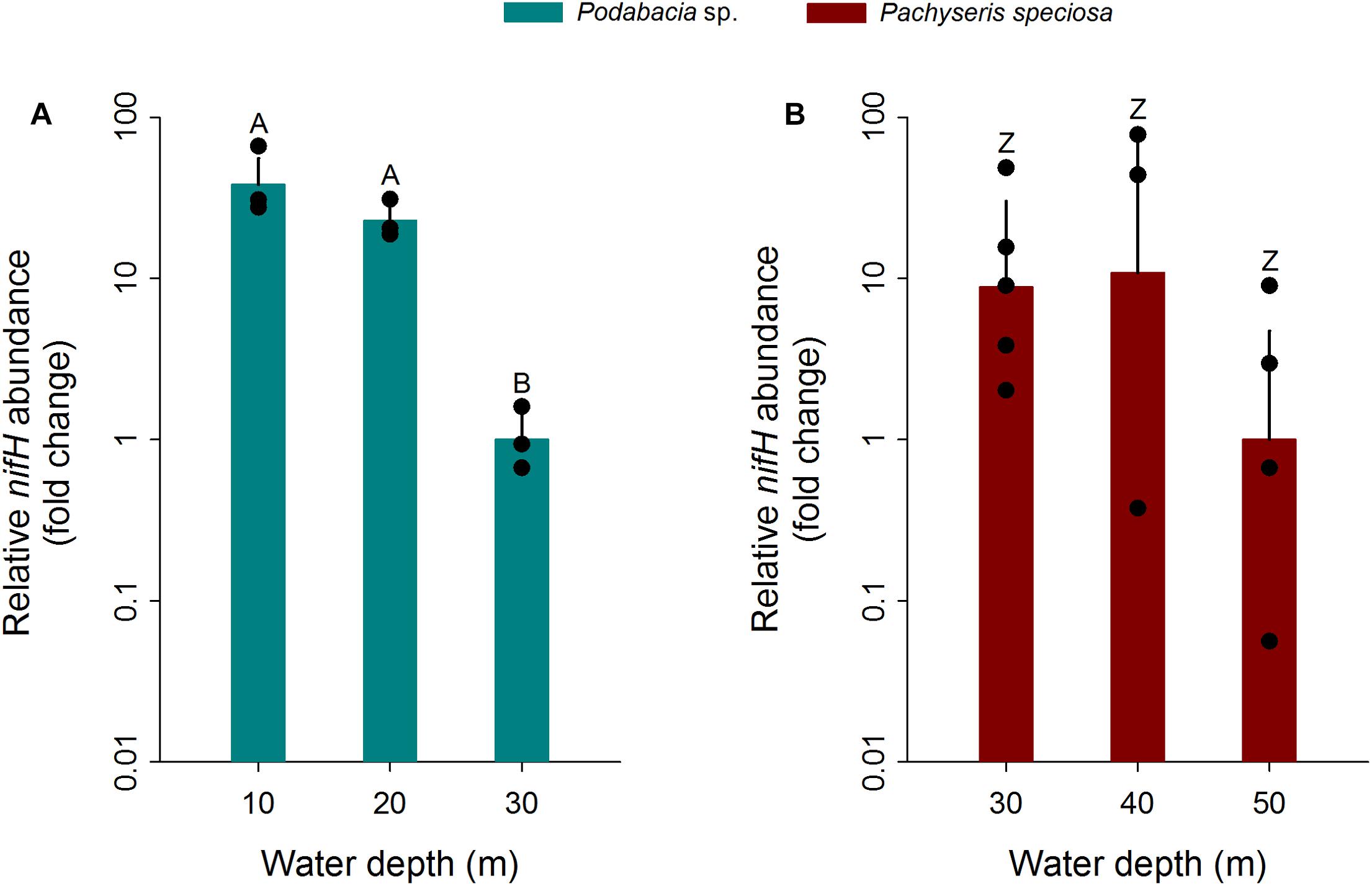
Figure 1. Fold changes in relative nifH gene copy numbers normalized to 16S rRNA gene copy numbers in the tissues of two Red Sea scleractinian corals (A) Podabacia sp. and (B) Pachyseris speciosa (n = 3–5) depending on water depth. Fold changes were calculated in relation to the greatest collection depth of the respective species; error bars indicate upper confidence intervals (+ 1 SE). Black circles represent data points. Different letters above bars indicate significant differences within species (p < 0.01). As both corals were collected at different depths (limited overlap only at 30 m), direct comparisons of relative nifH gene abundances between both species were precluded.
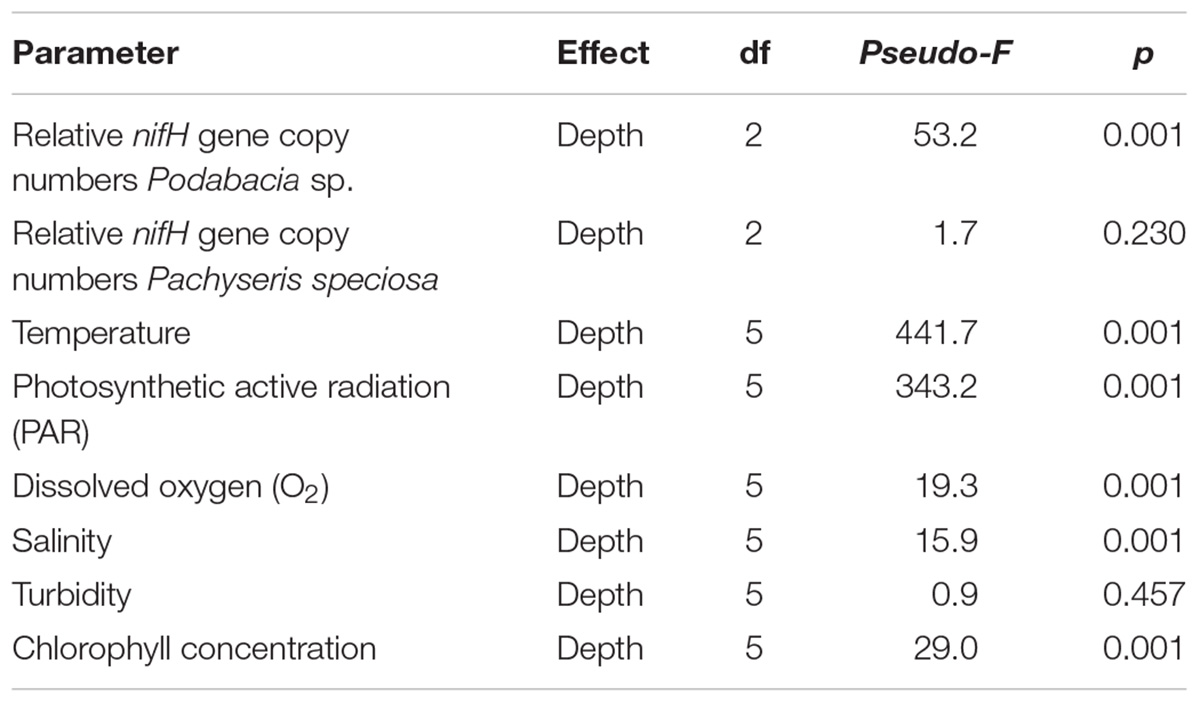
Table 2. Summary of depth effects on relative nifH gene copy numbers associated with the tissues of two Red Sea coral species and for environmental factors based on permutational multivariate analysis of variance (PERMANOVA).
Environmental Parameters
All environmental parameters, except for turbidity, showed significant differences with depth (PERMANOVA, p < 0.001; for full details, see Table 2). Temperature decreased by 3.8°C from 10 to 50 m water depth (30.1 and 26.3°C, respectively; Figure 2A) (pair-wise PERMANOVA, t = 40.21, p < 0.001). PAR exhibited an exponential decrease with water depth. Approximately 6% of the light available at 10 m water depth around noon (561 μmol photons m-2 s-1) penetrated down to 50 m water depth (35 μmol photons m-2 s-1) (Figure 2B). Both temperature and PAR decreased with each successive depth measurement (pair-wise PERMANOVA, p < 0.001). Dissolved O2 concentration decreased from 5.48 mg L-1 at 10 m to 5.28 mg L-1 at 50 m (pair-wise PERMANOVA, t = 10.51, p < 0.001; Figure 2C). Salinity decreased between 10 and 30 m from 39.4 PSU to 39.3 PSU (pair-wise PERMANOVA, t = 6.01, p < 0.001; Figure 2D), but increased again between 30 and 50 m from 39.3 PSU to 39.4 PSU (pair-wise PERMANOVA, t = 5.12, p < 0.001; Figure 2D). Turbidity remained stable along the water depth gradient (Figure 2E). Chlorophyll concentration increased significantly from 0.14 mg m-3 at 10 m to 0.72 mg m-3 at 50 m (pair-wise PERMANOVA, t = 16.90, p < 0.001; Figure 2F). See Table 3 for full details on pair-wise comparisons between all depths per environmental parameter.
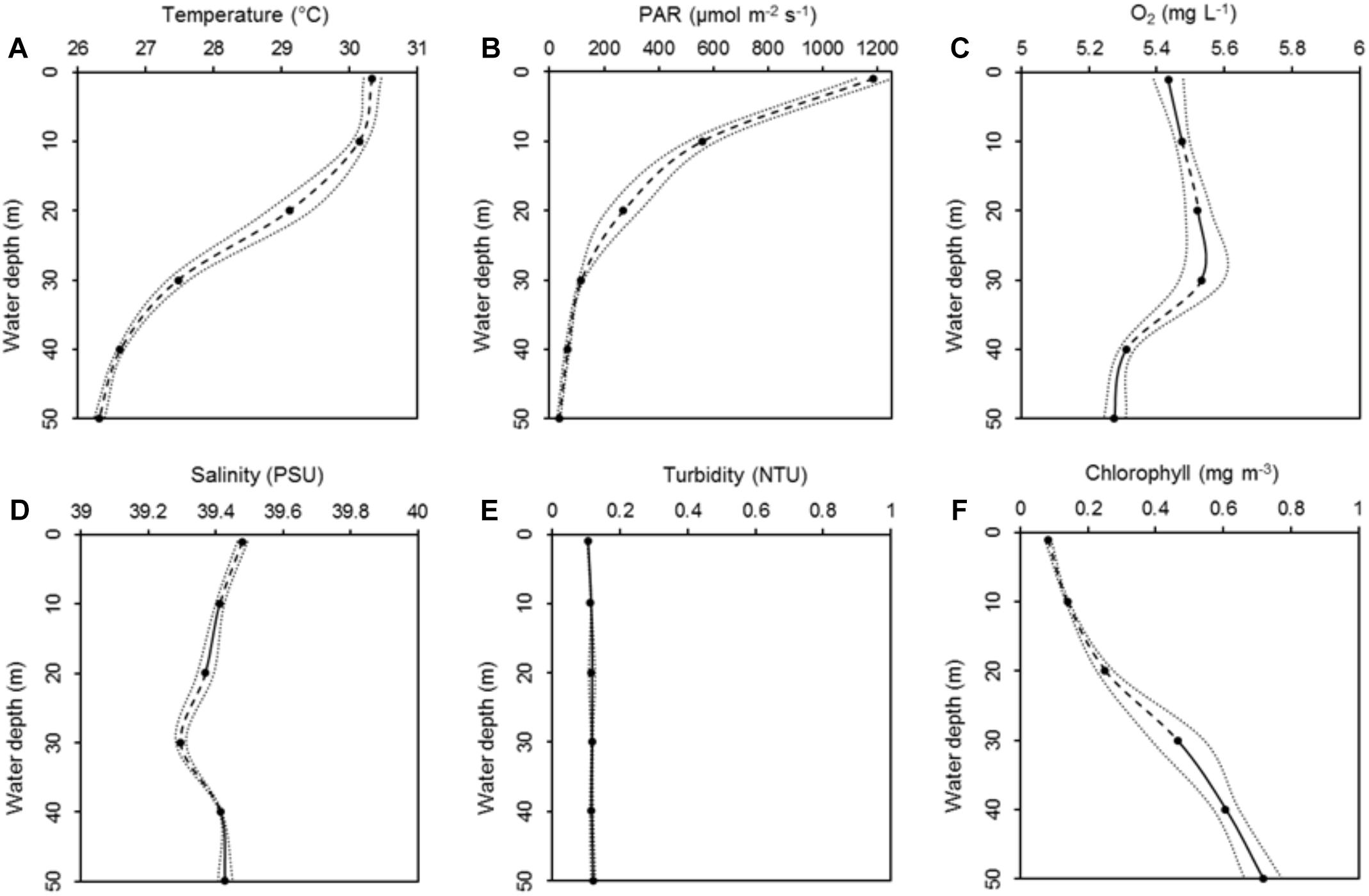
Figure 2. Means of six environmental parameters, i.e., temperature (A), photosynthetic active radiation (PAR) (B), dissolved oxygen (O2) (C), salinity (D), turbidity (E), and chlorophyll concentration (F), along a 50 m water depth gradient measured every 10 m. Data are presented as means ± 95% confidence intervals (dotted lines). Dashed lines represent significant differences between subsequent data points (p < 0.05). Solid lines represent non-significant differences between subsequent data points. Modified from Ziegler et al. (2015).
In general, environmental parameters changed more drastically along the depth distribution of Podabacia sp. compared to those along the depth distribution of Pachyseris speciosa: temperature decreased with 2.7 and 0.8°C, respectively; PAR decreased with 444 μmol m-2 s-1 and 82 μmol m-2 s-1, respectively; and chlorophyll concentrations increased by 0.33 mg m-3 and 0.25 mg m-3, respectively. Oxygen increased by 0.06 mg L-1 along the depth distribution of Podabacia sp., while it decreased with 0.26 mg L-1 along the depth distribution of Pachyseris speciosa.
Relationships of Relative nifH Gene Copy Numbers With Environmental Parameters
No correlation was found between depth and Symbiodiniaceae cell density for Podabacia sp. (Linear Regression, F = 0.059, r2 = 0.008, p = 0.82; Figure 3A) and Pachyseris speciosa (Linear Regression, F = 0.006, r2 = 0.001, p = 0.94; Figure 3B). A negative correlation was found between depth and relative nifH gene copy numbers (Linear Regression, F = 12.1, r2 = 0.388, p = 0.003; Figure 4), i.e., decreasing relative nifH gene copy numbers with increasing depth. The environmental parameter that best explained this pattern in the BIOENV routine was temperature (r = 0.317, p < 0.01; Supplementary Figure S2), followed by chlorophyll concentration (r = 0.289), PAR (r = 0.269) and dissolved O2 (r = 0.105).
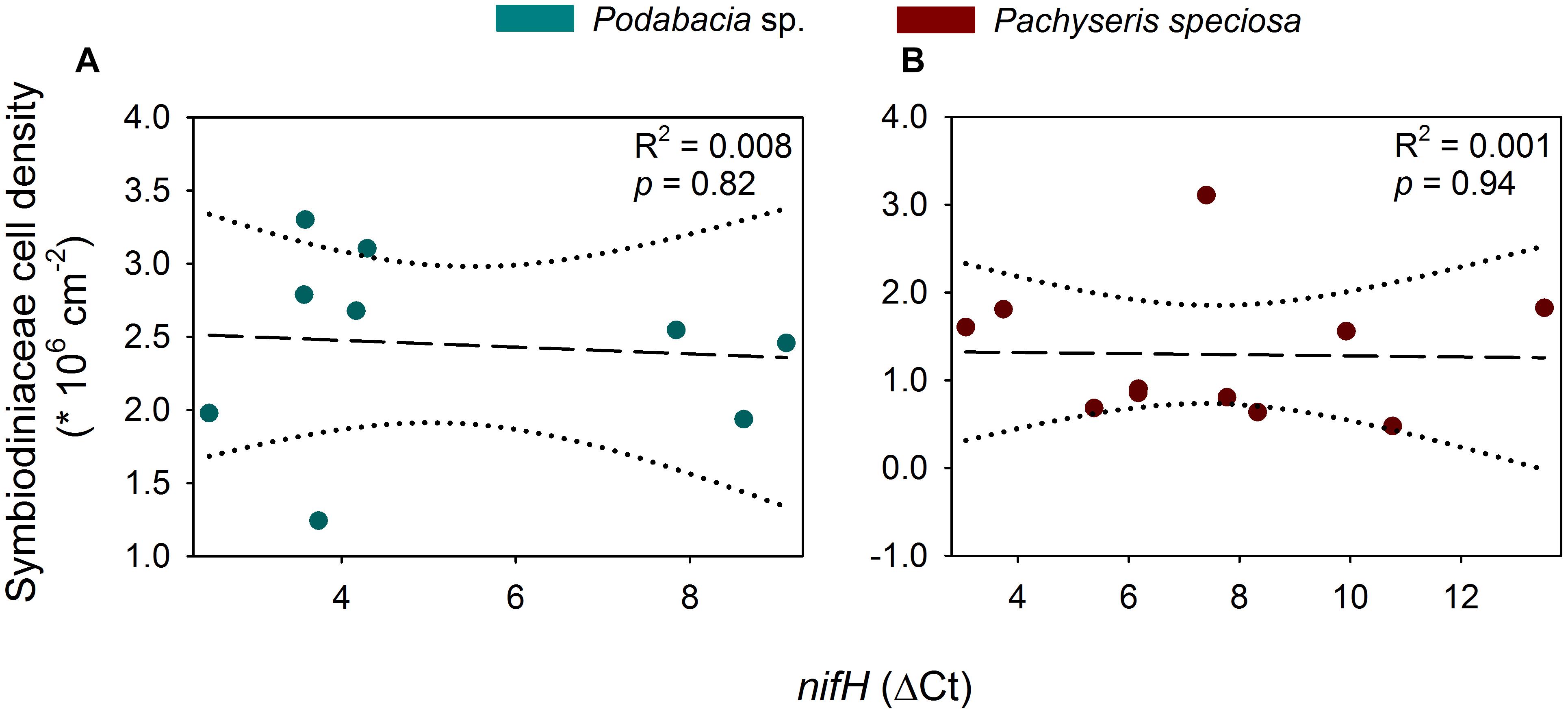
Figure 3. Linear regression analyses for cell density of Symbiodiniaceae and ΔCt of nifH (relative to the corresponding 16S rRNA Ct value) for Podabacia sp. (A) and Pachyseris speciosa (B). Dotted lines represent 95% confidence intervals. Symbiodiniaceae cell density data was obtained from Ziegler et al. (2015).
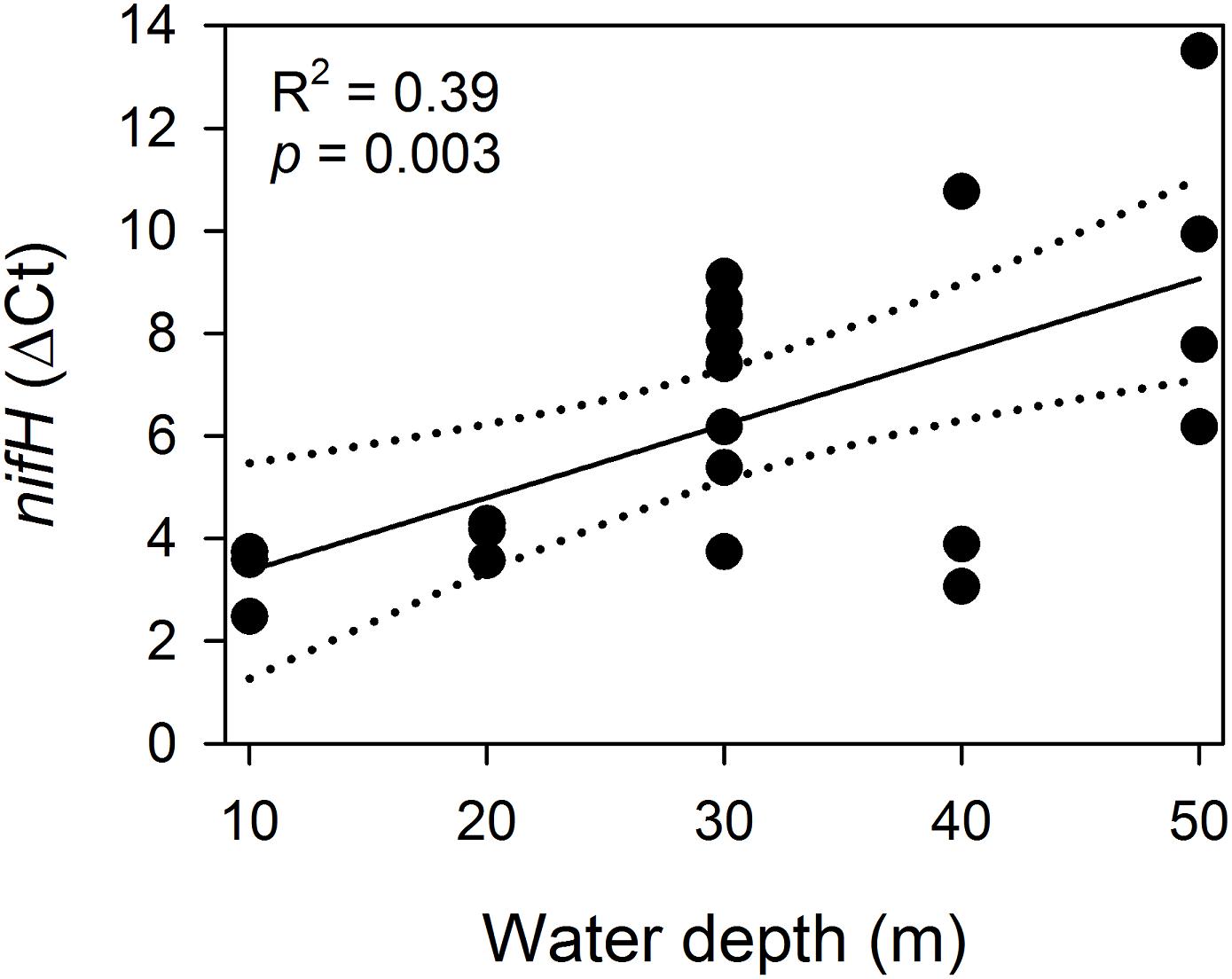
Figure 4. Linear regression analysis for ΔCt of nifH (relative to the corresponding 16S rRNA Ct value) and water depth. Dotted lines represent 95% confidence intervals. Note that lower ΔCt values correspond with higher relative nifH gene copy numbers.
Discussion
In order to explore patterns of N2 fixation activity along a depth gradient, we assessed relative nifH gene copy numbers in the tissues of two scleractinian corals species on a central Red Sea coral reef. Independent of their respective depth distribution range, which overlapped little, both coral species exhibited decreased relative nifH gene copy numbers at their greatest sampling depth. Temperature decreased from 30.1°C at 10 m water depth to 26.3°C at 50 m water depth and was, of the parameters tested, the environmental variable that best explained the observed patterns.
At the deepest sampling depths, nifH gene abundances for Podabacia sp. and Pachyseris speciosa were ∼97% and ∼90% lower, respectively, relative to conspecifics collected from shallower depths. This suggests a differential ecological dependence of these coral species on diazotrophy along their depth distribution range, as observed previously in other corals (Bednarz et al., 2017). This may be related to a shift from autotrophic to heterotrophic metabolism of the coral holobiont with increasing water depths. Indeed, coral species with higher heterotrophic potential may harbor lower abundances of diazotrophs in their tissue (Pogoreutz et al., 2017b). Furthermore, translocation of photosynthate from Symbiodiniaceae to the coral host, potentially fueling the energy-expensive activity of coral-associated diazotrophs (Pogoreutz et al., 2017a), are reported to decrease from >90% to around 30% along a (mesophotic) depth gradient in the coral Stylophora pistillata due to changes in holobiont resource partitioning (Muscatine et al., 1989). Thus, the observed decrease in relative nifH gene copy numbers, i.e., relative diazotroph abundances, with increasing water depth may indeed be linked to reduced photosynthate translocation by Symbiodiniaceae (Lesser et al., 2007; Bednarz et al., 2018). Other studies have reported increased dependence on heterotrophy by coral holobionts with increasing water depth, decreasing temperature and/or light availability (Anthony and Fabricius, 2000; Palardy et al., 2008; Einbinder et al., 2009; Ferrier-Pagès et al., 2011; Godinot et al., 2011; Mies et al., 2018; Williams et al., 2018). Thereby, heterotrophy could provide the coral holobiont with a large part of its nutrient demand at these greater depths (Houlbrèque and Ferrier-Pagès, 2009; Lesser et al., 2010; Wijgerde et al., 2011; Burmester et al., 2018). However, this depth-dependent rate of N2 fixation may be less pronounced when the supply of heterotrophic resources is not limited (Fox et al., 2018; Williams et al., 2018). It is therefore noteworthy to mention that data obtained here were collected in the highly oligotrophic Red Sea, with potentially limited heterotrophic resource abundance.
The results obtained here support the observation of low diazotroph abundances that align with increased heterotrophic potential. While previously investigated in a comparative taxonomic framework (Pogoreutz et al., 2017b), we here provide a spatial context. Both temperature and PAR decreased with depth at rates that are comparable to measurements in other coral reef systems (Frade et al., 2008; Mass et al., 2010; Schramek et al., 2018). Out of the measured parameters, temperature was the environmental variable that best explained the observed patterns in the present study. Indeed, temperature is a key environmental driver of N2 fixation activity and diazotroph abundance in scleractinian corals (Santos et al., 2014; Cardini et al., 2016; Pogoreutz et al., 2017a). Notably, seawater temperature decreased more strongly over the depth distribution range of Podabacia sp. than over the distribution range of Pachyseris speciosa. Thus, more pronounced differences in environment along the vertical depth distribution of Podabacia sp. compared to Pachyseris speciosa may explain the more pronounced differences in relative nifH relative gene copy numbers between sampling depths and coral species. Chlorophyll concentration was the second best environmental variable to explain the relative diazotrophic abundance pattern with depth. While no direct link between ambient chlorophyll concentrations and N2 fixation is known, the increasing chlorophyll concentration with depth could be due to increased nutrient availability (Tilstra et al., 2018), potentially leading to “ammonia switch-off” (Kessler et al., 2001), and/or increased abundance of zooplankton that graze on phytoplankton. Thus, potentially more N is available for heterotrophic uptake for the corals. This may, in part, explain the decreasing relative nifH gene copy numbers and supports the hypothesis of increased heterotrophic potential at greater depths.
Pachyseris speciosa appears to prefer a low light regime (DiPerna et al., 2018). Indeed, Pachyseris speciosa at our study site was only found in deeper (upper mesophotic) waters. Previously, Ziegler et al. (2015) demonstrated that the acclimatization potential of Red Sea corals with mesophotic distribution is related to their algal symbiont physiologies, which is first and foremost driven by PAR (and interactions of other environmental parameters). In the present study, relative nifH gene copy numbers decreased with decreasing PAR despite a lack of correlation with cell densities of their photosynthetic algal symbiont. PAR positively correlates with N2 fixation activity in benthic coral reef organisms (Bednarz et al., 2015; Tilstra et al., 2017), while increased O2 may negatively affect the nitrogenase enzyme complex (Compaoré and Stal, 2010). While the latter may in part explain the pattern observed in Podabacia sp. between 10 and 20 m water depth, the opposite effect is found at all other depths for both species. Additionally, many diazotrophs have mechanisms in place to protect them from the potentially harmful effect of O2 on the nitrogenase enzyme (Berman-Frank et al., 2001; Bednarz et al., 2018). Ambient dissolved O2 measured may therefore not be a main driver of relative nifH gene copy numbers in the present study.
It is worth mentioning that the central Red Sea experiences pronounced seasonal changes in environmental parameters (Sawall et al., 2014; Roik et al., 2016). The period of study (i.e., fall) is characterized by stratification with fairly stable environmental parameters (Roik et al., 2016), which is followed in winter by mixing and thus less pronounced differences in environmental parameters with depth. Thus, the results obtained here may not be representable for this site and these species throughout the entire year but merely during this season. Furthermore, the high variability of relative nifH gene copy numbers in Pachyseris speciosa may be due to the limited number of replicates used in the present study. For future research, this could be overcome by collecting a higher number of replicates.
In summary, our study suggests that corals in shallow water may rely more heavily on N acquisition through N2 fixation. In contrast, deep(er) water conspecifics likely rely more heavily on heterotrophy for nutrient acquisition, potentially conveying high intraspecific trophic plasticity. Results obtained here contribute to a growing body of knowledge highlighting fine-scale ecological differences of corals along their water depth distribution range (Rocha et al., 2018), and underline the importance of interpreting microbial functions and associated nutrient cycling processes within the holobiont in relation to water depth.
Author Contributions
CP, MZ, and CRV designed and conducted the experiments. CP analyzed the samples. AT and CP analyzed the data. AT wrote the manuscript. All authors revised drafts of the manuscript.
Funding
MZ was financially supported by a DAAD postgraduate research fellowship. Financial support was provided by KAUST baseline funds to CRV and the German Research Foundation (DFG) grant Wi 2677/9-1 to CW.
Conflict of Interest Statement
The authors declare that the research was conducted in the absence of any commercial or financial relationships that could be construed as a potential conflict of interest.
Acknowledgments
We thank KAUST CMOR staff and boat crews for their support with diving operations, in particular C. Walcher, D. Pallett, T. Sinclair-Taylor, T. Habis, and F. Mallon. We also thank C. Roder for assistance with coral collection and A. Baird for assistance in the taxonomic assignment of the coral species. We also thank the reviewers whose comments helped improve the manuscript.
Supplementary Material
The Supplementary Material for this article can be found online at: https://www.frontiersin.org/articles/10.3389/fmars.2019.00372/full#supplementary-material
FIGURE S1 | Ct values from quantitative PCR of 16S rRNA gene amplicons at three different water depths for Podabacia sp. (A) and Pachyseris speciosa (B). Black circles represent data points.
FIGURE S2 | Results of BIOENV analysis performed with PRIMER 6.
References
Anderson, M. J. (2001). A new method for non parametric multivariate analysis of variance. Austral. Ecol. 26, 32–46. doi: 10.1111/j.1442-9993.2001.01070.pp.x
Andersson, A. F., Lindberg, M., Jakobsson, H., Bäckhed, F., Nyrén, P., and Engstrand, L. (2008). Comparative analysis of human gut microbiota by barcoded pyrosequencing. PLoS One 3:e2836. doi: 10.1371/journal.pone.0002836
Anthony, K. R. N., and Fabricius, K. E. (2000). Shifting roles of heterotrophy and autotrophy in coral energetics under varying turbidity. J. Exp. Mar. Bio. Ecol. 252, 221–253. doi: 10.1016/S0022-0981(00)00237-239
Bednarz, V. N., Cardini, U., van Hoytema, N., Al-Rshaidat, M. M. D., and Wild, C. (2015). Seasonal variation in dinitrogen fixation and oxygen fluxes associated with two dominant zooxanthellate soft corals from the northern Red Sea. Mar. Ecol. Prog. Ser. 519, 141–152. doi: 10.3354/meps11091
Bednarz, V. N., Grover, R., Maguer, J-.F., Fine, M., and Ferrier-Pagès, C. (2017). The assimilation of diazotroph-derived nitrogen by scleractinian corals depends on their metabolic status. MBio 8, 1–14. doi: 10.1128/mBio.02058-16
Bednarz, V. N., Naumann, M. S., Cardini, U., van Hoytema, N., Rix, L., Al-Rshaidat, M. M. D., et al. (2018). Contrasting seasonal responses in dinitrogen fixation between shallow and deep-water colonies of the model coral Stylophora pistillata in the northern Red Sea. PLoS One 13:e0199022. doi: 10.1371/journal.pone.0199022
Benavides, M., Houlbrèque, F., Camps, M., Lorrain, A., Grosso, O., and Bonnet, S. (2016). Diazotrophs: a non-negligible source of nitrogen for the tropical coral Stylophora pistillata. J. Exp. Biol. 219, 2608–2612. doi: 10.1242/jeb.139451
Berman-Frank, I., Lundgren, P., Chen, Y.-B., Küpper, H., Kolber, Z., Bergman, B., et al. (2001). Segregation of nitrogen fixation and oxygenic photosynthesis in the marine cyanobacterium Trichodesmium. Science 294, 1534–1537. doi: 10.1126/science.1064082
Burmester, E. M., Breef-Pilz, A., Lawrence, N. F., Kaufman, L., Finnerty, J. R., and Rotjan, R. D. (2018). The impact of autotrophic versus heterotrophic nutritional pathways on colony health and wound recovery in corals. Ecol. Evol. 8, 10805–10816. doi: 10.1002/ece3.4531
Cardini, U., Bednarz, V. N., Naumann, M. S., van Hoytema, N., Rix, L., Foster, R. A., et al. (2015). Functional significance of dinitrogen fixation in sustaining coral productivity under oligotrophic conditions. Proc. R. Soc. B Biol. Sci. 282:20152257. doi: 10.1098/rspb.2015.2257
Cardini, U., van Hoytema, N., Bednarz, V. N., Rix, L., Foster, R. A., Al-Rshaidat, M. M. D., et al. (2016). Microbial dinitrogen fixation in coral holobionts exposed to thermal stress and bleaching. Environ. Microbiol. 18, 2620–2633. doi: 10.1111/1462-2920.13385
Clarke, K. R., and Gorley, R. N. (2006). PRIMER v6: Users Manual/Tutorial. Plymouth: Primer-E, 1–192.
Compaoré, J., and Stal, L. J. (2010). Effect of temperature on the sensitivity of nitrogenase to oxygen in two heterocystous cyanobacteria. J. Phycol. 46, 1172–1179. doi: 10.1111/j.1529-8817.2010.00899.x
DiPerna, S., Hoogenboom, M., Noonan, S., and Fabricius, K. (2018). Effects of variability in daily light integrals on the photophysiology of the corals Pachyseris speciosa and Acropora millepora. PLoS One 13:e0203882. doi: 10.1371/journal.pone.0203882
Dubinsky, Z., and Jokiel, P. L. (1994). Ratio of energy and nutrient fluxes regulates symbiosis between zooxanthellae and corals. Pacific Sci. 48, 313–324.
Einbinder, S., Mass, T., Brokovich, E., Dubinsky, Z., Erez, J., and Tchernov, D. (2009). Changes in morphology and diet of the coral Stylophora pistillata along a depth gradient. Mar. Ecol. Prog. Ser. 381, 167–174. doi: 10.3354/meps07908
Falkowski, P. G., Dubinsky, Z., Muscatine, L., and Porter, J. W. (1984). Light and the bioenergetics of a symbiotic coral. Bioscience 34, 705–709. doi: 10.2307/1309663
Ferrier-Pagès, C., Peirano, A., Abbate, M., Cocito, S., Negri, A., Rottier, C., et al. (2011). Summer autotrophy and winter heterotrophy in the temperate symbiotic coral Cladocora caespitosa. Limnol. Oceanogr. 56, 1429–1438. doi: 10.4319/lo.2011.56.4.1429
Ferrier-Pagès, C., Witting, J., Tambutté, E., and Sebens, K. P. (2003). Effect of natural zooplankton feeding on the tissue and skeletal growth of the scleractinian coral Stylophora pistillata. Coral Reefs 22, 229–240. doi: 10.1007/s00338-003-0312-317
Fox, M. D., Williams, G. J., Johnson, M. D., Radice, V. Z., Zgliczynski, B. J., Kelly, E. L. A., et al. (2018). Gradients in primary production predict trophic strategies of mixotrophic corals across spatial scales. Curr. Biol. 28, 3355.e–3363.e. doi: 10.1016/j.cub.2018.08.057
Frade, P. R., Bongaerts, P., Winkelhagen, A. J. S., Tonk, L., and Bak, R. P. M. (2008). In situ photobiology of corals over large depth ranges: a multivariate analysis on the roles of environment. Host Algal. Symbiont. Limnol. Oceanogr. 53, 2711–2723. doi: 10.4319/lo.2008.53.6.2711
Gaby, J. C., and Buckley, D. H. (2012). A comprehensive evaluation of PCR primers to amplify the nifh gene of nitrogenase. PLoS One 7:e42149. doi: 10.1371/journal.pone.0042149
Godinot, C., Grover, R., Allemand, D., and Ferrier-Pagès, C. (2011). High phosphate uptake requirements of the scleractinian coral Stylophora pistillata. J. Exp. Biol. 214, 2749–2754. doi: 10.1242/jeb.054239
Hernandez-Agreda, A., Leggat, W., Bongaerts, P., Herrera, C., and Ainsworth, T. D. (2018). Rethinking the coral microbiome: simplicity exists within a diverse microbial biosphere. MBio 9, e812–e818. doi: 10.1128/mBio.00812-818
Hoeksema, B. W., Rogers, A., and Quibilan, M. C. (2014a). Podabacia crustacea. IUCN Red List Threat. Species, e.T133158A54204422. Available at: http://dx.doi.org/10.2305/IUCN.UK.2014-1.RLTS.T133158A54204422.en (accessed September 6, 2018).
Hoeksema, B. W., Rogers, A., and Quibilan, M. C. (2014b). Pachyseris speciosa. IUCN Red List Threat. Species, e.T133410A54255088. Available at: http://dx.doi.org/10.2305/IUCN.UK.2014-1.RLTS.T133410A54255088.en (accessed September 6, 2018).
Houlbrèque, F., and Ferrier-Pagès, C. (2009). Heterotrophy in tropical scleractinian corals. Biol. Rev. 84, 1–17. doi: 10.1111/j.1469-185X.2008.00058.x
Kessler, P. S., Daniel, C., and Leigh, J. A. (2001). Ammonia switch-off of nitrogen fixation in the methanogenic archaeon Methanococcus maripaludis: mechanistic features and requirement for the novel GlnB homologues, Nifl1 and Nifl2. J. Bacteriol. 183, 882–889. doi: 10.1128/jb.183.3.882-889.2001
LaJeunesse, T. C., Parkinson, J. E., Gabrielson, P. W., Jeong, H. J., Reimer, J. D., Voolstra, C. R., et al. (2018). Systematic revision of Symbiodiniaceae highlights the antiquity and diversity of coral endosymbionts. Curr. Biol. 28, 2570.e–2580.e. doi: 10.1016/j.cub.2018.07.008
Lema, K. A., Bourne, D. G., and Willis, B. L. (2014). Onset and establishment of diazotrophs and other bacterial associates in the early life history stages of the coral Acropora millepora. Mol. Ecol. 23, 4682–4695. doi: 10.1111/mec.12899
Lema, K. A., Willis, B. L., and Bourne, D. G. (2012). Corals form characteristic associations with symbiotic nitrogen-fixing bacteria. Appl. Environ. Microbiol. 78, 3136–3144. doi: 10.1128/AEM.07800-7811
Lesser, M. P. (2004). Experimental biology of coral reef ecosystems. J. Exp. Mar. Bio. Ecol. 300, 217–252. doi: 10.1016/j.jembe.2003.12.027
Lesser, M. P., Falcón, L. I., Rodríguez-Román, A., Enríquez, S., Hoegh-Guldberg, O., and Iglesias-Prieto, R. (2007). Nitrogen fixation by symbiotic cyanobacteria provides a source of nitrogen for the scleractinian coral Montastraea cavernosa. Mar. Ecol. Prog. Ser. 346, 143–152. doi: 10.3354/meps07008
Lesser, M. P., Slattery, M., Stat, M., Ojimi, M., Gates, R. D., and Grottoli, A. (2010). Photoacclimatization by the coral Montastraea cavernosa in the mesophotic zone: light, food, and genetics. Ecology 91, 990–1003. doi: 10.1890/09-0313.1
Lesser, M. P., Slattery, M., and Leichter, J. J. (2009). Ecology of mesophotic coral reefs. J. Exp. Mar. Bio. Ecol. 375, 1–8. doi: 10.1016/j.jembe.2009.05.009
Mass, T., Kline, D. I., Roopin, M., Veal, C. J., Cohen, S., Iluz, D., et al. (2010). The spectral quality of light is a key driver of photosynthesis and photoadaptation in Stylophora pistillata colonies from different depths in the Red Sea. J. Exp. Biol. 213, 4084–4091. doi: 10.1242/jeb.039891
Mies, M., Güth, A. Z., Tenório, A. A., Banha, T. N. S., Waters, L. G., Polito, P. S., et al. (2018). In situ shifts of predominance between autotrophic and heterotrophic feeding in the reef-building coral Mussismilia hispida: an approach using fatty acid trophic markers. Coral Reefs 37, 677–689. doi: 10.1007/s00338-018-1692-z
Muscatine, L., and Kaplan, I. R. (1994). Resource partitioning by reef corals as determined from stable isotope composition. Pacific Sci. 48, 304–312. doi: 10.1007/BF00391957
Muscatine, L., Porter, J. W., and Kaplan, I. R. (1989). Resource partitioning by reef corals as determined from stable isotope composition. Mar. Biol. 100, 185–193. doi: 10.1007/BF00391957
Palardy, J. E., Rodrigues, L. J., and Grottoli, A. G. (2008). The importance of zooplankton to the daily metabolic carbon requirements of healthy and bleached corals at two depths. J. Exp. Mar. Bio. Ecol. 367, 180–188. doi: 10.1016/j.jembe.2008.09.015
Pogoreutz, C., Rädecker, N., Cárdenas, A., Gärdes, A., Voolstra, C. R., and Wild, C. (2017a). Sugar enrichment provides evidence for a role of nitrogen fixation in coral bleaching. Glob. Chang. Biol. 23, 3838–3848. doi: 10.1111/gcb.13695
Pogoreutz, C., Radecker, N., Cardenas, A., Gardes, A., Wild, C., Voolstra, C. R., et al. (2017b). Nitrogen fixation aligns with nifH abundance and expression in two coral trophic functional groups. Front. Microbiol. 8:1187. doi: 10.3389/fmicb.2017.01187
Rädecker, N., Meyer, F. W., Bednarz, V. N., Cardini, U., and Wild, C. (2014). Ocean acidification rapidly reduces dinitrogen fixation associated with the hermatypic coral Seriatopora hystrix. Mar. Ecol. Prog. Ser. 511, 297–302. doi: 10.3354/meps10912
Rädecker, N., Pogoreutz, C., Voolstra, C. R., Wiedenmann, J., and Wild, C. (2015). Nitrogen cycling in corals: the key to understanding holobiont functioning? Trends Microbiol. 23, 490–497. doi: 10.1016/j.tim.2015.03.008
Rocha, L. A., Pinheiro, H. T., Shepherd, B., Papastamatiou, Y. P., Luiz, O. J., Pyle, R. L., et al. (2018). Mesophotic coral ecosystems are threatened and ecologically distinct from shallow water reefs. Science 361, 281–284. doi: 10.1126/science.aaq1614
Rohwer, F., Seguritan, V., Azam, F., and Knowlton, N. (2002). Diversity and distribution of coral-associated bacteria. Mar. Ecol. Prog. Ser. 243, 1–10. doi: 10.3354/meps243001
Roik, A., Röthig, T., Roder, C., Ziegler, M., Kremb, S. G., and Voolstra, C. R. (2016). Year-long monitoring of physico-chemical and biological variables provide a comparative baseline of coral reef functioning in the central Red Sea. PLoS One 11:e0163939. doi: 10.1371/journal.pone.0163939
Santos, H. F., Carmo, F. L., Duarte, G., Dini-Andreote, F., Castro, C. B., Rosado, A. S., et al. (2014). Climate change affects key nitrogen-fixing bacterial populations on coral reefs. ISME J. 8, 2272–2279. doi: 10.1038/ismej.2014.70
Sawall, Y., Al-Sofyani, A., Banguera-Hinestroza, E., and Voolstra, C. R. (2014). Spatio-Temporal analyses of Symbiodinium physiology of the coral Pocillopora verrucosa along large-scale nutrient and temperature gradients in the Red Sea. PLoS One 9:e103179. doi: 10.1371/journal.pone.0103179
Schramek, T. A., Colin, P. L., Merrifield, M. A., and Terrill, E. J. (2018). Depth-dependent thermal stress around corals in the tropical Pacific Ocean. Geophys. Res. Lett. 45, 9739–9747. doi: 10.1029/2018GL078782
Shashar, N., Cohen, Y., Loya, Y., and Sar, N. (1994). Nitrogen fixation (acetylene reduction) in stony corals: evidence for coral-bacteria interactions. Mar. Ecol. Prog. Ser. 111, 259–264. doi: 10.3354/meps111259
Sorek, M., Yacobi, Y. Z., Roopin, M., Berman-Frank, I., and Levy, O. (2013). Photosynthetic circadian rhythmicity patterns of Symbiodium, the coral endosymbiotic algae. Proc. R. Soc. B Biol. Sci. 280:20122942. doi: 10.1098/rspb.2012.2942
Tilstra, A., Bednarz, V. N., Cardini, U., van Hoytema, N., Al-Rshaidat, M. M. D., and Wild, C. (2017). Seasonality affects dinitrogen fixation associated with two common macroalgae from a coral reef in the northern Red Sea. Mar. Ecol. Prog. Ser. 575, 69–80. doi: 10.3354/meps12206
Tilstra, A., van Hoytema, N., Cardini, U., Bednarz, V. N., Rix, L., Naumann, M. S., et al. (2018). Effects of water column mixing and stratification on planktonic primary production and dinitrogen fixation on a northern Red Sea coral reef. Front. Microbiol. 9:2351. doi: 10.3389/fmicb.2018.02351
Wegley, L., Edwards, R., Rodriguez-Brito, B., Liu, H., and Rohwer, F. (2007). Metagenomic analysis of the microbial community associated with the coral Porites astreoides. Environ. Microbiol. 9, 2707–2719. doi: 10.1111/j.1462-2920.2007.01383.x
Wijgerde, T., Diantari, R., Lewaru, M. W., Verreth, J. A. J., and Osinga, R. (2011). Extracoelenteric zooplankton feeding is a key mechanism of nutrient acquisition for the scleractinian coral Galaxea fascicularis. J. Exp. Biol. 214, 3351–3357. doi: 10.1242/jeb.058354
Williams, G. J., Sandin, S. A., Zgliczynski, B. J., Fox, M. D., Gove, J. M., Rogers, J. S., et al. (2018). Biophysical drivers of coral trophic depth zonation. Mar. Biol. 165:60. doi: 10.1007/s00227-018-3314-3312
Keywords: coral reefs, diazotrophy, heterotrophy, autotrophy, nitrogen fixation, depth gradient
Citation: Tilstra A, Pogoreutz C, Rädecker N, Ziegler M, Wild C and Voolstra CR (2019) Relative Diazotroph Abundance in Symbiotic Red Sea Corals Decreases With Water Depth. Front. Mar. Sci. 6:372. doi: 10.3389/fmars.2019.00372
Received: 02 January 2019; Accepted: 14 June 2019;
Published: 04 July 2019.
Edited by:
Michael Sweet, University of Derby, United KingdomReviewed by:
Julie L. Meyer, University of Florida, United StatesAlejandra Hernandez-Agreda, California Academy of Sciences, United States
Copyright © 2019 Tilstra, Pogoreutz, Rädecker, Ziegler, Wild and Voolstra. This is an open-access article distributed under the terms of the Creative Commons Attribution License (CC BY). The use, distribution or reproduction in other forums is permitted, provided the original author(s) and the copyright owner(s) are credited and that the original publication in this journal is cited, in accordance with accepted academic practice. No use, distribution or reproduction is permitted which does not comply with these terms.
*Correspondence: Christian Wild, Y2hyaXN0aWFuLndpbGRAdW5pLWJyZW1lbi5kZQ==; Christian R. Voolstra, Y2hyaXN0aWFuLnZvb2xzdHJhQGthdXN0LmVkdS5zYQ==; Y2hyaXMudm9vbHN0cmFAZ21haWwuY29t