- 1European Space Agency (ESA-ESRIN), Frascati, Italy
- 2LEGOS – Laboratoire d’Etudes en Géophysique et Océanographie Spatiales (Université Paul Sabatier (UPS), Centre National d’Études Spatiales (CNES), Centre National de la Recherche Scientifique (CNRS), Institut de Recherche pour le Développement (IRD)), Toulouse, France
- 3Istituto di Biofisica, Consiglio Nazionale delle Ricerche, Pisa, Italy
- 4Institute of Geodesy and Geoinformation, University of Bonn, Bonn, Germany
- 5Jet Propulsion Laboratory/California Institute of Technology, Pasadena, CA, United States
- 6DTU Space, Technical University of Denmark, Lyngby, Denmark
- 7Systèmes de Référence Temps Espace (SYRTE), Observatoire de Paris, Paris, France
- 8National Oceanography Centre, Liverpool, United Kingdom
- 9Institute of Space Sciences (ICE, CSIC), Spanish National Research Council, Institute of Space Studies of Catalonia (IEEC), Barcelona, Spain
- 10Telespazio VEGA UK for European Space Agency, Didcot, United Kingdom
- 11Bureau de Recherches Géologiques et Minières, Orléans, France
- 12Collecte Localisation Satellites, Toulouse, France
- 13Faculdade de Ciências, Universidade do Porto, Porto, Portugal
- 14Centro Interdisciplinar de Investigação Marinha e Ambiental, Matosinhos, Portugal
- 15School of Aeronautics and Astronautics, Purdue University, West Lafayette, IN, United States
- 16National Oceanography Centre, Southampton, United Kingdom
- 17Fisheries and Oceans Canada, Northwest Atlantic Fisheries Centre, St. John’s, NL, Canada
- 18Danish Meteorological Institute, Copenhagen, Denmark
- 19Rosenstiel School of Marine and Atmospheric Science, University of Miami, Miami, FL, United States
- 20Laboratory for Satellite Altimetry, National Oceanic and Atmospheric Administration, College Park, MD, United States
- 21University of the Littoral Opal Coast, University of Lille, National Center for Scientific Research, UMR 8187 Laboratoire d’Océanologie et de Géosciences, Wimereux, France
- 22Instituto Mediterráneo de Estudios Avanzados, University of the Balearic Islands–Spanish National Research Council, Esporles, Spain
- 23Mercator Ocean International, Ramonville-Saint-Agne, France
- 24Institut Mediterrani d’Estudis Avançats, Esporles, Spain
- 25Deutsches Geodätisches Forschungsinstitut, Technische Universität München, Munich, Germany
- 26European Organisation for the Exploitation of Meteorological Satellites (EUMETSAT), Darmstadt, Germany
- 27Laboratoire de Météorologie Dynamique, Ecole Normale Supérieure–Institut Pierre-Simon Laplace, Paris, France
- 28Department of Marine and Coastal Sciences, Rutgers University, New Brunswick, NJ, United States
- 29UMR 7266 Littoral, Environnement et Sociétés, National Center for Scientific Research–University of La Rochelle, La Rochelle, France
Coastal zones are highly dynamical systems affected by a variety of natural and anthropogenic forcing factors that include sea level rise, extreme events, local oceanic and atmospheric processes, ground subsidence, etc. However, so far, they remain poorly monitored on a global scale. To better understand changes affecting world coastal zones and to provide crucial information to decision-makers involved in adaptation to and mitigation of environmental risks, coastal observations of various types need to be collected and analyzed. In this white paper, we first discuss the main forcing agents acting on coastal regions (e.g., sea level, winds, waves and currents, river runoff, sediment supply and transport, vertical land motions, land use) and the induced coastal response (e.g., shoreline position, estuaries morphology, land topography at the land–sea interface and coastal bathymetry). We identify a number of space-based observational needs that have to be addressed in the near future to understand coastal zone evolution. Among these, improved monitoring of coastal sea level by satellite altimetry techniques is recognized as high priority. Classical altimeter data in the coastal zone are adversely affected by land contamination with degraded range and geophysical corrections. However, recent progress in coastal altimetry data processing and multi-sensor data synergy, offers new perspective to measure sea level change very close to the coast. This issue is discussed in much detail in this paper, including the development of a global coastal sea-level and sea state climate record with mission consistent coastal processing and products dedicated to coastal regimes. Finally, we present a new promising technology based on the use of Signals of Opportunity (SoOp), i.e., communication satellite transmissions that are reutilized as illumination sources in a bistatic radar configuration, for measuring coastal sea level. Since SoOp technology requires only receiver technology to be placed in orbit, small satellite platforms could be used, enabling a constellation to achieve high spatio-temporal resolutions of sea level in coastal zones.
Introduction
Today about 600 million people (mostly concentrated in several of the world’s largest megacities) live very close to the sea at an altitude less than 10 m and this number is expected to double by 2060 (Nicholls, 2010). In many of these regions, populations are exposed to a variety of natural hazards (e.g., extreme weather such as damaging cyclones and their associated storm surges), to the effects of global climate change (e.g., sea level rise), and to the impacts of human activities (e.g., urbanization). In low-lying coastal areas, several of these factors may combine to increase the risks significantly to coastal populations. For example, the risk of flooding and coastal erosion during extreme events, and of salt-water intrusion into rivers and coastal aquifers on which people depend, is exacerbated by climate-related sea level rise. Its negative impacts are amplified by land subsidence, caused by ground-water extraction in coastal megacities or oil exploration on shallow shelves. Coastal zones are already suffering ecological and biological stresses, for example poor water quality, pollution, destruction of marine ecosystems, etc. as a result of these strong anthropogenic pressures.
It has been reported that 24% of the world’s sandy beaches are eroding at a rate over 0.5 m per year (Luijendijk et al., 2018). This raises the issue of understanding the underlying causes. Shoreline change and coastal flooding are critical concerns for many coasts worldwide and they are expected to be strongly aggravated by sea-level rise (e.g., Ranasinghe, 2016; Arns et al., 2017; Vitousek et al., 2017; Vousdoukas et al., 2018). In the future, these risks are expected to increase due to the combined effects of climate change and human activities. The response of coastal environments to natural and anthropogenic forcing factors (including climate change) depends on the characteristics of the forcing agents, as well as on the internal properties of the coastal systems. These remain poorly known and mostly un-surveyed at global scale. Figure 1 summarizes the many processes currently affecting coastal zones.
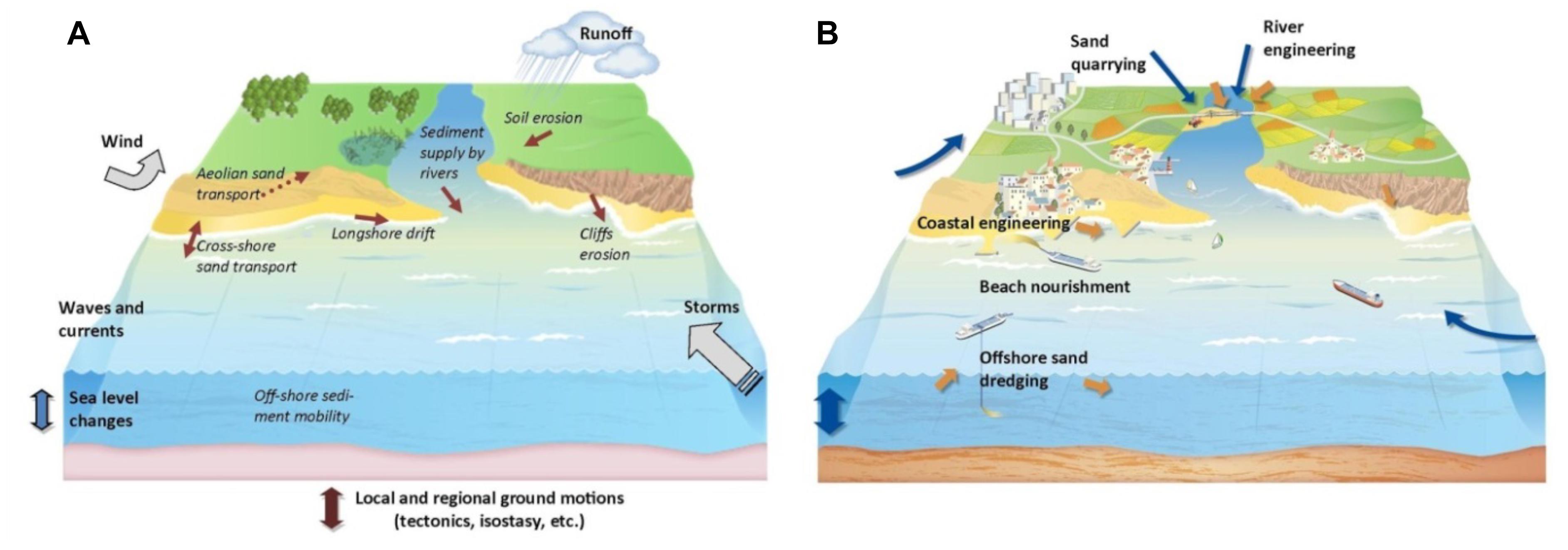
Figure 1. Natural (A) and anthropogenic (B) processes affecting shoreline changes (source: Cazenave and Le Cozannet, 2014).
Various types of long-term observations need to be collected and analyzed within an integrated framework, in order to: (1) gain information about the evolution of the coastal zones during the past few decades; (2) improve knowledge about the causes of this evolution, in particular sea level change, and (3) provide information to decision-makers and coastal zone managers.
In this article, we address the issue of coastal zones evolution in response to a variety of forcing agents. We propose a series of recommendations about systematic monitoring of coastal zones and associated observing systems needed to improve our understanding about the processes involved (see section “Monitoring Coastal Zones With Multiple Observing Systems”). However, monitoring of sea level in coastal areas is the main focus of the paper. Thus after a brief overview of the multiple factors affecting coastal zones and the need for monitoring them (see section “Monitoring Coastal Zones With Multiple Observing Systems”), a large part of the paper is devoted to the use of satellite altimetry to provide robust estimates of rates as close to the coast as possible (see section “Coastal Zone Altimetry Processing and Exploitation: Progress and Outlook for the Next Decade”). We also address new types of observations, namely the Ocean Surface Topography Using Wideband Signals of Opportunity (OST W-SoOp), which draws its heritage from altimetry concepts using the Global Navigation Satellite System Reflectometry (GNSS-R) technology and which is able to complement altimetry-based sea level measurements in coastal areas (see section “Wideband Signals of Opportunity Reflectometry for Coastal Ocean Applications”). In Section “Opportunities for Integration,” we discuss opportunities for integration and in Section “End Users Engagement,” we scrutinize how to engage end users.
Monitoring Coastal Zones With Multiple Observing Systems
Context
Coastal zones, where a large proportion of the world population lives, are under serious threat because of extreme events and flooding, urbanization, sand extraction, salinization of estuaries and of coastal aquifers, and shoreline erosion and retreat. These hazards are expected to increase due to the combined effects of sea level rise, climate change, and increase in human activities. The response of coastal environments to natural and direct/indirect anthropogenic forcing factors depend on the characteristics of the forcing agents, as well as on the internal properties of the highly dynamical coastal systems. The latter remain poorly known and mostly un-surveyed at a global scale. Coastal observations of various types need to be collected and analyzed to better understand changes affecting coastal zones and provide crucial information to decision-makers involved in adaptation and mitigation of environmental risks. The near-global observations from space of many coastal parameters are a fundamental complement to existing in situ coastal observing systems, which still remains relatively sparse (e.g., regional tide gauge networks, moorings, ship surveys, gliders) (Cazenave et al., 2017; Woodworth et al., 2017). In this section, we briefly review the need for systematic monitoring of the world coastal zones, with an emphasis on space observations of both forcing agents (e.g., sea level, winds, waves and currents, river runoff, sediment supply and transport, vertical land motions, land use) and coastal responses (e.g., shoreline position, estuaries morphology, land topography at the land–sea interface and coastal bathymetry).
Forcing Agents Affecting Coastal Zones and Observational Needs
Forcing agents acting on coastal zones include sea level change, extreme events, winds, waves and currents, vertical ground motions, sediment supply, river runoff, land use change and urbanization.
Mean Coastal Sea Level Variability and Change
Global mean sea level is currently rising and even accelerating (e.g., Cazenave et al., 2018; The WCRP Global Sea Level Budget Group, 2018). While satellite altimetry has considerably improved our understanding of sea level variations at global and regional scales, this is not the case for coastal areas. In terms of impacts, what counts at the coast is the total relative sea level, i.e., the sum of global mean rise, on which regional variability is superimposed over local ocean processes (e.g., shelf currents, tides, surges, ocean surface waves), and vertical land motions. Satellite altimetry, optimized for the open ocean, performs poorly in the last 20 km to the coast due to perturbing factors, including land contamination. Recent progress in reprocessing radar waveforms in coastal areas, and the use of new altimetry techniques (e.g., Ka–band altimetry and SAR mode), have enabled the development of new coastal altimetry datasets (Birol et al., 2017; Passaro et al., 2018). However, these products remain inhomogeneous in space and time, and efforts are needed to construct a consistent global gridded coastal altimetry database. A detailed discussion of coastal sea level monitoring by satellite altimetry is provided in Section “Coastal Zone Altimetry Processing and Exploitation: Progress and Outlook for the Next Decade.”
Extreme Water Levels
Extreme water levels result from a number of oceanic, atmospheric, and terrestrial processes. They generally occur from the combination of high astronomical tides, large storm surges, high mean sea level and strong waves. However, the importance of each of these factors varies around the world. Extreme levels due to the astronomical tides occur on timescales of approximately 4.5 and 18.6 years, respectively. Those due to storm surges and surface waves at mid and high latitudes occur primarily during winter. Seasonal variations result from surge-related water level changes above the mean sea level ‘baseline.’ At inter-annual to decadal timescale, there is strong influence of large-scale modes of climate variability, such as El Niño-Southern Oscillation (ENSO), the Pacific Decadal Oscillation (PDO) or the North Atlantic Oscillation (NAO). There are also higher frequency oscillations (in the order of a few minutes) that result from fast, abrupt changes in the atmospheric conditions combined with an amplified topographic response (i.e., seiches or meteo-tsunamis).
An example of extreme water level due to tide and surge is shown in Figure 2.
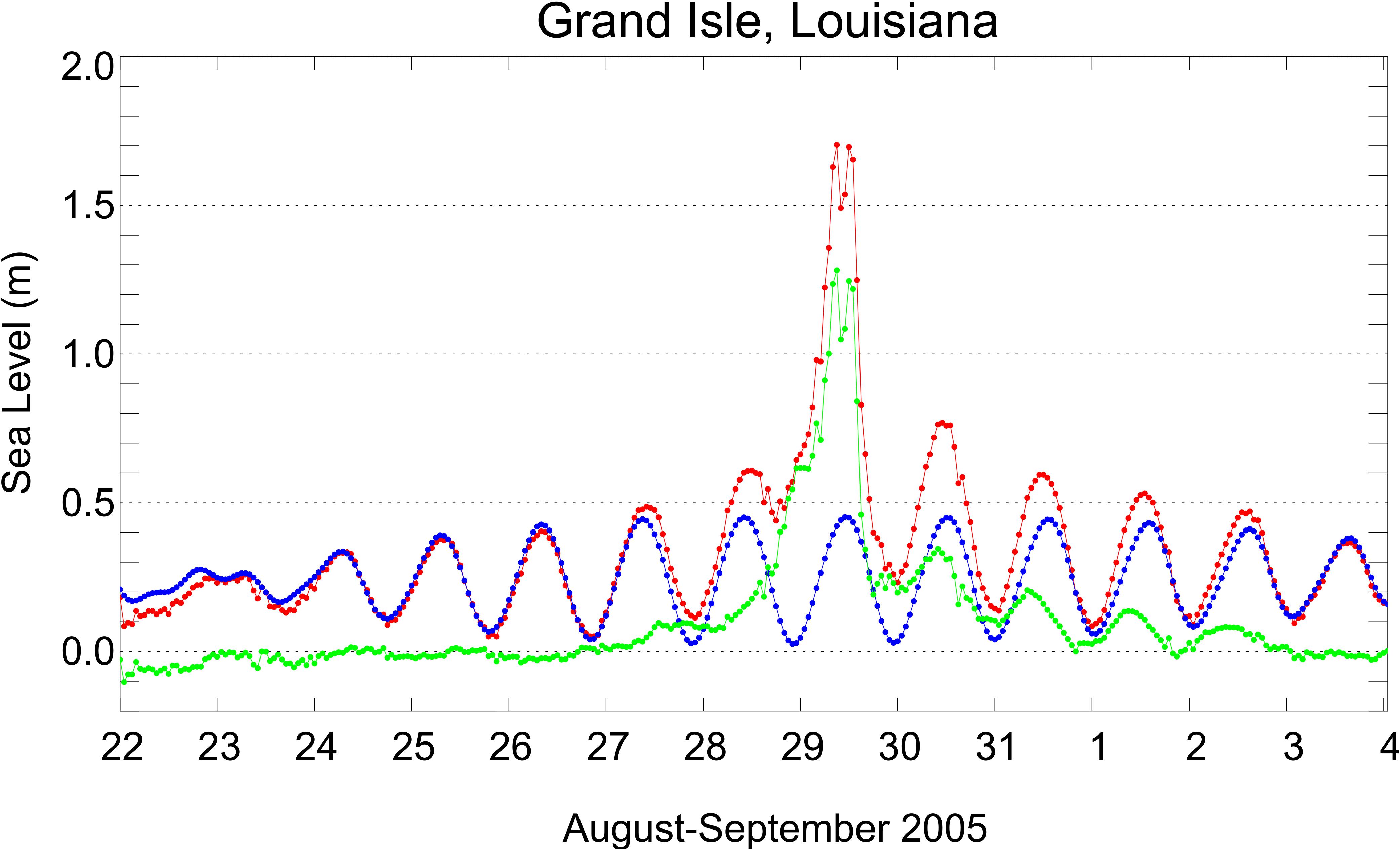
Figure 2. Observed (red), tidal (blue), and meteorological (green) variations of sea level at Grand Isle, Louisiana during Hurricane Katrina, 28–30, August 2005. From Pugh and Woodworth (2014).
A great deal of evidence show that extreme water levels depend on changes in the mean sea level (Menéndez and Woodworth, 2010; Woodworth et al., 2011). This is illustrated in Figure 3 showing that trends in either extremes (99-percentiles) in total water level (Figure 3A), or in skew surges (Figure 3C) can be largely removed if you subtract the mean sea level (not 100%, however) (Figures 3B,D respectively).
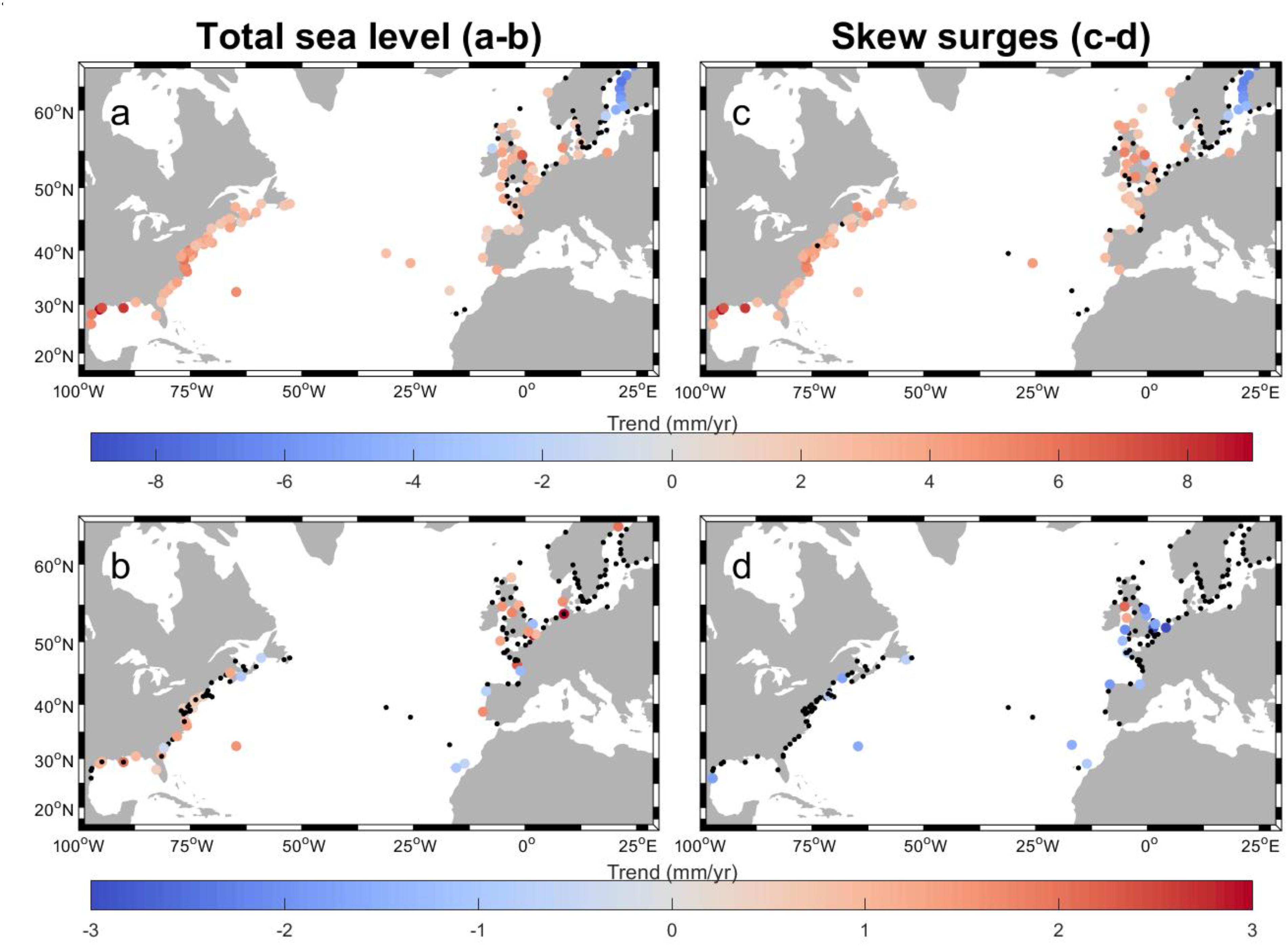
Figure 3. (a) Linear trends of annual 99th percentiles of total sea level and (b) with median removed. (c) Linear trends of annual 99th percentiles of skew surges and (d) with median removed. Tide gauge data from 1960 to present are used. Black dots indicate where the trends are not significant. From Woodworth et al. (2019).
However, changes in extremes also depend on other processes, such as changes in the climatology of storm surges and surface waves. Because of different generation mechanisms, storm surges caused by tropical cyclones are different than those produced by higher-latitude storms. Tropical storm surges tend to be of smaller spatial scale, and shorter duration, but are much larger in amplitude. Valuable sources of information on sea level extreme events come from the data banks of tide gauge records collected by the University of Hawaii Sea Level Center (UHSLC) and the British Oceanographic Data Centre (BODC), for the Global Sea Level Observing System (GLOSS, a program from the Intergovernmental Oceanographic Commission of United Nation Educational, Scientific and Cultural Organization – UNESCO; IOC, 2012). The most complete sea level data set assembled to date, combined from governmental and research sources, is called GESLA-2 (Woodworth et al., 2016).
Vertical Land Motions
At the coast, vertical land motions cause relative sea level change. These have different origins. For example, in active tectonic and volcanic regions, the Earth’s crust suffers important vertical displacements (e.g., Wöppelmann and Marcos, 2016). In other regions, natural causes (e.g., sediment loading in river deltas) or human activities (ground water pumping and oil/gas extraction) may give rise to significant ground subsidence that amplifies climate-related sea level rise (e.g., Raucoules et al., 2013). Post-glacial rebound, the visco-elastic response of the Earth crust to last deglaciation, also called “Glacial Isostatic Adjustment” (GIA), is another process that produces vertical land movements, in particular in high-latitude regions (Peltier, 2004; Tamisiea, 2011).
Faced with the difficulty of modeling all the relevant geophysical processes that cause vertical displacements in coastal zones, both globally and accurately, space-based geodetic techniques are an alternative approach. Available Global Navigation Satellite Systems (GNSS) can indeed help estimating land motions, in particular nearby tide gauges (Wöppelmann et al., 2007). However, presently, less than 14% of GLOSS tide-gauge stations are directly equipped with a permanent GNSS station (see Ponte et al., submitted to this OceanObs’19 FMS Special Issue).
Another important limitation is that even extensive GNSS networks, such as those deployed in Japan or North America, are essentially a collection of point measurements that are sparse compared to the short spatial scales of vertical land motion along many coastlines, where information is needed to determine relative sea level change. Brooks et al. (2007) proposed to combine point wise but accurate geocentric measurements from GNSS with spatially dense but relative (to an arbitrary point on land) measurements from Interferometric Synthetic Aperture Radar (InSAR; e.g., Massonnet and Feigl, 1998). They show that the combined GNSS and InSAR products yield deeper physical understanding and predictive power for beach morphology evolution. Subsequent studies have explored various methods based on InSAR measurements to further assess its applicability in different coastal environments (Raucoules et al., 2013; Wöppelmann et al., 2013; Le Cozannet et al., 2015), confirming its usefulness in sea level studies. InSAR, being more reliable in urban (or arid) areas than in vegetated areas, is particularly efficient for measuring surface displacements in coastal megacities. For mapping long-term deformation with a precision consistent with the sea-level rise rate, the performance of InSAR is related to the number of images in the archive (e.g., about 50 images are required on a multi-year period for reaching ∼1 mm/year precision). However, no project exists at global scale to combine GNSS and InSAR methods.
Vertical land motions at the coast can also be derived from the difference between altimetry-based and tide-gauge-based sea level trends. Although this approach is less accurate than direct ground motion measurements by GNSS, it represents an interesting alternative at tide-gauge sites not equipped with GNSS antennas. This topic is discussed in Section “Coastal Altimetry and Tide Gauges for Solving Vertical Land Motion.”
Waves and Winds in Coastal Zones
The retrieval of waves and winds in the coastal zone is as yet not as mature as sea level measurements. Yet recent studies have shown that trends in wave set-up and swash, which represent the wind-wave contributions to total water level at the coast, can significantly alter sea level changes at the coast over interannual-to-multidecadal timescales (e.g., Melet et al., 2018). Passaro et al. (2015b) showed that altimetry-based near coast estimations of Significant Wave Height (SWH) at 20-Hz frequency based on the ALES retracking (Passaro et al., 2014) are generally well correlated with buoy data. Besides, the spatial variation of the wave field being highly dependent on the local bathymetry, improved coastal bathymetry is also required (see subsection “Digital Elevation Models and Bathymetry”). In addition, wave setup and swash depend on the foreshore beach slope. While sandy beaches are considered as especially vulnerable to sea level rise, no estimates of foreshore beach slopes are available worldwide yet (Luijendijk et al., 2018; Vousdoukas et al., 2018). These slopes vary in space and time, and are required to refine estimates of wave setup and swash.
Although no study exists in coastal areas, several studies have highlighted the interest of using altimeter-derived winds over open ocean for climate studies. Young et al. (2011) have shown that altimeter-derived winds indicate a global increase of sea-surface wind field of roughly 3–4 cm/s/year (from 1991 to 2008) with large regional patterns between 1 cm/s/year and 11 cm/s/year. Similar sea-surface wind speed increase have been detected by Zheng et al. (2016) from Cross-Calibrated, Multi-Platform (CCMP) data, not based on altimetry measurements, but confirming the ability of altimetry measurements to measure the long-term evolution of sea surface winds. Further studies are necessary to better characterize and understand the long-term evolution and uncertainties of altimeter-based sea-surface winds in coastal areas. SAR and scatterometry data would also be useful in the interpretation of the results.
Sediment Transport, River Discharge, and Land Cover Change
Other forcing agents acting in coastal zones include river runoff, sediment supply, and land use change. Over the last decades, human activities have strongly modified river runoff and sediment transport, which greatly influences coastal erosion.
Sediment transport
The monitoring of Suspended Particulate Matter (SPM) in coastal waters is essential to understand the evolution of coastal zones (Ouillon et al., 2004; Warrick et al., 2004; Loisel et al., 2014). Solid water discharge from rivers, terrestrial matter from shore erosion, and re-suspension of bottom sediments occurring in shallow waters under local wind-waves and swell action, represent the main sources of suspended particles in coastal waters. Organic particles from terrestrial origin, or produced locally by biogeochemical processes also contribute to SPM. SPM variability is tightly linked to coastal erosion and accretion processes and then has a direct impact on the coastline evolution. Forecasting SPM dynamics in response to natural or anthropogenic forcing is crucial to better adapt to present and future coastal changes. Such an objective can only be achieved by combining modeling and observation efforts. Ocean-color satellite observations can provide information on the SPM variability of surface waters at relevant spatio-temporal scales. These data, which can be collected over long time periods, represent a valuable source of information to better constrain sediment transport models. While past ocean-color sensors were able to collect observations at the spatial resolution of 1 km × 1 km, the new ocean-color sensors generation, such as OLCI on Sentinel-3, allows sampling at a much better spatial resolution (i.e., 300 m × 300 m). Also, high spatial resolution sensors (10–60 m) such as OLI on Landsat-8 or MSI on Sentinel-2, both originally developed for land observation, have a sufficient radiometric resolution to be used for SPM assessment. Because current remote sensing observations only provide information about the surface layer, the assessment of the total load of sediment integrated on the whole water column requires the development of synergic approaches combining passive and active (i.e., LiDAR) remote sensing, and modeling.
River runoff
Accurate estimates of river runoff and sediment delivery to the coastal zone are also crucial. River Water Discharge (RWD) is one of the major processes that affect environmental conditions (such as currents and hydrography) in coastal waters. It reflects the drainage basin area dynamics and is a function of parameters such as geology, relief, precipitation, vegetation, climate and human influences. The RWD provides fresh waters that affect mixing and circulation processes in estuaries, thus modifying geomorphological and geochemical properties of the coast (Milliman and Farnsworth, 2013). Besides, building of reservoirs on rivers decrease water and sediment fluxes to the coast (Syvitski et al., 2005). Another important consequence of RWD control is the high flow amplitudes reduction, which decreases the carrying capacity for SPM, and modifies the seasonal estuarine circulation patterns and salinity distributions. All of these changes can greatly influence coastal erosion, the benthic environments, coral reefs, sea-grass communities, and coastal fisheries. Quantifying accurately fluvial delivery to the coastal zone is therefore crucial. Long-term high-resolution RWD and SPM measurements – in both time and space – are needed to assess the impact of global change on coastal zones.
Satellite altimetry now routinely measures water level on land from which river discharge can be derived, in particular over ungauged or poorly gauged hydrological basins (Crétaux et al., 2018). River discharge for medium size basins (<10,000 km2) is also indirectly estimated from satellite images in the visible and near infrared spectrum (e.g., MODerate-resolution Imaging Spectroradiometer – MODIS) (Tarpanelli et al., 2015). The Surface Water and Ocean Topography (SWOT) satellite mission planned for launch in 2021 will improve the characterization of global runoff processes with a ∼50 m resolution threshold.
Coastal Responses and Observational Needs
Depending on the time-scale of processes acting in coastal zones (from extreme events to long-term sea level rise), different types of observations are required to monitor and understand the response of coastal zones to the forcing agents and associated shoreline changes.
Shoreline Changes
Scientists and coastal managers concerned with shoreline changes focus on three different types of processes: (1) extreme events such as major storms or cyclones, (2) seasonal to decadal coastline variability, and (3) decadal to multidecadal shoreline changes.
Depending on the process of interest, different types of data and observational strategies are needed. For extreme events, the priority is given to comparing pre- and post-crisis coastal morphologies. In this case, both a background observational framework and a capacity to monitor the coastal sites just after the events are needed. Such capabilities are presently more or less available from both the space and in situ components of coastal observatories (international charter for disaster; post-crisis special missions organized by coastal communities).
Monitoring seasonal to longer-term changes requires high-resolution observations in space and time. However, the actual specifications depend on the coastal site of interest. For example, multi-decadal surveys in highly dynamic deltas can benefit from medium resolution data (e.g., Landsat data), which can be analyzed using a semi-automatic procedure (Luijendijk et al., 2018). However, most shorelines around the world are currently evolving at rates in the order of ±1 m/year or less (Bird, 1985). With pioneering global coastal evolution datasets being made available today (Luijendijk et al., 2018), meeting local user needs in terms of precision and accuracy (below 1 m/year) has become a next challenge for Earth Observations (EO).
Digital Elevation Models and Bathymetry
High resolution Digital Elevation Models (DEMs) and coastal bathymetric data are critical datasets for a number of applications in coastal zones, including accurate modeling of flooding during storm surges, and quantification of coastal morphological changes due to sedimentary processes or human interventions. High-resolution bathymetric and topographic LiDAR techniques have allowed important progresses over the last decade, but still require post-processing to remove trees and cars from the raw data. However, the lack of repetitiveness in current LiDAR acquisitions is presently limiting the applications, especially in highly dynamic coastal zones such as beaches, marshes, and estuaries. Further acquisition of high-resolution topography, near-shore bathymetry and foreshore beach slopes data is an observational priority, especially along densely populated coastlines. A second priority is the development of methods to cover the need for repeated acquisitions in highly dynamic areas. While this seems technically feasible, further research is needed to meet the users’ requirements in terms of temporal resolution, especially in near-shore areas.
Summary
To better understand how coastal zones react to various perturbing factors and change through time, a number of observing systems, either space-based, airborne or in situ should be implemented and combined. We summarize below a (non-exhaustive) series of observational needs related to the topics addressed in this section:
- Ensure that the global and regional monitoring networks of extreme sea levels are completed to their full technical requirements.
- Ensure that a global sea level database such as GESLA-2 is maintained and extended in the future.
- Ensure that extreme water levels observations are acquired in areas unaffected by the wave setup.
- Increase the number of GNSS stations co-located with tide gauges for monitoring vertical land motions (Woodworth et al., 2017).
- Develop precise positioning capabilities in coastal areas combining GNSS and InSAR approaches to monitor local to regional land motions.
- Altimetry can provide wave height, wind speed and sea level at the same time. However, systematic monitoring of the fine-scale variations of these parameters at the coast is still lacking. The high-resolution wave field in the coastal band is also relevant, as it helps developing more realistic wave models that can be used to estimate wave setup. R&D investigations using archived and coming altimeter data sets (in particular with the new SAR mode) need to be conducted. Moreover, multi-sensor approach for wind and waves in the coastal zone using altimetry, SAR and scatterometry where ground-truth is available would be highly useful.
- Coherent and homogenized SPM time series over the global coastal region should be collected from past and present space-based ocean-color data. This task requires inter-calibration between the different sensors, as well as the development of common atmospheric and bio-optical algorithms. Uncertainty maps should also be provided. Fusion of high-spatial-low-spectral and medium-spatial-high-spectral resolutions sensors should be undertaken to better sample the coastal environment at the relevant scales.
- Long-term high-resolution observations of river discharge, ground water discharge, SPM in global coastal zones are highly needed.
- The full potential of remote sensing for monitoring shoreline changes has not been fully investigated yet. Use of SAR data to monitor shorelines changes in tropical areas has been explored but the resolution of this data set (about 30 m for ASAR on-board Envisat) remains too limited. More recent high-resolution sensors (e.g., Cosmo-Skymed) and improvements in orbital parameters control (e.g., TerraSAR-X) offer useful alternative. Research to automatize the precise georectification of images (beyond what is classically provided by current workflows) and, ultimately, to retrieve shoreline proxies automatically would be much beneficial to the community.
- A high spatio-temporal resolution continuous marine-land topography and bathymetry database, with height precision better than 20 cm is needed. However, lower resolution datasets such as those that can be produced with Sentinel 2 or other data may still be useful for understanding coastal evolution (e.g., Poupardin et al., 2016).
Concerning that latter issue, present-day information on the evolution of coastal zones is currently acquired at local to regional scales by coastal observatories, which act as a means of transferring information between science, operational observations (including space-based), and coastal stakeholders (Suanez et al., 2012). Presently, the existing information at national, multinational, and global scales originates from these entities (e.g., Eurosion, 2004). However, different coastal observatories are far from having the same standards (resource datasets, including from satellite observations, workflows, procedures, and objectives), so that the information that currently exists is often extremely heterogeneous. Networks of coastal observatories are being established in different countries to resolve this issue.
Coastal Zone Altimetry Processing and Exploitation: Progress and Outlook for the Next Decade
Context
Coastal Altimetry is the extension of open ocean altimetry into the oceanic coastal zone. It has had a steady progress in recent years and has become a recognized mission target for present and future satellite altimeters (Vignudelli et al., 2011; Cipollini et al., 2017). Compared to conventional Ku band, Low Resolution Model (LRM) altimetry missions launched since the early 1990s (ERS, Topex/Poseidon and Jason series, Envisat), the SARAL/AltiKa mission, operating in Ka band, CryoSat-2 operating in Ku band but in Synthetic Aperture Radar (SAR) [also called Delay-Doppler Altimeter (DDA)] mode and in the interferometric mode (SARin) and Sentinel-3A/B operating in Ku and C bands in SAR mode, have proved their ability to obtain more precise and accurate measurements of sea surface heights. Because of their reduced footprint, measurement geometry and dedicated algorithms for both processing at level 1 and level 2, data can be acquired closer to the coastline with improving performances. Indeed, the most important achievement of Coastal Altimetry research in the last 10 years has been the reduction of the data gap near the coast, which is today less than 5 km (Cipollini et al., 2017).
Coastal altimetry has some complications beyond open ocean altimetry work. Two issues that have already been extensively studied are land interference and inhomogeneity of radar backscatter. As a consequence of the previous factors the waveform is distorted. Re-tracking algorithms applied to the radar echoes need to recognize and mitigate these distortions.
Dedicated corrections to correct altimeter ranges in the coastal zone are also crucial. All corrections in standard altimetry were optimized for the open ocean. They do not account for specificities of the coastal zone [bathymetry for tidal and atmospheric loading models; land interference for radiometer used to estimate water vapor; sea state variability for the sea state bias (SSB) correction].
Retracking Conventional and SAR Mode Altimetry
So far, quality and quantity of altimeter data retrieved in the coastal zone have improved thanks to new specific processing applied to conventional altimetry and use of the new DDA mode, and fitting processing. For conventional altimetry, new sub-waveform partitioning and fitting have been designed to tackle waveform inhomogeneities in the satellite footprint typical of coastal waters, due to presence of land or patches of calm water. This novel approach restricts the fitting to a portion of the reflected signal (Deng and Featherstone, 2006; Yang et al., 2012; Passaro et al., 2014, 2018; Dinardo et al., 2017; Roscher et al., 2017; Peng and Deng, 2018). A successful way of selecting this portion is based on an adaptive algorithm, which depends on the sea state (as in Passaro et al., 2014), which strongly influences the noise performances of an altimeter, leading to a significant improvement in terms of quality and quantity of the data retrievals, while improving the description of the middle scales of variability (10–80 km) in the open ocean as well (Smith et al., 2017).
A first example is provided in Passaro et al. (2015a) for the sea level estimated from the Adaptive Leading Edge Subwaveform (ALES) retracker, evaluated in coastal areas against open ocean products from the European Space Agency Sea Level Climate Change Initiative (ESA SL_cci) and Radar Altimetry Database (RADS), not tailored for coastal exploitation. The standard deviations and root mean square differences of the sea surface height anomalies (SSHa) from ALES are smaller than for the SL_cci and the RADS products (see Figure 4). A second example is provided in Roscher et al. (2017) by the Spatio-Temporal Altimetry Retracking (STAR) retracker, where sub-waveform analysis was modified through sparse representation and conditional random field (CRF) modeling. The latter compares well to the ALES sub-waveform method, with similar SSHa quality in open ocean and coastal zone (see Figure 5).
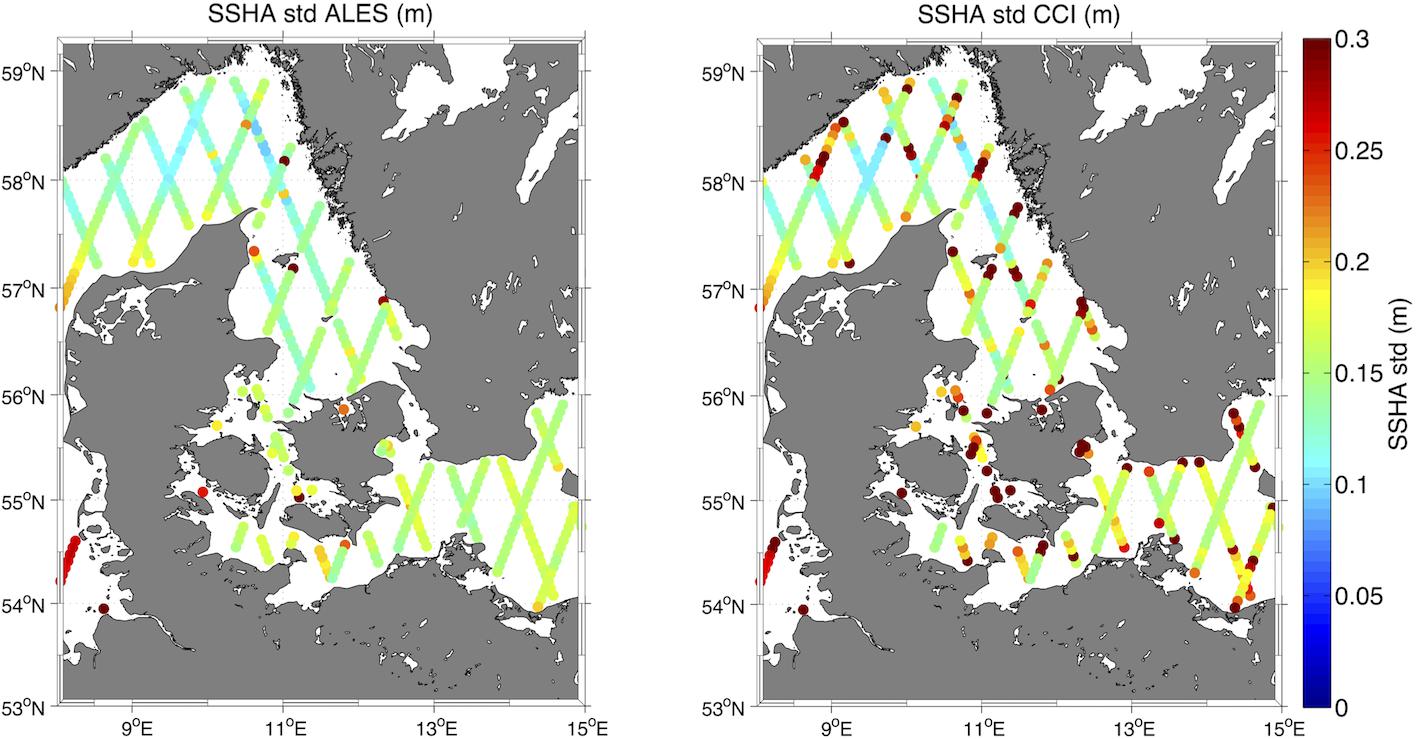
Figure 4. Comparison between (Left) ALES reprocessed and (Right) Envisat SL_cci data sets in terms of standard deviation of the Sea Surface Height Anomaly time series for each 1 Hz location (adapted from Passaro et al., 2015a, with permission).
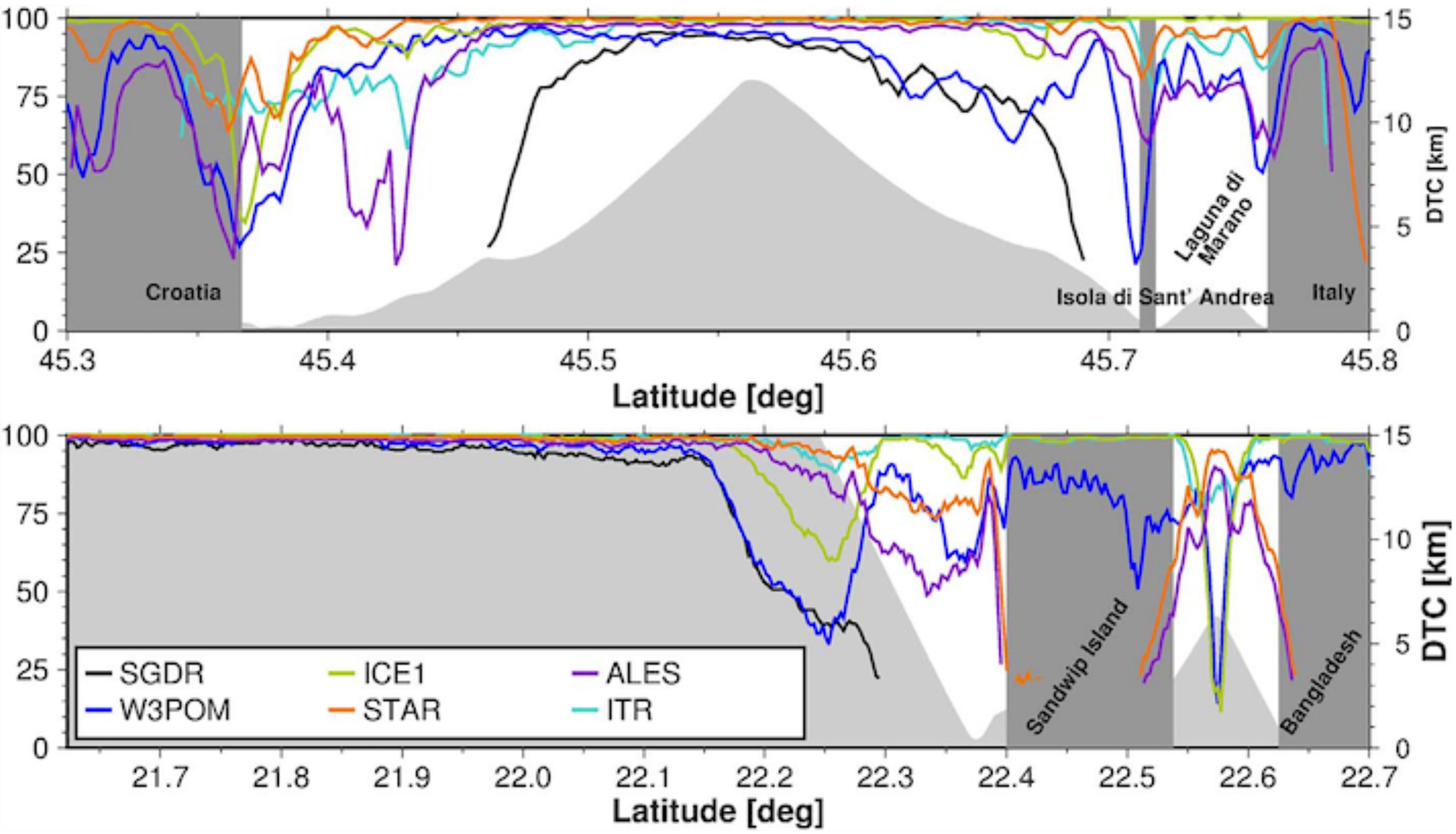
Figure 5. Percentage of the total number of available Jason-2 cycles (227) after applying outlier detection and minimum number of cycles requirement. (Top) Study site at the Gulf of Trieste. (Bottom) Study site at the coast of Bangladesh. The distance to the nearest coastline is provided in light gray. The colored lines correspond to the different retrackers mentioned by their acronym in the insert (adapted from Roscher et al., 2017, with permission).
A step forward has been made with the DDA technology on CryoSat-2 since 2010 and Sentinel-3 since 2016. Its novelty consists in exploiting the different Doppler shift experienced by different rows of the altimeter antenna beam, depending on the along-track angle with respect to nadir. It provides an improved along-track resolution of ∼300 m, while the across-track resolution is the same as in conventional altimetry. The coverage is improved in coastal region where conventional altimeters echoes are impacted by land contamination in both directions. Moreover, DDA has an enhanced signal-to-noise ratio to retrieve fine-scale oceanographic features. In open ocean both accuracy and precision are higher in DDA than in Pseudo LRM (PLRM), or Reduced SAR (RDSAR) (Fenoglio-Marc et al., 2015a; Buchhaupt et al., 2017). PLRM/RDSAR are derived from the Full Bit Rate (FBR) data by processing the pulse-limited echoes incoherently, like in the conventional LRM concept (Smith and Scharroo, 2015).
As for conventional and DDA altimetry, dedicated processing has been developed to improve the data in the coastal zone. This includes both fully focused SAR (Egido and Smith, 2017) and enhanced unfocused SAR processing. The standard unfocused SAR processing baseline consists of ground cell gridding at 20 Hz, Doppler Beam Forming, Doppler Beam Stacking, Slant Range Compensation, Range Compression and Multilooking (Raney, 1998; Cullen and Wingham, 2002) and of the open ocean SAMOSA SAR waveform model (Ray et al., 2015) in its formulation with zero- and first-order term (SAMOSA2). For coastal zone, Dinardo et al. (2017) extend the processing baseline SAMOSA2 to SAMOSA+, enhancing the processing with a set of options specifically tailored for the coastal domain both at the waveform generation level and in the retracking step. In coastal zone DDA is less noisy than PLRM/RDSAR and more data are retained by the outlier rejection as shown in Figure 6. Similarly, the standard deviation of SSHa, which increases near coast, is the smallest for DDA data processed with SAMOSA+ and within the expected range until 2–3 km from the coast. Standard deviation of differences and absolute biases between the SSHa and tide-gauge data are on the order of few centimeters (Bonnefond et al., 2018a; Fenoglio et al., 2019). Best results come from CryoSat-2 and Sentinel-3A SAR altimetry processed with SAMOSA+ at the Grid Processing On Demand (GPOD) service at ESRIN1. In situ and model validation of SWH and wind speed SAR show improvement with the new SAR data (Staneva et al., 2016; Wiese et al., 2018).
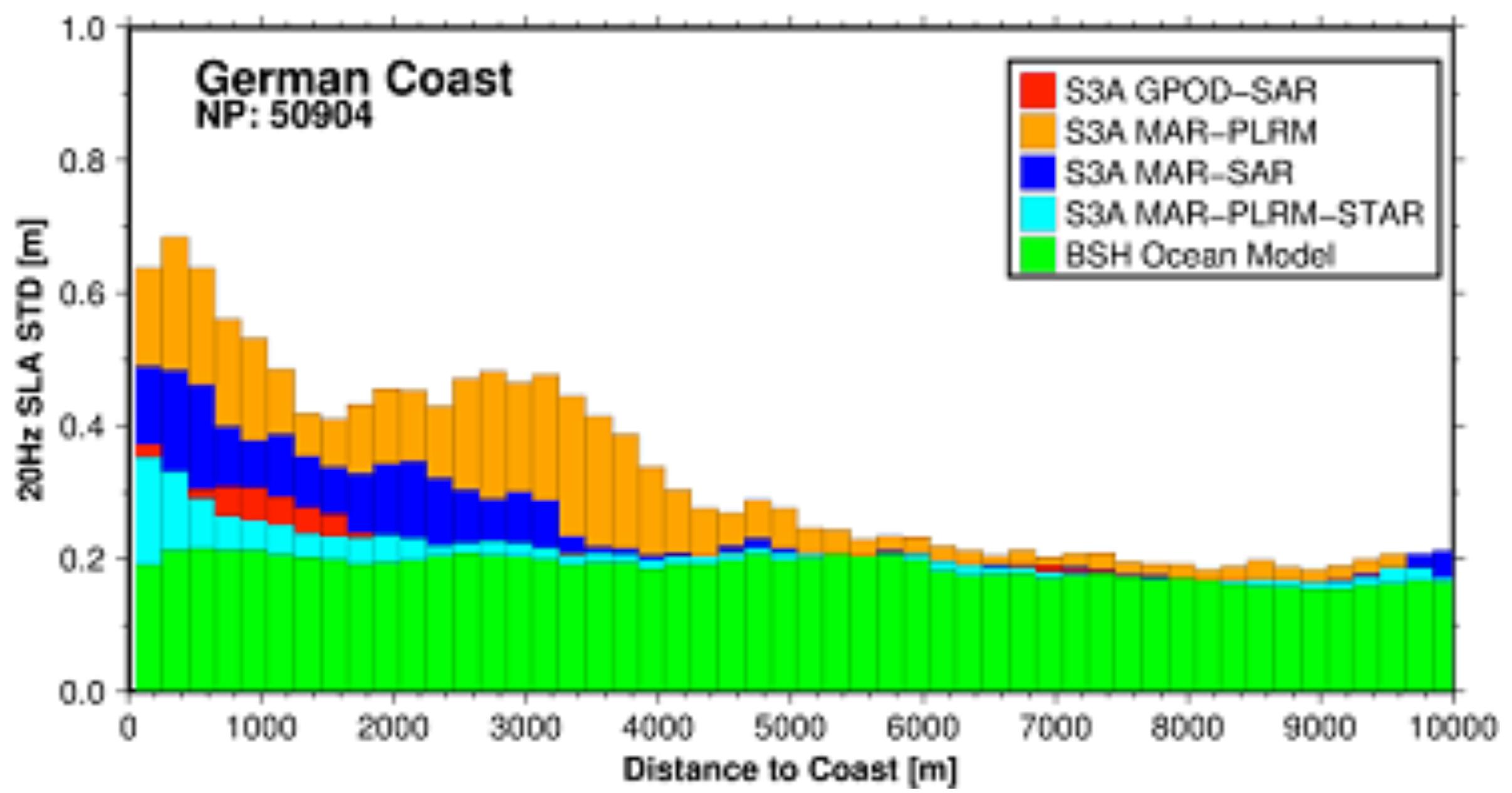
Figure 6. Sea level anomaly at 20 Hz standard deviation in 200 m bins of distance to coast for different Sentinel-3A processors and the BSH model along the German coast. The Sentinel-3A SAR altimetry processed with SAMOSA+ at the Grid Processing On Demand (GPOD) service at ESRIN exhibit the smallest standard deviation (adapted from Fenoglio et al., 2019, with permission).
SARin Mode and Fully Focused SAR Altimetry
The fully focused SAR (FF-SAR) processing technique promises an along-track resolution of 0.5 m for typical SAR altimeters (Egido and Smith, 2017), instead of the 300-m resolution of the unfocused DDA processing. Also relevant for coastal zone, but scarcely investigated in this area is the DDA interferometry (SARin), which permits to extract spatial and temporal derivatives of water levels, providing an entirely new observable area. Few studies in coastal zone so far use SARin CryoSat-2 data (see Bouffard et al., 2018 and Parrinello et al., 2018 for a recent review). Idžanović et al. (2017) selected Norwegian tide gauges close to CryoSat-2 tracks with data collected in SARin mode (Figure 7) and found that SARin data processed as SAR has higher correlation and better RMS with in situ on the rugged coast of Norway compared with conventional altimetry. Abulaitijiang et al. (2015) fully exploited the SARin interferometry and showed that data from 0 to 7 km inland can be re-allocated to the coast using the Off Nadir Range correction (ONR) (Figure 8). Data further in-land from 7 to 14 km can also be re-allocated to the coast by ONR and Phase Ambiguity correction (PA).
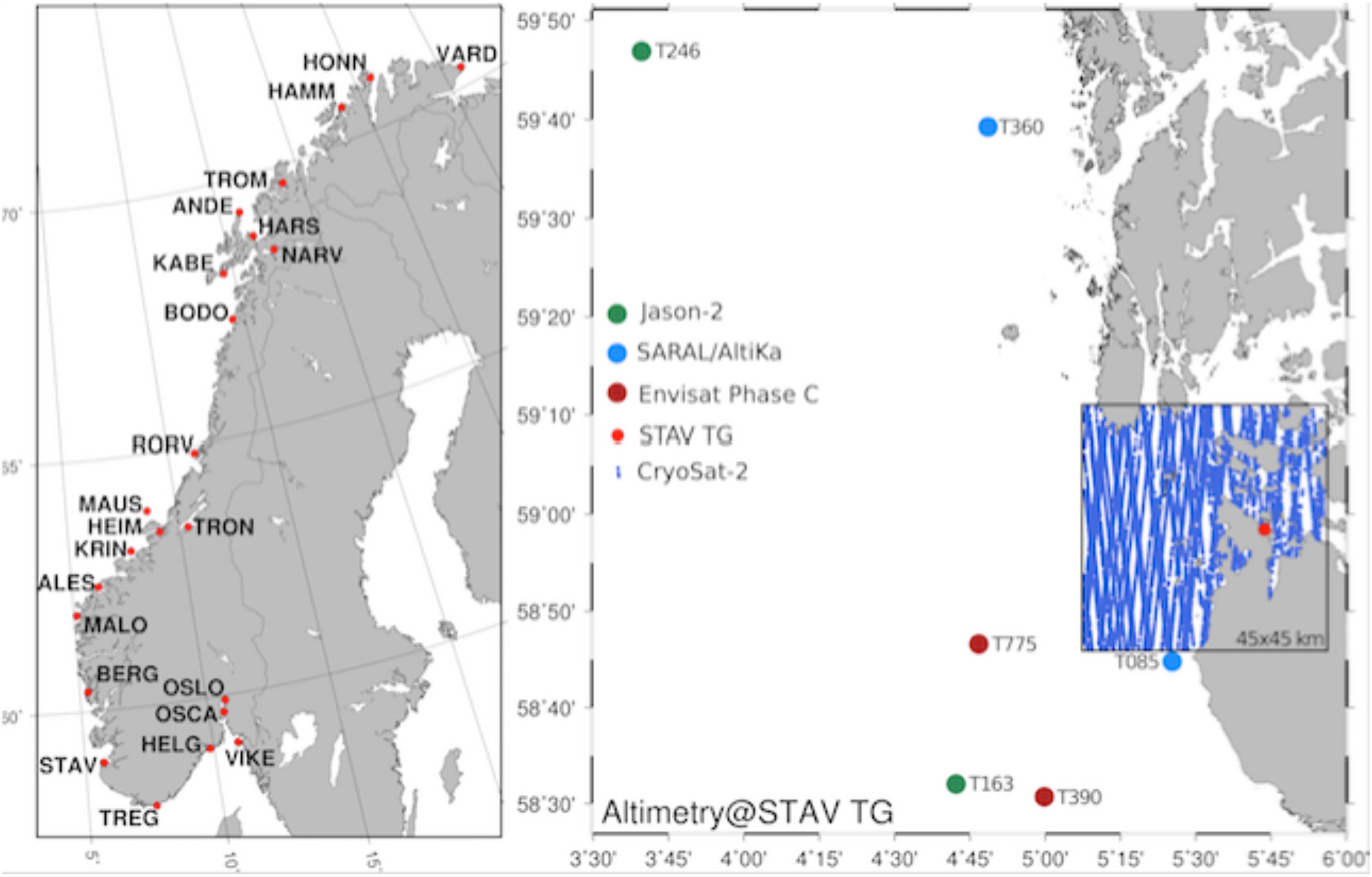
Figure 7. The Norwegian tide gauges (Left) used in the study and example of CryoSat-2 tracks with data collected in SARin mode and near STAV station (Right). Also shown are the closest Envisat, Jason-2 and SARAL/Altika ground points (adapted from Idžanović et al., 2017).
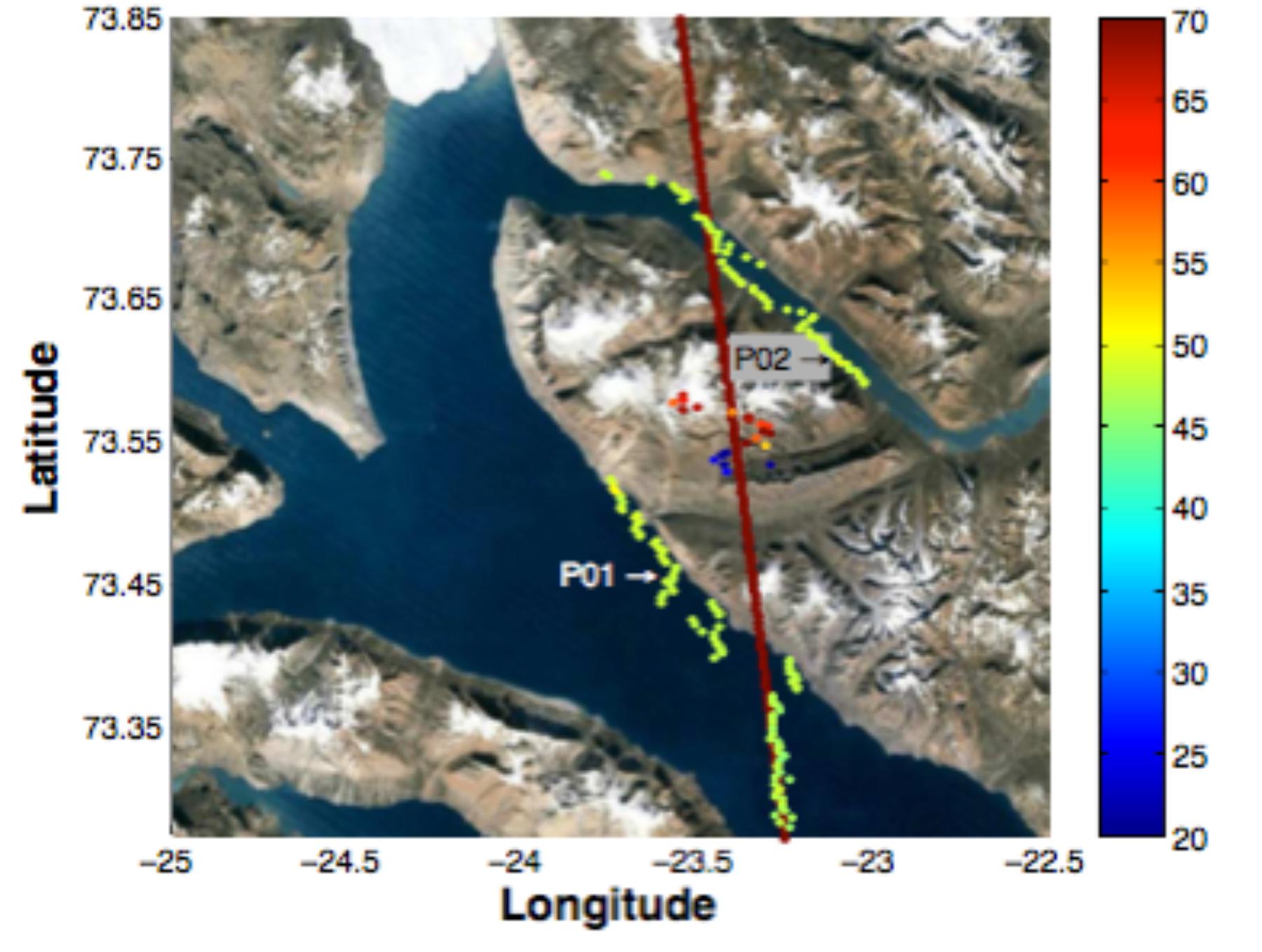
Figure 8. Ascending track crossing the Fjords of Eastern Greenland. Dark red track correspond to the satellite nadir. Scattered points are relocated positions after the ONR corrections are applied. Two points are marked by P01 and P02 in the figure. The elevations are referenced to WGS84 ellipsoid. Unit: meters (adapted from Abulaitijiang et al., 2015).
Geophysical Corrections
Together with accurate orbits and satellite-to-surface altimeter range retrievals, geophysical corrections, associated to coastal-oriented editing strategies (Vignudelli et al., 2005), are another crucial component in accurately deriving SSHa measurement at the coast. Much progress has been achieved in the last decade in retrieving accurate corrections over coastal and inland water regions, in particular the dry tropospheric correction (DTC) (Fernandes et al., 2013, 2014), the wet tropospheric correction (WTC) (Desportes et al., 2007; Brown, 2010; Fernandes et al., 2010, 2015; Fernandes and Lázaro, 2016, 2018) and ocean tides (Carrère et al., 2014). Regarding the WTC, increasing accuracy is obtained with the ERA5 atmospheric reanalysis from ECMWF, now provided at 1-h time sampling and also from the high frequency channels included in the microwave radiometers to be flown on Sentinel-6 and SWOT missions.
Regarding ocean tides, there is a need to increase the accuracy of tide models in the coastal zone, where they are known to be much less accurate than in the open ocean essentially because of the not-well-known bathymetry, which has a stronger impact in the shallow waters. In an iterative process it is crucial to improve the knowledge of the bathymetry and of the tide models with finer grids while approaching the coast. The ocean tide correction of the altimeter SSHa remain the largest error source of the whole altimetry end-to-end system in the coastal zone (Ray and Egbert, 2017; Toublanc et al., 2018).
Level 3 Altimetry Datasets
The development of dedicated altimetry datasets at level 2 (COASTALT, PEACHI) and level 3 (X-TRACK, ALES/COSTA, DUACS-HR) has been advancing toward the high along-track resolution and/or special processing to reduce noise and provide data near coastlines or in shallow shelf waters, offering an opportunity of exploitation for scientific applications. A table is published in Cipollini et al. (2017) and a regularly updated version is available at http://www.coastalt.eu/#datasets. Several studies compared these data sets with independent measurements (e.g., Gómez-Enri et al., 2016; Vu et al., 2018; Xu et al., 2018). Quality assessments for Sentinel-3A and CryoSat-2 (Bonnefond et al., 2018a; Fenoglio et al., 2019) and AltiKa data (Bonnefond et al., 2018b) have also been performed.
Storm surges are the major cause for coastal flooding, which can result in catastrophic damage to properties and loss of life in coastal communities. For example, over 1,800 people lost their lives during Hurricane Katrina, 2005 and many of those deaths were directly or indirectly caused by storm surge. Many coastal regions in the world are vulnerable to storm surges, with approximately 45% of the world’s population living within 150 km of the coast. Thus it is important to utilize satellite altimetry to enhance our capabilities of observing storm surges to complement traditional tide-gauge networks as demonstrated in Madsen et al. (2015).
These data sets have been shown to be useful to monitor coastal storm surges (Han et al., 2012, 2017; Lillibridge et al., 2013; Antony et al., 2014; Chen et al., 2014; Fenoglio-Marc et al., 2015b) and to improve their forecasting (Madsen et al., 2015; De Biasio et al., 2016, 2017; Bajo et al., 2017; Li et al., 2018). While it is opportunistic for a single altimeter to capture a storm surge, a constellation of altimeter missions especially with wide-swath altimetry could significantly enhance the chance (Antony et al., 2014; Turki et al., 2015; Han, 2017; Han et al., 2017).
Study of coastal sea level variability and change is another application. Use of coastal altimetry dataset have revealed higher annual amplitudes in sea level in the presence of narrow coastal currents (Passaro et al., 2015a, 2016) improving the determination of coastal tides (Piccioni et al., 2018). The coastal altimetry data also helped in improving knowledge about spatio-temporal changes of the coastal ocean circulation (e.g., in the Mediterranean Sea: Birol and Delebecque, 2014; Jebri et al., 2016; in the eastern shelf of the Gulf of Tehuantepec: Salazar-Ceciliano et al., 2018). Another recent study by Dong et al. (2018) showed that both 20-Hz and 1-Hz along-track sea SSHa data can be used to extract coastal tidal mixing front signals. The value of improved altimeter data in detecting coastal SSHa sea level anomalies due to river discharge has been demonstrated by Gómez-Enri et al. (2017) and appears promising to study linkages between the land–sea domains (Piecuch et al., 2018). Another example of application concerns the characterization of mesoscale coastal dynamics in the southeastern Bay of Biscay (Spain) in combination with other remote sensing platforms and High-Frequency Radar observations (Rubio et al., 2018).
Coastal Altimetry and Tide Gauges for Solving Vertical Land Motion
As discussed in Section “Monitoring Coastal Zones With Multiple Observing Systems,” knowledge of vertical land motions at the coast is important for several purposes. While GNSS techniques are the most direct approach to measure VLMs, differences between altimetry and tide gauge sea-level trends provide complementary information. Using monthly, 1-Hz sea level data from LRM altimetry missions and tide-gauge records, studies have shown that VLMs could be retrieved to 0.8 mm/year median accuracy (Wöppelmann and Marcos, 2016). Use of retracked coastal altimetry and DDA significantly improves the VLM estimates (Fenoglio et al., 2019).
Outlook and Recommendations
Great progress has been made during the past decade to improve SSHa measurements in the world’s coastal zones for a variety of oceanographic and climate applications. These data have also been useful for high-resolution ocean modeling, either in comparison or assimilation modes. Models help “fill the sampling gap” with model-based dynamical downscaling. The challenge is the interpretation (and exploitation) of the extra resolution and the understanding of the signature due to the oceanographic content. Additionally, high-resolution Mean Dynamic Topography is essential in a regional data assimilative ocean prediction system to capture the subtleties of coastal mean circulation [e.g., Levin et al. (2018) produced their own local for the Middle Atlantic Bay].
The small scales characterizing the variability of the coastal ocean are driving the interest toward the use of SSHa at high rate (typically 20-Hz instead of 1-Hz). Coverage and sampling are being improved today by DDA and constellation of altimeters, but limitations in resolution and space–time sampling do not allow a systematic mapping. A wide-swath altimetry, which uses radar interferometry at near-nadir incidence angle (Rodriguez et al., 2018), needs to be used instead. Nadir altimetry will be part of the analysis, which goes from profile to surface observations. A further challenge is the improvement in DDA mode in SAR and SARin and the integration of past conventional altimetry, present DDA and future (swath-) altimetry observations to construct an extended and more complete sea level change record (Ablain et al., 2016; Legeais et al., 2018).
The main future goals of coastal altimetry are:
(1) Continuing improvement of waveform modeling and retracking, as well as of the geophysical corrections and of the mean sea surface in coastal regions.
(2) Assessing the performance of coastal altimetry with tide gauges measurements.
(3) Developing global multi-mission along-track and gridded sea level products including data in coastal zone to be used in regional and local studies.
(4) Contributing to coastal observing systems (for dynamics/level/sea state/extreme events) through data assimilation in models.
(5) Exploiting long time series of coastal altimetry data for coastal sea level and sea state climate.
(6) Contributing to the preparation of the SWOT mission.
Recommendations
For a number of scientific and societal applications, what is urgently needed is a global-scale coastal sea level data base that provides long-term (at least 2 decades-long) sea level records within 10–20 km to the coast, with as high as possible resolution (<1 km), based on retracked LRM altimetry and use of SAR technology, together with improved geophysical corrections adapted to coastal regions. Both along-track and gridded products (the latter derived from combining different missions) have to be provided. Ideally, what would be useful to end-users is a seamless multi-mission gridded sea level record (with associated uncertainty) from the open ocean to the coast, with global coverage and varying spatial resolution (25 km offshore, <1 km when approaching the coast). The user community also requests regular updates of this product.
Wideband Signals of Opportunity Reflectometry for Coastal Ocean Applications
Context
Since the early 1990s, development of high precision altimetry has witnessed great advances in our view of the oceanic circulation. One fundamental advance reveals that the oceanic circulation expands across a wide spectrum of temporal and spatial scales, from the basin scale of thousands of kilometers, mesoscale eddies of tens to hundreds of kilometers, down to sub-mesoscales of a few kilometers. All these scales are dynamic and energetic and play significant roles in ocean dynamics, climate variability, and biological and chemical processes, and are important to maritime operations. However, today’s observations can resolve the circulations only down to large mesoscale scale eddies and temporal scales down to several days (e.g., Chelton et al., 2007).
To resolve the ocean circulation at spatial and temporal scales beyond what today’s altimeters can resolve, new altimetry technologies are required. As discussed in section “Coastal Zone Altimetry Processing and Exploitation: Progress and Outlook for the Next Decade,” DDA mode on new-generation of nadir altimeters significantly enhances the spatial resolution. Moreover, the SWOT mission, to be launched in 2021, will measure SSHa with a spatial resolution of order of 1 km, with a temporal revisit time driven by the 21-day repeat cycle and swath width (SWOT’s 120-km-wide swath will result in overlapping measurements over most of the globe with an average revisit time of 11 days). Temporal repeat time can only be improved through launching more altimeters in appropriately phased orbits, a high-cost option with the current radar altimeter technology.
Here, an alternative approach is discussed, namely the Ocean Surface Topography Using Wideband Signals of Opportunity (OST W-SoOp), which draws its heritage from altimetry concepts using the Global Navigation Satellite System Reflectometry (GNSS-R) technology. This technique assumes that the ocean-scattered signal is composed of multiple ray-paths with a statistical distribution in delays. Cross-correlation between direct and reflected signal can be applied to estimate the fraction of scattered power within each arriving delay and Doppler bin, which map to areas on the scattering surface. The basic observable generated in reflectometry is the distribution of scattered power as a function of both delay and Doppler called the delay-Doppler map (DDM) (Zavorotny and Voronovich, 2000; Garrison et al., 2002). SSHa retrieval are derived by retracking the DDM.
Overview of Wideband Signals of Opportunity Reflectometry
Background: GNSS-R
The origin of SoOp altimetry can be traced back to the PARIS (Passive Reflectometry and Interferometry System) concept proposed by Martín-Neira (1993), in which GNSS transmitters were proposed as the signal source. The essential problem of the low bandwidth of the GNSS signals was identified with suggestion that opportunistic signals of “a few hundred MHz” could give scientifically useful cm-level accuracy in SSHa measurements. Key error sources were identified and a preliminary link budget performed for a design requiring a 4 m × 4 m antenna with a gain of 37 dB.
In the following decades, there was an increase in the promise of GNSS altimetry in providing an enhanced spatial and temporal sampling with a constellation of small, low-cost satellites employing passive receivers. The key difficulties of GNSS signal sources, however, have also been identified, namely (1) low bandwidth, (2) low transmitted power, (3) large ionospheric delay at L-band, and (4) large physical antenna dimensions to meet gain requirements. Subsequent studies and mission proposals have further quantified the effects of these limitations and designed systems incorporating necessary compromises to meet science requirements in light of these limitations.
Hajj and Zuffada (2003) considered coherence time, the spatial density of reflection points, and the effect of ionosphere and neutral atmosphere to evaluate space-borne GNSS altimetry. A rough error analysis predicted a 1-m error using a 23 dB antenna and 4 s of averaging. Spatial averaging over 100 km and temporal averaging over 4 days reduced first-order height error to a few centimeters.
A more comprehensive study and model development was conducted for the PARIS In-Orbit Demonstrator (PARIS-IOD) proposal (Martín-Neira et al., 2011). This was specified as a single-satellite demonstration of the GNSS altimetry concept, to validate hardware operations and error models, as a step toward an operational follow-on mission. With an emphasis on synoptic coverage of the ocean, this operational mission would improve revisit time by approximately a factor of 20 over a traditional nadir altimeter, with science requirements of 5 cm height error and 100 km along-track resolution. That study evaluated issues with the ionospheric delay error and the low bandwidth of the open GNSS signals on both frequencies. Combined, multi-frequency observations were required to remove a significant part of the ionospheric delay. Interferometric methods (iGNSS-R), in which the direct signal is used as a reference for cross-correlation with the reflected signal to utilize full signal bandwidth, were determined to be necessary to meet the science requirements. The PARIS-IOD design requires a pair of 23 dBi electronically steered antennas and the error budget was found to be 17 cm (including instrument, speckle, and ionosphere) from 800 km orbit but with along-track averaging of 100 km, which would have a significant impact on the spatial resolution. Ionospheric delay, proportional to 1/f2, remained a significant error, due to the comparably lower frequency of L-band.
GEROS-ISS (Wickert et al., 2016) was a more recent mission proposal for a technology demonstration on the International Space Station (ISS). With the exception of some complications for implementing precision orbit determination (POD) on a large, maneuvering platform in Low Earth Orbits (LEO), the design approach for GEROS-ISS follows that of PARIS-IOD with a high-gain steerable antenna. Speckle was predicted to account for less than 15 cm error in 100 km of averaging. Similarly, the GNSS Transpolar Earth Reflectometry exploriNg (G-TERN) mission was suggested for GNSS altimetry from polar orbit (ESA Earth Explorer 9, Revised Call). The altimetric performance expected at polar regions was better than 10 cm in cells of 30 km × 30 km every 3 days (0.5° × 0.5° in 10 days over the rest of the oceans) (Cardellach et al., 2018).
Summarizing, a large body of analysis, simulations, and experimentation have shown the feasibility of GNSS-R and its utility in providing improved spatial and temporal coverage at a significantly lower cost than conventional active radar nadir or wide-swath altimetry. These studies have also confirmed the three key limitations of the GNSS signal, each of which can be improved through application of similar methods to Ku-K band communication satellite transmissions: (1) Increasing the bandwidth from 10’s of MHz in GNSS up to a maximum of 1 GHz in communication signals; (2) Increased EIRP due to the communication link budget requirement (50 dBW vs. 26 dBW); (3) Sensitivity to uncertainty in the ionospheric Total Electron Content (TEC) reduced to approximately 1–2% of that at L-band; and (4) Antenna dimensions reduced to approximately 9–13% of that at L-band (for center frequencies of 12–20 GHz) for the same gain.
Finally, although this section mainly covers the history on spaceborne GNSS-R for SSH measurement, it is worth noting that in past few years there has been some progress made on using ground based GNSS receivers to measure sea level (Larson et al., 2013, 2017). This technique uses existing network of geodetic GNSS antennas/receivers situated in view of the sea and receive the signal reflected by the sea (multipath) in the side lobes of the antenna. A recent 10-year comparison of water levels measured showed an RMS error of individual GPS water level estimates to be about 12 cm, with daily mean differences of about 2 cm with respect to conventional tide gauges (Larson et al., 2017). This is another promising method to measure coastal sea level (within a km from coast). This technique does, however, require the GPS stations to be properly sited near the shore and careful characterization of the study area for each of the station.
W-SoOp Overview – Theory and Algorithms
Modern digital satellite signals employ efficient compression and encryption, such that the transmitted signal can be approximated as a band-limited noise (Shah et al., 2012). Instruments designed to simultaneously measure these signals use similar interferometric techniques as those developed for GNSS-R, where the direct signal is used as a reference instead of a known pseudo-random noise (PRN) code. In this approach, existing wideband (∼400–500 MHz to 1 GHz) digital transmissions from communication and broadcast satellite services are re-utilized as illumination sources in a bistatic radar system. There are a number of satellite transmitters that operate from C- to K-band, close to the frequencies used for radar altimetry, as shown in Figure 9. Global coverage of coastal areas is possible over a small period of time using a constellation of receivers, due to the presence of large number of transmitters (as shown in an example in Figure 10). A constellation using W-SoOp is feasible because this technique requires low-cost passive receivers with small antennas, developed from satellite communication heritage, which can be launched on SmallSat or CubeSat.
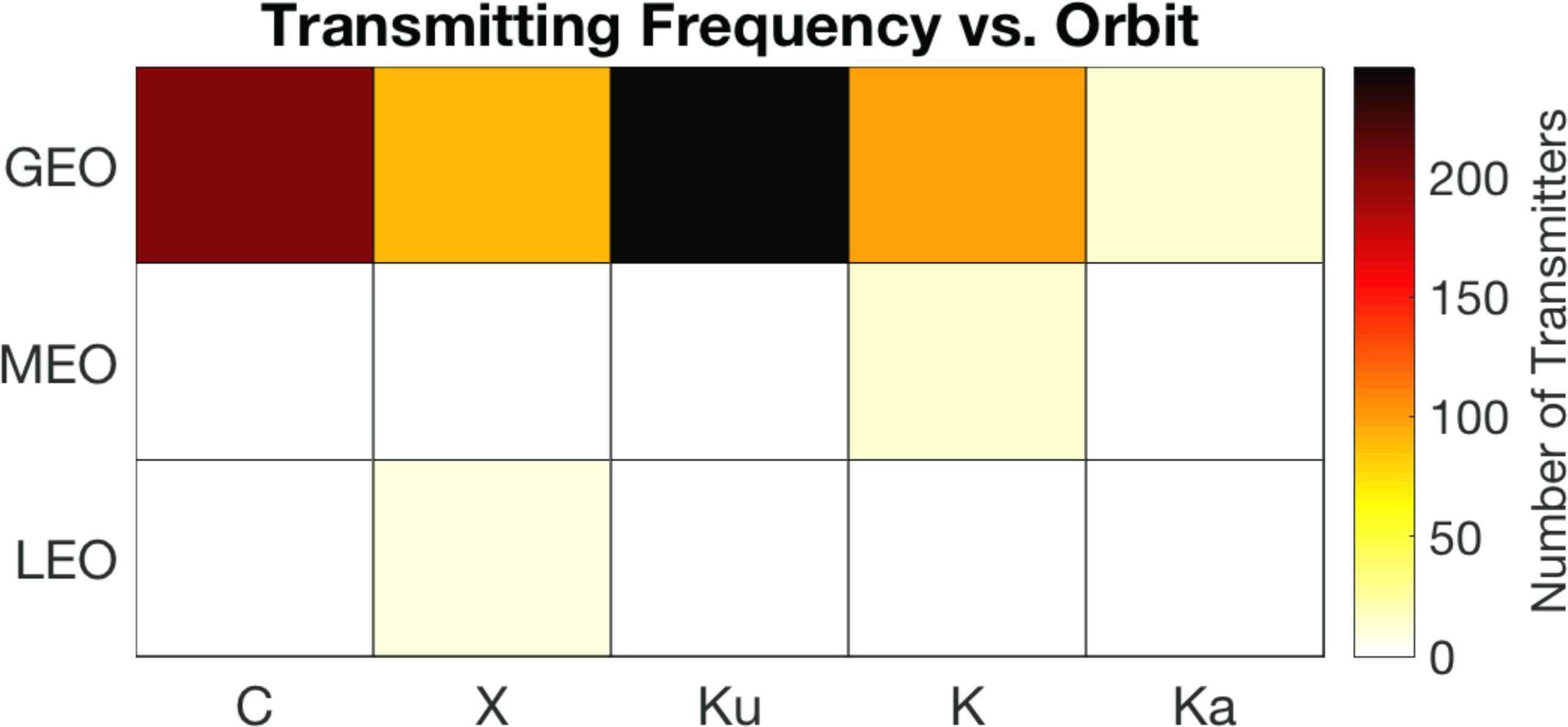
Figure 9. Number of currently operational spaceborne transmitters between C- and Ka-band frequencies.
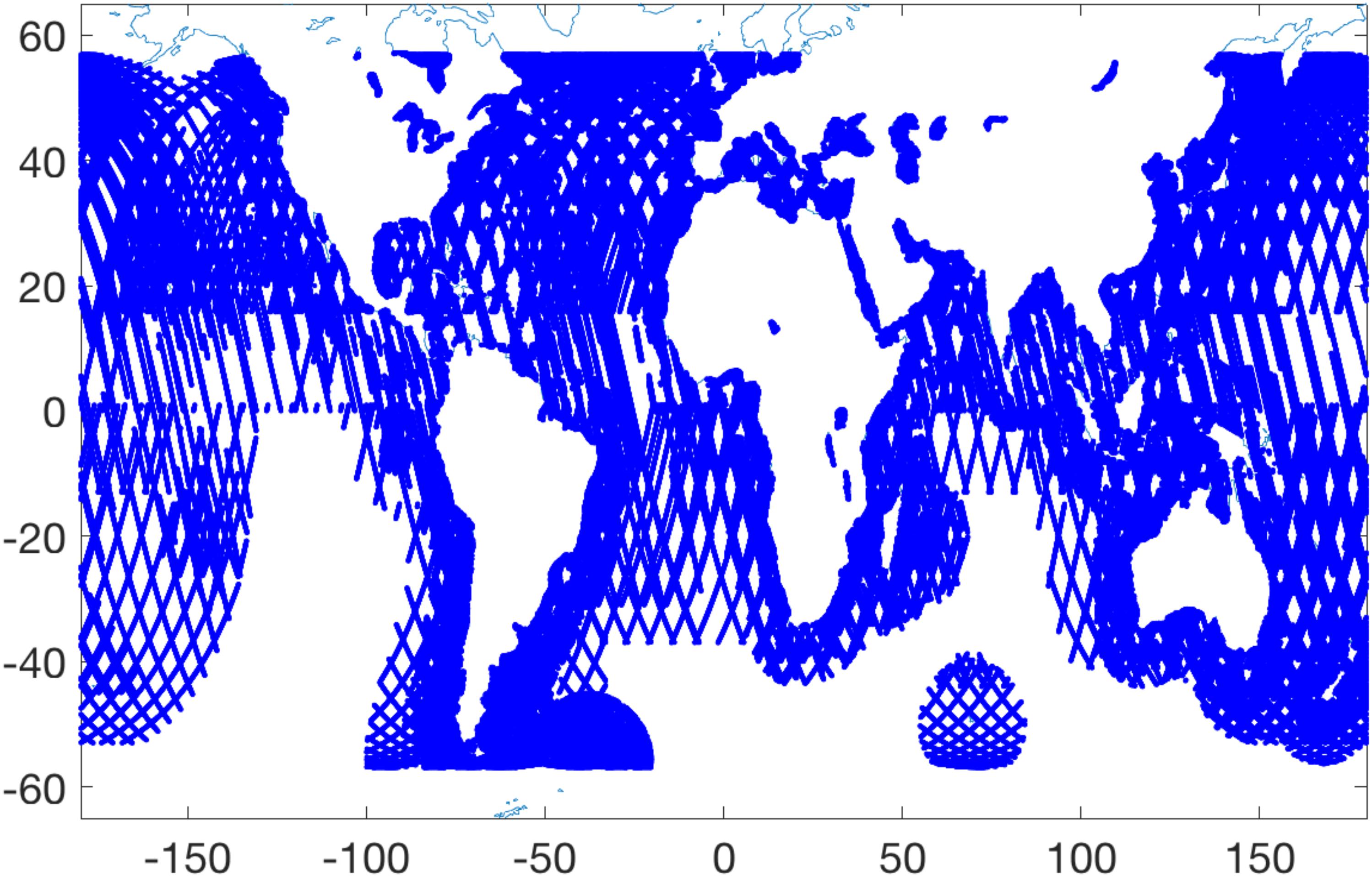
Figure 10. Possible coverage of coastal areas from SoOp LEO satellites receiving commercial Ku-band transmitters. This figure shows the extent of the coverage in coastal regions.
For W-SoOp measurement, simulations have shown that a 1–2 km spatial resolution is achievable from a space-based implementation using W-SoOp with 400–500 MHz bandwidth (Shah and Garrison, 2017). For altimetry measurement accuracy, a few cm in delay-precisions from coastal experiments was demonstrated using X-band digital TV signals (Ribó et al., 2014). Also, preliminary results from tower-based experiment that used both Ku and K-band transmissions from a commercial (DirecTV) direct broadcast satellite confirm the validity of the theoretical error model which predicts that the measurement precision from spaceborne receivers will be in the order of 5–6 cm (Shah and Garrison, 2017; Ho et al., 2019).
Challenges of W-SoOp
Unlike dedicated remote sensing instruments, where all design parameters are traded off to maximize the performance of the instrument, the use of SoOp faces unavoidable design constraints derived from the properties of the transmitting systems that were originally intended for other purposes, and operated independently of any proposed science mission. As mentioned above, challenges for GNSS-R were linked to the weak and narrow-band transmitted signals. These problems are well solved when using communication and digital TV broadcasting satellites as SoOp. In the trade for SoOp reflectometry, however, other problems emerge, such as insufficiently precise knowledge of the transmitters’ position, non-homogeneous coverage at the global (commercial operators will prefer to illuminate only densely inhabited areas, where their customers reside), the variable transmitted power for different transmitting channels, and the temporal variability of the allocation frequencies of a huge number of broadcast TV channels.
For coastal altimetry a major challenge is the estimation of the precise trajectory of the transmitting satellites. Although geosynchronous orbits are more predictable than LEO, the conventional positioning accuracy of the geostationary transmitters is in the range of few hundreds of meters (Rosengren et al., 2004; Guo et al., 2010). Frequent maneuvering of spacecraft and the limited public information available from the operators of these privately owned satellites adds even more uncertainty to estimates of their positioning. Several methods have been used or proposed in order to improve POD for geostationary orbits, including satellite laser ranging (SLR), the measurement of their angular position using ground-based telescopes (Montojo et al., 2011) and very-long-baseline interferometry (VLBI) delay and delay-rate tracking (Huang et al., 2011). Additional techniques, such as line-of-sight information from the LEO hosting a SoOp receiver and improved mathematical algorithms to numerically propagate orbit uncertainties still remain to be explored. An additional step forward in this direction is the incipient use of POD GNSS receivers aboard geosynchronous satellites. Although their use is mainly driven by their need during geosynchronous transfer orbits and electric thrusters (Marmet et al., 2015), next generation geosynchronous transmitters will also have POD (tens of meters). The most promising strategy will probably be a combination of several of these techniques to obtain POD of the GEOs that will enable altimetry with W-SoOp.
Commercial broadcast transmitters provide a large amount of transmitted TV channels, but they mainly transmit over densely populated areas. So, coverage with sufficient SNR over coastal areas is guaranteed, but not over open ocean. On the other hand, military transmitters with global coverage do exist (Kumar et al., 2005). These could be used to cover the illumination gap over open ocean if needed.
The periodic reallocation of TV channels at different frequency channels represents an additional logistic challenge for the instrument. Reprogramming capabilities of the receiver could easily solve the problem. In the long run, however, an intelligent instrument with decision making capabilities could increase the observational throughput.
Signal processing challenges are also envisaged, especially when the receiver has to cope with a large number of transmitters, channels, and polarizations within a very wide (1 GHz) bandwidth. Flexibility in the signal processing approach can be achieved with software defined radio (SDR) processors, although the current capacity of these technologies is limited to moderate bandwidths. A mixed processing solution based on SDR and highly efficient specialized hardware, as for instance hardware correlators or graphical processing units (GPU), may provide the necessary computational strength and flexibility at the same time. Finally, changes in the received power will have little effect in altimetric applications.
Scientific and Operational Applications
As mentioned above, in coastal oceans, small mesoscale eddies are energetic. As an example, Figure 11 top left shows daily means SSH features in the Monterey Bay region produced by a regional model at a high resolution of 3 km. The model is driven by realistic hourly atmospheric forcing and tidal forcing. The region teems with energetic eddies of smaller than 100 km, and the eddies change within days. Traditional altimeters are incapable of capturing these features as they sample this area with only a few passes in a given 10-day period (Figure 11, top right). Even the forthcoming SWOT mission cannot reliably observe these features because of its repeat cycle of 21 days.
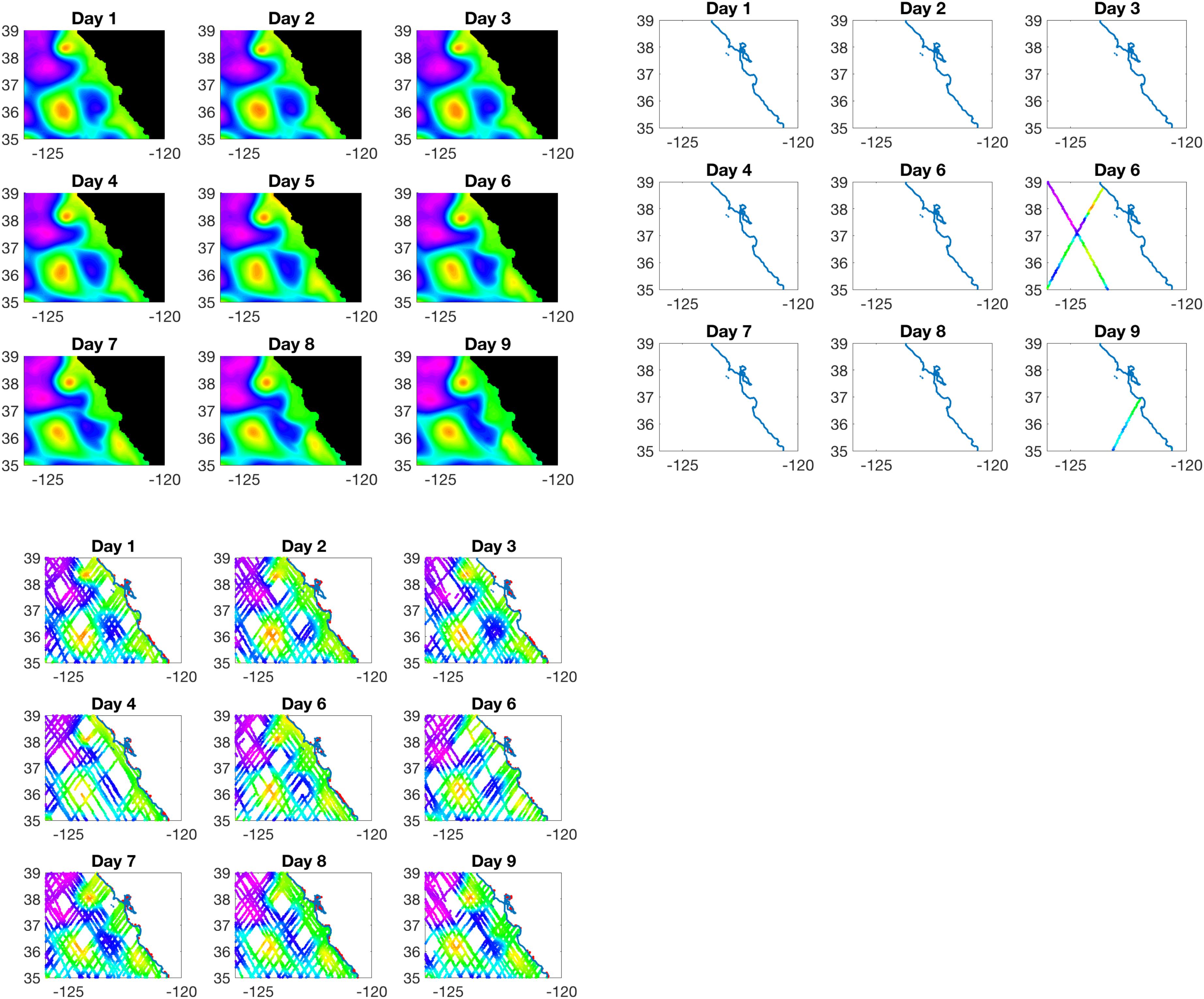
Figure 11. The top left figure shows how the features of Monterey Bay changes spatially and temporally through a 9-day simulation. The top right figure shows sampling by traditional altimeters (Jason-3) of Monterey Bay area in 9-day period. The bottom left figure shows how the features of Monterey Bay can be sampled spatially and temporally using a constellation of eight receivers capable of capturing Ku-band reflected signals.
Sampling of the area at higher temporal and spatial resolution is possible by a constellation of satellites recording Ku-band SoOp. This is shown in Figure 11 bottom left, where a coverage simulation was performed using 8 receivers in a 500 km orbit. The assumption is made that the receiver can pick any Ku-band signals and each receiver is capable of recording up to eight channels. It is observed that many distinct features can be observed from a constellation because of the dense spatial and temporal coverage. This is an improvement over the capabilities of existing orbital platforms.
Finally, the same coverage map is plotted against the known tsunamis on Pacific Ocean. Figure 12 shows the sampling of the tsunamis using eight SoOp receivers operating in Ku-band. It can be seen that if we have a modest constellation of eight receivers, it would be capable of observing most of the near-shore tsunamis. The near-shore observation is important for early warnings to save lives.
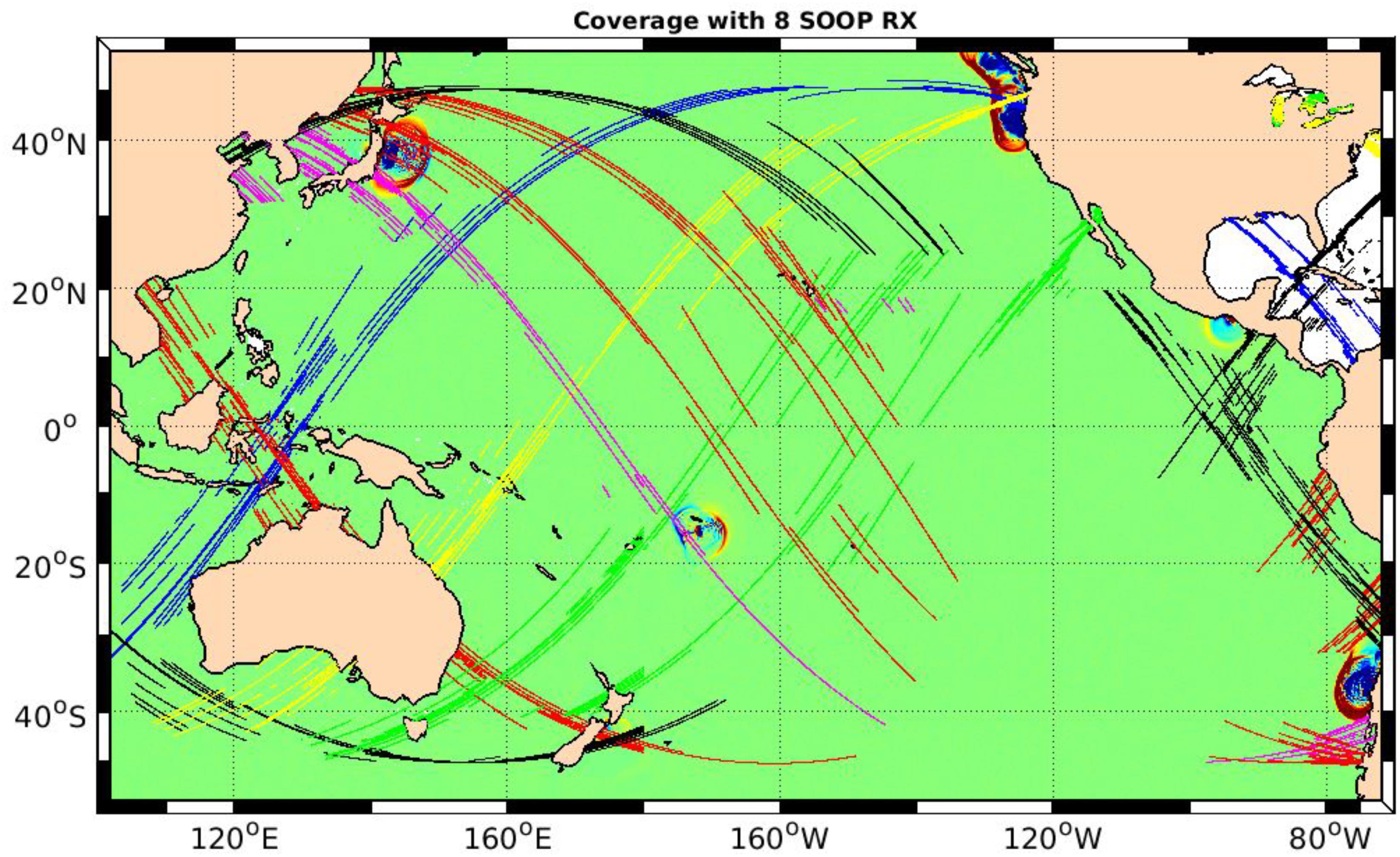
Figure 12. Previously occurred tsunamis in the Pacific. Eight SoOp Receivers (different colors) are simulated.
Summary
SSH W-SoOp has the potential to provide higher temporal and spatial resolution measurements enabling coastal ocean circulation measurements down to sub-mesoscale. These measurements will improve our understanding of multi-scale circulations and thus of biological and chemical variability. In addition to the science values, the spatial and temporal coverage of SoOp for both ocean and land has unique operational values for coastal management. Coastal zones, currently home to a large fraction of the world’s population, are under serious threat from sea level rise, coastal erosions, storm surges, and deadly tsunamis. As mentioned above, conventional satellite altimeters, as well as GNSS-R LEOs, have either too low temporal resolution or too weak SNR, which are inadequate for coastal applications. On the other hand, the W-SoOp has denser coverage in more populated areas; therefore, we believe the W-SoOp technology is ideal for coastal missions for both science and operational applications if the above-mentioned challenges can be resolved.
Roadmap and Recommendations for the Development of the SoOp Technique
The following steps need to be taken for the development of W-SoOp for coastal application measurements:
• Study the impact of POD uncertainty in the final altimetric retrieval, investigate possible ways to improve POD.
• Study the receiving capabilities: how many transmitting satellites are visible simultaneously from a given LEO scenario? How different the properties of these signals are?
• Study solutions for the 2-polarization, multi-beam steering receiving antennas and receiving RF chains, generate a technology development roadmap leveraging recent advances in satellite communications to the greatest extent possible.
• Study solutions for flexible onboard processing.
• Define achievable science objectives and applications: With current technology and anticipated improvements in the next 5–10 years, what are the new science objectives and applications of SoOp, which could be achieved by other missions at the same cost.
Opportunities for Integration
As mentioned in section “Monitoring Coastal Zones With Multiple Observing Systems,” the evolution of coastal zones results from several forcing factors (natural and anthropogenic). Assessing the impacts of present and future coastal evolution requires an understanding of the interactions between biophysical and socioeconomic systems and assets on land, and even in the adjacent sea. Looking at both land and sea, as well as natural and anthropogenic factors and related consequences, is the only way of pursuing the monitoring of such complex systems under various impacts (not only environmental). In addition, modeling and synthesis activities have to accompany the measurements so that the research can provide insight into the future evolution of coastal areas. To benefit a broad range of end users and a large variety of scientific and societal applications, a global database of various coastal products should be developed. International programs, e.g., in the context of Future Earth, could consider establishing a data repository gathering all needed coastal observations, whether collected locally or remotely.
Coastal altimetry is crucial for the coastal seas and is already enhancing the capabilities of coastal models to provide accurate physical parameters that can be integrated into biogeochemical applications of societal and economic importance. An example is how observed sea level falls due to El Niño impacted Indonesian corals (Ampou et al., 2017).
Data assimilation in coastal areas is currently not a routine practice. Recent advances in coastal ocean forecasting along the European Atlantic and Baltic coast arise from developments in numerical modeling, data assimilation and observational networks (Madsen et al., 2015; Stanev et al., 2016). The works combine observations and modeling and propose integrated coastal and regional ocean forecasting systems. Staneva et al. (2017) and Wiese et al. (2018) show the benefit of using atmospheric-wave regional coupled models when predicting extreme events. Comparison between model and Jason-2, SARAL/AltiKa and CryoSat-2 altimeter observations indicate that the two-way coupling improves the simulation of wind and wave parameters of the model (Wahle et al., 2017). Moreover, the quality of the Sentinel-3A SWH over coastal zone is found superior to previous altimeter data (Wiese et al., 2018).
Improving our understanding of coastal ocean processes requires multi-platform approaches combining innovative and traditional observing systems (both in situ and satellite) with high resolution numerical simulations (Kourafalou et al., 2015). During the last decade, progress has been made, for example in the Mediterranean Sea, a natural reduced-scale laboratory for the examination of processes of global importance with multiple interacting scales including shelf-slope exchanges.
Encouraging results concerning the use of autonomous underwater vehicles (gliders) in synergy with altimetry, in order to monitor dynamics in the Balearic Sea, have been obtained (Ruiz et al., 2009). Bouffard et al. (2010) developed innovative strategies to characterize horizontal ocean flows, specifically in terms of current velocity associated with filaments, eddies or shelf-slope flow modifications close to the coast. These methodologies were applied to a series of glider missions carried out almost simultaneously and co-localized along the altimeter tracks. The value added by combining remote and in situ sensors to validate, inter-calibrate, and improve observing data dedicated to coastal ocean studies has been shown (Pascual et al., 2013). For instance, high-resolution hydrographic fields from gliders revealed the presence relatively intense eddies, that were not correctly detected by standard altimeter fields. In this context, Escudier et al. (2013) proposed a two-step optimal interpolation scheme including a bathymetric constraint with the aim of improving the characterization of coastal and fine-scale features. Qualitative and quantitative comparisons with drifters, glider, and satellite sea surface temperature observations reveal that when the new altimetry products are used, a better agreement is obtained.
Another example is the integration of altimeter data in the coastal band (7–60 km from the seashore) together with measurements of the surface velocity from coastal High Frequency Radars that provide synoptic, high frequency and high-resolution data at the boundary between the ocean and the atmosphere. Troupin et al. (2015) shows clear evidence during a glider mission conducted along a track from the Franco-Indian SARAL/AltiKa altimeter (Verron et al., 2015) located in the Western Mediterranean close to Ibiza Island, where the SOCIB HF radar facility provided hourly surface current velocities. Surface drifters were also deployed in the studied region. Comparisons reveal a reasonable agreement between all platforms (drifter, SARAL/AltiKa, glider, and HF radar), with SARAL/AltiKa able to capture the northern edge of a meander, which lied on a shallow bathymetry less than 10 km from the coast. Pascual et al. (2015) and Verron et al. (2018) show that SARAL/AltiKa data can be retrieved at a distance of only 7 km from the coast. The derived velocities reveal coherent mesoscale features with high temporal variability among the different cycles and with general reasonable agreement with HF radar fields.
New generations of SAR altimeter missions have demonstrated that compared to conventional altimetry (or low-resolution mode), this technique significantly reduces the measurements level of noise and improves the along-track spatial resolution (Boy et al., 2017). The comparison between altimetry, ocean gliders and ADCP showed a significant improvement, order 30% in resolution and 42% in velocity accuracy using a synthetic aperture radar mode with respect to lower-resolution mode of conventional altimetry (Heslop et al., 2017). Integrating the three datasets provided valuable insight into the variability of oceanographic features, in an area of the Mediterranean that remains chronically under sampled and has demonstrated benefits to improve knowledge on coastal and fine-scale dynamics.
Future works will require expanded observing capabilities with new high-resolution experiments integrating multiplatform approaches with numerical simulations (see an example in Pascual et al., 2017 pointing to the predominance of fine-scale processes enhancing vertical exchanges between the upper ocean and the ocean interior). Particular emphasis will be devoted to the calibration and validation of the wide-swath SWOT altimeter, that will make an unprecedented contribution by enabling the first observations in the 40–100 km wavelength band (Gómez-Navarro et al., 2018).
The availability of processing platforms, such as the European Space Agency G-POD online service called SARvatore (SAR Versatile Altimetric Toolkit for Ocean Research and Exploitation) will further support the advanced exploitation of coastal altimetry data sets (Dinardo, 2014). Moreover, it is expected that new thematic processing platforms will permit integrating coastal altimetry with data from multiple sources (Clerc et al., 2016) enabling new opportunities to understand important ocean processes in the coastal zone that traditionally require sampling at appropriate temporal, spatial, and depths scales. Sustained funding mechanisms are essential to bring together end-user/modelers and coastal altimetry producers in order to go further in the integration.
W-SoOp is an emerging technology, which has potential to provide sub-diurnal temporal resolution through use of constellations. Because of the increased temporal resolution, this technique would be very complimentary to the existing as well as upcoming measurement in coastal zone in understanding short duration variation in ocean processes in coastal zone. However, before studies on integration with the existing observations are thoroughly explored, more work is needed in the technology development as elaborated in roadmap section in Section “Wideband Signals of Opportunity Reflectometry for Coastal Ocean Applications.”
End Users Engagement
Collecting information about coastal zones and their evolution has obvious societal interest. In addition to the science needed to increase knowledge about the processes that affect the coastal areas and about the complex changes these regions suffer, (e.g., in the context of international programs such as WCRP or Future Earth), a number of other stakeholders will benefit of multi-source, multi parameters information about coastal zones.
Although each country is organized differently, the management of coastal risks always includes users concerned with:
- The prevention of coastal hazards and adaptation to climate change, which generally involves private and public stakeholders such as coastal municipalities and economic stakeholders, within a process that can be part of a national or regional regulation or may follow sectoral guidance. For these stakeholders, the observational priority is to collect information allowing to assess present-days and future coastal hazards such as shoreline changes and flooding.
- Crisis management and preparedness, where the priorities is generally to save lives and where near real time services need to be set up, in order to inform the civil security or similar organization and organize the rescue appropriately. For example, the Copernicus Emergency Management system addresses this need by providing a service for rapid hazard mapping2.
- Post-disaster reconstruction, involving private and public stakeholders as well as the finance, insurance and reinsurance industries, during which coastal observations are required to assess future coastal risks in order to increase resilience and favor adaptation to climate change. In addition to these three traditional groups of users in the area of coastal risk management, a growing number of users is concerned with present-days climate change impacts (detection and attribution, e.g., Le Cozannet et al., 2014), its future impacts, and methods to evaluate the efficiency of adaptation strategies (Hallegatte, 2009). This usually include national to regional authorities and agencies in charge of developing adaptation strategies (Le Cozannet et al., 2017). To give an example, studies quantifying shoreline changes based on high-resolution imagery in atoll islands have allowed reconsidering adaptation priorities. Hence, the traditional debate focused on land losses has now shifted to a more integrated assessment of islands habitability, considering chronic and extreme flooding as well as the impacts of climate change on corals and water resources (Nurse et al., 2014). However, as the methods allowing maximizing the efficiency of adaptation are not well established yet, there are still research needs to assess how observations can best support adaptation.
In addition, in order to exploit the economic investments in space-based observing systems, the development of operational services based on EO data is a key goal. The operational services (downstream services) are based on the integration of multi-source information (EO, modeling, and in situ) with the institutional/policy, social and economic knowledge. The sustainability of these operational services can be guaranteed by their usefulness and thus their capacity to fulfill coastal user (intermediate and final) needs (Taramelli et al., 2014, 2015a, b).
Several coastal altimetry experiments showed that applying locally all the altimeter processing steps up to sea level anomalies at high-resolution is an efficient way to get a more accurate estimation of the coastal dynamics. In fact, outside the last tenth kilometers near the coast, ocean retracking algorithms perform well and a dedicated high-resolution processing, particularly data selection and noise reduction, enables to monitor small-scale patterns with more details, which is relevant for regional oceanographers. Figure 13 shows across-track geostrophic velocities computed from altimetric SLA in the Gulf of St Laurence (Canada) from the global (from Copernicus Marine Environment Monitoring Service, CMEMS) and regional high-resolution datasets. The statistical comparison of along-track altimeter to in situ sea level observation at three coastal tide gauges, gives better results with the HR regional product in term of correlation (between 0.6 and 0.9 depending on the altimeter point) and similar results in term of RMS of the difference (<0.06 m). This confirms that the good quality of the CMEMS altimeter data is maintained in the HR regional product.
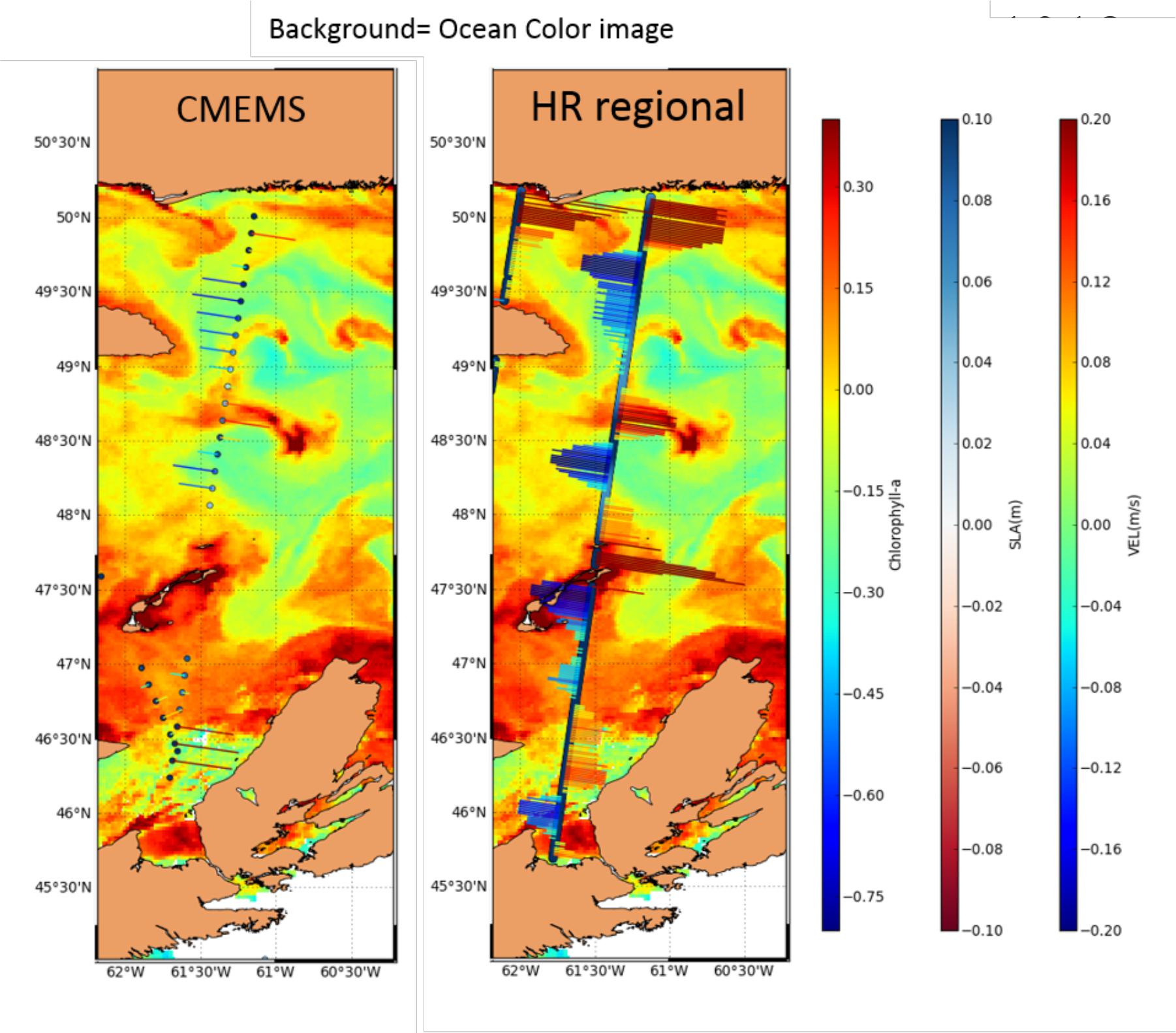
Figure 13. Stick-plot of across-track geostrophic velocities in the Gulf of St Lawrence (Canada) computed from altimeter SLA of the CMEMS global altimetry dataset (Left) and of a regional high-resolution altimetry dataset (Right). The background image deals with a high-resolution chlorophyll-a field produced by CLS processing chains for the same date on September 8, 2014. Copyright “Fisheries and Oceans Canada” and “Collecte Localisation Satellites.”
In terms of communication and capacity building, the Coastal Altimetry Workshop series organized for more than 10 years by ESA (Restano et al., 2018) have already attracted the regional ocean modeling community as end-users. A significant need has been expressed for continuous education of the user community on the data products and updates, as it is a daunting task for a user to determine which dataset to use. This objective can be achieved augmenting information for non-expert altimetrists and coastal oceanography users with, for example, clear statements of the time range of data availability for single altimeter platform data sets, and notes of proximity to the coast of valid data. Model users have also requested global unified multi-platform high along-track resolution products, such as what the TAPAS initiative (Tailored Altimeter Products for Assimilation Systems) is delivering for European seas. The TAPAS working group set up within CMEMS is a very good example of the way to proceed to engage end-users by establishing a strong link between Data Assimilation teams and Sea Level production center. Both communities are strengthened by the exchanges and more efficient research and development driven by data usage. A similar initiative called ARCOM for Altimetry for Regional and Coastal models was proposed in 2015 for extending the TAPAS experience to a larger community, targeting the GODAE OceanView Coastal and Shelf Seas Task Team (COSS-TT) group. A pilot workshop was conducted in 2015 in association with a COSS-TT international coordination meeting. The Coastal Altimetry community brought tutorials about altimetry processing and products to coastal modelers in order to feed discussions on the main issues (reference surfaces, geophysical corrections, observation errors). In 2017, a dedicated session was re-conducted in the COSS-TT meeting. The outcome of this last ARCOM event was a claim for more details to help making a choice between the available datasets (coverage in time and spatial, strength, etc.).
An example of end-user engagement is the ESA eSurge-Venice project that brought together research institutes and stakeholders (sea level forecast center of the Venice Municipality) and that has been set up to help improve the modeling of storm surges around city of Venice using coastal altimetry and scatterometry (De Biasio et al., 2017). The city of Venice is one example of worldwide places that are vulnerable to storm surges, being the city flooded several times a year. A barrier system, MOSE, is being operated to protect the city, however, for the opening/closure of the barrier accurate predictions of sea level is essential.
As for the W-SoOp technique, the technology is still in early days and the concept is still trying to carve out the best way to go get end-user engaged. Some of the modelers have recently started looking at OSSE studies to explore the potentials.
Conclusions
To understand the complexity of coastal zones and their evolution under multiple forcing processes, a broad variety of observations needs to be made with a long-term sustained perspective, and as global as possible coverage. This implies considerable investments in both space-based and in situ observing systems. In this paper, we have identified a number of parameters, which are require to be systematically monitored to improve knowledge on the forcing agents and the morphological changes of the shorelines in response to imposed forcing.
This paper recognizes the prominent role of local to regional coastal observatories in collecting, analyzing, and disseminating information derived from satellite, aerial and in situ coastal observations. This applies in the area of coastal sea-levels and related hazards (shoreline changes and flooding), as discussed in this paper, but also beyond that in the area of coastal environment and biodiversity monitoring (see e.g., Liu et al., 2015). We argue that a timely challenge for increasing the salience of coastal information is to connect global providers of EO data (such as space agencies) with the emerging networks of coastal observatories. In other fields of geosciences, several previous initiatives have efficiently established such links and stimulated the use of satellite-based observations in scientific and operational activities. This is the case for the ESA “supersites” or NASA “natural laboratories,” providing access to all geophysical data for key priority sites affected by earthquakes and volcanic eruptions. This could be an example to follow for coastal zone evolution.
Among the forcing agents, we have focused on coastal sea level monitoring by satellite altimetry in this paper. The spatial and temporal complexity of coastal sea level requires a fine-scale monitoring. Satellite altimetry is capable of providing a unique and global long-term observational dataset to characterize how sea level variability evolves from the open ocean to the coastal zone. It can help to fill the gap between open ocean and tide gauges. The extension of the satellite-based sea level record toward the coast with quality comparable to open ocean is a key requirement for a proper understanding of the variability of sea level at all temporal and spatial scales. Conventional altimetry fails to provide sea level data within 10 km from the coast. But efforts performed during the past decade by the international coastal altimetry community with support of space agencies, in particular ESA, have clearly demonstrated that retracking of radar echoes collected by the satellite as it approaches the coast and computation of new geophysical corrections optimized for the coastal areas (e.g., WTC, atmospheric loading, sea state bias, ocean tides) allow rescue of sea level data in this 10-km gap.
As discussed in Section “Coastal Zone Altimetry Processing and Exploitation: Progress and Outlook for the Next Decade,” detailed studies have been conducted in several pilot regions, showing that sea surface height data can be recovered with an improved precision near the coast, with the goal to reach the accuracy in open ocean. Studies have also shown that use of SAR/DDA data from the Sentinel-3 and CryoSat missions can provide even higher performances in coastal areas, both in terms of precision, 3–4 cm as close as 2–3 km to the coast (Dinardo et al., 2017), and resolution (up to 300 m to the coast – this can be improved by processing the data at the burst frequency, 80 Hz, 90 m, and further improved with the fully focused SAR processing). For scientific and societal applications, the next step is now to undertake systematic exploitation of altimetry-based data in order to deliver worldwide coastal sea level products, easily usable by a broad variety of end users. There is strong demand for such information from both the scientific community and other stakeholders.
On a longer perspective, we have also discussed promising new technology based on W-SoOp reflectometry to complement the information provided by satellite altimetry on sea level in the coastal zone. This technique can potentially get closer to the coast than conventional altimetry and with the use of constellation provide data at higher temporal resolution that can be used to study the dynamic coastal zone. The next step on this technique is to study the impact of POD uncertainty in the final altimetric retrieval, as this is currently the limiting error source for this technique. Additionally, in parallel, it is important to work with the scientific community to understand how these measurements can improve our understanding on coastal processes through OSSE studies.
Author Contributions
JBe assembled the manuscript. AC, SV, LF-M, and RSh wrote and edited most sections of the manuscript, other authors also wrote specific parts of the document. All authors contributed to manuscript revision, read and approved the submitted version.
Funding
Part of this work has been carried out with the financial support of the following research projects: Spanish research grant ESP2015-70014-C2-2-R (MINECO/FEDER) and Spanish Ministry of Science, Innovation and Universities: RTI2018-099008-B-C22, ESA contract Sea_Level_cci (No. 4000126561/19/I-NB).
Conflict of Interest Statement
The authors declare that the research was conducted in the absence of any commercial or financial relationships that could be construed as a potential conflict of interest.
Acknowledgments
This community white paper is the outcome of the merging, suggested by the OceanObs’19 organizers of three community white paper abstracts dedicated to the coastal zone, submitted by AC, SV, LF-M and RSh. JBe was designated as coordinator during the merging process. Part of the work that was carried out by the Jet Propulsion Laboratory, California Institute of Technology personnel in this manuscript was under a contract with the National Aeronautics and Space Administration.
Footnotes
References
Ablain, M., Legeais, J. F., Prandi, P., Marcos, M., Fenoglio-Marc, L., Benveniste, J., et al. (2016). Satellite altimetry-based sea level at global and regional scales. Surv. Geophys. 38, 7–31. doi: 10.1007/s10712-016-9389-8
Abulaitijiang, A., Andersen, O. B., and Stenseng, L. (2015). Coastal sea level from inland CryoSat-2 interferometric SAR altimetry. Geophys. Res. Lett. 42, 1841–1847. doi: 10.1002/2015GL063131
Ampou, E. E., Johan, O., Menkes, C. E., Niño, F., Birol, F., Ouillon, S., et al. (2017). Coral mortality induced by the 2015–2016 El-Niño in Indonesia: the effect of rapid sea level fall. Biogeosciences 14, 817–826. doi: 10.5194/bg-14-817-2017
Antony, C., Testut, L., and Unnikrishnan, A. S. (2014). Observing storm surges in the Bay of Bengal from satellite altimetry. Estuar. Coast. Shelf Sci. 151, 131–140. doi: 10.1016/j.ecss.2014.09.012
Arns, A., Dangendorf, S., Jensen, J., Talke, S., Bender, J., and Pattiaratchi, C. (2017). Sea-level rise induced amplification of coastal protection design heights. Sci. Rep. 7:40171. doi: 10.1038/srep40171
Bajo, M., De Biasio, F., Umgiesser, G., Vignudelli, S., and Zecchetto, S. (2017). Impact of using scatterometer and altimeter data on storm surge forecasting. Ocean Model. 113, 85–94. doi: 10.1016/j.ocemod.2017.03.014
Bird, E. C. F. (1985). Coastline changes: a global review. Geol. J. 152:108. doi: 10.1002/gj.3350210215
Birol, F., and Delebecque, C. (2014). Using high sampling rate (10/20 Hz) altimeter data for the observation of coastal surface currents: a case study over the northwestern Mediterranean Sea. J. Mar. Syst. 129, 318–333. doi: 10.1016/j.jmarsys.2013.07.009
Birol, F., Fuller, N. X., Lyard, F., Cancet, M., Niño, F., and Delebecque, C. (2017). Coastal applications from nadir altimetry: example of the X-TRACK regional products. Adv. Space Res. 59, 936–953. doi: 10.1016/j.asr.2016.11.005
Bonnefond, P., Laurain, O., Exertier, P., Boy, F., Guinle, T., Picot, N., et al. (2018a). Calibrating the SAR SSH of sentinel-3A and CryoSat-2 over the Corsica facilities. Remote Sens. 10:92. doi: 10.3390/rs10010092
Bonnefond, P., Verron, J., Aublanc, J., Babu, K. N., Bergé-Nguyen, M., Cancet, M., et al. (2018b). The benefits of the Ka-band as evidenced from the SARAL/AltiKa Altimetric mission: quality assessment and specificities of AltiKa data. Remote Sens. 10:83. doi: 10.3390/rs10010083
Bouffard, J., Naeije, M., Banks, C. J., Calafat, F. M., Cipollini, P., Snaith, H. M., et al. (2018). CryoSat ocean product quality status and future evolution. Adv. Space Res. 62, 1549–1563. doi: 10.1016/j.asr.2017.11.043
Bouffard, J., Pascual, A., Ruiz, S., Faugère, Y., and Tintoré, J. (2010). Coastal and mesoscale dynamics characterization using altimetry and gliders: a case study in the Balearic Sea. J. Geophys. Res. Oceans 115:C10029. doi: 10.1029/2009JC006087
Boy, F., Desjonquères, J.-D., Picot, N., Moreau, T., and Raynalm, M. (2017). CryoSat-2 SAR-mode over oceans: processing methods, global assessment, and benefits. IEEE Trans. Geosci. Remote Sens. 55, 148–158. doi: 10.1109/TGRS.2016.2601958
Brooks, B. A., Merrifield, M. A., Foster, J., Werner, C. L., Gomez, F., Bevis, M., et al. (2007). Space geodetic determination of spatial variability in relative sea level change, Los Angeles basin. Geophys. Res. Lett. 34:L01611. doi: 10.1029/2006GL028171
Brown, S. (2010). A novel near-land radiometer wet path-delay retrieval algorithm: application to the Jason-2/OSTM advanced microwave radiometer. IEEE Trans. Geosci. Remote Sens. 48, 1986–1992. doi: 10.1109/TGRS.2009.2037220
Buchhaupt, C., Fenoglio-Marc, L., Dinardo, S., Scharroo, R., and Becker, M. (2017). A fast convolution based waveform model for conventional and unfocused SAR altimetry. Adv. Space Res. 62, 1445–1463. doi: 10.1016/j.asr.2017.11.039
Cardellach, E., Wickert, J., Baggen, R., Benito, J., Camps, A., Catarino, N., et al. (2018). GNSS transpolar earth reflectometry exploriNg system (G-TERN): mission concept. IEEE Access 6, 13980–14018. doi: 10.1109/ACCESS.2018.2814072
Carrère, L., Lyard, F., Cancet, M., Guillot, A., and Picot, N. (2014). “FES 2014, a new tidal model - Validation results and perspectives for improvements,” in Proceedings of the ESA Living Planet Conference, 9–13 May 2016, ESA, Vol. 740, Prague.
Cazenave, A., and Le Cozannet, G. (2014). Sea level rise and its coastal impacts. Earths Future 2, 15–34. doi: 10.1002/2013EF000188
Cazenave, A., Le Cozannet, G., Benveniste, J., Woodworth, P., and Champollion, N. (2017). Monitoring the change of coastal zones from space. EOS 98. doi: 10.1029/2017EO085581
Cazenave, A., Palanisamy, H., and Ablain, M. (2018). Contemporary sea level changes from satellite altimetry: what have we learned? What are the new challenges? Adv. Space Res. 62, 1639–1653. doi: 10.1016/j.asr.2018.07.017
Chelton, D. B., Schlax, M. G., Samelson, R. M., and de Szoeke, R. A. (2007). Global observations of large oceanic eddies. Geophys. Res. Lett. 34:L15606. doi: 10.1029/2007GL030812
Chen, N., Han, G., Yang, J., and Chen, D. (2014). Hurricane Sandy storm surge observed by HY- 2A satellite altimetry and tide gauges. J. Geophys. Res. Oceans 119, 4542–4548. doi: 10.1002/2013JC009782
Cipollini, P., Benveniste, J., Birol, F., Fernandes, M. J., Obligis, E., Passaro, M., et al. (2017). “Satellite altimetry in coastal regions,” in Satellite Altimetry Over Oceans and Land Surfaces Earth Observation of Global Changes Book Series, eds D. Stammer and A. Cazenave (London: CRC Press), 644.
Clerc, S., Tuhoy, E., Mangin, A., Datcu, M., Vignudelli, S., Illuzzi, D., et al. (2016). New perspectives for the observation of coastal zones with the coastal thematic exploitation platform. Proc. Living Planet Symp. 740:27.
Crétaux, J.-F., Nielsen, K., Frappart, F., Papa, F., Calmant, S., and Benveniste, J. (2018). “Hydrological applications of satellite altimetry: rivers, lakes, man-made reservoirs, inundated areas,” in Satellite Altimetry Over Oceans and Land Surfaces, Earth Observation of Global Changes, eds D. Stammer and A. Cazenave (Boca Raton, FL: CRC Press), 459–504. doi: 10.1201/9781315151779-14
Cullen, R. A., and Wingham, D. J. (2002). “CryoSat level 1b processing algorithms and simulation results,” in Proceeding of Geoscience and Remote Sensing Symposium (IGARSS), Toronto. doi: 10.1109/IGARSS.2002.1026246
De Biasio, F., Bajo, M., Vignudelli, S., Umgiesser, G., and Zecchetto, S. (2017). Improvements of storm surge forecasting in the Gulf of Venice exploiting the potential of satellite data: the ESA DUE eSurge-Venice project. Eur. J. Remote Sens. 50, 428–441. doi: 10.1080/22797254.2017.1350558
De Biasio, F., Vignudelli, S., Della Valle, A., Umgiesser, G., Bajo, M., and Zecchetto, S. (2016). Exploiting the potential of satellite microwave remote sensing to hindcast the storm surge in the gulf of Venice. IEEE J. Sel. Top. Appl. Earth Obs. Remote Sens. 9, 5089–5105. doi: 10.1109/JSTARS.2016.2603235
Deng, X., and Featherstone, W. E. (2006). A coastal retracking system for satellite radar altimeter waveforms: application to ERS-2 around Australia. J. Geophys. Res. Oceans 111:C06012. doi: 10.1029/2005JC003039
Desportes, C., Obligis, E., and Eymard, L. (2007). On the wet tropospheric correction for altimetry in coastal regions. IEEE Trans. Geosci. Remote Sens. 45, 2139–2149. doi: 10.1109/TGRS.2006.888967
Dinardo, S. (2014). GPOD CryoSat-2 SARvatore Software Prototype User Manual. Available at: https://wiki.services.eoportal.org/tiki-index.php?page=GPOD+CryoSat-2+SARvatore+Software+Prototype+User+Manual (accessed 23 February 2018).
Dinardo, S., Fenoglio-Marc, L., Buchhaupt, C., Becker, M., Scharro, R., Fernandez, J., et al. (2017). Coastal SAR and PLRM altimetry in German bight and west Baltic sea. Adv. Space Res. 62, 1371–1404. doi: 10.1016/j.asr.2017.12.018
Dong, C., Xu, G., Han, G., Chen, N., He, Y., and Chen, D. (2018). Identification of tidal mixing fronts from high-resolution along-track altimetry data. Remote Sens. Environ. 209, 489–496. doi: 10.1016/j.rse.2018.02.047
Egido, A., and Smith, W. (2017). Fully focused SAR altimetry: ‘theory and applications. IEEE Trans. Geosci. Remote Sens. 55, 392–406. doi: 10.1109/TGRS.2016.2607122
Escudier, R., Bouffard, J., Pascual, A., Poulain, P. M., and Pujol, M. I. (2013). Improvement of coastal and mesoscale observation from space: application to the northwestern Mediterranean Sea. Geophys. Res. Lett. 40, 2148–2153. doi: 10.1002/grl.50324
Eurosion (2004). www.eurosion.org (accessed June 20, 2019).
Fenoglio, L., Dinardo, S., Buchhaupt, C., Uebbing, B., Scharroo, R., Kusche, J., et al. (2019). “Calibrating CryoSat-2 and Sentinel-3A sea surface heights along the German coast,” IAG Proceedings, Springer Verlag. doi: 10.1007/1345_2019_73
Fenoglio-Marc, L., Dinardo, S., Scharroo, R., Roland, A., Dutour, M., Lucas, B., et al. (2015a). The German bight: a validation of CryoSat-2 altimeter data in SAR mode. Adv. Space Res. 55, 2641–2656. doi: 10.1016/j.asr.2015.02.014
Fenoglio-Marc, L., Scharroo, R., Annunziato, A., Mendoza, L., Becker, M., and Lillibridge, J. (2015b). Cyclone Xaver seen by geodetic observations. Geophys. Res. Lett. 42, 9925–9932. doi: 10.1002/2015GL065989
Fernandes, M. J., and Lázaro, C. (2016). GPD+ wet tropospheric corrections for CryoSat-2 and GFO altimetry missions. Remote Sens. 8:851. doi: 10.3390/rs8100851
Fernandes, M. J., and Lázaro, C. (2018). Independent assessment of sentinel-3A wet tropospheric correction over the open and coastal ocean. Remote Sens. 10:484. doi: 10.3390/rs10030484
Fernandes, M. J., Lázaro, C., Ablain, M., and Pires, N. (2015). Improved wet path delays for all ESA and reference altimetric missions. Remote Sens. Environ. 169, 50–74. doi: 10.1016/j.rse.2015.07.023
Fernandes, M. J., Lazaro, C., Nunes, A. L., Pires, N., Bastos, L., and Mendes, V. B. (2010). GNSS-derived path delay: an approach to compute the wet tropospheric correction for coastal altimetry. IEEE Geosci. Remote Sens. Lett. 7, 596–600. doi: 10.1109/LGRS.2010.2042425
Fernandes, M. J., Lázaro, C., Nunes, A. L., and Scharroo, R. (2014). Atmospheric corrections for altimetry studies over inland water. Remote Sens. 6, 4952–4997. doi: 10.3390/rs6064952
Fernandes, M. J., Pires, N., Lázaro, C., and Nunes, A. L. (2013). Tropospheric delays from GNSS for application in coastal altimetry. Adv. Space Res. 51, 1352–1368. doi: 10.1016/j.asr.2012.04.025
Garrison, J. L., Komjathy, A., Zavorotny, V. U., and Katzberg, S. J. (2002). Wind speed measurement using forward scattered GPS signals. IEEE Trans. Geosci. Remote Sens. 40, 50–65. doi: 10.1109/36.981349
Gómez-Enri, J., Cipollini, P., Passaro, M., Vignudelli, S., Tejedor, B., and Coca, J. (2016). Coastal altimetry products in the strait of Gibraltar. IEEE Trans. Geosci. Remote Sens. 54, 5455–5466. doi: 10.1109/TGRS.2016.2565472
Gómez-Enri, J., Vignudelli, S., Cipollini, P., Coca, J., and González, C. J. (2017). Validation of CryoSat-2 SIRAL sea level data in the eastern continental shelf of the Gulf of Cadiz (Spain). Adv. Space Res. 62, 1405–1420. doi: 10.1016/j.asr.2017.10.042
Gómez-Navarro, L., Fablet, R., Mason, E., Pascual, A., Mourre, B., Cosme, E., et al. (2018). SWOT spatial scales in the western Mediterranean sea derived from pseudo-observations and an Ad Hoc filtering. Remote Sens. 10:599. doi: 10.3390/rs10040599
Guo, R., Hu, X., Tang, B., Huang, Y., Liu, L., Cheng, L., et al. (2010). Precise orbit determination for geostationary satellites with multiple tracking techniques. Chin. Sci. Bull. 55, 687–692. doi: 10.1007/s11434-010-0074-x
Hajj, G. A., and Zuffada, C. (2003). Theoretical description of a bistatic system for ocean altimetry using the GPS signal. Radio Sci. 38, 10–11. doi: 10.1029/2002RS002787
Hallegatte, S. (2009). Strategies to adapt to an uncertain climate change. Glob. Environ. Change 19, 240–247. doi: 10.1016/j.gloenvcha.2008.12.003
Han, G. (2017). “Altimeter surveys, coastal tides, and shelf circulation,” in Encyclopedia of Coastal Science, eds C. W. Finkl and C. Makowski (Berlin: Springer Science & Business Media). doi: 10.1007/978-3-319-48657-4_7-2
Han, G., Ma, Z., Chen, D., and Chen, N. (2012). Observing storm surges from space: hurricane Igor off Newfoundland. Sci. Rep. 2:1010. doi: 10.1038/srep01010
Han, G., Ma, Z., Chen, N., Chen, N., and Yang, J. (2017). Hurricane Isaac storm surges off Florida observed by Jason-1 and Jason-2 satellite altimeters. Remote Sens. Environ. 198, 244–253. doi: 10.1016/j.rse.2017.06.005
Heslop, E. E., Saìnchez-Romaìn, A., Pascual, A., Rodriìguez, D., Reeve, K. A., FaugeÌre, Y., et al. (2017). Sentinel-3A views ocean variability more accurately at finer resolution. Geophys. Res. Lett. 44, 12367–12374. doi: 10.1002/2017GL076244
Ho, S. C., Shah, R., Garrison, J. L., Mohammad, P. N., Scheonwald, A., Pannu, R., et al. (2019). Wideband ocean altimetry using Ku-band and K-band satellite signals of opportunity: proof of concept. IEEE Geosci. Remote Sens. Lett. 16, 1012–1016. doi: 10.1109/LGRS.2019.2891976
Huang, Y., Hu, X., Zhang, X., Jiang, D., Guo, R., Wang, H., et al. (2011). Improvement of orbit determination for geostationary satellites with VLBI tracking. Chin. Sci. Bull. 56, 2765–2772. doi: 10.3390/s130302911
Idžanović, M., Ophaug, V., and Andersen, O. B. (2017). Coastal sea level from CryoSat-2 SARIn altimetry in Norway. Adv. Space Res. 62, 1344–1357. doi: 10.1016/j.asr.2017.07.043
IOC (2012). Global Sea Level Observing System (GLOSS) Implementation Plan – 2012. UNESCO/IOC, IOC Technical Series No. 100. GOOS Report No. 194, JCOMM Technical Report No. 66, 41. Available at: http://unesdoc.unesco.org/images/0021/002178/217832e.pdf (accessed June 20, 2019).
Jebri, F., Birol, F., Zakardjian, B., Bouffard, J., and Sammari, C. (2016). Exploiting coastal altimetry to improve the surface circulation scheme over the central Mediterranean Sea. J. Geophys. Res. Oceans 121, 4888–4909. doi: 10.1002/2016jc011961
Kourafalou, V. H., De Mey, P., Le Hénaff, M., Charria, G., Edwards, C. A., He, R., et al. (2015). Coastal Ocean Forecasting: system integration and evaluation. J. Operat. Oceanogr. 8, s127–s146. doi: 10.1080/1755876X.2015.1022336
Kumar, R., Taggart, D., Monzingo, R., and Goo, G. (2005). “Wideband gapfiller satellite (WGS) system,” in Proceedings of the 2005 IEEE Aerospace Conference, (Big Sky, MT: IEEE), 1410–1417.
Larson, K. M., Ray, R. D., Nievinski, F. G., and Freymueller, J. T. (2013). The accidental tide gauge: a GPS reflection case study from Kachemak Bay, Alaska. IEEE Geosci. Remote Sensing Lett. 10, 1200–1204. doi: 10.1109/LGRS.2012.2236075
Larson, K. M., Ray, R. D., and Williams, S. D. P. (2017). A 10-year comparison of water levels measured with a geodetic GPS receiver versus a conventional tide gauge. J. Atmos. Ocean Technol. 34, 295–307. doi: 10.1175/JTECH-D-16-0101.1
Le Cozannet, G., Daniel, R., Guy, W., Manuel, G., Sylvestre, D. S., Benoit, M., et al. (2015). Vertical ground motion and historical sea-level records in Dakar (Senegal). Environ. Res. Lett. 10:084016. doi: 10.1088/1748-9326/10/8/084016
Le Cozannet, G., Garcin, M., Yates, M., Idier, D., and Meyssignac, B. (2014). Approaches to evaluate the recent impacts of sea-level rise on shoreline changes. Earth Sci. Rev. 138, 47–60. doi: 10.1016/j.earscirev.2014.08.005
Le Cozannet, G., Nicholls, R. J., Hinkel, J., Sweet, W. V., McInnes, K. L., Van de Wal, R. S., et al. (2017). Sea level change and coastal climate services: the way forward. J. Mar. Sci. Eng. 5:49. doi: 10.3390/jmse5040049
Legeais, J. F., Ablain, M., Zawadzki, L., Zuo, H., Johannessen, J., Schaffenberg, M., et al. (2018). An improved and homogeneous altimeter sea level record from the ESA climate change initiative. Earth Syst. Sci. Data 10, 281–301. doi: 10.5194/essd-10-281-2018
Levin, J., Wilkin, J., Fleming, N., and Zavala-Garay, J. (2018). Mean circulation of the Mid-Atlantic Bight from a climatological data assimilative model. Ocean Model. 128, 1–14. doi: 10.1016/j.ocemod.2018.05.003
Li, X. H., Yang, G., Chen, J., Zheng, D., and Chen, G. (2018). Using satellite altimetry to calibrate the simulation of typhoon Seth storm surge off Southeast China. Remote Sens. 10:657. doi: 10.3390/rs10040657
Lillibridge, J., Lin, M., and Shum, C. K. (2013). Hurricane Sandy storm surge measured by satellite altimetry. Oceanography 26, 8–9. doi: 10.5670/oceanog.2013.18
Liu, Y., Kerkering, H., and Weisberg, R. H. (2015). “Introduction to coastal ocean observing systems,” in Coastal Ocean Observing Systems, eds Y. Liu, H. Kerkering, and R. H. Weisberg (Cambridge, MA: Academic Press), 1–10. doi: 10.1016/b978-0-12-802022-7.00001-8
Loisel, H., Mangin, A., Vantrepotte, V., Dessailly, D., Dinh, D. N., Garnesson, P., et al. (2014). Variability of suspended particulate matter concentration in coastal waters under the Mekong’s influence from ocean color (MERIS) remote sensing over the last decade. Remote Sens. Environ. 150, 218–230. doi: 10.1016/j.rse.2014.05.006
Luijendijk, A., Hagenaars, G., Ranasinghe, R., Baart, F., Donchyts, G., and Aarninkhof, S. (2018). The state of the world’s beaches. Sci. Rep. 8:6641.
Madsen, K. S., Høyer, J. L., Fu, W., and Donlon, C. (2015). Blending of satellite and tide gauge sea level observations and its assimilation in a storm surge model of the North Sea and Baltic Sea. J. Geophys. Res. Oceans 120, 6405–6418. doi: 10.1002/2015JC011070
Marmet, F. X., Maureau, J., Calaprice, M., and Aguttes, J. P. (2015). GPS/Galileo navigation in GTO/GEO orbit. Acta Astronaut. 117, 263–276. doi: 10.1016/j.actaastro.2015.08.008
Martín-Neira, M. (1993). A passive reflectometry and interferometry system (PARIS): application to ocean altimetry. ESA J. 17, 331–355.
Martín-Neira, M., D’Addio, S., Buck, C., Floury, N., and Prieto-Cerdeira, R. (2011). The PARIS ocean altimeter in-Orbit demonstrator. IEEE Trans. Geosci. Remote Sens. 49(6 Part 2), 2209–2237. doi: 10.1109/TGRS.2010.2092431
Massonnet, D., and Feigl, K. L. (1998). Radar interferometry and its application to changes in the Earth’s surface. Rev. Geophys. 36, 441–500. doi: 10.1029/97RG03139
Melet, A., Meyssignac, B., Almar, R., and Le Cozannet, G. (2018). Under-estimated wave contribution to coastal sea-level rise. Nat. Clim. Change 8, 234–239. doi: 10.1038/s41558-018-0088-y
Menéndez, M., and Woodworth, P. L. (2010). Changes in extreme high water levels based on a quasi-global tide-gauge dataset. J. Geophys. Res. 115:C10011. doi: 10.1029/2009JC005997
Milliman, J. D., and Farnsworth, K. L. (2013). River Discharge to the Coastal Ocean: A Global Synthesis. Cambridge: Cambridge University Press.
Montojo, F. J., López Moratalla, T., and Abad, C. (2011). Astrometric positioning and orbit determination of geostationary satellites. Adv. Space Res. 47, 1043–1053. doi: 10.1016/j.asr.2010.11.025
Nicholls, R. J. (2010). “Impacts of and responses to sea level rise,” in Understanding Sea Level Rise and Variability, eds J. A. Church, P. L. Woodworth, T. Aarup, and W. S. Wilson (Hoboken, NJ: Wiley-Blackwell).
Nurse, L. A., McLean, R. F., Agard, J., Briguglio, L. P., Duvat-Magnan, V., Pelesikoti, N., et al. (2014). “Small islands,” in Climate Change : Impacts, Adaptation, and Vulnerability; Part B: Regional Aspects; Contribution of Working Group II to the Fifth Assessment Report of the Intergovernmental Panel on Climate Change, eds V. R. Barros, C. B. Field, D. J. Dokken, M. D. Mastrandrea, K. J. Mach, T. E. Bilir, et al. (Cambridge: Cambridge University Press), 1613–1654.
Ouillon, S., Douillet, P., and Andréfouët, S. (2004). Coupling satellite data with in situ measurements and numerical modeling to study fine suspended-sediment transport: a study for the lagoon of New Caledonia. Coral Reefs 23, 109–122. doi: 10.1007/s00338-003-0352-z
Parrinello, T., Shepherd, A., Bouffard, J., Badessi, S., Casal, T., Davidson, M., et al. (2018). CryoSat: ESA’s ice mission – Eight years in space. Adv. Space Res. 62, 1178–1190. doi: 10.1016/j.asr.2018.04.014
Pascual, A., Bouffard, J., Ruiz, S., Nardelli, B. B., Vidal-Vijande, E., Escudier, R., et al. (2013). Recent improvements in mesoscale characterization of the western Mediterranean Sea: synergy between satellite altimetry and other observational approaches. Sci. Mar. 77, 19–36. doi: 10.3989/scimar.03740.15A
Pascual, A., Lana, A., Troupin, C., Ruiz, S., Faugère, Y., Escudier, R., et al. (2015). Assessing SARAL/AltiKa data in the coastal zone: comparisons with HF radar observations. Mar. Geod. 38, 260–276. doi: 10.1080/01490419.2015.1019656
Pascual, A., Ruiz, S., Olita, A., Troupin, C., Claret, M., Casas, B., et al. (2017). A multiplatform experiment to unravel meso- and submesoscale processes in an intense front (AlborEx). Front. Mar. Sci. 4:39. doi: 10.3389/fmars.2017.00039
Passaro, M., Cipollini, P., and Benveniste, J. (2015a). Annual sea level variability of the coastal ocean: the Baltic Sea-North Sea transition zone. J. Geophys. Res. Oceans 120, 3061–3078. doi: 10.1002/2014JC010510
Passaro, M., Fenoglio-Marc, L., and Cipollini, P. (2015b). Validation of significant wave height from improved satellite altimetry in the German Bight. IEEE Trans. Geosci. Remote Sens. 53, 2146–2156. doi: 10.1109/TGRS.2014.2356331
Passaro, M., Cipollini, P., Vignudelli, S., Quartly, G. D., and Snaith, H. M. (2014). ALES: a multi-mission adaptive subwaveform retracker for coastal and open ocean altimetry. Remote Sens. Environ. 145, 173–189. doi: 10.1016/j.rse.2014.02.008
Passaro, M., Dinardo, S., Quartly, G. D., Snaith, H. M., Benveniste, J., Cipollini, P., et al. (2016). Cross-calibrating ALES Envisat and CryoSat-2 Delay–Doppler: a coastal altimetry study in the Indonesian Seas. Adv. Space Res. 58, 289–303. doi: 10.1016/j.asr.2016.04.011
Passaro, M., Rose, S. K., Andersen, O. B., Boergens, E., Calafat, F. M., Dettmering, D., et al. (2018). ALES+: adapting a homogenous ocean retracker for satellite altimetry to sea ice leads, coastal and inland waters. Remote Sens. Environ. 211, 456–471. doi: 10.1016/j.rse.2018.02.074
Peltier, W. R. (2004). Global glacial isostasy and the surface of the ice-age Earth. Annu. Rev. Earth Planet. Sci. 32, 111–149. doi: 10.1146/annurev.earth.32.082503.144359
Peng, F., and Deng, X. (2018). A new retracking technique for Brown peaky altimetric waveforms. Mar. Geod. 41, 99–125. doi: 10.1080/01490419.2017.1381656
Piccioni, G., Dettmering, D., Passaro, M., Schwatke, C., Bosch, W., and Seitz, F. (2018). Coastal improvements for tide models: the impact of ALES retracker. Remote Sens. 10:700. doi: 10.3390/rs10050700
Piecuch, C. G., Bittermann, K., Kemp, A. C., Ponte, R. M., Little, C. M., Engelhart, S. E., et al. (2018). River-discharge effects on United States Atlantic and Gulf coast sea-level changes. Proc. Natl. Acad. Sci. U.S.A. 115, 7729–7734. doi: 10.1073/pnas.1805428115
Poupardin, A., Poupardin, A., Idier, D., Michele, M., and Raucoules, D. (2016). Water depth inversion from a single SPOT-5 dataset. IEEE Trans. Geosci. Remote Sens. 54, 2329–2342. doi: 10.1109/TGRS.2015.2499379
Pugh, D. T., and Woodworth, P. L. (2014). Sea-Level Science: Understanding Tides, Surges, Tsunamis and Mean Sea-Level Changes. Cambridge: Cambridge University Press.
Ranasinghe, R. (2016). Assessing climate change impacts on open sandy coasts: a review. Earth Sci. Rev. 160, 320–332. doi: 10.1016/j.earscirev.2016.07.011
Raney, K. R. (1998). The Delay Doppler radar altimeter. IEEE Trans. Geosci. Remote Sens. 36, 1578–1588. doi: 10.1109/36.718861
Raucoules, D., Cozanneta, G., Wöppelmann, G., Michele, M., Gravelle, M., Daag, A., et al. (2013). High nonlinear urban ground motion in Manila (Philippines) from 1993 to 2010 observed by DInSAR: implications for sea-level measurement. Remote Sens. Environ. 139, 386–397. doi: 10.1016/j.rse.2013.08.021
Ray, C., Martin-Puig, C., Clarizia, M. P., Ruffini, G., Dinardo, S., Gommenginger, C., et al. (2015). SAR altimeter backscattered waveform model. IEEE Trans. Geosci. Remote Sens. 53, 911–919. doi: 10.1109/TGRS.2014.2330423
Ray, R. D., and Egbert, G. D. (2017). “Tides and satellite altimetry,” in Satellite Altimetry over Oceans and Land Surfaces, eds D. Stammer and A. Cazenave (Boca Raton, FL: CRC Press), 427–458. doi: 10.1201/9781315151779-13
Restano, M., Passaro, M., and Benveniste, J. (2018). New achievements in coastal altimetry. Eos 99. doi: 10.1029/2018EO106087
Ribó, S., Arco, J. C., Oliveras, S., Cardellach, E., Rius, A., and Buck, C. (2014). Experimental results of an X-Band PARIS receiver using digital satellite TV opportunity signals scattered on the sea surface. IEEE Trans. Geosci. Remote Sens. 52, 5704–5711. doi: 10.1109/TGRS.2013.2292007
Rodriguez, E., Fernandez, D. E., Peral, E., Curtis, C., De Bleser, J. W., and Williams, B. (2018). “Wide-swath altimetry a review, in satellite altimetry over oceans and land surfaces,” in Satellite Altimetry Over Oceans and Land Surfaces Earth Observation of Global Changes Book Series, eds D. Stammer and A. Cazenave (London: CRC Press), 644.
Roscher, R., Uebbing, B., and Kusche, J. (2017). STAR: spatio-temporal altimeter waveform retracking using sparse representation and conditional random fields. Remote Sens. Environ. 201, 148–164. doi: 10.1016/j.rse.2017.07.024
Rosengren, M., De Vicente-Olmedo, J., and Pedersen, F. (2004). Keeping track of geostationary satellites - a novel and less costly approach. ESA Bull. 119, 64–68.
Rubio, A., Caballero, A., Orfila, A., Hernández-Carrasco, I., Ferrer, L., González, M., et al. (2018). Eddy-induced cross-shelf export of high Chl-a coastal waters in the SE Bay of Biscay. Remote Sens. Environ. 205, 290–304. doi: 10.1016/j.rse.2017.10.037
Ruiz, S., Pascual, A., Garau, B., Faugère, Y., Alvarez, A., and Tintoré, J. (2009). Mesoscale dynamics of the Balearic Front, integrating glider, ship and satellite data. J. Mar. Syst. 78, S3–S16. doi: 10.1016/j.jmarsys.2009.01.007
Salazar-Ceciliano, J., Trasviña-Castro, A., and González-Rodríguez, E. (2018). Coastal currents in the Eastern Gulf of Tehuantepec from coastal altimetry. Adv. Space Res. 62, 866–873. doi: 10.1016/j.asr.2018.05.033
Shah, R., and Garrison, J. L. (2017). Precision of Ku-band reflected signals of opportunity altimetry. IEEE Geosci. Remote Sens. Lett. 14, 1840–1844. doi: 10.1109/LGRS.2017.2737949
Shah, R., Garrison, J. L., and Grant, M. S. (2012). Demonstration of bistatic radar for ocean remote sensing using communication satellite signals. IEEE Geosci. Remote Sens. Lett. 9, 619–623. doi: 10.1109/LGRS.2011.2177061
Smith, W. H., Leuliette, E. W., Passaro, M., Quartly, G., and Cipollini, P. (2017). “Covariant errors in ocean retrackers evaluated using along-track cross-spectra,” in Proceedings of the Ocean Surface Topography Science Team (OSTST) Meeting, Miami, FL.
Smith, W. H., and Scharroo, R. (2015). Waveform aliasing in satellite radar altimetry. IEEE Trans. Geosci. Remote Sens. 53, 1671–1682. doi: 10.1109/TGRS.2014.2331193
Stanev, E., Schulz-Stellenfleth, J., Staneva, J., Grayek, S., Grashorn, S., Behrens, A., et al. (2016). Ocean forecasting for the German Bight: from regional to coastal scales. Ocean Sci. 12, 1105–1136. doi: 10.5194/os-12-1105-2016
Staneva, J., Alari, V., Breivik, Ø., Bidlot, J.-R., and Mogensen, K., (2017). Effects of wave-induced forcing on a circulation model of the North Sea. Ocean Dyn. 67, 81–101. doi: 10.1007/s10236-016-1009-0
Staneva, J., Wahle, K., Koch, W., Behrens, A., Fenoglio-Marc, L., and Stanev, E. (2016). Coastal flooding: impact of waves on storm surge during extremes. A case study for the German Bight. Nat. Hazards Earth Syst. Sci. 16, 2373–2389. doi: 10.5194/nhess-2016-227
Suanez, S., Manuel, G., Thomas, B., Mathias, R., Loïc, L., Laurence, D., et al. (2012). Les observatoires du trait de côte en France métropolitaine et dans les DOM. EchoGéo 19. doi: 10.4000/echogeo.12942
Syvitski, J. P., Vörösmarty, C. J., Kettner, A. J., and Green, P. (2005). Impact of humans on the flux of terrestrial sediment to the global coastal ocean. Science 308, 376–380. doi: 10.1126/science.1109454
Tamisiea, M. E. (2011). Ongoing glacial isostatic contributions to observations of sea level change. Geophys. J. Int. 186, 1036–1044. doi: 10.1111/j.1365-246X.2011.05116.x
Taramelli, A., Matteo, L. D., Ciavola, P., Guadagnano, F., and Tolomeid, C. (2015a). Temporal evolution of patterns and processes of the coastal area in Bevano Estuary (Northern Adriatic) Italy. Ocean Coast. Manag. 108, 74–88. doi: 10.1016/j.ocecoaman.2014.06.021
Taramelli, A., Valentini, E., and Cornacchia, L. (2015b). “Remote sensing solutions to monitor biotic and abiotic dynamics in coastal ecosystems,” in Coastal Zones Solutions for the 21st Century, Vol. 8, eds J. Baztan, O. Chouinard, B. Jorgensen, P. Tett, J.-P. Vanderlinden, and L. Vasseur (Amsterdam: Elsevier), 125–135.
Taramelli, A., Valentini, E., Cornacchia, L., Monbaliu, J., Thompson, R. C., Mandrone, S., et al. (2014). Modeling uncertainty in estuarine system by means of combined approach of optical and radar remote sensing. Coast. Eng. 87, 77–96. doi: 10.1016/j.coastaleng.2013.11.001
Tarpanelli, A., Brocca, L., Barbetta, S., Faruolo, M., Lacava, T., and Moramarco, T. (2015). Coupling MODIS and radar altimetry data for discharge estimation in poorly gauged river basins. IEEE J. Sel. Top. Appl. 8, 141–148. doi: 10.1109/jstars.2014.2320582
The WCRP Global Sea Level Budget Group (2018). Global sea level budget, 1993-present. Earth Syst. Sci. Data 10, 1551–1590. doi: 10.5194/essd-10-1551-2018
Toublanc, F., Ayoub, N. K., Lyard, F., Marsaleix, P., and Allain, D. J. (2018). Tidal downscaling from the open ocean to the coast: a new approach applied to the Bay of Biscay. Ocean Model. 124, 16–32. doi: 10.1016/j.ocemod.2018.02.001
Troupin, C., Pascual, A., Valladeau, G., Pujol, I., Lana, A., Heslop, E., et al. (2015). Illustration of the emerging capabilities of SARAL/AltiKa in the coastal zone using a multi-platform approach. Adv. Space Res. 55, 51–59. doi: 10.1016/j.asr.2014.09.011
Turki, I., Laignel, B., Chevalier, L., Costa, S., and Massei, N. (2015). On the investigation of the sea level variability in coastal zones using SWOT satellitemission: example of the eastern English Channel (western France). IEEE J. Sel. Top. Appl. Earth Obs. Remote Sens. 8, 1564–1569. doi: 10.1109/JSTARS.2015.2419693
Verron, J., Bonnefond, P., Aouf, L., Birol, F., Bhowmick, S. A., and Calmant, S. (2018). The benefits of the Ka-Band as evidenced from the SARAL/AltiKa altimetric mission: scientific applications. Remote Sens. 10:163. doi: 10.3390/rs10020163
Verron, J., Sengenes, P., Lambin, J., Noubel, J., Steunou, N., Guillot, A., et al. (2015). The SARAL/AltiKa altimetry satellite mission. Mar. Geod. 38, 2–21. doi: 10.1080/01490419.2014.1000471
Vignudelli, S., Cipollini, P., Roblou, L., Lyard, F., Gasparini, G. P., Manzella, G., et al. (2005). Improved satellite altimetry in coastal systems: case study of the Corsica Channel (Mediterranean Sea). Geophys. Res. Lett. 32:L07608. doi: 10.1029/2005GL022602
Vignudelli, S., Kostianoy, A. G., Cipollini, P., and Benveniste, J. (eds). (2011). Coastal Altimetry. Berlin: Springer-Verlag, 578. doi: 10.1007/978-3-642-12796-0
Vitousek, S., Barnard, P. L., Fletcher, C. H., Frazer, N., Erikson, L., Curt, D., et al. (2017). Doubling of coastal flooding frequency within decades due to sea-level rise. Sci. Rep. 7:1399. doi: 10.1038/s41598-017-01362-7
Vousdoukas, M. I., Mentaschi, L., Voukouvalas, E., Verlaan, M., Jevrejeva, S., and Jackson, L. P. (2018). Global probabilistic projections of extreme sea levels show intensification of coastal flood hazard. Nat. Commun. 9:2360. doi: 10.1038/s41467-018-04692-w
Vu, P. L., Frappart, F., Darrozes, J., Marieu, V., Blarel, F., Ramillien, G., et al. (2018). Multi-satellite altimeter validation along the French Atlantic coast in the southern bay of Biscay from ERS-2 to SARAL. Remote Sens. 10:93. doi: 10.3390/rs10010093
Wahle, K., Staneva, J., Koch, W., Fenoglio, L., Ho-Hagemann Ha, T., and Stanev, E. (2017). An atmosphere-wave regional coupled model: improving predictions of wave heights and surface winds in the southern North Sea. Ocean Sci. 13, 289–301. doi: 10.5194/os-13-289-2017
Warrick, J. A., Mertes, L. A. K., Siegel, D. A., and Mackenzie, C. (2004). Estimating suspended sediment concentrations in turbid coastal waters of the Santa Barbara Channel with SeaWiFS. Int. J. Remote Sens. 25, 1995–2002. doi: 10.1080/01431160310001619535
Wickert, J., Cardellach, E., Martin-Neira, M., Bandeiras, J., Bertino, L., Andersen, O. B., et al. (2016). GEROS-ISS: GNSS REflectometry, radio occultation, and scatterometry onboard the international space station. IEEE J. Sel. Top. Appl. Earth Obs. Remote Sens. 9, 4552–4581. doi: 10.1109/JSTARS.2016.2614428
Wiese, A., Staneva, J., Schultz-Stellenfleth, J., Behrens, A., Fenoglio-Marc, L., and Bidlot, J. R. (2018). Synergy between satellite observations and model simulations during extreme events. Ocean Sci. 14, 1503–1521. doi: 10.5194/os-14-1503-2018
Woodworth, P. L., Hunter, J. R., Marcos, M., Caldwell, P., Menéndez, M., and Haigh, I. (2016). Towards a global higher-frequency sea level data set. Geosci. Data J. 3, 50–59. doi: 10.1002/gdj3.42
Woodworth, P. L., Melet, A., Marcos, M., Ray, R. D., Wöppelmann, G., Sasaki, Y. N., et al. (2019). Forcing factors affecting sea level changes at the coast. Surv. Geophys. doi: 10.1007/s10712-019-09531-1
Woodworth, P. L., Melissa, M., and Roland, G. W. (2011). Evidence for century-timescale acceleration in mean sea levels and for recent changes in extreme sea levels. Surv. Geophys. 32, 603–618. doi: 10.1007/978-94-007-2063-3_18
Woodworth, P. L., Wöppelmann, G., Marcos, M., Gravelle, M., and Bingley, R. M. (2017). Why we must tie satellite positioning to tide gauge data. Eos 98, 13–15. doi: 10.1029/2017EO064037
Wöppelmann, G., Cozannet, G. L., Michele, M., Raucoules, D., Cazenave, A., Garcin, M., et al. (2013). Is land subsidence increasing the exposure to sea level rise in Alexandria, Egypt? Geophys. Res. Lett. 40, 2953–2957. doi: 10.1002/grl.50568
Wöppelmann, G., and Marcos, M. (2016). Vertical land motion as a key to understanding sea level change and variability. Rev. Geophys. 54, 64–92. doi: 10.1002/2015RG000502
Wöppelmann, G., Martin-Miguez, B., Bouin, M. N., and Altamimi, Z. (2007). Geocentric sea-level trend estimates from GPS analyses at relevant tide gauges world-wide. Glob. Planet. Change 57, 396–406. doi: 10.1016/j.gloplacha.2007.02.002
Xu, X. Y., Birol, F., and Cazenave, A. (2018). Evaluation of coastal sea level offshore Hong Kong from Jason-2 altimetry. Remote Sens. 10:282. doi: 10.3390/rs10020282
Yang, L., Lin, M., Liu, Q., and Pan, D. (2012). A coastal altimetry retracking strategy based on waveform classification and sub-waveform extraction. Int. J. Remote Sens. 33, 7806–7819. doi: 10.1080/01431161.2012.701350
Young, I. R., Zieger, S., and Babanin, A. V. (2011). Global trends in wind speed and wave height. Science 332, 701–702. doi: 10.1038/ngeo2262
Zavorotny, V. U., and Voronovich, A. G. (2000). Scattering of GPS signals from the ocean with wind remote sensing application. IEEE Trans. Geosci. Remote Sens. 38, 951–964. doi: 10.1109/36.841977
Keywords: SAR/Delay-Doppler Radar Altimetry, retracking, coastal zone, sea level, coastal modeling, storm surge, hazards, Reflectometry Wideband Signals of Opportunity (GNSS-R/W-SoOp)
Citation: Benveniste J, Cazenave A, Vignudelli S, Fenoglio-Marc L, Shah R, Almar R, Andersen O, Birol F, Bonnefond P, Bouffard J, Calafat F, Cardellach E, Cipollini P, Le Cozannet G, Dufau C, Fernandes MJ, Frappart F, Garrison J, Gommenginger C, Han G, Høyer JL, Kourafalou V, Leuliette E, Li Z, Loisel H, Madsen KS, Marcos M, Melet A, Meyssignac B, Pascual A, Passaro M, Ribó S, Scharroo R, Song YT, Speich S, Wilkin J, Woodworth P and Wöppelmann G (2019) Requirements for a Coastal Hazards Observing System. Front. Mar. Sci. 6:348. doi: 10.3389/fmars.2019.00348
Received: 19 November 2018; Accepted: 06 June 2019;
Published: 17 July 2019.
Edited by:
Fei Chai, Second Institute of Oceanography, ChinaReviewed by:
Jinyu Sheng, Dalhousie University, CanadaRui Caldeira, Agência Regional para o Desenvolvimento da Investigação Tecnologia e Inovação (ARDITI), Portugal
Copyright © 2019 Benveniste, Cazenave, Vignudelli, Fenoglio-Marc, Shah, Almar, Andersen, Birol, Bonnefond, Bouffard, Calafat, Cardellach, Cipollini, Le Cozannet, Dufau, Fernandes, Frappart, Garrison, Gommenginger, Han, Høyer, Kourafalou, Leuliette, Li, Loisel, Madsen, Marcos, Melet, Meyssignac, Pascual, Passaro, Ribó, Scharroo, Song, Speich, Wilkin, Woodworth and Wöppelmann. This is an open-access article distributed under the terms of the Creative Commons Attribution License (CC BY). The use, distribution or reproduction in other forums is permitted, provided the original author(s) and the copyright owner(s) are credited and that the original publication in this journal is cited, in accordance with accepted academic practice. No use, distribution or reproduction is permitted which does not comply with these terms.
*Correspondence: Jérôme Benveniste, SmVyb21lLkJlbnZlbmlzdGVAZXNhLmludA==