- 1State Key Laboratory of Marine Environmental Science and College of Ocean and Earth Sciences, Xiamen University, Xiamen, China
- 2School of Biological Sciences, Monash University, Clayton, VIC, Australia
- 3Department of Biology, University of Erlangen-Nuremberg, Erlangen, Germany
- 4School of Biological and Marine Sciences, University of Plymouth, Plymouth, United Kingdom
- 5Shimoda Marine Research Center, University of Tsukuba, Shimoda, Japan
- 6Marine Environmental Biology, Department of Biological Sciences, University of Southern California, Los Angeles, CA, United States
The oceans take up over 1 million tons of anthropogenic CO2 per hour, increasing dissolved pCO2 and decreasing seawater pH in a process called ocean acidification (OA). At the same time greenhouse warming of the surface ocean results in enhanced stratification and shoaling of upper mixed layers, exposing photosynthetic organisms dwelling there to increased visible and UV radiation as well as to a decreased nutrient supply. In addition, ocean warming and anthropogenic eutrophication reduce the concentration of dissolved O2 in seawater, contributing to the spread of hypoxic zones. All of these global changes interact to affect marine primary producers. Such interactions have been documented, but to a much smaller extent compared to the responses to each single driver. The combined effects could be synergistic, neutral, or antagonistic depending on species or the physiological processes involved as well as experimental setups. For most calcifying algae, the combined impacts of acidification, solar UV, and/or elevated temperature clearly reduce their calcification; for diatoms, elevated CO2 and light levels interact to enhance their growth at low levels of sunlight but inhibit it at high levels. For most photosynthetic nitrogen fixers (diazotrophs), acidification associated with elevated CO2 may enhance their N2 fixation activity, but interactions with other environmental variables such as trace metal availability may neutralize or even reverse these effects. Macroalgae, on the other hand, either as juveniles or adults, appear to benefit from elevated CO2 with enhanced growth rates and tolerance to lowered pH. There has been little documentation of deoxygenation effects on primary producers, although theoretically elevated CO2 and decreased O2 concentrations could selectively enhance carboxylation over oxygenation catalyzed by ribulose-1,5-bisphosphate carboxylase/oxygenase and thereby benefit autotrophs. Overall, most ocean-based global change biology studies have used single and/or double stressors in laboratory tests. This overview examines the combined effects of OA with other features such as warming, solar UV radiation, and deoxygenation, focusing on primary producers.
Introduction
Fossil fuel burning, tropical deforestation, and altered land use have caused the atmospheric CO2 concentration to rise from 280 ppmv before the Industrial Revolution to 409 ppmv in 2018. These increases in atmospheric CO2 of about 0.5% per year1 result in global warming and, after dissolving in surface seawater, also cause ocean acidification (OA). Ocean warming induces stratification and shoaling of the upper mixed layer (UML). This hinders the injection of nutrients from deeper layers and also causes the organisms dwelling in the UML to be exposed to increased daily exposures of PAR and UV radiation (Gao et al., 2012a; Hutchins and Fu, 2017; Figure 1). O2 solubility in seawater decreases due to increasing temperatures, leading to global ocean deoxygenation and contributing to the expansion of anoxic zones. Intensified stratification also results in deoxygenation of deeper water due to lack of ventilation, and human nutrient over-enrichment can deplete dissolved oxygen in coastal regions by promoting excessive levels of primary production and consequent elevated bacterial respiration (Schmidtko et al., 2017; Breitburg et al., 2018).
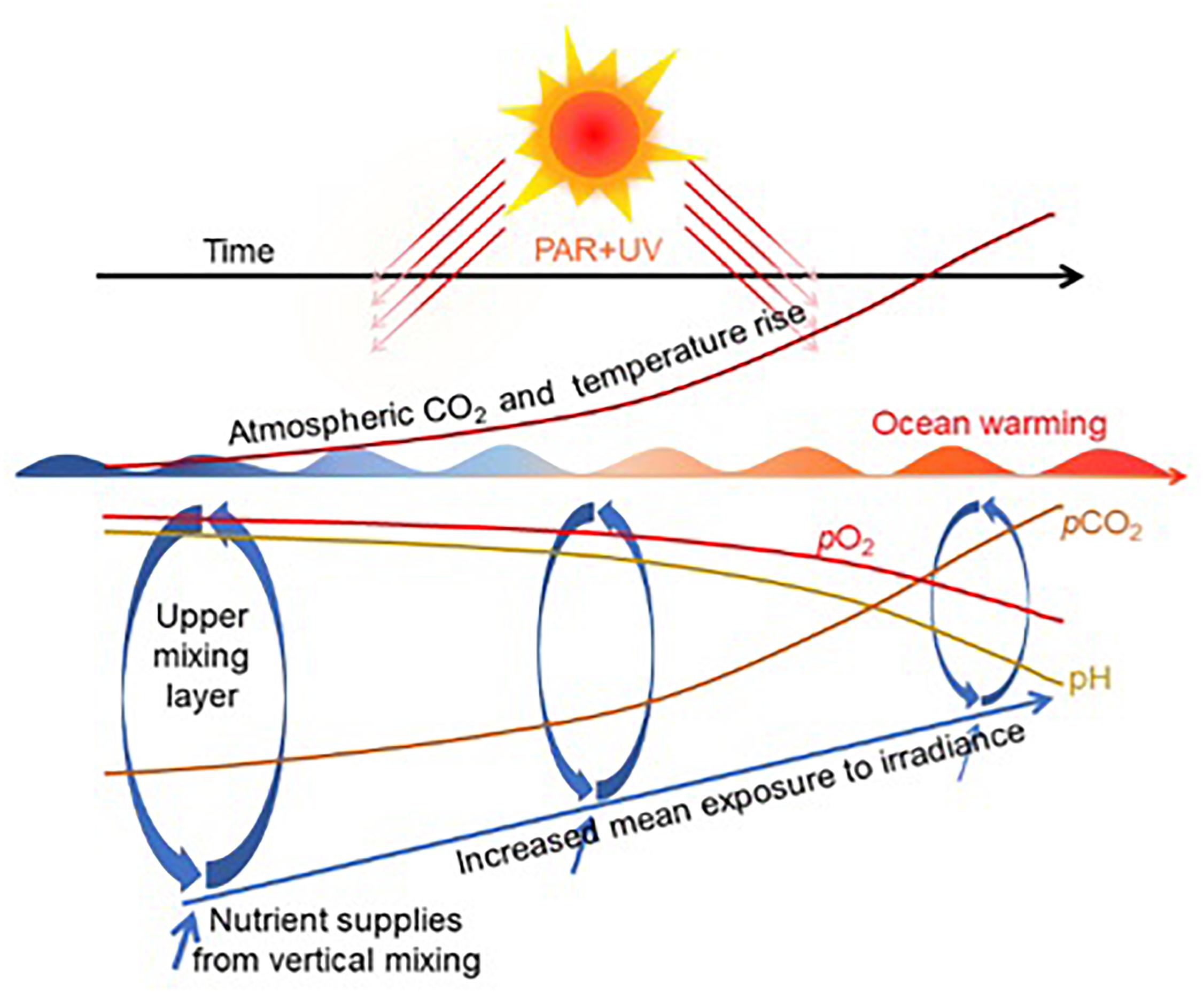
Figure 1. Ocean acidification, warming, and deoxygenation associated with increasing atmospheric CO2 rise. Shoaling of the upper mixed layer (UML) due to warming exposes organisms dwelling there to higher levels of solar radiation [redrawn based on Gao et al. (2012a) and Hutchins and Fu (2017)].
Most investigations into the biological and ecological effects of OA have been based on experiments using single species under controlled conditions, and data on the effects of OA on complex communities in natural environments are relatively limited (Riebesell and Gattuso, 2015; Boyd et al., 2018), although experiments at volcanic seeps and using mesocosms have shown that increased pCO2 benefits some algae more than others and that lower carbonate saturation states harm some algae more than others. So while most benthic algae are tolerant of OA conditions, changes in carbonate chemistry cause large changes in algal community composition and ecosystem function (Hofmann et al., 2012; Hall-Spencer and Harvey, 2019). The combined effects of OA with other drivers, such as warming, increased UV exposure or deoxygenation, are expected to alter marine communities with effects that differ regionally. Further research is needed to plan for the risks associated with the ways in which OA is interacting with warming, UV radiation, and deoxygenation (Boyd et al., 2018).
Marine photosynthetic organisms account for about half of global photosynthetic carbon fixation (Falkowski and Raven, 2013). Across most of the oceans, the dominant photoautotrophs are mainly microalgae and cyanobacteria, with macroalgae and seagrasses making a higher proportional contribution in coastal environments. These organisms are influenced by both increasing pCO2 and declining pH with ongoing OA. While increased CO2 availability can potentially stimulate photosynthesis, stress due to the associated pH drop may be deleterious. This is especially a problem during night in the absence of photosynthetic CO2 removal and with respiratory CO2 release, which may lead to more pronounced impacts of acidification during the night period.
While there are many reports in the literature on the responses of primary producers to OA [see recent reviews by Hutchins and Fu (2017) and Boyd et al. (2018) and references therein], contradictory results have often been documented in relation to different phytoplankton species or communities (Gao and Campbell, 2014; Hong et al., 2017). In macroalgae, most studies show algal tolerance of diel pH fluctuations and benefits from rising pCO2 (Gao et al., 1991, 1993; Cornwall et al., 2012, 2015); in calcifying micro- and macro-algae, it is widely accepted that OA reduces their calcification (Gao et al., 1993; Riebesell et al., 2000; Feng et al., 2008; Gao et al., 2009; Gao and Zheng, 2010; Sinutok et al., 2011). Nevertheless, changes in temperature, light, UV, and nutrient availability are known to modulate the responses of photosynthetic organisms to OA. In this review, we first briefly consider the individual effects of major components of global climate changes (OA, warming, etc.) and then go on to focus on recent advances in our understanding of the effects of OA when combined with other factors, including results from different experimental protocols or algae from different biogeographic regions, and analyze underlining processes and/or mechanisms with special reference to interactive effects of OA with warming, UV, nutrient availability, and dissolved O2 levels.
Ocean Acidification
Since the Industrial Revolution the ocean has been absorbing anthropogenic CO2 emissions, and this absorption is currently running at an average rate of over 1 million tons per hour (Sabine et al., 2004), a process that leads to OA. This alters marine carbonate chemistry, changing the concentration ratios of different inorganic carbon species (CO2, , ) and reducing the saturation state of CaCO3 (Ω).
where Kc is the product of [Ca2+] × [] when the CaCO3 solution is saturated.
Since the Ca2+ concentration of seawater is relatively stable (about 10 mM), Ω mainly depends on the concentration of , as shown in the above equation. Increased dissolution of CO2 into seawater results in increased concentrations of dissolved CO2, , and H+ and decreased concentration of , leading to a decrease in the saturation state of CaCO3. The concentration of varies in different regions, depending mainly on temperature and salinity; for instance, the concentration of in polar waters is only about 41% of that in tropical waters, with the former decreasing faster than the latter under the influence of OA (Orr et al., 2005). In the geological past, mass extinctions of marine life have been associated with OA events, and the contemporary seawater pH and carbonate saturation state are declining faster than it has been in about the last 300 million years (Hönisch et al., 2012; Garbelli et al., 2017). Therefore, OA has been ranked as a major research priority in the NOAA Ocean Exploration 2020 report2.
Ocean acidification is known to reduce calcification of many calcifying organisms. In coralline algae, elevated pCO2 reduces calcification of Corallina pilulifera due to lowered pH and decreased concentration of carbonate (Gao et al., 1993). This species exhibits higher calcification rates during daytime than during the night period and increases its calcification with increased dissolved inorganic carbon concentration when pH is maintained constant (Gao et al., 1993). In contrast, another coralline alga, Jana rubens, when transplanted to lower pH site near a CO2 seep, showed much reduced photochemical efficiency, implying its calcification might be reduced due to reduced photosynthetic energy supply, though the short-term exposure did not bring about significant change in calcification (Porzio et al., 2018). It is clear that OA causes a decrease in competitiveness and fitness for coralline algae compared to brown and turf algae.
Ocean acidification can reduce the content of biogenic silica in diatoms (Milligan et al., 2004; Tatters et al., 2012; Xu et al., 2014), although this response to high CO2 does not appear to be shared by all diatoms (Qu et al., 2018; Li et al., 2019). Cellular silicification is known to influence predation rates by zooplankton as well as the number of fecal pellets (Liu et al., 2016). Acidification thus might reduce the settling of particulate organic carbon (POC) by lowering diatom silicification and density. With increasing pCO2 and acidification, surface diatom abundance in the oligotrophic South China Sea decreases, resulting in decreased primary productivity (Gao et al., 2012b). On the other hand, under nutrient replete conditions, growth of diatoms tends to be enhanced (Wu et al., 2014). In coastal eutrophic water, increased partial pressure of CO2 increased primary production of phytoplankton assemblages dominated by diatoms in a mesocosm study (Huang et al., 2018).
Different diatom species may have entirely different responses to OA, mostly due to differences in species or phenotypes. Under OA conditions, the coastal diatom Thalassiosira weissflogii shows a faster particulate carbon production rate, whereas a pelagic species, Thalassiosira oceanica, grows slower, implying that under diel pH fluctuations the large coastal diatom appears to be more tolerant of the pH decline (Li et al., 2016). While there are species-specific responses to OA, diatoms with lower CCM efficiencies showed more pronounced responses to OA in terms of growth rate (Shi et al., 2019).
Natural gradients of CO2 at volcanic seeps show that a reduction in mean seawater pH from 8.1 to 7.8 results in around a 30% reduction in animal biodiversity compared with only a 5% reduction in algal diversity, although the algal communities are completely changed due to dissolution of calcified algae and shellfish (Hall-Spencer et al., 2008; Fabricius et al., 2011; Agostini et al., 2018). Ecological impacts seen at CO2 seeps contrast to those caused by OA events in the geological past when the species and communities of marine organisms were unlike those that we see today (Hönisch et al., 2012).
Seawater uptake of CO2 at volcanic seeps changes seawater chemistry in a process that is a natural analog for anthropogenic OA (González-Delgado and Hernández, 2018). The seeps create steep gradients in seawater carbonate chemistry with falling pH, falling carbonate, and increasing bicarbonate levels with increasing proximity to the seabed release of CO2 (Linares et al., 2015). The biological effects of this acidification are found at seep systems worldwide, allowing investigations into the underlying mechanisms that drive community level responses (Cornwall et al., 2017; Connell et al., 2018). As phytoplankton drift into these seep systems calcareous coccolithophores are damaged and dissolve, but this is a shock response to rapid changes in seawater carbonate chemistry (Ziveri et al., 2014). The microphytobenthos at shallow-water seeps, on the other hand, is exposed to long-term acidification over multiple generations. Diatom- and cyanobacteria-dominated biofilms proliferate at shallow-water marine CO2 seeps. Broad scale analysis of diversity has shown that microphytobenthic communities in high CO2 water are substantially modified compared with ambient conditions, with a stimulation of diatom growth on sand, mud, bedrock, glass, and plastic substrata (Johnson et al., 2015).
Marine volcanic seeps reveal ecological responses to moderate increases in pCO2 that retain natural pH variability (Foo et al., 2018). They show the consequences of long-term exposure to acidified waters as well as the implications of more frequent low pH excursions for a wide range of organisms (Agostini et al., 2018). At Mediterranean CO2 seeps, invasive macroalgal taxa such as Sargassum, Caulerpa, and Asparagopsis species thrive, whereas calcareous macroalgae are sensitive to acidification (Figure 2). This causes algal community shifts, fleshy algal dominance, and a loss of coastal biodiversity (Hall-Spencer et al., 2008). Although most macroalgae are resilient to the effects of OA, with only around a 5% loss in species diversity at levels projected under IPCC RCP 8.5, shifts in algal community composition greatly alter habitats (Porzio et al., 2011; Enochs et al., 2015). Increased availability of bicarbonate and pCO2 stimulates primary production in seagrasses and in some algae but not others, depending on their physiology (Cornwall et al., 2017). In areas sheltered from wave action, this increases carbon fixation and the standing stock of seagrasses and large phaeophytes (Linares et al., 2015; Sunday et al., 2017). However, acidification lowers ecosystem resilience in more exposed conditions, such that only microalgal biofilms and weedy small turf algae persist at high CO2 levels after storms (Hall-Spencer and Harvey, 2019).
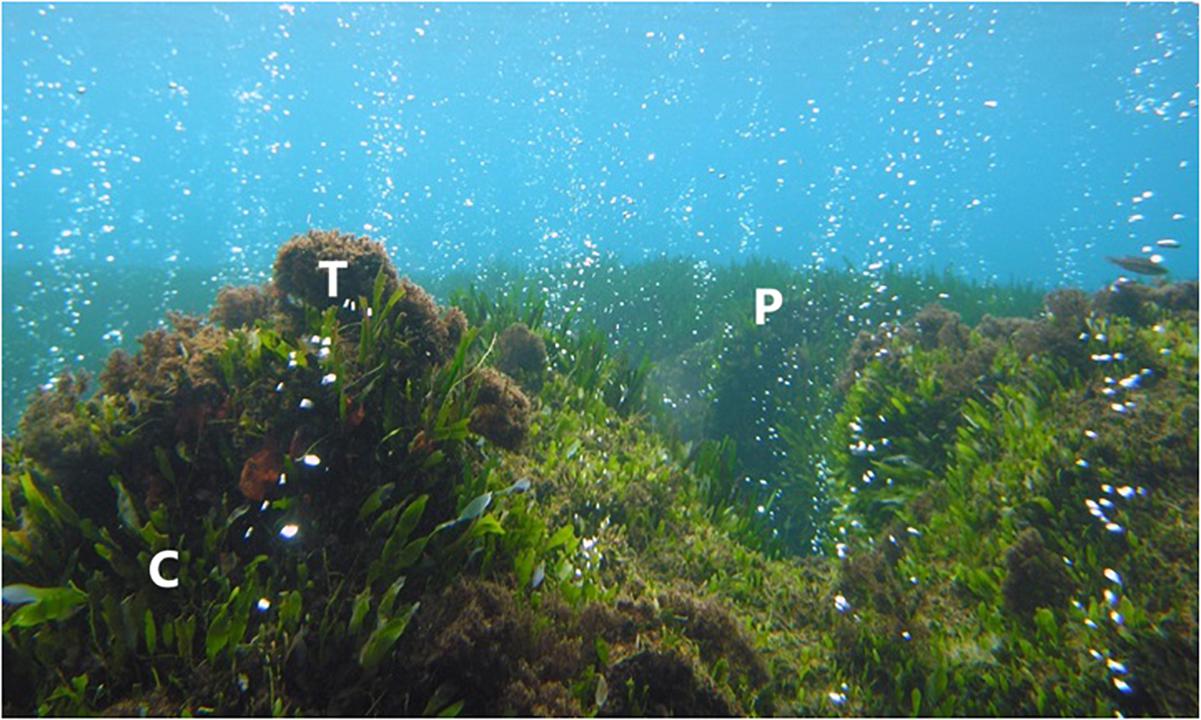
Figure 2. Bubbles of CO2 rising from the seafloor at a depth of 1 m at a volcanic seep off Ischia Island, Italy (40°43.84′ N, 13°57.08′ E). At a mean pH of 7.8 turf algae (T), seagrass Posidonia oceanica (P) and green seaweed Caulerpa prolifera (C) cover the seabed. Epiphytic and epilithic coralline algae are scarce, yet they are abundant 300 m away from the CO2 seep where the seawater returns to the normal mean of pH 8.1. (Photo taken by J. Hall-Spencer).
Studies in areas with naturally high levels of CO2 show that coastal ecosystems are altered especially if elevated DIC is combined with high levels of nutrients (Celis-Plá et al., 2017; Rastrick et al., 2018). In upwelling areas such as Namibia and Peru, these acidified waters have productive food webs that support major fisheries. However, if the acidification is strong enough to cause periods of carbonate under-saturation this is detrimental to shellfish fisheries and calcareous biogenic reefs. Very similar patterns are seen in tropical, sub-tropical, and temperate coastal systems, with stimulated diatom growth and opportunistic algal dominance, calcareous biogenic habitat degradation, and loss of biodiversity. This potentially lowers the resilience of coastal habitats to a cluster of other drivers associated with climate change (global warming, sea level rise, increased storminess), increasing the risk of marine regime shifts and the loss of critical ecosystem functions and services, though it should be noted that in some areas, such as the coastal waters of California, upwellings bring elevated levels of nutrients as well as CO2 into surface waters, leading to high levels of primary productivity (Ryther, 1969; Pauly and Christensen, 1995). Modeling suggests enhancement of some coastal upwellings in the future, and also indicates that the ability to predict flow on effects to ecosystems is complicated by the range of contributing factors and scales involved (Xiu et al., 2018).
There are obvious diel and seasonal variations in the chemistry of coastal seawaters (Dai et al., 2009; Wang et al., 2014), and coastal seawater acidification often coincides with hypoxia, which is frequently enhanced after algal blooms (Zhai et al., 2012). For example, in the Bohai Sea off northern China, pH drops by 0.29 units (H+ concentration doubles) during the summer period (Zhai et al., 2012). Nevertheless, it is predicted that by the end of the 21st century OA will cause the average pH of the oceanic surface waters to decrease from the preindustrial level of 8.2 down to 7.8 (H+ concentration rises by 150%) (Gattuso et al., 2015; Guy et al., 2015), endangering marine organisms and ecosystem services (Broadgate et al., 2013).
Ocean Warming
Since the Industrial Revolution, increasing global average temperature has been linearly correlated with atmospheric CO2 concentrations (IPCC, 2013a). Since the 1970s, the oceans have absorbed over 90% of Earth’s heat gain, leading to ocean warming (Gattuso et al., 2015). Ocean warming can be detected to a depth of 1,000 m (Levitus et al., 2000), and during the past century the global ocean surface temperature has increased by about 1°C (Fischetti, 2013). By the end of the 21st century, the average Earth surface temperature is predicted to rise by 2–4 °C (Gattuso et al., 2015). Compared with many other regions in the world, the marginal waters in the East China Sea and the South China Sea have shown a faster rate of temperature increase over the past 50 years (Bao and Ren, 2014; Williams et al., 2016; Cai R. et al., 2017), though polar regions have warmed fastest (Wassmann, 2015). Global warming is predicted to have other consequences such as increasing storm activity that can influence marine biota. For instance, a rise in temperature of 1°C has been shown to increase the number of typhoon events by up to 25% (Bigg and Hanna, 2016). At the same time, the frequency of marine heat waves, prolonged discrete anomalously warm water events (Hobday et al., 2016), has been predicted to increase tens of fold with a 3.5°C rise in sea surface temperature (Frölicher et al., 2018). Marine heat waves are postulated as drivers of ecological disasters such as the triggering of toxic algal blooms along the North American west coast (McCabe et al., 2016).
Temperature is a key factor affecting enzyme activity and metabolism. Metabolic rates usually increase with temperature up to a maximum value and thereafter rapidly decline, exhibiting an energy activation to deactivation transition. Shallow water marine organisms can be exposed to diurnal, seasonal, and current-driven sudden changes in temperature. These changes involve the thermocline, tides, typhoons, and cloud cover as well as the long-term changes due to natural climate cycles and human activities (IPCC, 2013b). Ocean warming affects organism physiology (Pörtner, 2008; Sinclair et al., 2016) and changes biogeographic boundaries, community composition, and phenology (Hutchins and Fu, 2017). In laboratory experiments, increasing temperature below optimal values can increase phytoplankton growth (Fu et al., 2014; Summers et al., 2016; O’Donnell et al., 2017; Jiang et al., 2018). However, on a global scale, warming may decrease primary productivity due to nutrient limitation driven by enhanced stratification of the upper mixing layer (UML); it may also enhance fixed carbon remineralization by heterotrophic microorganisms and so reduce the strength of the ocean carbon sink (Danovaro et al., 2016; Cavan et al., 2019).
Deoxygenation
One additional effect of ocean warming is that it decreases the solubility of oxygen (and gases generally) in seawater. In oceanic and coastal waters the dissolved oxygen content has declined over the past 50 years (Schmidtko et al., 2017; Breitburg et al., 2018). In addition, shoaling of the UML or enhanced ocean stratification reduces ventilation from the surface to deeper layers, further exacerbating the deoxygenation phenomenon. As POC sinks, marine bacteria feed on it, consuming O2 and releasing CO2. This results in an oxygen minimum zone at depths of 500–600 m in the eastern tropical Pacific (Brewer and Peltzer, 2009). Over the past 50 years the depth of a hypoxic layer (<2 mg O2 L-1) in the Pacific Ocean has shoaled from 400 to 300 m, and the dissolved oxygen content has decreased significantly (Whitney et al., 2007) due to sea surface warming (Keeling et al., 2010); and ocean deoxygenation has been recently shown to cause deterioration in a number of processes including biogeochemistry and ecosystem function (Breitburg et al., 2018). In coastal regions deoxygenation is driven mainly by eutrophication, leading to excess oxygen consumption and the development of hypoxia and “dead zones.” However, decreasing solubility of O2 through warming can exacerbate this process.
Most marine organisms need O2 in their metabolic processes. When the dissolved O2 concentration is below a certain value, these organisms suffer from stressed respiration, and hypoxic events may lead to the death of many organisms. For different organisms, the half lethal concentration (LC50) of dissolved O2 is different, and the critical value for one organism might be several times that for others. In typical anoxic zones, the oxygen content is below 2 mg L-1 and the hypoxia is usually associated with high pCO2 and low pH. In coastal ecosystems, the frequency of hypoxia is increasing at a rate of about 5.5% per year due to the interaction between deoxygenation and eutrophication (Vaquer-Sunyer and Duarte, 2008), which is responsible for a faster rate of OA in coastal, compared to oceanic waters (Cai et al., 2011; Breitburg et al., 2018). Eutrophication frequently leads to algal blooms. When these blooms collapse, the algae die, leading to enhanced organic carbon loads, the breakdown of which, through microbial action, contributes to anoxia and in turn renders spawning grounds for fish such as herring untenable. In terms of biogeochemical impacts, deoxygenation promotes denitrification, reducing the concentration of nitrate and affecting the ocean N cycle, primary productivity, and the efficiency of the marine biological carbon pump (Hutchins and Fu, 2017).
Lower oxygen levels would be expected to have the most effect in photoautotrophic species on the ratio of oxygenase to carboxylase activity of the CO2-fixing enzyme ribulose-1,5-bisphosphate carboxylase/oxygenase (Rubisco). Diminished oxygen concentrations favor the carboxylase activity of Rubisco and diminish photorespiratory activity. However, in practice low oxygen concentrations, i.e., below air-equilibrium levels, appear to have little effect on physiology and growth of algae, though levels above air-equilibrium can be damaging (Black et al., 1976; Drechsler and Beer, 1991; Beer et al., 2000; Kitaya et al., 2005, 2008; Kliphuis et al., 2011; Raso et al., 2012; Haas et al., 2014). Kim et al. (2018) showed a stimulation of photosynthetic rates of the seagrass Zostera marina at sub-saturating, but not saturating, light intensities when oxygen concentrations dropped from 231 to 8 μmol O2 L-1. At the same time respiration rates dropped fivefold at the lower oxygen level. In part, the lack of effect of low oxygen is likely to be due to the presence in most algae of active CO2 concentrating mechanisms (CCMs), which maintain a sufficiently high CO2:O2 ratio at the active site of Rubisco to minimize oxygenase activity and photorespiration (Giordano et al., 2005). Of the other oxygen consuming reactions found in algae and cyanobacteria, only the Mehler reaction and glycolate oxidase (in those organisms that possess that enzyme rather than glycolate dehydrogenase) have a K0.5 O2 high enough to be affected by oxygen concentrations around air equilibrium (Beardall et al., 2003; Raven and Beardall, 2005). Cytochrome oxidase for example has a K0.5 O2 of ≤2 μmol O2 L-1 (see Table 3.2 of Raven and Beardall, 2005).
However, to date there have been no studies on the interaction between low oxygen concentration and other stressors associated with ocean global changes. In the study of Kim et al. (2018) low oxygen resulted in a 2.8-fold downregulation of γ-carbonic anhydrase (γ-CA) genes, which they suggest may play a role in bicarbonate transport rather than as a standard CA. It might be expected that elevated CO2 (OA) and diminished O2 concentrations lead to further downregulation, though this has yet to be tested. Anoxia also stimulates nitrogen fixation in the marine cyanobacterium Trichodesmium (Staal et al., 2007), with aerobic conditions leading to N2 fixation being limited by the availability of reducing equivalents, a result interpreted by Staal et al. (2007) as suggesting a competition for electrons between nitrogenase and respiration when oxygen is present.
Stratification and Increased Exposure to UV
It is commonly known that the UML is much shallower during summer than in winter. For example, in pelagic areas of the South China Sea, the UML can be over 30 m shallower in summer than in winter (Liu et al., 2007). In addition to less wind and storm mixing than in winter, the higher temperatures in summer are mainly responsible for the enhanced stratification.
On a global scale, ocean warming enhances stratification in the upper oceans due to reduced advective mixing, leading to shoaling of the UML. Such stratification hinders the upward transport of nutrients from deeper layers. Consequently, phytoplankton cells are exposed to a reduced availability of nutrients, provided that the contribution from other sources such as nitrogen fixation or deposition into the oceans from run-off and atmospheric sources is unaltered by global climate changes. Phytoplankton cells dwelling in a shallower UML are exposed to higher doses of UV radiation since they cannot actively move downward to deeper waters (Jin et al., 2013). Since the vertical migration path for cells within a shallower UML is shorter, they experience much higher daytime average or integrated levels of solar radiation [see reviews by Riebesell and Tortell (2011) and by Gao et al. (2012b)]. Since UV irradiances can penetrate as deep as 80 m in the pelagic ocean, down to the middle or lower layers of the euphotic zone, phytoplankton cells are inevitably exposed to increased levels of UV radiation due to enhanced stratification [see the review by Gao et al. (2012b) and literature cited therein].
UV radiation is divided into UV-A (315–400 nm), UV-B (280–315 nm), and UV-C (<280 nm). The stratospheric ozone layer completely absorbs UV-C (the most biologically harmful band). The major portion of the UV-B is also absorbed by ozone, mainly in the stratosphere. Only a small fraction of UV-B reaches the Earth’s surface through the stratosphere and the upper troposphere. On the other hand, most of the UV-A and visible light reaches the surface of the Earth. In the subtropics, UV-B radiation reaching the sea surface usually accounts for <1% of the total sunlight energy; UV-A and visible light (PAR: 400–700 nm) account for 7–8 and 40–50%, respectively, with infrared radiation making up the balance. At noon, the UV-B:UV-A:PAR ratio is about 0.5:16:100. The UV-B:PAR ratio is highest at noon, while the UV-A:PAR ratio remains unchanged during the day (Gao, 2018). Solar radiation is reduced by moisture in the atmosphere, sea surface reflectivity, and inorganic and organic substances in the water, and has obvious latitudinal, diel, and seasonal dependencies. UV-B radiation increases by 2% for each 1% decrease in stratospheric ozone concentration (Kerr and McElroy, 1993). Ozone destruction has been curbed, with stratospheric ozone expected to return to pre-1980 levels by the mid-21st century (Plummer et al., 2010). However, global warming, the presence of trace gases (Ossó et al., 2011) and changes in atmospheric circulation might increase UV-B level at low latitudes by 2–3% (Williamson et al., 2014).
Many oceanographic biological observations of primary productivity have neglected the influence of UV radiation because of traditional recommendations for use of transparent vessels, which, unless made of quartz and UV-transparent materials, are mainly glass or polycarbonate bottles that are opaque to UV wavelengths. With progressive ocean climate changes, more attention should be given to the interactive impacts of UV with other global environmental change drivers (Häder and Gao, 2015).
Even though the irradiance of UV-B is only a few percent (in tropical and subtropical areas, <1%) of the total solar UV reaching the Earth’s surface, it is the most deleterious wavelength band encountered by photosynthetic organisms dwelling in the photic zone. UV-B can damage proteins, lipids, and other bioactive components of the cells (Ganapathy et al., 2017). One of the main targets is photosynthetic electron transport, since UV-B damages the D1 protein in photosystem II, reducing the photosynthetic capacity (García-Gómez et al., 2016). This damage is repaired by removing the faulty protein and replacing it with a newly synthesized molecule. This enzymatic process is favored by increased temperature (Gao et al., 2008). When moving actively or passively in the UML, cells are affected most near the surface and use the time in deeper water closer to the thermocline for repair (Helbling et al., 2003). Another important target of solar UV-B is DNA (Gao et al., 2008; Rajneesh et al., 2018). UV-B radiation mainly induces the formation of cyclobutane pyrimidine dimers, leading to mutations and cell death if these are not removed by a photolyase which uses the energy of UV-A and blue light to split the dimers (García-Gómez et al., 2014).
Many prokaryotic and eukaryotic phytoplankton as well as macroalgae synthesize UV-absorbing substances, such as scytonemin (only cyanobacteria) and mycosporine-like amino acids (MAAs), which convert the energy of short-wavelength UV radiation into heat before it can hit sensitive targets (Singh et al., 2008). Another mechanism of damage by UV radiation is the production of reactive oxygen species (ROS) such as peroxides, superoxide, hydroxyl radicals, and singlet oxygen which can oxidatively damage proteins, lipids, and other cell structures (Williamson et al., 2019). ROS can be detoxified either enzymatically (e.g., by superoxide dismutase, catalase, peroxidase) or non-enzymatically by antioxidants (e.g., thiols, ascorbic acid, glutathione, carotenoids) (Martínez, 2007; Goiris et al., 2015).
In addition to the negative effects of UV radiation, it is known that moderate levels of UVA radiation enhance primary production by enhancing photosynthetic light use efficiency, as shown in the photosynthetic rate vs. PAR curves determined in the presence and absence of UV radiation (Gao et al., 2007a). When PAR was filtered out, UVA stimulates carbon fixation of phytoplankton assemblages in coastal waters of the South China Sea, and addition of UV-B reduces the carbon fixation (Gao et al., 2007a). UV-induced inhibition of photosynthetic carbon fixation in surface seawater increases with distance from the coast toward the open ocean, probably due to differences in attenuation coefficient, phytoplankton community structure, and nutrient availability as well as mixing rate (Tedetti and Sempéré, 2006; Li et al., 2011). Faster mixing reduces UV-induced photosynthetic inhibition (Helbling et al., 2003; Jin et al., 2013).
Interactions Between Factors Associated With Climate Change
OA and Solar Radiation (PAR and UVR)
Changing PAR levels modulate diatom responses to OA. For instance, when three diatom species were grown under different levels of sunlight, lowered pH with elevated CO2 stimulated growth under low to moderate levels of light, but inhibited it under high levels (Gao et al., 2012a; Figure 3). However, such a reversed response to OA with increasing light levels was not found in coccolithophores, which showed enhanced growth rates (with reduced calcification) under the elevated pCO2 under all tested PAR levels (Jin et al., 2017; Figure 3). Likewise, Feng et al. (2008) found that the interactive effects of high light and elevated CO2 on a subtropical coccolithophore increased growth rates but strongly decreased calcification.
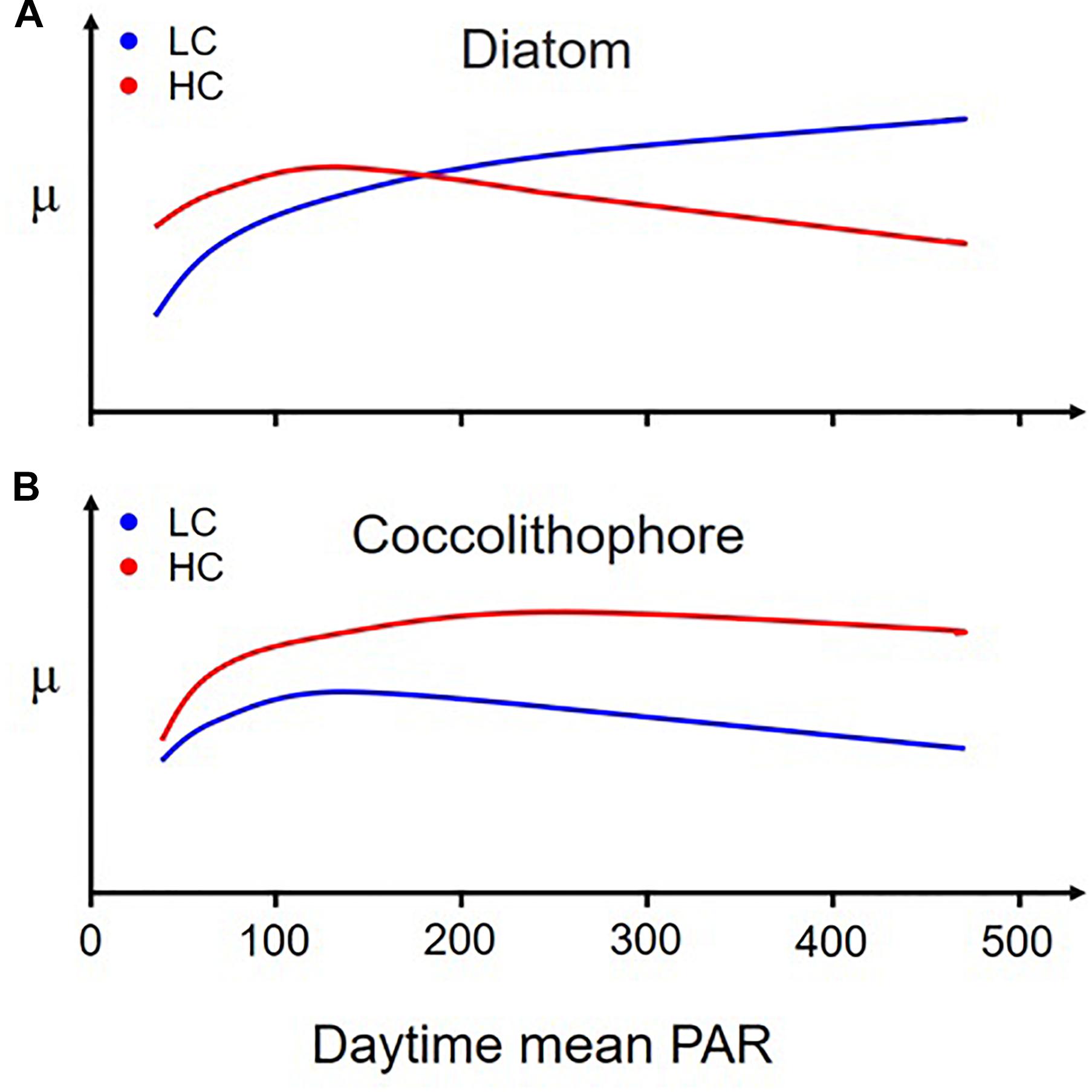
Figure 3. A schematic representation of the differing relationship between sunlight intensity and the growth rates of diatoms (A) and coccolithophores (B) grown at low (390 μatm, blue lines) and high (1,000 μatm, red lines) CO2 concentrations, respectively. Note that elevated CO2 stimulates growth in diatoms at low light but inhibits them at high light levels. However, this is not the case in E. huxleyi, which grows faster under elevated CO2 regardless of sunlight levels, though its calcification decreases. [Derived from Gao et al. (2012b) for panel “A” and from Jin et al. (2017) for panel “B”].
Solar UV radiation may interact with OA to affect primary producers. To date, most OA effects have been observed under UV-free light conditions, either under indoor artificial light or under solar radiation with vessels that are opaque to UV irradiances. Therefore, relatively little information is available on this aspect. OA exacerbates the impact of UV on calcification in the coccolithophore Emiliania huxleyi (Gao et al., 2009) and on calcification of a coralline alga (Gao and Zheng, 2010). Decreased thickness of the calcified layer increased cellular exposure to UV radiation and consequently led to enhanced photoinhibition of photosynthesis by UV (Gao et al., 2009; Gao and Zheng, 2010). Elevated CO2 increased the sensitivity of freshwater lake phytoplankton populations to UV-B (Sobrino et al., 2009) and also exacerbated the harmful effect of UVR on PSII function in the marine diatom T. weissflogii through reducing the PsbD removal rate and the ratio of Rubisco to PsbA during UVR exposure (Gao et al., 2018c). In contrast, the marine microalga Nannochloris atomus increased its growth in response to elevated CO2 with an insignificant photosynthetic response to UVR (Sobrino et al., 2005). Furthermore, the rETRmax of Corallina officinalis was stimulated by elevated CO2, and exposure to UVR led to further stimulation (Yildiz et al., 2013). The divergence between these findings may be due to the differences in species or light intensity.
Interactions Between OA Effects and Nitrogen Fixation and With the Availability of Other Nutrients
Marine diazotrophs, N2-fixing cyanobacteria such as Trichodesmium spp., play an important role in remediating N-limitation in oligotrophic waters (Capone and Carpenter, 1982; Sohm et al., 2011; Hutchins et al., 2015). Trichodesmium has a high abundance in the oligotrophic China Sea, frequently forming blooms in the East and South China Seas (Chen et al., 2014). Because of the N2 fixation capacity of Trichodesmium and subsequent microbial cycles, biologically available nutrients can be replenished, which promotes growth of phytoplankton, thus enhancing primary and secondary productivities, playing an important role in driving the marine biological CO2 pump (Hutchins and Fu, 2017).
Numerous studies have demonstrated that N2 fixation increases under elevated CO2 levels in nutrient-replete cultures of both the filamentous species Trichodesmium (Barcelos e Ramos et al., 2007; Hutchins et al., 2007, 2013, 2015; Kranz et al., 2009, 2010; Levitan et al., 2010a,b; Eichner et al., 2014; Walworth et al., 2016a,b) and the unicellular Crocosphaera (Fu et al., 2008; Garcia et al., 2013a,b). However, there are a few studies showing that OA decreased N2 fixation of the diazotroph (Hong et al., 2017; Luo et al., 2019). While there are disputes over the positive and negative effects of elevated CO2 concentrations on N2 fixation in Trichodesmium (Hutchins et al., 2017), it is generally agreed that severe iron (Fe) limitation cancels out (or even reverses) the positive effects of CO2 on cyanobacterial diazotrophs (Fu et al., 2008; Shi et al., 2012; Walworth et al., 2016a; Hong et al., 2017). In contrast, growth limitation by phosphorus (P) appears to operate independently, regardless of any effects of changing pCO2 (Hutchins et al., 2007; Garcia et al., 2013b; Walworth et al., 2016a). A nearly decade-long experimental evolution study with Trichodesmium selected under two CO2 levels yielded an unexpected result: the increases in growth and N2 fixation commonly observed under exposure to short-term high CO2 conditions became irreversible after selection under OA conditions for several years. Thus, high CO2-adapted cultures retained their increased N2 fixation and growth rates indefinitely, even when moved back to lower pCO2 levels (Hutchins et al., 2015; Walworth et al., 2016a). This constitutive upregulation occurs through the process of “genetic assimilation,” whereby following extended natural selection, traits such as elevated nitrogen fixation rates transition from being physiologically plastic responses to being genetically fixed ones (Walworth et al., 2016b).
Nevertheless, OA can sometimes show different effects on other nitrogen fixing taxa. For heterocystous cyanobacteria, studies showed that OA can either promote nitrogen fixation (Wannicke et al., 2012) or inhibit it (Czerny et al., 2009). Ship-board studies during research cruises have shown that OA treatment can either stimulate N2 fixation (Lomas et al., 2012), did not bring any significant effects, or negatively affected cyanobacterial N2 fixation (Böttjer et al., 2014; Gradoville et al., 2014). These field experiments are difficult to interpret though, as N2-fixing cyanobacteria such as Trichodesmium are famous for surviving poorly in shipboard incubations, and Gradoville et al. (2014) were unable to demonstrate consistent limitation of N2 fixation not only by CO2 but also by any other potentially limiting resources, including iron and P. There are inherent differences in CO2 affinities and growth responses among strains and species of N2-fixing cyanobacteria (Hutchins et al., 2013), and different culturing or light conditions can lead to discrepancies in experimental results. Nevertheless, it is clear that species-specific differences in responses of N2-fixing cyanobacteria to OA may lead to changes in community structure and diversity of marine diazotrophs in future oceans (Hutchins et al., 2013; Gradoville et al., 2014).
UV radiation has been shown to adversely affect cyanobacterial N2 fixation, including inhibiting development of heterocysts and reducing chlorophyll content in the fresh water N2 fixer Anabaena sp. (Gao et al., 2007b). In the marine diazotroph Trichodesmium, UV radiation reduces rates of photosynthesis, N2 fixation, and growth (Cai X. et al., 2017), though moderate or low levels of UV-A may be stimulating. Another recent study showed that growth, N2 fixation, and CO2 fixation rates of the Trichodesmium strain IMS 101 and Crocosphaera WH0005 were both negatively affected by UV-B exposure (Zhu et al., 2019). This inhibition was much greater for Trichodesmium IMS 101, which fixes N2 during the day, than for Crocosphaera, which fixes N2 only during the night; however, another Trichodesmium isolate (GBR) was much more resistant to UV-B inhibition (Zhu et al., 2019). To the best of our knowledge, however, nothing has been documented on the interactive effects of OA and UV radiation on marine diazotrophs.
OA and Warming
Laboratory studies have shown that combined OA and warming have different ecological and physiological effects on different species. For example, in the picoplanktonic cyanobacterium Synechococcus, warming and OA together promote its growth, but they have no effects on Prochlorococcus (Fu et al., 2007). Similarly, simultaneous OA and warming by 4°C promoted the growth of the diatom Skeletonema (Kremp et al., 2012), but had no obvious effects on Thalassiosira and Chaetoceros (Hyun et al., 2014). Under acidification and warming conditions, the growth rate and calcification of the coccolithophore Coccolithus decreased (Schlüter et al., 2014), and OA and warming together lowered the optimum growth temperature and maximum growth rate of E. huxleyi (Listmann et al., 2016). However, other work shows that warming combined with partial pressures of 20–6,000 μatm CO2 increases the production rate of particulate inorganic carbon and POC of E. huxleyi and Gephyrocapsa oceanica (Sett et al., 2014). In a natural North Atlantic Bloom community, simultaneous OA and warming stimulated coccolithophore growth while at the same time significantly reducing their calcification (Feng et al., 2009).
In general, acidification and warming have usually been found to promote the growth and nitrogen fixation rates of open ocean diazotrophic cyanobacteria such as Trichodesmium and Crocosphaera (Hutchins et al., 2007, 2019; Fu et al., 2014; Hutchins and Fu, 2017). Warming alone also favors the growth of globally important open ocean nitrogen-fixing cyanobacteria taxa, as long as temperatures do not exceed ∼32–33°C. The stimulatory effects of higher temperatures and pCO2 together have been suggested to be roughly additive (Hutchins et al., 2007; Levitan et al., 2010a). Temperatures in the low-latitude tropical oceans are predicted to eventually exceed optimum growth levels in many regions, leading to potential future thermal exclusion of these N2 fixers from core parts of their current biogeographic range (Breitbarth et al., 2007; Thomas et al., 2012; Fu et al., 2014; Jiang et al., 2018).
Ocean acidification and warming are known interactively to affect key physiological performances of macroalgae. For instance, the respiratory coefficient (the rate of change of the respiratory rate as the temperature increases) in Sargassum fusiforme increases under OA conditions, indicating that acidification and warming additively increase the respiratory rate (Zou et al., 2011). Furthermore, warming and OA synergistically enhance reproduction events and shorten the generation span in the “green tide” alga Ulva rigida (Gao et al., 2017, 2018b), suggesting more severe green tides may occur in future oceans.
Research on reef-building coralline algae shows that acidification causes a reduction in their calcification, and warming and acidification together further lower the calcium quality (Martin and Hall-Spencer, 2017). Acidification and warming together have been shown to improve the nitrogen fixation of nitrogen-fixing cyanobacteria (Hutchins et al., 2007). In the Bay of Kiel, warming is believed to drive changes in the phytoplankton community structure and an increase in the zooplankton biomass; under in situ water temperature conditions, the predation rate of zooplankton decreases due to the negative effects of acidification (Paul et al., 2016). For some marine algae, the combined effects of acidification and warming are manifested as acidification changing their capacity to cope with temperature changes and alterations in the range of their optimum survival temperature. For example, the calcification rate of the coral Acropora pulchra is controlled by temperature and acidification (Comeau et al., 2016). For the warming and acidification effects, there may be regional differences due to different biotic populations and physical and chemical environments. This phenomenon needs to be further verified and its mechanisms need to be discussed.
Temperature also influences the responses of algae to UV radiation. We might expect a priori that temperature would exert an effect on net UV-B impacts by affecting enzyme-driven repair mechanisms (which are strongly temperature dependent) more than photochemical damage (since photochemistry is temperature independent). In the model diatom Phaeodactylum tricornutum, elevated temperature increased the repair rate of PSII either under ambient or elevated CO2 levels in the presence of UV-A or UV-B (Li and Gao, 2012). On the other hand, elevated temperature increased inhibition of photochemical quantum yield by UVR in freshwater phytoplankton assemblages from Patagonia in Argentina (Villafane et al., 2013); however, increased temperature in this region helped to counter the magnitude of daytime yield decrease during the onset of a phytoplankton bloom (Helbling et al., 2013).
Recently, it has been suggested that ocean warming would affect the marine biological carbon pump (MBP) and microbial carbon pump (MCP) as well as their interactions (Jiao and Zheng, 2011). However, it has been shown that acidification has no effect on the composition of dissolved organic carbon (DOC) in a plankton ecosystem (Zark et al., 2015), while other findings show that acidification promotes the production of POC (Czerny et al., 2013). Whether acidification and warming will produce combined effects and further affect the composition and lability of DOC and the production of POC in different waters is not yet known. In the oceans, most DOC will be converted to CO2 in the short term through bacterial action; however, some DOC resists bacterial breakdown and survives for a long time (for hundreds or even thousands of years), playing a steady role in the carbon sink (Jiao et al., 2010). Therefore, it is particularly important to understand the carbon sink/source processes in marine organisms and the response of assimilation and dissimilation to acidification and warming. Nevertheless, most of the present research findings on the combined effects of the warming and acidification have been obtained under constant laboratory conditions, which may not reflect natural conditions where factors such as light exposure, temperature, and concentrations of dissolved CO2 can vary even on short time scales. They are still quite controversial, and the combined effects and their mechanisms are still unclear.
Nutrient Availability and Interactions With Other Components of Global Change
With progressive ocean warming, intensified stratification of the UML is expected, and consequently, upward transport of nutrients across the thermocline will be reduced. Therefore, phytoplankton cells dwelling in this layer are expected to be exposed to decreasing availability of nutrients. Levels of nutrients and/or ratios of different nutrients are known to affect algal physiology. Interactions among nutrient availability, warming, and OA are thus to be expected. For instance, in the diatom Thalassiosira pseudonana, nitrate limitation interacts with OA and warming, leading to increased respiration and decreased photosynthetic rates and growth (Li et al., 2018). Likewise, in the large centric diatom Coscinodiscus, OA and warming together reduced growth affinity for nitrate, and so increased nitrate concentrations required for growth (Qu et al., 2018).
In the diatom Chaetoceros didymus, OA had no effect on growth rate under N-replete conditions, but stimulated growth under N-limitation. N-limitation and OA also interacted to increase sinking rates, which were less affected under normal CO2 conditions when N supply was high (Mannfolk, 2016). Toxic microalgae are influenced by interactive effects of elevated CO2 and nutrient supply. Fu et al. (2010) showed that under P-limited conditions, toxin production by Karlodinium veneficum was greatly stimulated by elevated CO2. Tatters et al. (2013) found saxitoxin production by the dinoflagellate Alexandrium was greatly increased by the combination of OA and P limitation, but warming to some extent counteracted these effects. Tatters et al. (2012) reported that elevated CO2 enhanced domoic acid production by the harmful bloom diatom Pseudo-nitzschia fraudulenta under nutrient-limited conditions. In general, it seems that the combination of OA with nutrient limitation could lead to much more toxic and damaging blooms of harmful algae in the future ocean.
In addition to microalgae, coastal eutrophication can also lead to macroalgal blooms, including green tides and golden tides. Ulva-dominated green tides and Sargassum-dominated golden tides have increased worldwide in recent years (Smetacek and Zingone, 2013). Kang and Chung (2017) reported that ammonium enrichment stimulated growth of Ulva pertusa, with further stimulation when combined with OA. Gao et al. (2017, 2018b) showing that the combination of eutrophication, OA, and warming enhanced the settlement, germination, and growth of U. rigida. The combination of phosphate enrichment and OA also enhanced growth rate in the golden tide alga Sargassum muticum (Xu et al., 2017). These findings suggest that the rising trend of macroalgal blooms that occurred in Chinese coastal waters (Liu et al., 2013) may correlate with ocean climate changes. Eutrophication is also likely to interact with other stressors, negatively impacting coral reef systems (Bell et al., 2014).
Nutrient limitation favors smaller-celled organisms such as the picophytoplankton Micromonas and Ostreococcus and nanophytoplankton such as the coccolithophorid E. huxleyi by virtue of their higher surface area to volume ratio. Nutrient supply and concentrations in the UMLs of the oceans are often reported as being drivers of variation in the size structure and taxonomic composition of phytoplankton communities (Eppley and Peterson, 1979; Chisholm, 1992; Coale et al., 1996; Finkel et al., 2010). With warming-enhanced stratification, such cells will be subjected to higher average exposures to solar radiation, including periodic acute exposure to high fluxes of UV-B close to the surface, which is known to do more harm to smaller cells (Wu et al., 2015).
As stated earlier, it is generally agreed that severe iron (Fe) limitation cancels out (or even reverses) the positive effects of CO2 on cyanobacterial diazotrophs (Fu et al., 2008; Shi et al., 2012; Walworth et al., 2016a; Hong et al., 2017). In contrast, growth limitation by P appears to operate independently, regardless of any effects of changing pCO2 (Hutchins et al., 2007; Garcia et al., 2013b; Walworth et al., 2016a). Trichodesmium cultures simultaneously co-limited by both Fe and P grow considerably faster than either Fe-limited or P-limited cells (Garcia et al., 2015; Walworth et al., 2016a). However, their phenotype relative to acidification is more similar to Fe-limited than P-limited cultures, in that high CO2 does not increase their physiological rates. These high CO2, Fe/P co-limited cells express a unique complement of proteins that is quite distinct from those seen in low CO2 or single nutrient-limited cultures, representing perhaps the manifestation of a genetically determined compensatory mechanism (Walworth et al., 2016a).
Limitation by the micronutrient iron (Fe) is a key control of marine nitrogen fixation and, along with other important variables, Fe supplies are also changing with a shifting climate (Hutchins and Boyd, 2016). Unlike CO2 and P, though, interactions between Fe availability and temperature in N2 fixers do not appear to be linear and additive (Jiang et al., 2018). Warming from 27 to 32°C decreases growth and N2 fixation rates in Fe-replete Trichodesmium, but 32°C is the temperature at which maximum rates are observed in Fe-limited cultures. Consequently, warming across this temperature range nearly erases the negative effects of Fe limitation by greatly increasing cellular iron use efficiencies (moles N fixed per mol cellular Fe per hour) by up to 470%. The net effect of this non-linear interaction between Fe and warming in a global biogeochemical model is a predicted ∼22% increase in total global marine N2 fixation over the next 90 years. This potential alleviation of Fe limitation by warming throughout large parts of the ocean (particularly the oligotrophic Pacific) could also enable currently Fe-limited diazotroph populations to respond positively to concurrently increasing atmospheric pCO2 (Jiang et al., 2018).
Nutrient limitation appears to increase the sensitivity of algae to UV-B radiation. N-limitation for instance can impair the capacity of cells to synthesize UV-B-screening compounds such as MAAs or influence the ability of cells to carry out repair [particularly of the D1 protein in PSII (Shelly et al., 2002)]. P-limitation also appears to decrease the capacity for repair processes under UV-B (Heraud et al., 2005). Similar effects of nutrient availability on UV sensitivity have also been reported in macroalgae. Zheng and Gao (2009) reported higher MAA concentrations in Gracilaria lemaneiformis under nitrate enrichment, which led to significantly decreased UVR-induced inhibition of growth and photosynthesis. In the same organism UV-B significantly reduced the net photosynthetic rate, but this was alleviated by enrichment with ammonia (which also stimulated the accumulation of UV-absorbing compounds) (Xu and Gao, 2012). Similar data have been reported for Porphyra (Korbee et al., 2005) and Gracilaria tenuistipitata (Barufi et al., 2011). In the red alga G. lemaneiformis, UV-induced inhibition of photosynthesis and growth was exacerbated under P-limited conditions (Xu and Gao, 2009). These data and other work cited by Figueroa and Korbee (2010) imply that in macroalgae as well as microalgae, low N-availability enhances UVR sensitivity of photosynthesis and growth, perhaps by decreasing the capacity to minimize damage through MAA synthesis. There are many reports in the literature on this topic, as reviewed by Beardall et al. (2014). However, how such nutrient-related responses to UVR are modulated under the additional stress of OA is not yet known.
OA Effects Under Multiple Stressors
Ocean acidification is a global phenomenon but is usually overlaid by pronounced regional variability modulated by local physics, chemistry, and biology (Boyd et al., 2018; Hurd et al., 2018). Biotic responses to multiple environmental drivers depend on the response to the single dominant driver, and the chance of a driver of larger effect being present increases with the number of drivers (Brennan and Collins, 2015). In addition to the effect of OA together with one additional stressor, the combined effects of OA with two drivers have also been investigated, although relatively fewer papers addressing this can be found. As mentioned above, enhanced stratification due to warming leads to decreased upward transport of nutrients from deeper layers and this in turn can lead to nutrient limitation. Therefore, the effects of OA and warming under changing nutrient levels are of general significance. Li et al. (2018) showed that OA or warming did not affect the specific growth rate of T. pseudonana under nitrate-replete conditions, but both conditions reduced its growth rate under nitrate-limited conditions, suggesting that a decreased upward transport of nutrients due to enhanced stratification could act synergistically with OA and warming to reduce its growth. Under influences of natural environmental conditions, a mesocosm test showed that the toxic microalga Vicicitus globosus has a selective advantage under OA, increasing its abundance in natural plankton communities in oligotrophic subtropical waters, which has had a dramatic impact on the plankton community, disrupting trophic transfer of primary produced organic matter (Riebesell et al., 2018).
In regard to macroalgae, both OA and warming increased germination and juvenile growth in a green tide alga U. rigida regardless of nutrient availability, while the stimulatory effect of OA and warming was reduced by nitrate limitation (Gao et al., 2017). In addition to physiological performance, OA, warming, and nitrate abundance synergistically increased fatty acid content in U. rigida (Gao et al., 2018a), affecting the food quality of this green macroalga. Some macroalgae growing near CO2 seeps appear to use more CO2 rather than bicarbonate except coralline algae, whose abundance declined (Cornwall et al., 2017). In coastal waters, where nutrient availability is high and diel pH fluctuation is typical, most fleshy macroalgae appear to benefit from increasing CO2 concentration, while calcifying algae are harmed by OA (Gao et al., 1993; Gao and Zheng, 2010; Sinutok et al., 2011). It seems that algae respond differentially to OA under influences of multiple stressors. Obviously, more studies are needed in order to visualize comprehensive pictures of how marine photosynthetic organisms in different ecosystems respond to the concurrent ocean climate changes.
Future Perspectives
Microalgae are known to exhibit evolutionary responses to elevated CO2 over hundreds of generations, including downregulated CCMs in a green alga (Collins et al., 2006), irreversible capacity of reduced calcification in a coccolithophorid (Tong et al., 2018), smaller cell size and decreased respiration and photosynthesis in a model diatom (Li et al., 2017), and increased cellular N/C ratio in E. huxleyi (Jin et al., 2013). The evolutionary response of a diazotroph to OA treatment led to irreversible enhancement of growth and N2 fixation over hundreds of generations, driven by “genetic assimilation” of plastic traits into adaptive ones (Hutchins et al., 2015; Walworth et al., 2016a). Adaptation to high CO2 is also associated with the imprint of cytosine methylation of the Trichodesmium genome (Walworth et al., 2016b).
Phytoplankton also shows obvious evolutionary responses to warming. Cultured diatoms from tropical waters increased their optimal growth temperature, maximum growth rate, or maximum critical thermal limit after 200–600 generations of acclimation to elevated temperature, suggesting a fast adaptation to ocean warming (Jin and Agustí, 2018). A decade-long experiment in outdoor mesocosms showed warm-adapted green alga, Chlamydomonas reinhardtii, had higher optimal growth temperatures, higher competitive fitness, and increasing rates of net photosynthesis compared to its control (Schaum et al., 2017).
Ocean acidification and warming can interactively disturb the advection of nutrients and trace elements in the ocean systems, thereby affecting biogeochemical processes and ecosystem stability. Coastal ecosystems and their services are often degraded due to the effects of human activities and global ocean changes. However, these possible macro-changes need micro-mechanism-based explanations to enhance forecasting reliability. Organisms in the upper pelagic zone of the oceans face multiple environmental stresses, such as OA, warming, lack of nutrients (except in coastal waters near human influences), and increased exposures to UVR (Figures 1, 4). OA and warming together could additively increase respiratory rate, since respiration increases with temperature, and acclimation to OA-induced acid–base disturbance requires extra energy to allow cells to cope with. As a result, OA and warming may cumulatively affect the marine biological pump (MBP) and the MCP, which is suggested to be sensitive to warming (Jiao and Zheng, 2011). Nevertheless, the net effects of OA and warming on MBP/MCP are still quite uncertain.
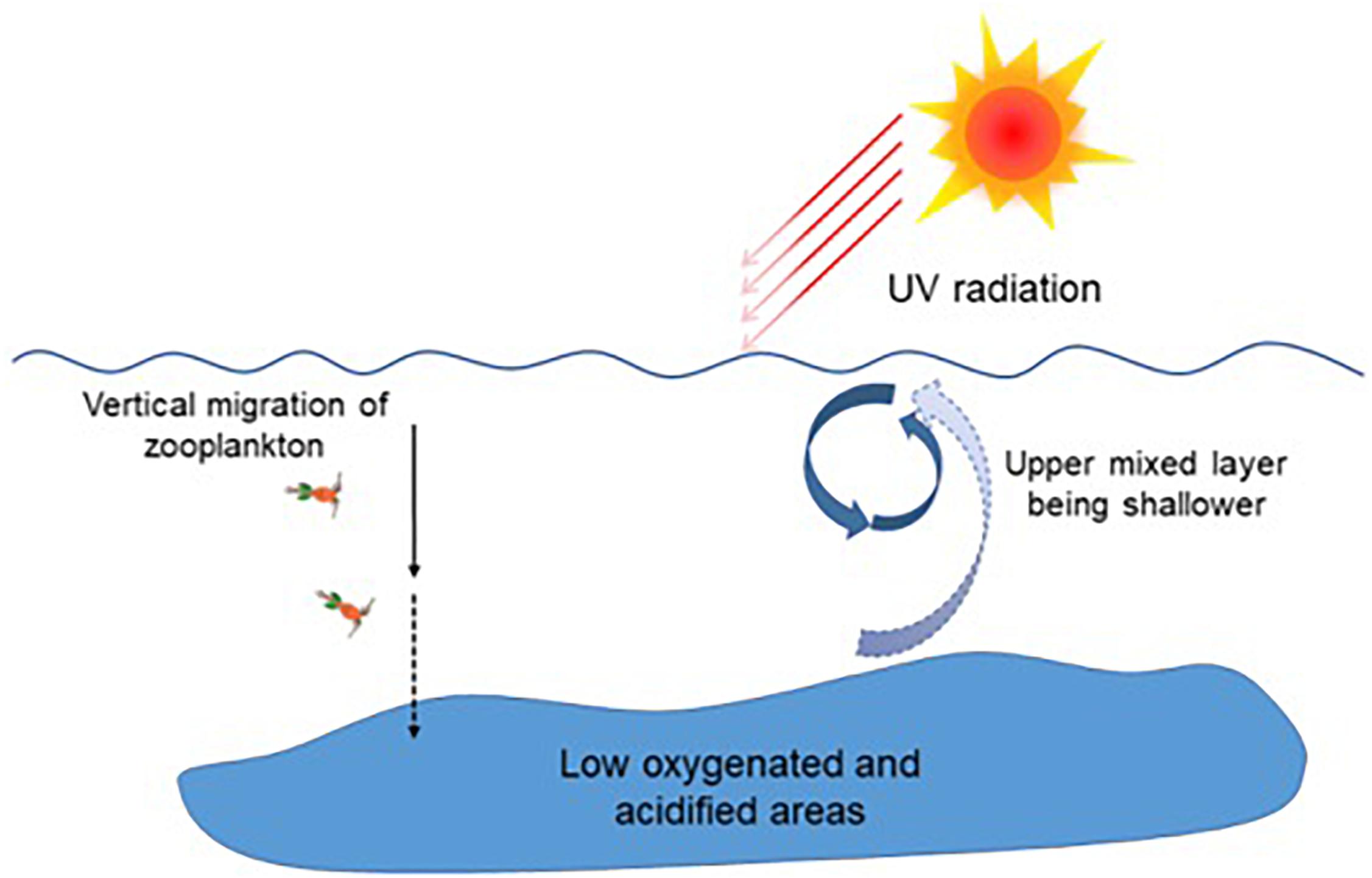
Figure 4. Ocean climate changes and the habitat degradation hypothesis. Ocean warming, acidification, and deoxygenation associated with increasing atmospheric CO2 rise. A shoaled UML due to warming exposes organisms dwelling there to higher levels of solar radiation. The habitable niche degradation hypothesis: phytoplankton abundance and community structure can be altered within the UML under multiple stressors associated with ocean climate changes; and motile organisms dwelling within the UML are stressed due to increased exposure to solar UV radiation and high levels of PAR, which traps more heat; however, the low O2 and pH waters below the UML hamper downward migration. (Re-drawn based on Gao, 2018).
Deoxygenated waters typically coincide with low pH (Cai et al., 2011); therefore, the combined effects of OA and deoxygenation are of ecological relevance. Along with progressive OA and warming, a consequent drop in the pO2/pCO2 of seawater is unavoidable (Figures 1, 4). This ratio has been defined as the respiratory index for animals and may be defined as the photorespiratory index for photosynthetic organisms. Although lower values of pO2/pCO2 are harmful or fatal to many aerobic organisms, decreased pO2/pCO2 ratios may benefit primary producers since their photosynthetic carboxylation enzyme, Rubisco, catalyzes both carboxylation and oxygenation reactions (Gao and Campbell, 2014). Theoretically, lower pO2/pCO2 values will likely correlate with higher carboxylation and lower photorespiration.
Primary producers provide basic energy flows for ecosystems, and consequently, they are closely related to activities of other organisms. The habitable waters for marine organisms are degrading under progressive OA and other ocean climate changes, such as ocean warming, stratification, deoxygenation, and enhanced exposure to UV radiation (Figure 4; Gao et al., 2012a; Hutchins and Fu, 2017). Based on documented data and theoretical reasoning, a habitable niche degradation hypothesis can be established as follows (Figure 4): phytoplankton food quality is supposed to decline in response to OA (Riebesell et al., 2007; Jin et al., 2015), leading to reduced energy supply to grazers; at the same time, motile organisms dwelling within the UML, such as zooplankton or fish, tend to move to deeper layers to avoid exposure to harmful solar UV radiation (Rhode et al., 2001) and high levels of PAR that traps more heat; however, stressful suboxic or hypoxic and acidified seawater below the thermocline may act as a barrier preventing some species from moving deeper. As a result, the habitable niches for such organisms will shrink, and their habitat will degrade. This might exacerbate the effects of other anthropogenic stressors such as over-fishing, and so contribute to declining fish catches in previously productive waters. As a result, more algal blooms will be expected in eutrophicated waters with increasing levels of ocean climate changes and decreasing top-down pressure. This hypothesis could be tested by laboratory microcosm or mesocosm experiments in combination with in situ investigations.
Effects of OA can be positive, neutral, and negative under influences of other drivers or in different ecosystems where chemical and physical environments are contrastingly different. As discussed above, in coastal waters, fleshy macroalgae such as Porphyra sp. (Gao et al., 1991), Gracilaria sp. (Gao et al., 1993), Ulva (Gao et al., 2016), and Sargassum sp. (Xu et al., 2017), usually increase their growth in response to elevated pCO2 levels up to 1,000–2,000 μatm, showing tolerance to acidification. Larger diatom species are found to show stimulated growth (Wu et al., 2014) or enhanced carbon assimilation rate (Li et al., 2016), while smaller diatoms tend to show reduced photosynthetic and growth rates under influence of OA (Wu et al., 2014), nitrate limitation, and temperature rise (Li et al., 2018). On the other hand, smaller phytoplankton cells in open ocean showed higher photosynthetic inhibition caused by UV radiation (Li et al., 2011). It is most likely that the combined effects of OA and solar UVR induce more carbon loss from the cost to open ocean in phytoplankton assemblages (Figure 5). Such a hypothesis needs observational and experimental data to be tested.
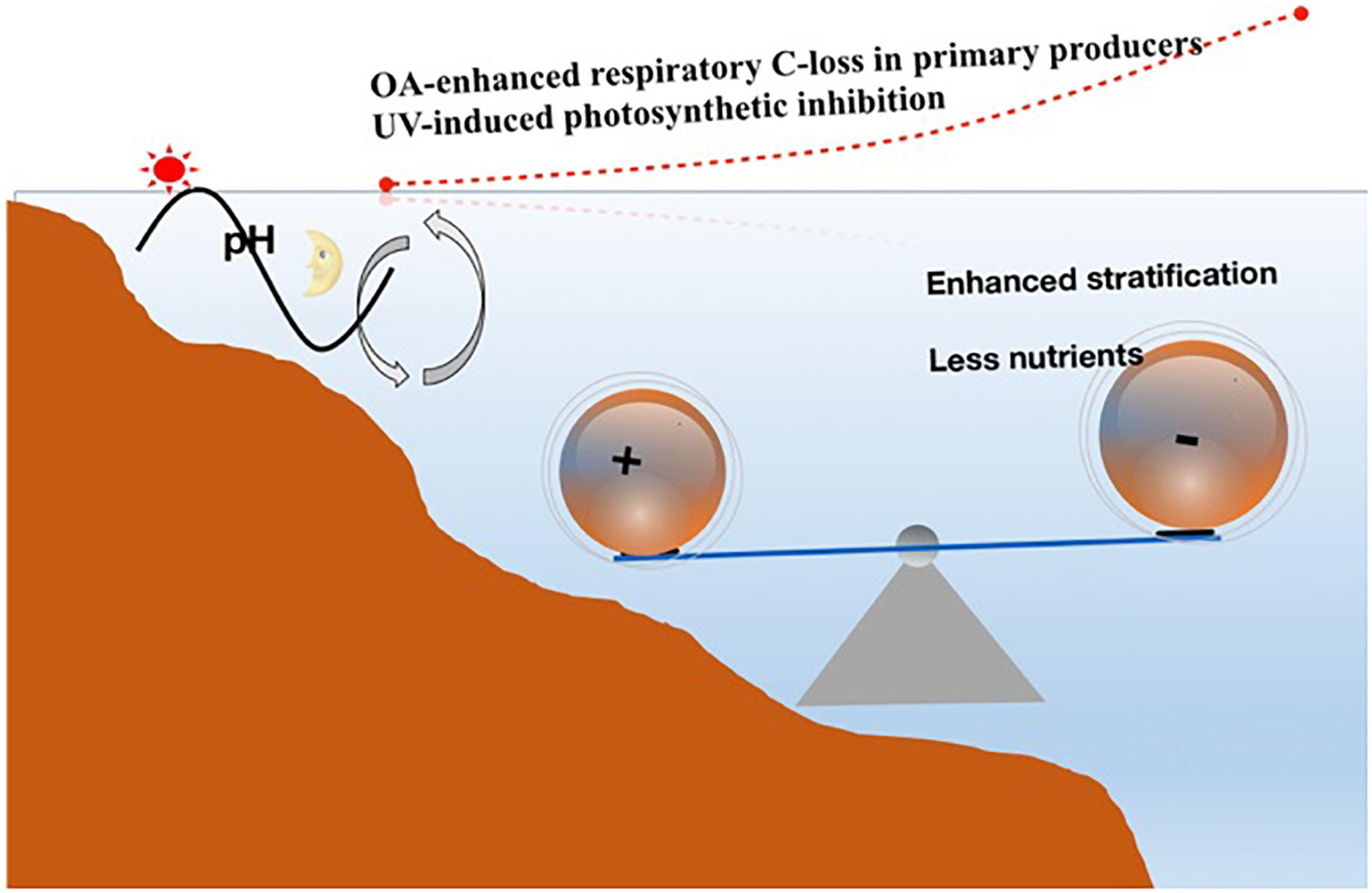
Figure 5. Illustration of the hypothesis that OA and UV synergistically induce carbon loss in surface primary producers, based on the observational data on UV impacts (Li et al., 2011) and results that smaller diatoms decrease their growth rate under OA and nutrient-limitation conditions (Li et al., 2018). Diel pH changes in highly productive coastal waters are shown with a sun and a moon symbol to indicate pH rise with increasing photosynthetic C removal during daytime and pH decline with respiratory CO2 release during night. Note that benthic macroalgae contribute greatly to the diel pH fluctuations, and that their growth and photosynthesis are usually stimulated by OA. Furthermore, they are more tolerant of solar UV radiation [see the review by Gao et al. (2012) and literature therein]. The symbols “+” and “–” indicate more positive effects due to OA and UV in coastal non-nutrient-limited waters and negative effects due to OA and UV in oligotrophic offshore waters, where shoaling of UMLs owing to warming reduces upward transport of nutrients from deeper layers.
In summary, OA effects under multiple stressors have been documented but only in association with a very limited number of drivers. Future work toward understanding the ecological impacts of ocean climate changes should include both scenario-oriented and mechanism-directed studies. Considering the impractical nature of the number of treatments and replicates needed to accommodate all combinations of possible drivers in experimental designs, it is highly recommended to refer to the recently published guide for multiple drivers marine research (Boyd et al., 2018, 2019). With increasing understanding of multiple stressors effects using controlled experiments in combination with field observational findings, OA effects in different ecosystems under multiple stressors can thus be understood both mechanistically and predictively.
Author Contributions
KG contributed to the theoretical designs, data analysis, and writing of the manuscript. JB, D-PH, JH-S, GG, and DH contributed to the analysis of the data and writing of the manuscript.
Funding
This study was supported by the National Key R&D Program (2016YFA0601400), National Natural Science Foundation (41430967, 41720104005, and 41721005), Joint Project of National Natural Science Foundation of China and Shandong Province (No. U1606404), and the U.S. National Science Foundation (OCE 1538525, 1638804, 1657757, and 1851222).
Conflict of Interest Statement
The authors declare that the research was conducted in the absence of any commercial or financial relationships that could be construed as a potential conflict of interest.
Footnotes
- ^https://www.esrl.noaa.gov/gmd/ccgg/trends/gl_trend.html
- ^https://oceanexplorer.noaa.gov/oceanexploration2020
References
Agostini, S., Harvey, B. P., Wada, S., Kon, K., Milazzo, M., Inaba, K., et al. (2018). Ocean acidification drives community shifts towards simplified non-calcified habitats in a subtropical-temperate transition zone. Sci. Rep. 8:11354. doi: 10.1038/s41598-018-29251-7
Bao, B., and Ren, G. (2014). Climatological characteristics and long-term change of SST over the marginal seas of China. Cont. Shelf Res. 77, 96–106. doi: 10.1016/j.csr.2014.01.013
Barcelos e Ramos, B. J., Biswas, H., Schulz, K., LaRoche, J., and Riebesell, U. (2007). Effect of rising atmospheric carbon dioxide on the marine nitrogen fixer Trichodesmium. Glob. Biogeochem. Cycles 21:GB2028. doi: 10.1029/2006GB002898
Barufi, J. B., Korbee, N., Oliviera, M. C., and Figueroa, F. L. (2011). Effects of N supply on the accumulation of photosynthetic pigments and photoprotectors in Gracilaria tenuistipitata (Rhodophyta) cultured under UV radiation. J. Appl. Phycol. 23, 457–466. doi: 10.1007/s10811-010-9603-x
Beardall, J., Quigg, A. S., and Raven, J. A. (2003). “Oxygen consumption: Photorespiration and chlororespiration,” in Photosynthesis in the Algae, eds A. W. D. Larkum, S. E. Douglas, and J. A. Raven (Dordrecht: Springer), 157–181. doi: 10.1007/978-94-007-1038-2_8
Beardall, J., Stojkovic, S., and Gao, K. (2014). Interactive effects of nutrient supply and other environmental factors on the sensitivity of marine primary producers to ultraviolet radiation: implications for the impacts of global change. Aqu. Biol. 22, 5–23. doi: 10.3354/ab00582
Beer, S., Larsson, C., Poryan, O., and Axelsson, L. (2000). Photosynthetic rates of Ulva (Chlorophyta) measured by pulse amplitude modulated (PAM) fluorometry. Eur. J. Phycol. 35, 69–74. doi: 10.1017/s0967026299002553
Bell, P. R., Elmetri, I., and Lapointe, B. E. (2014). Evidence of large-scale chronic eutrophication in the great barrier reef: quantification of chlorophyll a thresholds for sustaining coral reef communities. Ambio 43, 361–376. doi: 10.1007/s13280-013-0443-1
Bigg, G., and Hanna, E. (2016). “Impacts and effects of ocean warming on the weather,” in Explaining Ocean Warming: Causes, Scale, Effects and Consequences, eds D. Laffoley and J. M. Baxter (Gland: IUCN), 359–372.
Black, C., Burris, J., and Everson, R. (1976). Influence of oxygen concentration on photosynthesis in marine plants. Funct. Plant Biol. 3, 81–86.
Böttjer, D., Karl, D. M., Letelier, R. M., Viviani, D. A., and Church, M. J. (2014). Experimental assessment of diazotroph responses to elevated seawater pCO2 in the North Pacific Subtropical Gyre. Glob. Biogeochem. Cycles 28, 601–616. doi: 10.1002/2013gb004690
Boyd, P., Collins, S., Dupont, S., Fabricius, K., Gattuso, J.-P., Havenhand, J., et al. (2018). Experimental strategies to assess the biological ramifications of multiple drivers of ocean global ocean—a review. Glob. Change Biol. 24, 2239–2261. doi: 10.1111/gcb.14102
Boyd, P. W., Collins, S., Dupont, S., Fabricius, K., Gattuso, J.-P., Havenhand, J., et al. (2019). SCOR WG149 Handbook to support the SCOR Best Practice Guide for ‘Multiple Drivers’ Marine Research. Hobart: University of Tasmania for Scientific Committee on Oceanic Research (SCOR).
Breitbarth, E., Oschilles, A., and LaRoche, J. L. (2007). Physiological constraints on the global distribution of Trichodesmium - effect of temperature on diazotrophy. Biogeosciences 4, 53–61. doi: 10.5194/bg-4-53-2007
Breitburg, D., Levin, L. A., Oschlies, A., Grégoire, M., Chavez, F. P., Conley, D. J., et al. (2018). Declining oxygen in the global ocean and coastal waters. Science 359:eaam7240. doi: 10.1126/science.aam7240
Brennan, G., and Collins, S. (2015). Growth responses of a green alga to multiple environmental drivers. Nat. Clim. Change 5, 892–897. doi: 10.1038/nclimate2682
Brewer, P. G., and Peltzer, E. T. (2009). Oceans: limits to marine life. Science 324, 347–348. doi: 10.1126/science.1170756
Broadgate, W., Riebesell, U., Armstrong, C., Brewer, P., Denman, K., Feely, R., et al. (2013). Ocean Acidification Summary for Policymakers–Third Symposium on the Ocean in a High-CO2 World. Paris: UNESCO.
Cai, R., Tan, H., and Kontoyiannis, H. (2017). Robust surface warming in offshore China seas and its relationship to the East Asian monsoon wind field and ocean forcing on interdecadal time scales. J. Clim. 30, 8987–9004. doi: 10.1175/JCLI-D-16-0016.1
Cai, W.-J., Hu, X., Huang, W.-J., Murrell, M. C., Lehrter, J. C., Lohrenz, S. E., et al. (2011). Acidification of subsurface coastal waters enhanced by eutrophication. Nat. Geosci. 4, 766–770.
Cai, X., Hutchins, D. A., Fu, F., and Gao, K. (2017). Effects of ultraviolet radiation on photosynthetic performance and N2 fixation in Trichodesmium erythraeum IMS 101. Biogeosciences 14:4455. doi: 10.5194/bg-14-4455-2017
Capone, D. G., and Carpenter, E. J. (1982). Nitrogen fixation in the marine environment. Science 217, 1140–1142. doi: 10.1126/science.217.4565.1140
Cavan, E. L., Henson, S. A., and Boyd, P. W. (2019). The sensitivity of subsurface microbes to ocean warming accentuates future declines in particulate carbon export. Front. Ecol. Evol. 6:230. doi: 10.3389/fevo.2018.00230
Celis-Plá, P. S., Martínez, B., Korbee, N., Hall-Spencer, J. M., and Figueroa, F. L. (2017). Ecophysiological responses to elevated CO2 and temperature in Cystoseira tamariscifolia (Phaeophyceae). Clim. Change 142, 67–81. doi: 10.1007/s10584-017-1943-y
Chen, Y. L. L., Chen, H. Y., Lin, Y. H., Yong, T. C., Taniuchi, Y., and Tuo, S. H. (2014). The relative contributions of unicellular and filamentous diazotrophs to N2 fixation in the South China Sea and the upstream Kuroshio. Deep Sea Res. Part I Oceanogr. Res. Pap. 85, 56–71. doi: 10.1016/j.dsr.2013.11.006
Chisholm, S. W. (1992). “Phytoplankton size,” in Primary Productivity and Biogeochemical Cycles in the Sea, eds P. G. Falkowski and A. D. Woodhead (Boston, MA: Springer), 213–237. doi: 10.1007/978-1-4899-0762-2_12
Coale, K. H., Johnson, K. S., Fitzwater, S. E., Gordon, R. M., Tanner, S., Chavez, F. P., et al. (1996). A massive phytoplankton bloom induced by an ecosystem-scale iron fertilization experiment in the equatorial Pacific Ocean. Nature 383, 495–501. doi: 10.1038/383495a0
Collins, S., Sueltemeyer, D., and Bell, G. (2006). Changes in C uptake in populations of Chlamydomonas reinhardtii selected at high CO2. Plant Cell Environ. 29, 1812–1819. doi: 10.1111/j.1365-3040.2006.01559.x
Comeau, S., Carpenter, R., Lantz, C., and Edmunds, P. (2016). Parameterization of the response of calcification to temperature and pCO2 in the coral Acropora pulchra and the alga Lithophyllum kotschyanum. Coral Reefs 35, 929–939. doi: 10.1007/s00338-016-1425-0
Connell, S. D., Doubleday, Z. A., Foster, N. R., Hamlyn, S. B., Harley, C. D. G., Helmuth, B., et al. (2018). The duality of ocean acidification as a resource and a stressor. Ecology 99, 1005–1010. doi: 10.1002/ecy.2209
Cornwall, C. E., Hepburn, C. D., Pritchard, D., Currie, K. I., McGraw, C. M., Hunter, K. A., et al. (2012). Carbon-use strategies in macroalgae: Differential responses to lowered pH and implications for ocean acidification. J. Phycol. 48, 137–144. doi: 10.1111/j.1529-8817.2011.01085.x
Cornwall, C. E., Revill, A. T., Hall-Spencer, J. M., Milazzo, M., Raven, J. A., and Hurd, C. L. (2017). Inorganic carbon physiology underpins macroalgal responses to elevated CO2. Sci. Rep. 7:46297. doi: 10.1038/srep46297
Cornwall, C. E., Revill, A. T., and Hurd, C. L. (2015). High prevalence of diffusive uptake of CO2 by macroalgae in a temperate subtidal ecosystem. Photosyn. Res. 124, 181–190. doi: 10.1007/s11120-015-0114-0
Czerny, J., Barcelos, E., Ramos, J., and Riebesell, U. (2009). Influence of elevated CO2 concentrations on cell division and nitrogen fixation rates in the bloom-forming cyanobacterium Nodularia spumigena. Biogeosciences 6, 1865–1875. doi: 10.5194/bg-6-1865-2009
Czerny, J., Schulz, K. G., Boxhammer, T., Bellerby, R., Büdenbender, J., Engel, A., et al. (2013). Implications of elevated CO2 on pelagic carbon fluxes in an Arctic mesocosm study–an elemental mass balance approach. Biogeosciences 10, 3109–3125. doi: 10.5194/bg-10-3109-2013
Dai, M., Lu, Z., Zhai, W., Chen, B., Cao, Z., Zhou, K., et al. (2009). Diurnal variations of surface seawater pCO2 in contrasting coastal environments. Limnol. Oceanogr. 54, 735–745. doi: 10.4319/lo.2009.54.3.0735
Danovaro, R., Corinaldesi, C., Rastelli, E., and Dell’anno, A. (2016). “Impacts and effects of ocean warming on microorganisms,” in Explaining Ocean Warming: Causes, Scale, Effects and Consequences, eds D. Laffoley and J. M. Baxter (Gland: IUCN), 57–74.
Drechsler, Z., and Beer, S. (1991). Utilization of inorganic carbon by Ulva lactuca. Plant Physiol. 97, 1439–1444. doi: 10.1104/pp.97.4.1439
Eichner, M., Rost, B., and Kranz, S. A. (2014). Diversity of ocean acidification effects on marine N2 fixers. J. Exp. Mar. Biol. Ecol. 457, 199–207. doi: 10.1016/j.jembe.2014.04.015
Enochs, I. C., Manzello, D. P., Donham, E. M., Kolodziej, G., Okano, R., Johnston, L., et al. (2015). Shift from coral to macroalgae dominance on a volcanically acidified reef. Nat. Clim. Change 5, 1083–1088. doi: 10.1038/nclimate2758
Eppley, R. W., and Peterson, B. J. (1979). Particulate organic matter flux and planktonic new production in the deep ocean. Nature 282, 677–680. doi: 10.1038/282677a0
Fabricius, K. E., Langdon, C., Uthicke, S., Humphrey, C., Noonan, S., De’ath, G., et al. (2011). Losers and winners in coral reefs acclimatized to elevated carbon dioxide concentrations. Nat. Clim. Change 1, 165–169. doi: 10.1038/nclimate1122
Falkowski, P. G., and Raven, J. A. (2013). Aquatic Photosynthesis. Princeton, NY: Princeton University Press.
Feng, Y., Hare, C. E., Leblanc, K., Rose, J., Zhang, Y., DiTullio, G. R., et al. (2009). The effects of increased pCO2 and temperature on the North Atlantic Spring Bloom: I. the phytoplankton community and biogeochemical response. Mar. Ecol. Prog. Ser. 388, 13–25. doi: 10.3354/meps08133
Feng, Y., Warner, M. E., Zhang, Y., Sun, J., Fu, F.-X., and Hutchins, D. A. (2008). Interactive effects of increased pCO2, temperature and irradiance on the marine coccolithophore Emiliania huxleyi (Prymnesiophyceae). Eur. J. Phycol. 43, 87–98. doi: 10.1080/09670260701664674
Figueroa, F. L., and Korbee, N. (2010). “Interactive effects of UV radiation and nutrients on ecophysiology:vulnerability and adaptation to climate change,” in Seaweeds and Their Role in Globally Changing Environments, eds A. Israel, R. Einvav, and J. Seckbach (Dordrecht: Springer), 157–182. doi: 10.1007/978-90-481-8569-6_10
Finkel, Z. V., Beardall, J., Flynn, K. J., Quigg, A., Rees, T. A. V., and Raven, J. A. (2010). Phytoplankton in a changing world: cell size and elemental stoichiometry. J. Plankt. Res. 32, 119–137. doi: 10.1093/plankt/fbp098
Fischetti, M. (2013). Deep heat threatens marine life. Sci. Am. 308:92. doi: 10.1038/scientificamerican0413-92
Foo, S. A., Byrne, M., Ricevuto, E., and Gambi, M. C. (2018). “The carbon dioxide vents of Ischia, Italy, a natural system to assess impacts of ocean acidification on marine ecosystems: An overview of research and comparisons with other vent systems,” in Oceanography and Marine Biology An Annual Review, Vol. 56, eds R. N. Gibson, R. J. A. Atkinson, and J. D. M. Gordon (Didcot: Taylor & Francis), 237–310. doi: 10.1201/9780429454455-4
Frölicher, T. L., Fischer, E. M., and Gruber, N. (2018). Marine heatwaves under global warming. Nature 560, 360–364. doi: 10.1038/s41586-018-0383-9
Fu, F.-X., Mulholland, M. R., Garcia, N., Beck, A., Bernhardt, P. W., Warner, M. E., et al. (2008). Interactions between changing pCO2, N2 fixation, and Fe limitation in the marine unicellular cyanobacterium Crocosphaera. Limnol. Oceanogr. 53, 2472–2484. doi: 10.4319/lo.2008.53.6.2472
Fu, F.-X., Place, A. R., Garcia, N. S., and Hutchins, D. A. (2010). CO2 and phosphate availability control the toxicity of the harmful bloom dinoflagellate Karlodinium veneficum. Aqu. Microb. Ecol. 59, 55–65. doi: 10.3354/ame01396
Fu, F. X., Warner, M. E., Zhang, Y., Feng, Y., and Hutchins, D. A. (2007). Effects of increased temperature and CO2 on photosynthesis, growth, and elemental ratios in marine Synechococcus and Prochlorococcus (Cyanobacteria). J. Phycol. 43, 485–496. doi: 10.1111/j.1529-8817.2007.00355.x
Fu, F.-X., Yu, E., Garcia, N. S., Gale, J., Luo, Y., Webb, E. A., et al. (2014). Differing responses of marine N2-fixers to warming and consequences for future diazotroph community structure. Aqu. Microb. Ecol. 72, 33–46. doi: 10.3354/ame01683
Ganapathy, K., Chidambaram, K., Janarthanan, R., and Ramasamy, R. (2017). Effect of UV-B radiation on growth, photosynthetic activity and metabolic activities of Chlorella vulgaris. J. Microbiol. Biotechnol. 6, 53–60.
Gao, G., Clare, A. S., Rose, C., and Caldwell, G. S. (2017). Eutrophication and warming-driven green tides (Ulva rigida) are predicted to increase under future climate change scenarios. Mar. Pollut. Bull. 114, 439–447. doi: 10.1016/j.marpolbul.2016.10.003
Gao, G., Liu, Y., Li, X., Feng, Z., and Xu, J. (2016). An ocean acidification acclimatised green tide alga is robust to changes of seawater carbon chemistry but vulnerable to light stress. PLoS One 11:e0169040. doi: 10.1371/journal.pone.0169040
Gao, G., Clare, A. S., Chatzidimitriou, E., Rose, C., and Caldwell, G. (2018a). Effects of ocean warming and acidification, combined with nutrient enrichment, on chemical composition and functional properties of Ulva rigida. Food Chem. 258, 71–78. doi: 10.1016/j.foodchem.2018.03.040
Gao, G., Clare, A. S., Rose, C., and Caldwell, G. S. (2018b). Ulva rigida in the future ocean: potential for carbon capture, bioremediation and biomethane production. Glob. Change Biol. Bioenerg. 10, 39–51. doi: 10.1111/gcbb.12465
Gao, G., Xu, Z., Shi, Q., and Wu, H. (2018c). Increased CO2 exacerbates the stress of ultraviolet radiation on photosystem II function in the diatom Thalassiosira weissflogii. Environ. Exp. Bot. 156, 96–105. doi: 10.1016/j.envexpbot.2018.08.031
Gao, K. (2018). Ecological and physiological effects of ocean acidification and their correlations with warming, UV radiation and deoxygenation. J. Xiamen Univ. 57, 800–810.
Gao, K., Aruga, Y., Asada, K., Ishihara, T., Akano, T., and Kiyohara, M. (1991). Enhanced growth of the red alga Porphyra yezoensis Ueda in high CO2 concentrations. J. Appl. Phycol. 3, 355–362.
Gao, K., Aruga, Y., Asada, K., Ishihara, T., Akano, T., and Kiyohara, M. (1993). Calcification in the articulated coralline alga Corallina pilulifera, with special reference to the effect of elevated CO2 concentration. Mar. Biol. 117, 129–132. doi: 10.1007/bf00346434
Gao, K., and Campbell, D. (2014). Photophysiological responses of marine diatoms to elevated CO2 and decreased pH: a review. Funct. Plant Biol. 41, 449–459. doi: 10.1071/FP13247
Gao, K., Helbling, E. W., Häder, D. P., and Hutchins, D. A. (2012a). Responses of marine primary producers to interactions between ocean acidification, solar radiation, and warming. Mar. Ecol. Prog. Ser. 470, 167–189. doi: 10.3354/meps10043
Gao, K., Xu, J., Gao, G., Li, Y., Hutchins, D. A., Huang, B., et al. (2012b). Rising carbon dioxide and increasing light exposure act synergistically to reduce marine primary productivity. Nat. Clim. Change 2, 519–523. doi: 10.1038/nclimate1507
Gao, K., Li, P., Watanabe, T., and Helbling, E. W. (2008). Combined effects of ultraviolet radiation and temperature on morphology, photosynthesis and DNA of Arthrospira (Spirulina) platensis (cyanophyta). J. Phycol. 44, 777–786. doi: 10.1111/j.1529-8817.2008.00512.x
Gao, K., Ruan, Z., Villafane, V. E., Gattuso, J.-P., and Helbling, E. W. (2009). Ocean acidification exacerbates the effect of UV radiation on the calcifying phytoplankter Emiliania huxleyi. Limnol. Oceanogr. 54, 1855–1862. doi: 10.4319/lo.2009.54.6.1855
Gao, K., Wu, Y., Li, G., Wu, H., Villafañe, E. V., and Helbling, E. W. (2007a). Solar UV-radiation drives CO2-fixation in marine phytoplankton: a double-edged sword. Plant Physiol. 144, 54–59. doi: 10.1104/pp.107.098491
Gao, K., Yu, H., and Brown, M. (2007b). Solar PAR and UV radiation affects the physiology and morphology of the cyanobacterium Anabaena sp. PCC 7120. J. Photochem. Photobiol. B Biol. 89, 117–124. doi: 10.1016/j.jphotobiol.2007.09.006
Gao, K., and Zheng, Y. (2010). Combined effects of ocean acidification and solar UV radiation on photosynthesis, growth, pigmentation and calcification of the coralline alga Corallina sessilis (Rhodophyta). Glob. Change Biol. 16, 2388–2398. doi: 10.1111/j.1365-2486.2009.02113.x
Garbelli, C., Angiolini, L., and Shen, S. Z. (2017). Biomineralization and global change: a new perspective for understanding the end-Perm extinction. Geology 45, 19–21. doi: 10.1130/G38430.1
Garcia, N. S., Fu, F. X., Breene, C. L., Yu, E., Bernhardt, P. W., Mulholland, M. R., et al. (2013a). Combined effects of CO2 and light on large and small isolates of the unicellular N2-fixing cyanobacterium Crocosphaera watsonii from the western tropical Atlantic Ocean. Eur. J. Phycol. 48, 128–139. doi: 10.1080/09670262.2013.773383
Garcia, N. S., Fu, F.-X., and Hutchins, D. A. (2013b). Colimitation of the the unicellular photosynthetic diazotroph Crocosphaera watsonii by phosphorus, light and carbon dioxide. Limnol. Oceanogr. 58, 1501–1512. doi: 10.4319/lo.2013.58.4.1501
Garcia, N. S., Fu, F.-X., Sedwick, P. N., and Hutchins, D. A. (2015). Iron deficiency increases growth and nitrogen fixation rates of phosphorus-deficient marine cyanobacteria. ISME J. 9, 238–245. doi: 10.1038/ismej.2014.104
García-Gómez, C., Gordillo, F. J., Palma, A., Lorenzo, M. R., and Segovia, M. (2014). Elevated CO2 alleviates high PAR and UV stress in the unicellular chlorophyte Dunaliella tertiolecta. Photochem. Photobiol. Sci. 13, 1347–1358. doi: 10.1039/c4pp00044g
García-Gómez, C., Mata, M. T., Van Breusegem, F., and Segovia, M. (2016). Low-steady-state metabolism induced by elevated CO2 increases resilience to UV radiation in the unicellular green-algae Dunaliella tertiolecta. Environ. Exp. Bot. 132, 163–174. doi: 10.1016/j.envexpbot.2016.09.001
Gattuso, J.-P., Magnan, A., Billé, R., Cheung, W., Howes, E., Joos, F., et al. (2015). Contrasting futures for ocean and society from different anthropogenic CO2 emissions scenarios. Science 349:aac4722. doi: 10.1126/science.aac4722
Giordano, M., Beardall, J., and Raven, J. A. (2005). CO2 concentrating mechanisms in algae: mechanisms, environmental modulation and evolution. Ann. Rev. Plant Biol. 56, 99–131. doi: 10.1146/annurev.arplant.56.032604.144052
Goiris, K., Van Colen, W., Wilches, I., León-Tamariz, F., De Cooman, L., and Muylaert, K. (2015). Impact of nutrient stress on antioxidant production in three species of microalgae. Algal Res. 7, 51–57. doi: 10.1016/j.algal.2014.12.002
González-Delgado, S., and Hernández, J. C. (2018). The importance of natural acidified systems in the study of ocean acidification: what have we learned? Adv. Mar. Biol. 80, 57–99. doi: 10.1016/bs.amb.2018.08.001
Gradoville, M. R., White, A. E., Böttjer, D., Church, M. J., and Letelier, R. M. (2014). Diversity trumps acidification: Lack of evidence for carbon dioxide enhancement of Trichodesmium community nitrogen or carbon fixation at Station ALOHA. Limnol. Oceanogr. 59, 645–659. doi: 10.4319/lo.2014.59.3.0645
Guy, G. P. Jr., Machlin, S. R., Ekwueme, D. U., and Yabroff, K. R. (2015). Prevalence and costs of skin cancer treatment in the U.S., 2002-2006 and 2007-2011. Am. J. Prev. Med. 48, 183–187. doi: 10.1016/j.amepre.2014.08.036
Haas, A. F., Smith, J. E., Thompson, M., and Deheyn, D. D. (2014). Effects of reduced dissolved oxygen concentrations on physiology and fluorescence of hermatypic corals and benthic algae. PeerJ 2:e235. doi: 10.7717/peerj.235
Häder, D.-P., and Gao, K. (2015). Interactions of anthropogenic stress factors on marine phytoplankton. Front. Environ. Sci. 3:14.
Hall-Spencer, J. M., and Harvey, B. (2019). Ocean acidification impacts on coastal ecosystem services due to habitat degradation. Emerging Top. Life Sci. 3, 197–206. doi: 10.1042/ETLS20180117
Hall-Spencer, J. M., Rodolfo-Metalpa, R., Martin, S., Ransome, E., Fine, M., Turner, S. M., et al. (2008). Volcanic carbon dioxide vents show ecosystem effects of ocean acidification. Nature 454, 96–99. doi: 10.1038/nature07051
Helbling, E. W., Carrillo, P., Medina-Sánchez, J. M., Durán, C., Herrera, G., Villar-Argaiz, M., et al. (2013). Interactive effects of vertical mixing, nutrients and ultraviolet radiation: in situ photosynthetic responses of phytoplankton from high mountain lakes in Southern Europe. Biogeosciences 10, 1037–1050. doi: 10.5194/bg-10-1037-2013
Helbling, E. W., Gao, K., Gonçalves, R. J., Wu, H., and Villafañe, V. E. (2003). Utilization of solar UV radiation by coastal phytoplankton assemblages off SE China when exposed to fast mixing. Mar. Ecol. Prog. Ser. 259, 59–66. doi: 10.3354/meps259059
Heraud, P., Roberts, S., Shelly, K., and Beardall, J. (2005). Interactions between UVB exposure and phosphorus nutrition: II. effects on rates of damage and repair. J. Phycol. 41, 1212–1218. doi: 10.1111/j.1529-8817.2005.00149.x
Hobday, A. J., Alexander, L. V., Perkins, S. E., Smale, D. A., Straub, S. C., Oliver, E. C., et al. (2016). A hierarchical approach to defining marine heatwaves. Prog. Oceanogr. 141, 227–238. doi: 10.1016/j.pocean.2015.12.014
Hofmann, L. C., Straub, S., and Bischof, K. (2012). Competition between calcifying and noncalcifying temperate marine macroalgae under elevated CO2 levels. Mar. Ecol. Prog. Ser. 464, 89–105. doi: 10.3354/meps09892
Hong, H., Shen, R., Zhang, F., Wen, Z., Chang, S., Lin, W., et al. (2017). The complex effects of ocean acidification on the prominent N2-fixing cyanobacterium Trichodesmium. Science 356, 527–531. doi: 10.1126/science.aal2981
Hönisch, B., Ridgwell, A., Schmidt, D. N., Thomas, E., Gibbs, S. J., Sluijs, A., et al. (2012). The geological record of ocean acidification. Science 335, 1058–1063. doi: 10.1126/science.1208277
Huang, Y., Liu, X., Laws, E. A., Bingzhang, C., Li, Y., Xie, Y., et al. (2018). Effects of increasing atmospheric CO2 on the marine phytoplankton and bacterial metabolism during a bloom: a coastal mesocosm study. Sci. Total Environ. 633, 618–629. doi: 10.1016/j.scitotenv.2018.03.222
Hurd, C. L., Lenton, A., Tilbrook, B., and Boyd, P. W. (2018). Current understanding and challenges for oceans in a higher-CO2 world. Nat. Clim. Change 8, 686–694. doi: 10.1038/s41558-018-0211-0
Hutchins, D., Fu, F.-X., Zhang, Y., Warner, M. E., Feng, Y., Portune, K., et al. (2007). CO2 control of Trichodesmium N2 fixation, photosynthesis, growth rates, and elemental ratios: implications for past, present, and future ocean biogeochemistry. Limnol. Oceanogr. 52, 1293–1304. doi: 10.4319/lo.2007.52.4.1293
Hutchins, D. A., and Boyd, P. W. (2016). Marine phytoplankton and the changing ocean iron cycle. Nat. Clim. Change 6, 1071–1079.
Hutchins, D. A., and Fu, F. X. (2017). Microorganisms and ocean global change. Nat. Microbiol. 2:17508. doi: 10.1038/nmicrobiol.2017.58
Hutchins, D. A., Fu, F.-X., Walworth, N. G., Lee, M. D., Saito, M. A., and Webb, E. A. (2017). Comment on “The complex effects of ocean acidification on the prominent N2-fixing cyanobacterium Trichodesmium”. Science 357:eaao006. doi: 10.1126/science.aao0067
Hutchins, D. A., Fu, F.-X., Webb, E. A., Walworth, N., and Tagliabue, A. (2013). Taxon-specific response of marine nitrogen fixers to elevated carbon dioxide concentrations. Nat. Geosci. 6, 790–795. doi: 10.1038/ngeo1858
Hutchins, D. A., Jansson, J. K., Remais, J., Rich, V. I, Singh, B. K., and Trivedi, P. (2019). Climate change microbiology—problems and perspectives. Nat. Rev. Microbiol. 17, 391–396. doi: 10.1038/s41579-019-0178-5
Hutchins, D. A., Walworth, N. G., Webb, E. A., Saito, M. A., Moran, D., Mcilvin, M. R., et al. (2015). Irreversibly increased nitrogen fixation in Trichodesmium experimentally adapted to elevated carbon dioxide. Nat. Commun. 6:8155. doi: 10.1038/ncomms9155
Hyun, B., Choi, K.-H., Jang, P.-G., Jang, M.-C., Lee, W.-J., Moon, C.-H., et al. (2014). Effects of increased CO2 and temperature on the growth of four diatom species (Chaetoceros debilis, Chaetoceros didymus, Skeletonema costatum and Thalassiosira nordenskioeldii) in laboratory experiments. J. Environ. Sci. Int. 23, 1003–1012.
IPCC (2013a). (Intergovernmental Panel on Climate Change): Working Group I Contribution to the IPCC Fifth Assessment Report Climate Change 2013: The Physical Science Basis. Cambridge: Cambridge University Press.
IPCC (2013b). “Summary for Policymakers: Climate change 2013 - The physical science basis,” in Working Group 1 Contribution to the IPCC Fifth Assessment Report, eds T. F. S. T. G. Stocker, D. Qin, G.-K. Plattner, M. Tignor, S. K. Allen, J. Boschung, et al. (Cambridge: Cambridge University Press).
Jiang, H.-B., Fu, F.-X., Rivero-Calle, S., Levine, N., Sañudo-Wilhelmy, S. A., Qu, P.-P., et al. (2018). Ocean warming alleviates iron limitation of marine nitrogen fixation. Nat. Clim. Change 8, 709–712. doi: 10.1038/s41558-018-0216-8
Jiao, N., Herndl, G. J., Hansell, D. A., Benner, R., Kattner, G., Wilhelm, S. W., et al. (2010). Microbial production of recalcitrant dissolved organic matter: long-term carbon storage in the global ocean. Nat. Rev. Microbiol. 8:593. doi: 10.1038/nrmicro2386
Jiao, N., and Zheng, Q. (2011). The microbial carbon pump–from genes to ecosystems. Appl. Environ. Microbiol. 77, 7439–7444. doi: 10.1128/AEM.05640-11
Jin, P., and Agustí, S. (2018). Fast adaptation of tropical diatoms to increased warming with trade-offs. Sci. Rep. 8:17771. doi: 10.1038/s41598-018-36091-y
Jin, P., Ding, J. C., Xing, T., Riebesell, U., and Gao, K. (2017). High levels of solar radiation offset impacts of ocean acidification on calcifying and non-calcifying strains of Emiliania huxleyi. Mar. Ecol. Prog. Ser. 568, 47–58. doi: 10.3354/meps12042
Jin, P., Gao, K., Villafañe, V. E., Campbell, D. A., and Helbling, E. W. (2013). Ocean acidification alters the photosynthetic responses of a coccolithophorid to fluctuating ultraviolet and visible radiation. Plant Physiol. 162, 2084–2094. doi: 10.1104/pp.113.219543
Jin, P., Wang, T., Liu, N., Dupont, S., Beardall, J., Boyd, P. W., et al. (2015). Ocean acidification increases the accumulation of toxic phenolic compounds across trophic levels. Nat. Commun. 6:8714. doi: 10.1038/ncomms9714
Johnson, V. R., Brownlee, C., Milazzo, M., and Hall-Spencer, J. M. (2015). Microalgal assemblage shift along a marine CO2 gradient subjected to multiple environmental stressors. J. Mar. Sci. Eng. 3, 1425–1447. doi: 10.3390/jmse3041425
Kang, J. W., and Chung, I. K. (2017). The effects of eutrophication and acidification on the ecophysiology of Ulva pertusa Kjellman. J. Appl. Phycol. 29, 2675–2683. doi: 10.1007/s10811-017-1087-5
Keeling, R. F., Körtzinger, A., and Gruber, N. (2010). Ocean deoxygenation in a warming world. Ann. Rev. Mar. Sci. 2, 199–229. doi: 10.1146/annurev.marine.010908.163855
Kerr, J. B., and McElroy, C. T. (1993). Evidence for large upward trends of ultraviolet-B radiation linked to ozone depletion. Science 262, 1032–1034. doi: 10.1126/science.262.5136.1032
Kim, M., Brodersen, K. E., Szabó, M., Larkum, A. W. D., Raven, J. A., and Peter, J. (2018). Low oxygen affects photophysiology and the level of expression of two-carbon metabolism genes in the seagrass Zostera muelleri. Photosyn. Res. 136, 147–160. doi: 10.1007/s11120-017-0452-1
Kitaya, Y., Azuma, H., and Kiyota, M. (2005). Effects of temperature, CO2/O2 concentrations and light intensity on cellular multiplication of microalgae Euglena gracilis. Adv. Space Res. 35, 1584–1588. doi: 10.1016/j.asr.2005.03.039
Kitaya, Y., Xiao, L., Masuda, A., Ozawa, T., Tsuda, M., and Omasa, K. (2008). Effects of temperature, photosynthetic photon flux density, photoperiod and O2 and CO2 concentrations on growth rates of the symbiotic dinoflagellate, Amphidinium sp. J. Appl. Phycol. 20, 737–742. doi: 10.1007/s10811-008-9331-7
Kliphuis, A. M., Martens, D. E., Janssen, M., and Wijffels, R. H. (2011). Effect of O2:CO2 ratio on the primary metabolism of Chlamydomonas reinhardtii. Biotechnol. Bioeng. 108, 2390–2402. doi: 10.1002/bit.23194
Korbee, N., Huovinen, P., Figueroa, F. L., Aguilera, J., and Karsten, U. (2005). Availability of ammonium influences photosynthesis and the accumulation of mycosporine-like amino acids in two Porphyra species (Bangiales, Rhodophyta). Mar. Biol. 146, 645–654. doi: 10.1007/s00227-004-1484-6
Kranz, S., Sultemeyer, D., Richter, K.-U., and Rost, B. (2009). Carbon acquisition by Trichodesmium: the effect of pCO2 and diurnal changes. Limnol. Oceanogr. 54, 548–559.
Kranz, S. A., Levitan, O., Richter, K. U., Prasil, O., and Berman-Frank, I. (2010). Combined effects of pCO2 and light on the N2 fixing cyanobacteria Trichodesmium IMS101: physiological responses. Plant Physiol. 154, 334–345. doi: 10.1104/pp.110.159145
Kremp, A., Godhe, A., Egardt, J., Dupont, S., Suikkanen, S., Casabianca, S., et al. (2012). Intraspecific variability in the response of bloom-forming marine microalgae to changed climate conditions. Ecol. Evol. 2, 1195–1207. doi: 10.1002/ece3.245
Levitan, O., Brown, C. M., Sudhaus, S., Campbell, D., LaRoche, J., and Berman-Frank, I. (2010a). Regulation of nitrogen metabolism in the marine diazotroph Trichodesmium IMS101 under varying temperatures and atmospheric CO2 concentrations. Environ. Microbiol. 12, 1899–1912. doi: 10.1111/j.1462-2920.2010.02195.x
Levitan, O., Kranz, S. A., Spungin, D., Prasil, O., and Rost, B. (2010b). The combined effects of pCO2 and light on the N2 fixing cyanobacterium Trichodesmium IMS101: a mechanistic view. Plant Physiol. 154, 346–356. doi: 10.1104/pp.110.159285
Levitus, S., Antonov, J. I., Boyer, T. P., and Stephens, C. (2000). Warming of the world ocean. Science 287, 2225–2229. doi: 10.1126/science.287.5461.2225
Li, F., Beardall, J., Collins, S., and Gao, K. (2017). Decreased photosynthesis and growth with reduced respiration in the model diatom Phaeodactylum tricornutum grown under elevated CO2 over 1800 generations. Glob. Change Biol. 23, 117–137. doi: 10.1111/gcb.13501
Li, F., Beardall, J., and Gao, K. (2018). Diatom performance in a future ocean: interactions between nitrogen limitation, temperature, and CO2-induced seawater acidification. ICES J. Mar. Sci. 75, 1451–1464. doi: 10.1093/icesjms/fsx239
Li, F., Wu, Y., Hutchins, D. A., Fu, F., and Gao, K. (2016). Physiological responses of coastal and oceanic diatoms to diurnal fluctuations in seawater carbonate chemistry under two CO2 concentrations. Biogeosciences 13, 6247–6259. doi: 10.5194/bg-13-6247-2016
Li, G., Gao, K. S., and Gao, G. (2011). Differential impacts of solar UV radiation on photosynthetic carbon fixation from the coastal to offshore surface waters in the South China Sea. Photochem. Photobiol. 87, 329–334. doi: 10.1111/j.1751-1097.2010.00862.x
Li, W., Ding, J., Li, F., Wang, T., Yang, Y., Li, Y., et al. (2019). Functional responses of smaller and larger diatoms to gradual CO2 rise. Sci. Total Environ. 680, 79–90. doi: 10.1016/j.scitotenv.2019.05.035
Li, W., and Gao, K. (2012). A marine secondary producer respires and feeds more in a high CO2 ocean. Mar. Pollut. Bull. 64, 699–703. doi: 10.1016/j.marpolbul.2012.01.033
Linares, C., Vidal, M., Canals, M., Kersting, D. K., Amblas, D., Aspillaga, E., et al. (2015). Persistent natural acidification drives major distribution shifts in marine benthic ecosystems. Proc. R. Soc. B Biol. Sci. 282:20150587. doi: 10.1098/rspb.2015.0587
Listmann, L., Leroch, M., Schlüter, L., Thomas, M. K., and Reusch, T. B. (2016). Swift thermal reaction norm evolution in a key marine phytoplankton species. Evol. Appl. 9, 1156–1164. doi: 10.1111/eva.12362
Liu, D., Keesing, J. K., He, P., Wang, Z., Shi, Y., and Wang, Y. (2013). The world’s largest macroalgal bloom in the Yellow Sea, China: formation and implications. Estuar. Coast. Shelf Sci. 129, 2–10. doi: 10.1016/j.ecss.2013.05.021
Liu, H., Chang, J., Tseng, C.-M., Wen, L.-S., and Liu, K. K. (2007). Seasonal variability of picoplankton in the Northern South China Sea at the SEATS station. Deep Sea Res. II 54, 1602–1616. doi: 10.1016/j.dsr2.2007.05.004
Liu, H., Chen, M., Zhu, F., and Harrison, P. J. (2016). Effect of diatom silica content on copepod grazing, growth and reproduction. Front. Mar. Sci. 3:89.
Lomas, M. W., Hopkinson, B. M., Ryan, J. L., Shi, D. L., Xu, Y., and Morel, F. M. M. (2012). Effect of ocean acidification on cyanobacteria in the subtropical North Atlantic. Aqu. Microb. Ecol. 66, 211–222. doi: 10.3354/ame01576
Luo, Y. W., Shi, D., Kranz, S. A., Hopkinson, B. M., Hong, H., Shen, R., et al. (2019). Reduced nitrogenase efficiency dominates response of the globally important nitrogen fixer Trichodesmium to ocean acidification. Nat. Commun. 10:1521. doi: 10.1038/s41467-019-09554-7
Mannfolk, A. (2016). Will Nitrogen Limitation and High CO2 Concentrations Impact Upon the Sinking Velocity of Phytoplankton? Ph.D. Thesis, Monash University, Melbourne.
Martin, S., and Hall-Spencer, J. M. (2017). “Effects of ocean warming and acidification on rhodolith/maërl beds,” in Rhodolith/Maërl Beds: A Global Perspective, eds R. Riosmena-Rodríguez, W. Nelson, and J. Aguirre (Cham: Springer), 55–85. doi: 10.1007/978-3-319-29315-8_3
Martínez, R. (2007). Effects of ultraviolet radiation on protein content, respiratory electron transport system (ETS) activity and superoxide dismutase (SOD) activity of Antarctic plankton. Polar Biol. 30, 1159–1172. doi: 10.1007/s00300-007-0273-3
McCabe, R. M., Hickey, B. M., Kudela, R. M., Lefebvre, K. A., Adams, N. G., Bill, B. D., et al. (2016). An unprecedented coastwide toxic algal bloom linked to anomalous ocean conditions. Geophys. Res. Lett. 43, 310–366.
Milligan, A. J., Varela, D. E., Brzezinski, M. A., and Morel, F. M. (2004). Dynamics of silicon metabolism and silicon isotopic discrimination in a marine diatomas a function of pCO2. Limnol. Oceanogr. 49, 322–329. doi: 10.4319/lo.2004.49.2.0322
O’Donnell, D. R., Hamman, C. R., Johnson, E. C., Klausmeier, C. A., and Litchman, E. (2017). Temperature selection drives evolution of function-valued traits in a marine diatom. bioRxiv
Orr, J. C., Fabry, V. J., Aumont, O., Bopp, L., Doney, S. C., Feely, R. A., et al. (2005). Anthropogenic ocean acidification over the twenty-first century and its impact on calcifying organisms. Nature 437, 681–686. doi: 10.1038/nature04095
Ossó, A., Sola, Y., Bech, J., and Lorente, J. (2011). Evidence for the influence of the North Atlantic Oscillation on the total ozone column at northern low latitudes and midlatitudes during winter and summer seasons. J. Geophys. Res. Atmos. 116:D24122.
Paul, C., Sommer, U., Garzke, J., Moustaka-Gouni, M., Paul, A., and Matthiessen, B. (2016). Effects of increased CO2 concentration on nutrient limited coastal summer plankton depend on temperature. Limnol. Oceanogr. 61, 853–868. doi: 10.1002/lno.10256
Pauly, D., and Christensen, V. (1995). Primary productivity required to sustain global fisheries. Nature 374, 255–257. doi: 10.1038/374255a0
Plummer, D., Scinocca, J., Shepherd, T., Reader, M., and Jonsson, A. (2010). Quantifying the contributions to stratospheric ozone changes from ozone depleting substances and greenhouse gases. Atmos. Chem. Phys. 10, 8803–8820. doi: 10.5194/acp-10-8803-2010
Pörtner, H.-O. (2008). Ecosystem effects of ocean acidification in times of ocean warming: a physiologist’s view. Mar. Ecol. Prog. Ser. 373, 203–217. doi: 10.3354/meps07768
Porzio, L., Buia, M. C., Ferretti, V., Lorenti, M., Rossi, M., Trifuoggi, M., et al. (2018). Photosynthesis and mineralogy of Jania rubens at low pH/high pCO2: a future perspective. Sci. Total Environ. 628, 375–383. doi: 10.1016/j.scitotenv.2018.02.065
Porzio, L., Buia, M. C., and Hall-Spencer, J. M. (2011). Effects of ocean acidification on macroalgal communities. J. Exp. Mar. Biol. Ecol. 400, 278–287. doi: 10.1016/j.jembe.2011.02.011
Qu, P., Fu, F. X., and Hutchins, D. A. (2018). Responses of the large centric diatom Coscinodiscus sp. to interactions between warming, elevated CO2, and nitrate availability. Limnol. Oceanogr. 63, 1407–1424. doi: 10.1002/lno.10781
Rajneesh, R., Chatterjee, A., Pathak, J., Ahmed, H., Singh, V., Singh, D. K., et al. (2018). “Ultraviolet radiation-induced DNA damage and mechanisms of repair in cyanobacteria: an overview,” in Biotechnology in Agriculture, Industry and Medicine. Trends in Life Science Research, eds R. P. Sinha and U. P. Shrivastava (New York, NY: Nova Biomedical), 169–218.
Raso, S., van Genugten, B., Vermuë, M., and Wijffels, R. H. (2012). Effect of oxygen concentration on the growth of Nannochloropsis sp. at low light intensity. J. Appl. Phycol. 24, 863–871. doi: 10.1007/s10811-011-9706-z
Rastrick, S. S., Graham, H., Azetsu-Scott, K., Calosi, P., Chierici, M., Fransson, A., et al. (2018). Using natural analogues to investigate the effects of climate change and ocean acidification on Northern ecosystems. ICES J. Mar. Sci. 75, 2299–2311. doi: 10.1093/icesjms/fsy128
Raven, J. A., and Beardall, J. (2005). “Respiration in Aquatic Photolithotrophs,” in Respiration in Aquatic Ecosystems, eds P. A. Del Giorgio and P. J. L. B. Williams (Oxford: Oxford University Press), 36–46. doi: 10.1093/acprof:oso/9780198527084.003.0003
Rhode, S. C., Pawlowski, M., and Tollrian, R. (2001). The impact of ultraviolet radiation on the vertical distribution of zooplankton of the genus Daphnia. Nature 412, 69–72. doi: 10.1038/35083567
Riebesell, U., Aberle-Malzahn, N., Achterberg, E. P., Algueró-Muñiz, M., Alvarez-Fernandez, S., Arístegui, J., et al. (2018). Toxic algal bloom induced by ocean acidification disrupts the pelagic food web. Nat. Clim. Change 8, 1082–1086. doi: 10.1038/s41558-018-0344-1
Riebesell, U., and Gattuso, J.-P. (2015). Lessons learned from ocean acidification research. Nat. Clim. Change 5, 12–14. doi: 10.1038/nclimate2456
Riebesell, U., Schulz, K. G., Bellerby, R., Botros, M., Fritsche, P., Meyerhöfer, M., et al. (2007). Enhanced biological carbon consumption in a high CO2 ocean. Nature 450:545. doi: 10.1038/nature06267
Riebesell, U., and Tortell, P. D. (2011). “Effects of ocean acidification on pelagic organisms and ecosystems,” in Ocean Acidification, eds J. P. Gattuso and L. Hansson (Oxford: Oxford University Press), 99–116.
Riebesell, U., Zondervan, I., Rost, B., Tortell, P. D., Zeebe, R. E., and Morel, F. M. (2000). Reduced calcification of marine plankton in response to increased atmospheric CO2. Nature 407, 364–367. doi: 10.1038/35030078
Ryther, J. H. (1969). Photosynthesis and fish production in the Sea. Science 66, 72–76. doi: 10.1126/science.166.3901.72
Sabine, C. L., Feely, R. A., Gruber, N., Key, R. M., Lee, K., Bullister, J. L., et al. (2004). The oceanic sink for anthropogenic CO2. Science 305, 367–371. doi: 10.1126/science.1097403
Schaum, C. E., Barton, S., Bestion, E., Buckling, A., Garcia-Carreras, B., Lopez, P., et al. (2017). Adaptation of phytoplankton to a decade of experimental warming linked to increased photosynthesis. Nat. Ecol. Evol. 1:0094. doi: 10.1038/s41559-017-0094
Schlüter, L., Lohbeck, K. T., Gutowska, M. A., Gröger, J. P., Riebesell, U., and Reusch, T. B. (2014). Adaptation of a globally important coccolithophore to ocean warming and acidification. Nat. Clim. Change 4, 1024–1030.
Schmidtko, S., Stramma, L., and Visbeck, M. (2017). Decline in global oceanic oxygen content during the 1017 past five decades. Nature 542, 335–339. doi: 10.1038/nature21399
Sett, S., Bach, L. T., Schulz, K. G., Koch-Klavsen, S., Lebrato, M., and Riebesell, U. (2014). Temperature modulates coccolithophorid sensitivity of growth, photosynthesis and calcification to increasing seawater pCO2. PLoS One 9:e88308. doi: 10.1371/journal.pone.0088308
Shelly, K., Heraud, P., and Beardall, J. (2002). Nitrogen limitation in Dunaliella tertiolecta Butcher (Chlorophyceae) leads to increased susceptibility to damage by ultraviolet-B radiation but also increased repair capacity. J. Phycol. 38, 1–8.
Shi, D., Hong, H., Su, X., Liao, L., Chang, S., and Lin, W. (2019). The physiological response of marine diatoms to ocean acidification: differential roles of seawater pCO2 and pH. J. Phycol. doi: 10.1111/jpy.12855 [Epub ahead of print].
Shi, D., Kranz, S. A., Kim, J.-M., and Morel, F. M. (2012). Ocean acidification slows nitrogen fixation and growth in the dominant diazotroph Trichodesmium under low-iron conditions. Proc. Natl. Acad. Sci. U.S.A. 109, E3094–E3100. doi: 10.1073/pnas.1216012109
Sinclair, B. J., Marshall, K. E., Sewell, M. A., Levesque, D. L., Willett, C. S., Slotsbo, S., et al. (2016). Can we predict ectotherm responses to climate change using thermal performance curves and body temperatures? Ecol. Lett. 19, 1372–1385. doi: 10.1111/ele.12686
Singh, S. P., Klisch, M., Sinha, R. P., and Häder, D.-P. (2008). Effects of abiotic stressors on synthesis of the mycosporine-like amino acid shinorine in the cyanobacterium Anabaena variabilis PCC 7937. Photochem. Photobiol. 84, 1500–1505. doi: 10.1111/j.1751-1097.2008.00376.x
Sinutok, S., Hill, R., Doblin, M. A., Wuhrer, R., and Ralph, P. J. (2011). Warmer more acidic conditions cause decreased productivity and calcification in subtropical coral reef sediment-dwelling calcifiers. Limnol. Oceanogr. 56, 1200–1212. doi: 10.4319/lo.2011.56.4.1200
Smetacek, V., and Zingone, A. (2013). Green and golden seaweed tides on the rise. Nature 504, 84–88. doi: 10.1038/nature12860
Sobrino, C., Neale, P. J., and Lubián, L. M. (2005). Interaction of UV radiation and inorganic carbon supply in the inhibition of photosynthesis: spectral and temporal responses of two marine picoplankters. Photochem. Photobiol. 81, 384–393. doi: 10.1111/j.1751-1097.2005.tb00198.x
Sobrino, C., Neale, P. J., Phillips-Kress, J. D., Moeller, R. E., and Portec, J. A. (2009). Elevated CO2 increases sensitivity to ultraviolet radiation in lacustrine phytoplankton assemblages. Limnol. Oceanogr. 54, 2448–2459. doi: 10.4319/lo.2009.54.6_part_2.2448
Sohm, J. A., Webb, E. A., and Capone, D. G. (2011). Emerging patterns of marine nitrogen fixation. Nat. Rev. Microbiol. 9, 499–508. doi: 10.1038/nrmicro2594
Staal, M., Rabouille, S., and Stal, L. J. (2007). On the role of oxygen for nitrogen fixation in the marine cyanobacterium Trichodesmium sp. Environ. Microbiol. 9, 727–736. doi: 10.1111/j.1462-2920.2006.01195.x
Summers, J. C., Kurek, J., Kirk, J. L., Muir, D. C., Wang, X., Wiklund, J. A., et al. (2016). Recent warming, rather than industrial emissions of bioavailable nutrients, is the dominant driver of lake primary production shifts across the athabasca oil sands region. PLoS One 11:e0153987. doi: 10.1371/journal.pone.0153987
Sunday, J. M., Fabricius, K. E., Kroeker, K. J., Anderson, K. M., Brown, N. E., Barry, J. P., et al. (2017). Ocean acidification can mediate biodiversity shifts by changing biogenic habitat. Nat. Clim. Change 7, 81–85. doi: 10.1038/nclimate3161
Tatters, A. O., Flewelling, L. J., Fu, F., Granholm, A. A., and Hutchins, D. A. (2013). High CO2 promotes the production of paralytic shellfish poisoning toxins by Alexandrium catenella from Southern California waters. Harmful Algae 30, 37–43. doi: 10.1016/j.hal.2013.08.007
Tatters, A. O., Fu, F. X., and Hutchins, D. A. (2012). High CO2 and silicate limitation synergistically increase the toxicity of Pseudo-nitzschia fraudulenta. PLoS One 7:e32116. doi: 10.1371/journal.pone.0032116
Tedetti, M., and Sempéré, R. (2006). Penetration of ultraviolet radiation in the marine environment. a review. Photochem. Photobiol. 82, 389–397.
Thomas, M. K., Kremer, C. T., Klausmeie, C. A., and Litchman, E. (2012). A global pattern of thermal adaptation in marine phytoplankton. Science 338:1085. doi: 10.1126/science.1224836
Tong, S., Gao, K., and Hutchins, D. A. (2018). Adaptive evolution in the coccolithophore Gephyrocapsa oceanica following 1,000 generations of selection under elevated CO2. Glob. Change Biol. 2, 3055–3064. doi: 10.1111/gcb.14065
Vaquer-Sunyer, R., and Duarte, C. M. (2008). Thresholds of hypoxia for marine biodiversity. Proc. Natl. Acad. Sci. U.S.A. 105, 15452–15457. doi: 10.1073/pnas.0803833105
Villafane, V. E., Banaszak, A. T., Guendulain-Garcia, S. D., Strauch, S. M., Halac, S. R., and Helbling, E. W. (2013). Influence of seasonal variables associated with climate change on photochemical diurnal cycles of marine phytoplankton from Patagonia (Argentina). Limnol. Oceanogr. 58, 203–214. doi: 10.4319/lo.2013.58.1.0203
Walworth, N. G., Fu, F.-X., Webb, E. A., Saito, M. A., Moran, D., Mcllvin, M. R., et al. (2016a). Mechanisms of increased Trichodesmium fitness under iron and phosphorus co-limitation in the present and future ocean. Nat. Commun. 7:12081. doi: 10.1038/NCOMMS12081
Walworth, N. G., Lee, M. D., Fu, F.-X., Hutchins, D. A., and Webb, E. A. (2016b). Molecular and physiological evidence of genetic assimilation to high CO2 in the marine nitrogen fixer Trichodesmium. Proc. Natl. Acad. Sci. U.S.A. 11, E7367–E7374.
Wang, G., Jing, W., Wang, S., Xu, Y., Wang, Z., Zhang, Z., et al. (2014). Coastal acidification induced by tidal-driven submarine groundwater discharge in a coastal coral reef system. Environ. Sci. Technol. 48, 13069–13075. doi: 10.1021/es5026867
Wannicke, N., Endres, S., Engel, A., Grossart, H.-P., Nausch, M., Unger, J., et al. (2012). Response of Nodularia spumigena to pCO2–part 1: growth, production and nitrogen cycling. Biogeosciences 9, 2973–2988. doi: 10.5194/bg-9-2973-2012
Wassmann, P. (2015). Overarching perspectives of contemporary and future ecosystems in the Arctic Ocean. Prog. Oceanogr. 139, 1–12. doi: 10.1016/j.pocean.2015.08.004
Whitney, F. A., Freeland, H. J., and Robert, M. (2007). Persistently declining oxygen levels in the interior waters of the eastern subarctic Pacific. Prog. Oceanogr. 75, 179–199. doi: 10.1016/j.pocean.2007.08.007
Williams, G. A., Helmuth, B., Russell, B. D., Dong, Y.-W., Thiyagarajan, V., and Seuront, L. (2016). Meeting the climate change challenge: Pressing issues in southern China and SE Asian coastal ecosystems. Reg. Stud. Mar. Sci. 8, 373–381. doi: 10.1016/j.rsma.2016.07.002
Williamson, C. E., Neale, P. J., Hylander, S., Rose, K. C., Figueroa, F. L., Robinson, S. A., et al. (2019). The interactive effects of stratospheric ozone depletion, UV radiation, and climate change on aquatic ecosystems. Photochem. Photobiol. Sci. 18, 717–746. doi: 10.1039/c8pp90063a
Williamson, C. E., Zepp, R. G., Lucas, R. M., Madronich, S., Austin, A. T., Ballaré, C. L., et al. (2014). Solar ultraviolet radiation in a changing climate. Nat. Clim. Change 4, 434–441.
Wu, Y., Campbell, D. A., Irwin, A. J., Suggett, D. J., and Finkel, Z. V. (2014). Ocean acidification enhances the growth rate of larger diatoms. Limnol. Oceanogr. 59, 1027–1034. doi: 10.4319/lo.2014.59.3.1027
Wu, Y., Li, Z., Du, W., and Gao, K. (2015). Physiological response of marine centric diatoms to ultraviolet radiation, with special reference to cell size. J. Photochem. Photobiol. B 153, 1–6. doi: 10.1016/j.jphotobiol.2015.08.035
Xiu, P., Chai, F., Curchitser, E. N., and Castruccio, F. S. (2018). Future changes in coastal upwelling ecosystems with global warming: The case of the California Current System. Sci. Rep. 8:2866. doi: 10.1038/s41598-018-21247-7
Xu, K., Fu, F. X., and Hutchins, D. A. (2014). Comparative responses of two dominant Antarctic phytoplankton taxa to interactions between ocean acidification, warming, irradiance, and iron availability. Limnol. Oceanogr. 59, 1919–1931. doi: 10.4319/lo.2014.59.6.1919
Xu, Z., Gao, G., Xu, J., and Wu, H. (2017). Physiological response of a golden tide alga (Sargassum muticum) to the interaction of ocean acidification and phosphorus enrichment. Biogeosciences 14, 671–681. doi: 10.5194/bg-14-671-2017
Xu, Z., and Gao, K. (2009). Impacts of UV radiation on growth and photosynthetic carbon acquisition in Gracilaria lemaneiformis (Rhodophyta) under phosphorus-limited and replete conditions. Funct. Plant Biol. 36, 1057–1064.
Xu, Z., and Gao, K. (2012). enrichment and UV radiation interact to affect the photosynthesis and nitrogen uptake of Gracilaria lemaneiformis (Rhodophyta). Mar. Pollut. Bull. 64, 99–105. doi: 10.1016/j.marpolbul.2011.10.016
Yildiz, G., Hofmann, L. C., Bischof, K., and Dere, Ş (2013). Ultraviolet radiation modulates the physiological responses of the calcified rhodophyte Corallina officinalis to elevated CO2. Bot. Mar. 56, 161–168.
Zark, M., Riebesell, U., and Dittmar, T. (2015). Effects of ocean acidification on marine dissolved organic matter are not detectable over the succession of phytoplankton blooms. ScienceAdvances 1:e1500531. doi: 10.1126/sciadv.1500531
Zhai, W., Zhao, H., Zheng, N., and Xu, Y. (2012). Coastal acidification in summer bottom oxygen-depleted waters in northwestern-northern Bohai Sea from June to August in 2011. Chin. Sci. Bull. 57, 1062–1068. doi: 10.1007/s11434-011-4949-2
Zheng, Y., and Gao, K. (2009). Impacts of solar UV radiation on the photosynthesis, growth and UV-absorbing compounds in Gracilaria lemaneiformis (Rhodophyta) grown at different nitrate concentrations. J. Phycol. 45, 314–323. doi: 10.1111/j.1529-8817.2009.00654.x
Zhu, Z., Fu, F.-X., Qu, P., Mak, E. W. K., Jiang, H., Zhang, R., et al. (2019). Interactions between ultraviolet radiation exposure and phosphorus limitation in the marine nitrogen-fixing cyanobacteria Trichodesmium and Crocosphaera. Limnol. Oceanogr.
Ziveri, P., Passaro, M., Incarbona, A., Milazzo, M., Rodolfo-Metalpa, R., and Hall-Spencer, J. M. (2014). Decline in coccolithophore diversity and impact on coccolith morphogenesis along a natural CO2 gradient. Biol. Bull. 226, 282–290. doi: 10.1086/bblv226n3p282
Keywords: algae, global warming, light, multiple stressors, nutrients, hypoxia, phytoplankton, primary productivity
Citation: Gao K, Beardall J, Häder D-P, Hall-Spencer JM, Gao G and Hutchins DA (2019) Effects of Ocean Acidification on Marine Photosynthetic Organisms Under the Concurrent Influences of Warming, UV Radiation, and Deoxygenation. Front. Mar. Sci. 6:322. doi: 10.3389/fmars.2019.00322
Received: 01 April 2019; Accepted: 28 May 2019;
Published: 18 June 2019.
Edited by:
Elizabeth Fulton, Commonwealth Scientific and Industrial Research Organisation (CSIRO), AustraliaReviewed by:
Laurie Carol Hofmann, Alfred Wegener Institute, Helmholtz Centre for Polar and Marine Research (AWI), GermanyShiguo Li, Research Center for Eco-Environmental Sciences (CAS), China
Copyright © 2019 Gao, Beardall, Häder, Hall-Spencer, Gao and Hutchins. This is an open-access article distributed under the terms of the Creative Commons Attribution License (CC BY). The use, distribution or reproduction in other forums is permitted, provided the original author(s) and the copyright owner(s) are credited and that the original publication in this journal is cited, in accordance with accepted academic practice. No use, distribution or reproduction is permitted which does not comply with these terms.
*Correspondence: Kunshan Gao, a3NnYW9AeG11LmVkdS5jbg==