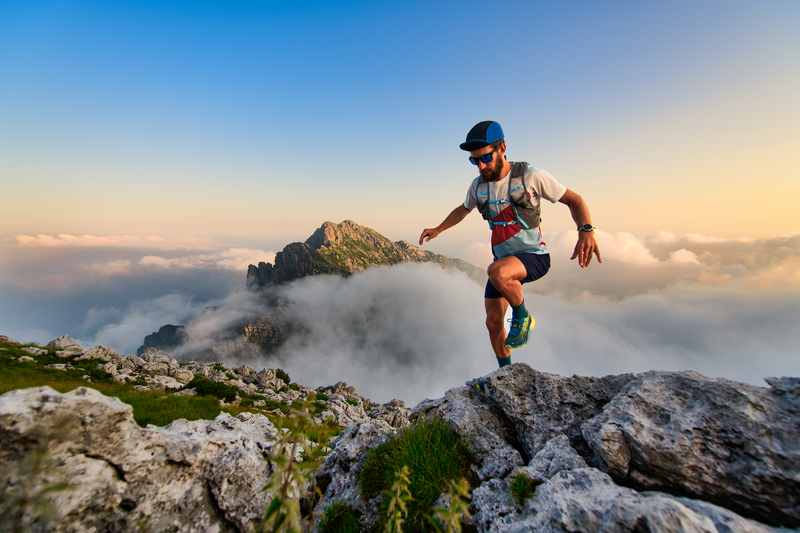
95% of researchers rate our articles as excellent or good
Learn more about the work of our research integrity team to safeguard the quality of each article we publish.
Find out more
ORIGINAL RESEARCH article
Front. Mar. Sci. , 05 June 2019
Sec. Global Change and the Future Ocean
Volume 6 - 2019 | https://doi.org/10.3389/fmars.2019.00293
This article is part of the Research Topic Carbon Bridge to the Arctic View all 20 articles
The Arctic Ocean is changing rapidly with respect to ice cover extent and volume, growth season duration and biological production. Zooplankton are important components in the arctic marine food web, and tightly coupled to the strong seasonality in primary production. In this study, we investigate zooplankton composition, including microzooplankton, copepod nauplii, as well as small and large copepod taxa, and primary productivity in the dynamic Atlantic water inflow area north of Svalbard in May and August 2014. We focus on seasonal differences in the zooplankton community and in primary productivity regimes. More specifically, we examine how a shift from “new” (nitrate based) spring bloom to a “regenerated” (ammonium based) post bloom primary production is reflected in the diversity, life history adaptations and productivity of the dominant zooplankton. North of Svalbard, the seasonal differences in planktonic communities were significant. In spring, the large copepod Calanus finmarchicus dominated, but the estimated production and ingestion rates were low compared to the total primary production. In summer, the zooplankton community was composed of microzooplankton and the small copepod Oithona similis. The zooplankton production and ingestion rates were high in summer, and probably depended heavily on the regenerated primary production associated with the microbial loop. There was clear alteration from dominance of calanoid copepod nauplii in spring to Oithona spp. nauplii in summer, which indicates different reproductive strategies of the dominating large and small copepod species. Our study confirms the dependence and tight coupling between the new (spring bloom) primary production and reproductive adaptations of C. glacialis and C. hyperboreus. In contrast, C. finmarchicus appears able to take advantage of the regenerated summer primary production, which allows it to reach the overwintering stage within one growth season in this region north of Svalbard. This suggests that C. finmarchicus will be able to profit from the predicted increased primary production in the Arctic, a strategy also recognized in small copepod species such as O. similis. We speculate that the ability of the copepod species to utilize the regenerated summer primary production and microbial food web may determine the winners and losers in the future Arctic Ocean.
The extreme seasonality of polar marine ecosystems is widely recognized. During winter, the sun is below the horizon (polar night) and the lack of light prevents phytoplankton growth. In seasonally ice-covered regions, the spring bloom of primary producers usually initiates after sea ice melting and lasts only a few weeks, until the surface nitrate is depleted. When nitrate is depleted and stratification prevents new influx of nitrate, phytoplankton will use alternative nitrogen (N) sources, such as ammonium and urea (Kristiansen et al., 1994). The shift from “new” nitrate (NO3-) to regenerated forms of N such as ammonium (NH4+) is known as the dichotomy of “new” and “regenerated” primary production [sensu Dugdale and Goering (1967)], respectively. The fraction of new primary production to total (new and regenerated) primary production is defined by the f-ratio. From the perspective of the grazer communities, the source of nitrogen triggers different autotrophic communities (Shilova et al., 2017). The nutrient replete spring-scenario is typically dominated by large phytoplankton cells (such as diatoms) utilizing nitrate as their N source, and the post bloom phytoplankton community is often dominated by smaller cells that grow efficiently on recycled N and dissolved organic carbon (Paulsen et al., 2018). This transition from spring bloom to post bloom is also associated with a change in phytoplankton lipid composition, with higher contributions of the essential polyunsaturated fatty acids (PUFAs) during spring bloom than during post bloom (Parrish et al., 2005; Leu et al., 2006).
The strong seasonality in food quality and quantity has direct implications for the grazer communities. Most obvious is perhaps the direct effect on the large herbivorous copepods, with life cycles tailored to utilize the short and intense spring bloom for reproduction and lipid synthesis (Falk-Petersen et al., 2009). For example, the large Arctic Calanus hyperboreus reproduce in winter, prior to the productive season (Falk-Petersen et al., 2009; Kvile et al., 2018), C. glacialis reproduce prior to and during the ice algae bloom (Varpe et al., 2009; Søreide et al., 2010) and C. finmarchicus has its main reproductive period during the open water spring bloom (Hirche, 1996; Pedersen et al., 2001). When the large Calanus species have built sufficient lipid storages, they enter diapause at depth to survive the long and less productive winter season. When leaving the surface habitat, a niche is created for the smaller copepod species with different life history strategies (Hansen et al., 1999; Svensen et al., 2011). Therefore, the shift in major primary productivity regimes from spring to summer can also be reflected in the grazer communities both with respect to feeding and reproductive strategies. While a number of studies at high latitudes focus on the zooplankton community composition and life history adaptations during the ice algae- and open water spring bloom (Søreide et al., 2010; Leu et al., 2011; Feng et al., 2016), there has been less focus on links between the zooplankton and microbial food webs at the end of the summer when the large Calanus spp. leave the surface waters (Hansen et al., 1999; Svensen et al., 2011). Likewise, small copepod taxa, nauplii and microzooplankton are often not well represented due to predominant use of plankton nets targeting the larger size-fraction of the plankton community. Presently, the Arctic climate is undergoing rapid changes with potential severe effects on the ecosystem. With an already documented earlier sea ice-melt and delayed sea-ice formation in the Barents Sea and Arctic Ocean (Onarheim et al., 2018), the future Arctic Ocean is expected to experience an increase in open water area, increased light transmission to the surface ocean, and a prolonged growing season for phytoplankton (Arrigo and Van Dijken, 2011). A 20% increase of total annual net primary production from 1998–2009 has already been documented (Arrigo and Van Dijken, 2011). However, it is not clear if this increase is based on new or regenerated production. During summer with stratified water masses, a large fraction of the increased production is likely to be fueled by regenerated nutrients (Randelhoff et al., 2016). A direct consequence is a shift from larger to smaller phytoplankton cells (Li et al., 2009), which again will affect the composition of the grazers. The seasonal shift from new to regenerated production and the consequences for zooplankton life history adaptations has not received sufficient attention in Arctic regions.
We investigate seasonal differences in the zooplankton community and in the primary productivity regimes in the Atlantic water inflow area north of Svalbard. Also, we evaluate how a shift from “new” (nitrate based) spring bloom to a “regenerated” (ammonium based) post bloom situation is reflected in the diversity, life history adaptations and productivity of the major zooplankton. We approach this by investigating the composition of the total zooplankton community in the upper 100 m in May and August and by evaluating estimated production and ingestion rates of the main grazers in light of new and regenerated primary production in this area. By applying different zooplankton sampling tools that catches both the large (MultiNet) and small (Go-Flo water samplers) copepods, as well as microzooplankton (Niskin type water samplers), we present a more comprehensive picture of the zooplankton community in spring and summer, taking into account the role of zooplankters representing a wider spectrum of size fractions.
This study was conducted at six “process stations” (where the ship stayed at the station for 30 h to allow rate measurements), located in the Atlantic inflow area north of Svalbard in May (P1, P3, P4) and August (P5, P6, P7) in 2014 (Table 1 and Figure 1). In both study periods, the stations were located along the ice edge, and we aimed for sampling as far north and east as possible without breaking far into the fast ice (Figure 1). Due to adverse ice conditions, only stations P1 and P5 represent one spatial location sampled twice (P1 sampled in May and P5 in August), but in this study we focus more on seasonal than spatial differences. This dynamic area, following the continental slope north and west of Svalbard, is characterized by advection of warm, saline and nutrient-rich Atlantic Water (Randelhoff et al., 2016, 2018; Renner et al., 2018). The strong influx of warm Atlantic water makes this area relatively ice-free. The ice-extent during our study was variable, ranging from 0% at P5 to 90% at P6 in August (Figure 1) and the distribution of drift ice was strongly influenced by wind fields (Randelhoff et al., 2018).
Table 1. Overview of process stations in May and August 2014, providing date sampled, latitude, and longitude at the arrival of the station and depth at the start (arrival) and end of the station.
Figure 1. Map of the Atlantic inflow area north of Svalbard, showing the ice extension and sampled stations in May and August. Maps were generated using GSHHG data from the National Oceanic and Atmospheric Administration (US) and ice data were provided by the Norwegian Ice Service (MET Norway) for the dates May 23, 2014 and August 12, 2014. The maps were modified from Wilson et al. (2017).
Hydrographic properties of the water column were obtained with a CTD (conductivity, temperature, depth) sensor system (Seabird SBE-911 plus) mounted on a General Oceanics rosette sampler, equipped with 8-L Niskin bottles and a Seapoint Fluorometer. Physical (temperature, salinity, density, photosynthetically available radiation, PAR) and biochemical properties (inorganic nutrients, fugacity of CO2), of the water column were obtained for all stations, and are presented elsewhere (Randelhoff et al., 2018). In this paper, to characterize the environment, we present only the temperature within the upper 100 m of the water column where the bulk of the primary production processes take place. In May, stations P1, P3, and P4 were relatively similar with regard to temperature, with surface temperatures (0–10 m) between -1 and 1°C. Warm Atlantic water was found below 10 depth, with temperatures from 2.5 to 3.5°C (Figure 2). In August, the water at station P5 was warm, 6°C, and the water column was mixed within the 0–100 m. At station P6, a layer of cold water <-1°C was found in the upper 50 m, on top of warmer Atlantic water. At station P7, the cold layer was restricted to the upper 10 m (Figure 2).
Figure 2. Temperature (°C) in the upper 100 m at stations P1, P3, and P4 in May and P5, P6, and P7 in August 2014.
Water samples for particulate organic carbon (POC) and chlorophyll a (Chl a) were collected with Niskin water bottles from 1, 5, 10, 20, 30, 40, 50, 75, 100, and 200 m depth. Triplicate subsamples of 100–500 mL were filtered onto pre-combusted Whatman GF/F filters for POC, while triplicate subsamples of 5–300 mL were filtered onto Whatman GF/F filters for Chl a concentration measurements. The POC and Chl a filters were analyzed according to procedures described in Paulsen et al. (2018). For each station, we present the POC and Chl a concentration in the upper 100 m as integrated values (by trapezoid integration).
Primary production rates were measured using the 14C method (Steemann Nielsen, 1952). Seawater was sampled at 1, 5, 10, 15, and 30 m to characterize the water mass both within and below the mixed layer (9–15 m) (Randelhoff et al., 2018). Samples were incubated in situ by deploying the experimental bottles attached to a line that was anchored to an ice floe. At each depth, two light bottles and one dark bottle were incubated for approximately 22 h. Ten μCuries of 14C-labelled bicarbonate was dispensed into each bottle, and a Time Zero bottle filtered immediately in order to account for adsorption processes. In addition, for each depth, a 100 μL aliquot was sampled into a 6 mL scintillation vial in order to estimate the initial 14C-bicarbonate concentration by fixing 14C with 0.1 mL 6N NaOH. After the incubation, 200 μL of 20% HCl was dispensed into each scintillation vial containing 2 mL of seawater in order to release any inorganic 14C remaining in the sample. After 24 h, 5 ml of Ultima Gold (Perkin Elmer, United States) was added and the samples stored in the dark until 14C activity was measured with a Perkin Elmer scintillation counter. Primary production was calculated as 14C incorporation into the sample, measured in units of disintegrations per minute (Vernet et al., 1998). Dissolved inorganic carbon was measured in every sample, and 1.05 was used as the discrimination factor between incorporation of 14C and 12C. The 14C incorporation in the light bottle was corrected by subtracting the 14C incorporation in the dark bottle.
New and regenerated primary production was estimated by experimental determination of phytoplankton uptake of nitrate (NO3-) and ammonium (NH4+), respectively. The uptake measurements were conducted by incubation experiments during both cruises, as described in Randelhoff et al. (2016). From the uptake ratios of nitrate and ammonium, the f-ratio was calculated, defined as the fraction of nitrate (NO3-) uptake to the total N uptake (NO3- + NH4+). Hence, an f-ratio of 1 means that all the production can be considered as “new” (nitrate-based) while an f-ratio of 0 imply that all the production was “regenerated.”
In this study we use the term microzooplankton sensu lato, defined as grazers in 15–300 μm size, including phagotrophic ciliates, dinoflagellates, and sarcodines with or without functional chloroplasts. Thus, the functional role of microzooplankton in this study is associated with activity of protists. Microzooplankton were collected within the upper 100 m using 8L Niskin bottles. Samples were preserved in 2% (final concentration) acid Lugol’s iodine, stored at 4°C and post-fixed with 1% formaldehyde (final concentration). Additional samples for determination of pigmented microzooplankton were preserved in 1% formaldehyde. In the laboratory, microzooplankton were settled onto Utermöhl chambers (50–100 ml) and enumerated by scanning the entire surface area of the chamber at 200×. Microzooplankton cells were sized with an eyepiece micrometer at 400–600× and converted to carbon based on approximated geometric shapes and volume-carbon conversions (Putt and Stoecker, 1989; Menden-Deuer and Lessard, 2000). All ciliates were included in microzooplankton, whereas dinoflagellates <15 μm in maximum dimension were not. Additionally, microzooplankton cells were examined for chloroplasts in formaldehyde-preserved samples using differential interference contrast and chlorophyll autofluorescence and allocated into heterotrophs and mixotrophs (i.e., pigmented ciliates and dinoflagellates). For details on microzooplankton analysis see Lavrentyev et al. (2019).
Mesozooplankton were sampled at all six stations, with a special focus on the relative contribution of large and small copepods and nauplii. We define mesozooplankton as multicellular heterotrophic organisms, but in this study, we focus on the role of Copepoda. Hence, the fraction mesozooplankton here includes only members of this subclass, ranging from nauplii (lower size approx. 0.09 mm; first nauplii of Microsetella norvegica) to adult copepods (upper size 12.0 mm; adult females of Paraeuchaeta barbata). Within the group “large copepods,” species with an adult body size > 2 mm are included. This embraces Calanus finmarchicus, C. glacialis, and C. hyperboreus, with their developmental stages from CI to adult. Less common large copepods (mainly Metridia spp., Pseudocalanus spp., Paraeuchaeta spp.) were grouped as “other large.” The group termed “small copepods” includes only Oithona spp. (predominantly Oithona similis) and the remaining smaller taxa (e.g., Triconia borealis, Microcalanus spp., and Microsetella norvegica) were grouped as “other small.” Copepod nauplii were divided in two groups, calanoid copepod nauplii (predominantly Calanus spp.) and Oithona spp. nauplii.
To obtain robust data both on smaller and larger size-groups of mesozooplankton (here copepods), we used two different sampling approaches. Large copepods were collected with a MultiNet plankton sampler type Midi (Hydro-Bios, Germany, net aperture area 0.25 m2), which was equipped with net bags with 180 μm mesh gauze, and was towed vertically in the depth-intervals 0–20, 20–50, 50–100, 100–200 m and 200-bottom. The content of each cod-end was concentrated on a 180 μm meshed sieve and transferred to polycarbonate bottles. Small copepods and nauplii were collected with Go-Flo water bottles (General Oceanic, volume 30 L) at 1, 10, 20, 30, 50, and 100 m depth. The water samples collected with Go-Flo bottle were emptied with a silicon tube and the content collected on a 20 μm mesh sieve. All mesozooplankton samples were preserved with buffered formaldehyde at 4% final concentration.
The mesozooplankton samples, both collected with MultiNet and Go-Flo bottle, were identified and counted in the laboratory on land, using Olympus stereoscopic microscopes with 7–90× magnification, and following standard sub-sampling procedure (Postel et al., 2000). Each sample was first scanned for macrozooplankton (organisms with total length > 0.5 cm), which were picked out, identified and counted in the entire sample. Mesozooplankton was identified and counted in subsamples (2 ml in volume), taken from the fixed sample volume (typically between 100 and 200 ml) using a macropipette (an equivalent of the Stempel pipette), and all organisms in each subsample were identified and counted. The number of subsamples was determined individually to count at least 500 individuals per sample. However, in this paper we focus on the copepods, which were the dominating (in terms of abundance and biomass), component of the mesozooplankton fraction. Representatives of Calanus were identified to the species level based on the description given in Kwasniewski et al. (2003). We are aware that distinguishing the species C. finmarchicus, C. glacialis, and C. hyperboreus based on morphology is associated with some uncertainty because prosome lengths of the three species can be overlapping (Choquet et al., 2018).
Copepod contribution to the plankton community was expressed in terms of carbon (biomass), by converting prosome lengths, using individual dry mass data and carbon to dry mass relationships from the literature (Supplementary Table 1).
In May, the integrated (0–50 m) total particulate primary production was generally high, ranging from 0.34 g C m-2 d-1 at P4 to 0.85 g C m-2 d-1 at P1 (Figure 3). In August, the total primary production ranged from 0.19 g C m-2 d-1 at P5 to 0.70 g C m-2 d-1 at P7 (Figure 3). The f-ratio, i.e., the fraction of “new” to total (new + regenerated) primary production, ranged from 0.6 to 0.9 in May and was below 0.007 at all stations in August (Figure 3). Hence, the primary production in May was dominated by “new production,” while in August the primary production was predominantly “regenerated.”
Figure 3. Total, integrated (0–50 m) particulate primary production (mg C m-2 d-1), measured by the 14C uptake method, in May and August (columns) and the calculated f-ratio at each station.
The 0–100 m integrated biomass of POC in May was 12, 23, and 17 g C m-2 at P1, P3, and P4, respectively (Figure 4). In August it ranged from 8 to 10 g C m-2, and was hence less variable between stations. The ratio of POC to chlorophyll a (Chl a) increased from 40–70 in May to 100–200 in August (Figure 4), pointing to a more autotrophic community in May than in August.
Figure 4. Particulate organic carbon, POC (g m-2), integrated in the upper 100 m and the mean ratio of POC to Chl a (POC/Chl a) at all stations.
The integrated (0–100 m) total microzooplankton biomass ranged from 0.25 to 0.39 g C m-2 in May. Ciliates, considering both heterotrophic and mixotrophic taxa, represented between 90 and 66% of the total microzooplankton biomass at P1 and P4, respectively (Figure 5). In August, the integrated biomass was significantly higher at all stations (1.2–1.4 g C m-2) and reached the highest value at P5 (Figure 5). Ciliates and dinoflagellates contributed equally to the total microzooplankton biomass representing on average 45 and 55%, respectively. Mixotrophic taxa, including both ciliates and dinoflagellates, contributed between 55 and 82% to the total microzooplankton biomass both seasons. P4 (sampled in May) was the only station where the heterotrophic taxa were dominant (59%). For detailed information on microzooplankton community composition, see Lavrentyev et al. (2019).
Figure 5. Microzooplankton biomass (g C m-2), integrated in the upper 100 m. Bars show contributions of mixotrophic (MCIL) and heterotrophic (HCIL) ciliates and mixotrophic (MDIN) and heterotrophic (HDIN) dinoflagellates.
Numerically, the mesozooplankton copepod community in May was dominated by calanoid copepod nauplii (predominantly Calanus spp.; Figure 6). In contrast, in August small copepods and Oithona spp. nauplii prevailed (Figure 6). The highest total abundances of copepods and nauplii were found at station P6, with almost 4 000 × 103 individuals m-2 in the 0–100 m depth interval. Compared to the other groups, the abundance of large copepods was negligible in May and August (Figure 6). However, in terms of biomass, the large copepods were important, especially in May. The integrated biomass of the large copepods, small copepods and nauplii ranged from 1.7 to 2.8 g C m-2 in May and from 1.3 to 2.4 g C m-2 in August (Figure 6). Although the biomass contribution of the large copepods was overall substantial, calanoid nauplii and small copepods also contributed considerably to the total copepod biomass in May and August, respectively (Figure 6).
Figure 6. Integrated (0–100 m) abundance (103 individuals m-2, upper panel) and biomass (g C m-2, lower panel) of large copepods, small copepods, calanoid copepod nauplii (predominantly Calanus spp.), and Oithona spp. nauplii.
In terms of species composition, the large copepods were numerically dominated by C. finmarchicus both in May and August (Figure 7). In May, the biomass of C. hyperboreus was substantial, but in August C. finmarchicus made up the largest fraction of the biomass of the large copepods (Figure 7). At all six stations, the small copepods were dominated by O. similis, both in terms of abundance and biomass (Figure 7).
Figure 7. Integrated (0–100 m) abundance (103 ind m-2, upper panel) and biomass (g C m-2, lower panel) of large copepods (dominated by C. finmarchicus, C. glacialis, C. hyperboreus) and small copepods (dominated by O. similis). The group “other large” consisted mainly of Pseudocalanus spp., and the group “other small” consisted mainly of Triconia borealis. Note different scales on the y-axes.
The majority of the population of all three Calanus species stayed in the upper 100 m in May (Table 2). In August, the majority of the C. finmarchicus and C. glacialis older copepodids (CV and females) were situated below 100 m, while the young stages CI–CIV were still mostly inhabiting the upper water layers. Except for some CV copepodids in the surface at station P5, the whole population of C. hyperboreus was found below 100 m in August (Table 2).
In May, all stages (except males) of C. finmarchicus were present, although in low abundances. In August, the population consisted mostly of young stages CI–CIII, and it had increased in abundance nearly four times (except for station P5, Figure 8). The population of C. glacialis, which was in general four times less numerous than the population of C. finmarchicus, was completely dominated by younger stages CI–CIII in May, with a few females also present. By August, the population was dominated by older developmental stages CIV–CV, and its abundance decreased pronouncedly (Figure 8).
Figure 8. Abundance (individuals m-2) and stage composition of the dominating copepod species: C. finmarchicus, C. glacialis, C. hyperboreus, and O. similis, and abundance of calanoid copepod nauplii (predominantly Calanus spp.) and Oithona spp. nauplii in May and August. Note different scales on the y-axes.
Oithona similis was overall the numerically dominating copepod species. In May, the population was dominated by females, although all other copepodid stages were also found. In August, the population size had increased substantially. The younger stages CI–CIII contributed the most, but copepodids CIV, CV and females made up nearly the other halve of the population (Figure 8).
The total abundance of copepod nauplii was exceptionally high both in May (500 000–1 500 000 nauplii m-2) and in August (800–2 500 000 nauplii m-2) in the 0–100 m water column. However, in May there was a complete dominance of calanoid copepod nauplii (mostly of Calanus spp.), while in August there were few calanoid nauplii and the nauplii stock was totally dominated by Oithona spp. nauplii (Figure 8).
In the Atlantic water inflow area north of Svalbard, the plankton community displayed a strong seasonality during the two investigated periods. Although the mean particulate primary production (as measured by 14C uptake) was high both in May (578 ± 257 mg C m-2 d-1) and in August (370 ± 288 mg C m-2 d-1), the associated plankton communities were different. In May, we observed an intensive ice-edge spring bloom based on nitrate and with high f-ratio (0.7–0.9) and the dominance of Phaeocystis pouchetii and large diatoms (Randelhoff et al., 2016). However, the stations were at different stages of the bloom succession: growing bloom (P1), peak bloom (P3) and decaying bloom (P4) (Paulsen et al., 2018). In August, a post bloom situation was seen at all stations (P5, P6, P7), with low f-ratios (0.001–0.007) and a phytoplankton community dominated by small flagellates. Hence, the two sources of N (nitrate and ammonia) were associated with different microbial communities, which represent different food quality for the grazers. The different pools of N have also different sources and rates of productivity and turnover. While nitrate must be added to surface water through external processes such as upwelling or turbulent diffusion across the pycnocline, ammonia is entering the system through internal biological processes such as regeneration by heterotrophic bacteria, and release by zooplankton (Kristiansen et al., 1994; Legendre and Rassoulzadegan, 1995; Shilova et al., 2017). In a study conducted simultaneously with the present one, Randelhoff et al. (2016) examined seasonal vertical nitrate fluxes in relation to upper ocean stratification at the process stations P1–P7. The authors highlight the importance of turbulent diffusion across the pycnocline as the main pathway for nutrient supply to a post bloom ocean surface. For our study area, the authors found that upwelling in this area is not very likely during summer, and the upward turbulent nitrate fluxes across the seasonal nitracline are small (Randelhoff et al., 2016). This supports our finding that the relatively high carbon production occurring during post bloom in August was based on regenerated nutrients.
The nutrient dynamics and uptake rates, along with the phytoplankton community composition and primary production rates, both suggest that the grazer communities had to face a strong seasonal shift in their food stock. In the following, we discuss how the seasonal shift at the base of the food web from new production in spring to a post bloom, regenerated production, affect the seasonal patterns of the major grazers. The focus on the dominant copepod species and microzooplankton in the upper 100 m allowed us to link productivity patterns with the active (non-hibernating) part of the planktonic populations.
The large copepod species C. finmarchicus and C. hyperboreus dominated the biomass of the grazer community in May, whereas the copepod nauplii stock, both in terms of abundance (up to 1100 × 103 ind m-2) and biomass (up to 1.2 g C m-2) was represented by calanoid copepod nauplii. On average, the contribution of calanoid copepod nauplii to the total copepod community (sum of the small and large copepods and nauplii) in May was 69% in terms of abundance and 30% in terms of biomass. The exceptionally high nauplii abundance indicates high reproductive success of Calanus in May. In August, on the other hand, the stock of the three Calanus species displayed notably different structures, with C. finmarchicus predominating in abundance as well as in biomass. This likely reflects different reproductive strategies between the three Calanus species, which is also thoroughly documented in previous studies from adjacent areas (Arnkværn et al., 2005; Søreide et al., 2010).
The dominance of C. glacialis and C. hyperboreus young copepodids CI–CIII in spring indicates that the main reproductive period for these species happened before our investigation, and hence prior to the onset of the spring bloom. By reproducing prior to the (open water) spring bloom, the new cohorts are ready to feed and grow during the short and intensive pelagic bloom, and have a chance to reach the overwintering stage later during the growth period. This reproductive strategy is referred to as capital breeding and is an adaptation to strong seasonality (Varpe et al., 2009). In addition, because the developmental time and survival of C. glacialis nauplii are sensitive to food quality, the chances to survive are higher when feeding on algae with high proportions of PUFAs (Daase et al., 2011). Due to efficient lipid synthesis and storage, both species can overwinter relatively young; C. glacialis mainly as CIII–CIV (Madsen et al., 2001; Søreide et al., 2010) and C. hyperboreus already as CIII (Kvile et al., 2018). The early egg laying, and the accessibility to high-quality and lipid-rich phytoplankton such as diatoms for the developing nauplii and young copepodids, allows these species to reach the overwintering stage within the first year. However, they may use two or more years to reach the reproductive stage (Diel, 1991). We suggest that C. glacialis and C. hyperboreus populations in the Atlantic inflow areas north of Spitsbergen depend to a large extent on the new production (nitrate-fueled) for the recruiting generation to reach the first overwintering stage. This is also in agreement with previous investigations of Calanus spp. feeding preferences (Levinsen et al., 2000b; Søreide et al., 2008).
Calanus finmarchicus abundance and biomass were higher in August than in May, in contrast to what was observed for C. glacialis and C. hyperboreus. The C. finmarchicus population found during this study in August was still largely composed of younger stages CI–CIII, with only a few older stage CIV–CV (Figure 8). For the younger developmental stages CI–CIII to continue development and reach the overwintering stages CIV–CV, C. finmarchicus needs access to a stable food source, also after the short spring bloom period. In our study, this condition was met by a high rate of regenerated production, and possibly also the large availability of heterotrophic and mixotrophic microzooplankton in August. Madsen et al. (2001) made similar observations, showing that nauplii and protists may form a substantial part of the diet of the Calanus community in the post bloom period in Disco Bay, western Greenland. Our findings support the existing knowledge on the reproductive strategy of C. finmarchicus. This species is defined as an income breeder (Varpe et al., 2009), whose females need to feed on the open water spring bloom to produce eggs. The new cohorts develops from egg to young copepodid during the spring bloom (Arnkværn et al., 2005). However, we stress that the new production is important during the early phase of the life cycle (fueling egg production in the females, and the development from eggs to CIII copepodids) whereas the regenerated production appears essential for C. finmarchicus to reach the hibernating stage (CIV–CV) within the same growth year in our study area.
Among the small copepods, O. similis was the most abundant species both in spring and summer, but the population size was significantly larger in August than in May. Nauplii of Oithona spp. were found at all stations and occurred in extreme abundances in August (exceeding 2000 × 103 ind m-2 at P6). The high contribution of copepod nauplii to the total copepod community at all stations was notable, and the clear shift from dominance of Calanus spp. nauplii in May to Oithona spp. nauplii in August reflects differences in reproductive strategies between the two copepod genera. The life history strategy of the cyclopoid copepod O. similis is in strong contrast to the strategy of calanoid copepod Calanus spp. (Svensen et al., 2011). O. similis does not overwinter at great depths and it can reproduce year-round, except in mid-winter (Madsen et al., 2001, 2008). At high latitudes, main reproductive periods are suggested to occur in May and September (Lischka and Hagen, 2005; Madsen et al., 2008; Narcy et al., 2009). O. similis is a strict ambush feeder with a preference for ciliates and dinoflagellates (Svensen and Kiørboe, 2000). Analyses of fatty acid of O. similis in the Arctic Kongsfjorden (Svalbard) demonstrated high abundance of the 18:1 (n - 9) fatty acid in all stages and seasons, which indicates an omnivorous diet that does not change notably with season (Lischka and Hagen, 2007). Since it is not directly dependent on the spring bloom to reproduce or to complete its life cycle, O. similis can instead take advantage of the post bloom regenerated production in summer to support its mass reproduction and successful population growth. In turn, through sloppy feeding, Oithona can release dissolved organic carbon (Svensen and Vernet, 2016) fueling the microbial loop, bacterial growth and eventually a buildup of the microzooplankton.
The biomass of microzooplankton was more than three times higher in August than in May. This could reflect both better feeding conditions and decreased copepod predation in August compared to May. In August, the predominance of nanophytoplankton and the increase in Synechococcus abundance (Paulsen et al., 2016) could have supported the higher and more diverse microzooplankton biomass (Lavrentyev et al., 2019). In fact, although low temperature can affect microzooplankton physiology, when adapted to cold environment, Arctic microzooplankton can grow (Franzè and Lavrentyev, 2014, 2017; Menden-Deuer et al., 2018) and graze phytoplankton (Franzè and Lavrentyev, 2017; Lavrentyev et al., 2019) at rates comparable to their temperate counterparts. Microzooplankton can respond quickly to changes in primary production by increasing their biomass (Levinsen et al., 2000a) and ingestion rates (Calbet, 2001). At the same time, microzooplankton are preferred prey of copepods (Campbell et al., 2009), and their biomass can be suppressed by copepod grazers. In our study, the older developmental stages of C. finmarchicus and C. glacialis were located mostly below 100 m in August, and this may have reduced the grazing pressure on the microzooplankton, which were distributed above 100 m. A comparable scenario has also been reported in other Arctic areas in summer (Levinsen et al., 1999, 2000b).
The biomass of microzooplankton and O. similis increased significantly from May to August (Table 3). While the biomass of C. hyperboreus decreased from May to August, the total biomass of C. finmarchicus and C. glacialis was relatively similar in the two sampling periods (Table 3). How well were the different grazer groups supported by the new and regenerated autotrophic production during the two seasons? We calculated production rates of the microzooplankton and the dominating copepod species (C. finmarchicus, C. glacialis, C. hyperboreus, and O. similis) in the upper 100 m, based on published production/biomass (P/B) ratios (Table 3) and ingestion rates by assuming a gross growth efficiency of 30% (Omori and Ikeda, 1984; Straile, 1997). These estimations, although somewhat crude, provide the possibility to evaluate the energy demand of the zooplankton communities in relation to spring and summer productivity state.
Table 3. Daily production to biomass (P/B) ratios (literature values), integrated biomass (mg C m-2, seasonal mean ± SD), and estimated production and ingestion rates (mg C m-2 d-1) for microzooplankton and the dominating copepod species in the upper 100 m.
The estimated production rates in May were high for the microzooplankton community (70 ± 14 mg C m-2 d-1), and generally low for the dominating copepod species (Table 3). Furthermore, the estimated ingestion rates in May were well below the measured total primary production rate (Table 4) and did not exceed the total estimated new production for this time (Table 4). Hence, based on these rough calculations, we can assume that both the microzooplankton and the Calanus spp. populations were sufficiently supported by the new primary production resulting from the activity of the dominating phytoplankton community in May. Similar findings are available from a study in Disko Bay (Greenland), where during the early phase of the bloom, C. finmarchicus were predominantly herbivorous with a very small contribution of microzooplankton to their diet (Levinsen et al., 2000b).
Table 4. Total (monthly mean ± SD) particulate primary production (PP, mg C m-2 d-1) based on integrated values 0–50 m depth and f-ratio (at the depth of highest PP).
In August, the total zooplankton production was dominated by microzooplankton (237 ± 47 mg C m-2 d-1) and O. similis (30 ± 4 mg C m-2 d-1), followed by C. finmarchicus (10 ± 4 mg C m-2 d-1) (Table 3). The estimated ingestion rates of this grazer community was 948 mg C m-2 d-1 (Table 3) and exceeded the measured total primary production in August (Table 4). The apparent discrepancy between the total primary production and the estimated ingestion rates of the main zooplankton could indicate that there were additional food sources than autotrophic phytoplankton available during the post bloom period. Paulsen et al. (2018) found that dissolved organic nitrate (DON) accumulated during summer, resulting from microbial activity. The bacteria biomass and production rates at the investigated stations were also higher in August than in May (Paulsen et al., 2018). The bacteria were likely grazed by picophytoplankton and heterotrophic flagellates (Paulsen et al., 2018), which are important food sources for microzooplankton (Franzè and Lavrentyev, 2017). This could explain the high standing stock of microzooplankton found in August. Consequently, the carbon-demands of O. similis, C. finmarchicus and other copepods present were probably met through a diet consisting mainly of microzooplankton during this time, pointing to the importance of the post bloom microbial food webs in this season and area. A similar structure of the grazer food chain in summer has also been reported in other Arctic ecosystems (Levinsen et al., 2000b).
The Arctic is undoubtedly changing. A main driver of these changes is the rapid loss of sea ice, causing a longer productive period and increased primary production due to increased light penetration in open versus ice covered water (Arrigo and Van Dijken, 2015). However, there is little knowledge on the changes in nutrient dynamics in the future Arctic scenarios, making it difficult to foresee if the increased production will be “new” or “regenerated.” Our study was limited geographically to the Atlantic inflow area north of Svalbard, representing a region of the Arctic that is seasonally ice covered and strongly influenced by Atlantic water masses. Historically, few studies have focused on the food web implications of new and regenerated production in this area. Our investigation may not be extrapolated to all parts of the Arctic, since the different Arctic regions are very heterogenous with respect to nutrient dynamics over the productive season. However, the scenario encountered in our study is still relevant for large parts of seasonally ice covered areas in the Arctic.
For an increase in new production, nitrate must be added to the productive surface waters from deep water reservoirs through processes such as upwelling and diffusion across the pycnocline (Randelhoff et al., 2016; Randelhoff and Sundfjord, 2018). In the investigated area north of Svalbard, a summer upwelling event has been considered rather unlikely (Randelhoff and Sundfjord, 2018), and an oligotrophic post bloom situation may be the governing situation after the spring bloom decline. In oligotrophic areas, regenerated production supported by recycled N accounts for 90% of the gross primary production (Eppley and Peterson, 1979). It therefore appears reasonable to assume that a large fraction of the increased primary production in this part of the Arctic in summer will be based on recycled N (or e.g., dissolved organic carbon). This is in accordance with Randelhoff et al. (2015), who found that the summer primary production was nutrient limited, and concluded that the potential for an increase in new production in a scenario with less sea ice is limited in the area north-east of Svalbard.
The question is, if the magnitude of the new production remains the same due to nitrate limitation (provided the net influx of nitrate will not change), who will benefit from an increased regenerated production in a future Arctic characterized by decreased seasonal ice cover and an increased productive season? A short growth season favors large bodied capital breeders because the adults have large storage capacity for lipids that increases their fecundity, and the new generation appearing prior to the bloom can utilize the pulsed production (Varpe et al., 2009; Sainmont et al., 2014). We argue that an extension of the growth season by a prolonged period of regenerated production may favor small bodied copepods with short generation times, low lipid storage capacity, low metabolic rates and low fecundity. The regenerated production can be sustained on reduced form of inorganic N (such as ammonium), but also on dissolved organic carbon (DOC) and nitrogen (DON) (Paulsen et al., 2018). We argue that an active microbial food web, fueled by DOC and DON, may support a large heterotrophic community and high secondary production after the sources of inorganic N have been used up.
Our study confirms the dependence and tight coupling between the early spring bloom and life history adaptations (large lipid storage capacity, early start of diapause) of C. glacialis and C. hyperboreus. Although the remaining surface-active populations of C. glacialis and C. hyperboreus graze the microzooplankton in summer (Levinsen et al., 2000b), it seems that it is the diatom-dominated spring bloom that is the most important food source for the new cohort to reach the overwintering stage (Søreide et al., 2008). In contrast, the younger fraction of the C. finmarchicus population may remain in the surface waters for a longer time (Hansen et al., 1999) and may use the post bloom regenerated production and microbial food web to reach the overwintering stage within one growth season. This indicates that C. finmarchicus may be able to profit from an increased primary production in the Arctic, even if the primary production is based on regenerated nutrients. This practice could support a northward extension of this species’ habitat range. This is also a likely strategy for dominating small copepod species such as O. similis, that could most likely fulfill its full life cycle on regenerated production only. We suggest that the degree of coupling to the regenerated production and microbial food web may be of crucial importance for the success of the heterotrophic planktonic grazers in the future Arctic Ocean.
CS analyzed the data and wrote the manuscript. CS and EH sampled the zooplankton. MV conducted the primary production measurements. GF and PL collected and analyzed the microzooplankton samples. KD and SK were responsible for analyzing the mesozooplankton samples. All authors contributed to the interpretation of data and commented on the text.
This work was conducted within the project CarbonBridge (RCN 226415) funded by the Research Council of Norway. The publication charges for this article have been funded by a grant from the publication fund of UiT – The Arctic University of Norway. This study was funded by the National Science Foundation (Award OCE-1357168) and the University of Akron.
The authors declare that the research was conducted in the absence of any commercial or financial relationships that could be construed as a potential conflict of interest.
We thank the crew on R/V “Helmer Hanssen” for assistance during sampling, and Sigrid Øygarden for technical support during the cruses and in the laboratory. Maria Antonsen helped with zooplankton sampling during the May cruise, and Anna Olszewska helped with examination of MultiNet samples. We thank Jean-Eric Tremblay for providing data on f-ratios, Achim Randelhoff for temperature data, and the project leader Marit Reigstad for support and effort on the CarbonBridge project.
The Supplementary Material for this article can be found online at: https://www.frontiersin.org/articles/10.3389/fmars.2019.00293/full#supplementary-material
Arnkværn, G., Daase, M., and Eiane, K. (2005). Dynamics of coexisting Calanus finmarchicus, Calanus glacialis and Calanus hyperboreus populations in a high-Arctic fjord. Polar Biol. 28, 528–538. doi: 10.1007/s00300-005-0715-8
Arrigo, K. R., and Van Dijken, G. L. (2011). Secular trends in Arctic Ocean net primary production. J. Geophys. Res. Oceans 116:C09011.
Arrigo, K. R., and Van Dijken, G. L. (2015). Continued increases in Arctic Ocean primary production. Prog. Oceanogr. 136, 60–70. doi: 10.1016/j.pocean.2015.05.002
Calbet, A. (2001). Mesozooplankton grazing effect on primary production: a global comparative analysis in marine ecosystems. Limnol. Oceanogr. 46, 1824–1830. doi: 10.4319/lo.2001.46.7.1824
Campbell, R. G., Sherr, E. B., Ashjian, C. J., Plourde, S., Sherr, B. F., Hill, V., et al. (2009). Mesozooplankton prey preference and grazing impact in the western Arctic Ocean. Deep Sea Res. Part II Top. Stud. Oceanogr. 56, 1274–1289. doi: 10.1016/j.dsr2.2008.10.027
Choquet, M., Kosobokova, K., Kwasniewski, S., Hatlebakk, M., Dhanasiri, A. K. S., Melle, W., et al. (2018). Can morphology reliably distinguish between the copepods Calanus finmarchicus and C. glacialis, or is DNA the only way?. Limnol. Oceanogr. Methods 16, 237–252. doi: 10.1002/lom3.10240
Daase, M., Søreide, J. E., and Martynova, D. (2011). Effects of food quality on naupliar development in Calanus glacialis at subzero temperatures. Mar. Ecol. Prog. Ser. 429, 111–124. doi: 10.3354/meps09075
Diel, S. (1991). On the life history of dominant copepod species (Calanus finmarchicus, C. glacialis, C. hyperboreus, Metridia longa) in the Fram Strait. Ber. Polarforsch. 88, 111–113.
Diel, S., and Tande, K. (1992). Does the spawning of Calanus finmarchicus in high-latitudes follow a reproducible pattern. Mar. Biol. 113, 21–31. doi: 10.1007/bf00367634
Dugdale, R. C., and Goering, J. J. (1967). Uptake of new and regenerated forms of nitrogen in primary productivity. Limnol. Oceanogr. 12, 196–206. doi: 10.4319/lo.1967.12.2.0196
Eppley, R. W., and Peterson, B. J. (1979). Particulate organic matter flux and planktonic new production in the deep ocean. Nature 282, 677–680. doi: 10.1038/282677a0
Falk-Petersen, S., Mayzaud, P., Kattner, G., and Sargent, J. (2009). Lipids and life strategy of Arctic Calanus. Mar. Biol. Res. 5, 18–39.
Feng, Z. X., Ji, R. B., Campbell, R. G., Ashjian, C. J., and Zhang, J. L. (2016). Early ice retreat and ocean warming may induce copepod biogeographic boundary shifts in the Arctic Ocean. J. Geophys. Res.Oceans 121, 6137–6158. doi: 10.1002/2016jc011784
Franze, G., and Lavrentyev, P. J. (2014). Microzooplankton growth rates examined across a temperature gradient in the Barents Sea. PLoS One 9:14. doi: 10.1371/journal.pone.0086429
Franzè, G., and Lavrentyev, P. J. (2017). Microbial food web structure and dynamics across a natural temperature gradient in a productive polar shelf system. Mar. Ecol. Prog. Ser. 569, 89–102. doi: 10.3354/meps12072
Hansen, B. W., Nielsen, T. G., and Levinsen, H. (1999). Plankton community structure and carbon cycling on the western coast of Greenland during the stratified summer situation. III. Mesozooplankton. Aquat. Microb. Ecol. 16, 233–249. doi: 10.3354/ame016233
Hirche, H. J. (1996). Diapause in the marine copepod, Calanus finmarchicus - A review. Ophelia 44, 129–143. doi: 10.1080/00785326.1995.10429843
Kristiansen, S., Farbrot, T., and Wheeler, P. A. (1994). Nitrogen Cycling in the Barents Sea - Seasonal Dynamics of New and Regenerated Production in the Marginal Ice-Zone. Limnol. Oceanogr. 39, 1630–1642. doi: 10.4319/lo.1994.39.7.1630
Kvile, K. O., Ashjian, C., Feng, Z. X., Zhang, J. L., and Ji, R. B. (2018). Pushing the limit: resilience of an Arctic copepod to environmental fluctuations. Glob. Change Biol. 24, 5426–5439. doi: 10.1111/gcb.14419
Kwasniewski, S., Hop, H., Falk-Petersen, S., and Pedersen, G. (2003). Distribution of Calanus species in Kongsfjorden, a glacial fjord in Svalbard. J. Plankton Res. 25, 1–20. doi: 10.1007/s10482-015-0580-6
Lavrentyev, P. J., Franzé, G., and Moore, F. B. (2019). Microzooplankton distribution and dynamics in the eastern Fram Strait and the Arctic Ocean in May and August 2014. Front. Mar. Sci. 6:264. doi: 10.3389/fmars.2019.00264
Legendre, L., and Rassoulzadegan, F. (1995). Plankton and nutrient dynamics in marine waters. Ophelia 41, 153–172. doi: 10.1080/00785236.1995.10422042
Leu, E., Falk-Petersen, S., Kwasniewski, S., Wulff, A., Edvardsen, K., and Hessen, D. O. (2006). Fatty acid dynamics during the spring bloom in a High Arctic fjord: importance of abiotic factors versus community changes. Can. J. Fish. Aqua. Sci. 63, 2760–2779. doi: 10.1139/f06-159
Leu, E., Soreide, J. E., Hessen, D. O., Falk-Petersen, S., and Berge, J. (2011). Consequences of changing sea-ice cover for primary and secondary producers in the European Arctic shelf seas: timing, quantity, and quality. Prog. Oceanogr. 90, 18–32. doi: 10.1016/j.pocean.2011.02.004
Levinsen, H., Nielsen, T. G., and Hansen, B. W. (1999). Plankton community structure and carbon cycling on the western coast of Greenland during the stratified summer situation. II. heterotrophic dinoflagellates and ciliates. Aquat. Microb. Ecol. 16, 217–232. doi: 10.3354/ame016217
Levinsen, H., Nielsen, T. G., and Hansen, B. W. (2000a). Annual succession of marine pelagic protozoans in Disko Bay, West Greenland, with emphasis on winter dynamics. Mar. Ecol. Prog. Ser. 206, 119–134. doi: 10.3354/meps206119
Levinsen, H., Turner, J. T., Nielsen, T. G., and Hansen, B. W. (2000b). On the trophic coupling between protists and copepods in arctic marine ecosystems. Mar. Ecol. Prog. Ser. 204, 65–77. doi: 10.3354/meps204065
Li, W. K. W., Mclaughlin, F. A., Lovejoy, C., and Carmack, E. C. (2009). Smallest algae thrive as the arctic ocean freshens. Science 326, 539–539. doi: 10.1126/science.1179798
Lischka, S., and Hagen, W. (2005). Life histories of the copepods Pseudocalanus minutus, P. acuspes (Calanoida) and Oithona similis (Cyclopoida) in the Arctic Kongsfjorden (Svalbard). Polar Biol. 28, 910–921. doi: 10.1007/s00300-005-0017-1
Lischka, S., and Hagen, W. (2007). Seasonal lipid dynamics of the copepods Pseudocalanus minutus (Calanoida) and Oithona similis (Cyclopoida) in the Arctic Kongsfjorden (Svalbard). Mar. Biol. 150, 443–454. doi: 10.1007/s00227-006-0359-4
Madsen, S. D., Nielsen, T. G., and Hansen, B. W. (2001). Annual population development and production by Calanus finmarchicus, C. glacialis and C. hyperboreus in Disko Bay, western Greenland. Mar. Biol. 139, 75–93. doi: 10.1007/s002270100552
Madsen, S. D., Nielsen, T. G., and Hansen, B. W. (2008). Annual population development and production by small copepods in Disko Bay, western Greenland. Mar. Biol. 155, 63–77. doi: 10.1007/s00227-008-1007-y
Menden-Deuer, S., Lawrence, C., and Franze, G. (2018). Herbivorous protist growth and grazing rates at in situ and artificially elevated temperatures during an Arctic phytoplankton spring bloom. PeerJ 6:e5264. doi: 10.7717/peerj.5264
Menden-Deuer, S., and Lessard, E. J. (2000). Carbon to volume relationships for dinoflagellates, diatoms, and other protist plankton. Limnol. Oceanogr. 45, 569–579. doi: 10.4319/lo.2000.45.3.0569
Narcy, F., Gasparini, S., Falk-Petersen, S., and Mayzaud, P. (2009). Seasonal and individual variability of lipid reserves in Oithona similis (Cyclopoida) in an Arctic fjord. Polar Biol. 32, 233–242. doi: 10.1007/s00300-008-0524-y
Onarheim, I. H., Eldevik, T., Smedsrud, L. H., and Stroeve, J. C. (2018). Seasonal and regional manifestation of Arctic Sea Ice Loss. J. Clim. 31, 4917–4932. doi: 10.1175/jcli-d-17-0427.1
Parrish, C. C., Thompson, R. J., and Deibel, D. (2005). Lipid classes and fatty acids in plankton and settling matter during the spring bloom in a cold ocean coastal environment. Mar. Ecol. Prog. Ser. 286, 57–68. doi: 10.3354/meps286057
Paulsen, M. L., Doré, H., Garczarek, L., Seuthe, L., Müller, O., Sandaa, R.-A., et al. (2016). Synechococcus in the Atlantic Gateway to the Arctic Ocean. Front. Mar. Sci. 3:191. doi: 10.3389/fmars.2016.00191
Paulsen, M. L., Seuthe, L., Reigstad, M., Larsen, A., Cape, M. R., and Vernet, M. (2018). Asynchronous accumulation of organic carbon and nitrogen in the Atlantic Gateway to the Arctic Ocean. Front. Mar. Sci. 5:416. doi: 10.3389/fmars.2018.00416
Pedersen, O. P., Tande, K. S., and Slagstad, D. (2001). A model study of demography and spatial distribution of Calanus finmarchicus at the Norwegian coast. Deep Sea Res. Part II-Top. Stud. Oceanogr. 48, 567–587. doi: 10.1016/s0967-0645(00)00127-2
Postel, L., Fock, H., and Hagen, W. (2000). “Biomass and abundance,” in ICES Zooplankton Methodology Manual, eds R. Harris, J. Lenz, M. Huntley, P. Wiebe, and H. R. Skjoldal (Cambridge: Academic Press),83–192.
Putt, M., and Stoecker, D. K. (1989). An experimentally determined carbon: volume ratio for marine “oilgotrichous” Ciliates from estuarine and coastal waters. Limnol. Oceanogr. 34, 1097–1103. doi: 10.4319/lo.1989.34.6.1097
Randelhoff, A., Fer, I., Sundfjord, A., Tremblay, J. E., and Reigstad, M. (2016). Vertical fluxes of nitrate in the seasonal nitracline of the Atlantic sector of the Arctic Ocean. J. Geophys. Res. Oceans 121, 5282–5295. doi: 10.1002/2016jc011779
Randelhoff, A., Reigstad, M., Chierici, M., Sundfjord, A., Ivanov, V., Cape, M., et al. (2018). Seasonality of the physical and biogeochemical hydrography in the inflow to the Arctic Ocean Through Fram Strait. Front. Mar. Sci. 5:224. doi: 10.3389/fmars.2018.00224
Randelhoff, A., and Sundfjord, A. (2018). Short commentary on marine productivity at Arctic shelf breaks: upwelling, advection and vertical mixing. Ocean Sci. 14, 293–300. doi: 10.5194/os-14-293-2018
Randelhoff, A., Sundfjord, A., and Reigstad, M. (2015). Seasonal variability and fluxes of nitrate in the surface waters over the Arctic shelf slope. Geophys. Res. Lett. 42, 3442–3449. doi: 10.1002/2015gl063655
Renner, A. H. H., Sundfjord, A., Janout, M. A., Ingvaldsen, R. B., Beszczynska-Moller, A., Pickart, R. S., et al. (2018). Variability and Redistribution of Heat in the Atlantic Water Boundary Current North of Svalbard. J. Geophys. Res. Oceans 123, 6373–6391. doi: 10.1029/2018jc013814
Sainmont, J., Andersen, K. H., Varpe, O., and Visser, A. W. (2014). Capital versus income breeding in a seasonal environment. Am. Nat. 184, 466–476. doi: 10.1086/677926
Shilova, I. N., Mills, M. M., Robidart, J. C., Turk-Kubo, K. A., Bjorkman, K. M., Kolber, Z., et al. (2017). Differential effects of nitrate, ammonium, and urea as N sources for microbial communities in the North Pacific Ocean. Limnol. Oceanogr. 62, 2550–2574. doi: 10.1002/lno.10590
Søreide, J. E., Falk-Petersen, S., Hegseth, E. N., Hop, H., Carroll, M. L., Hobson, K. A., et al. (2008). Seasonal feeding strategies of Calanus in the high-Arctic Svalbard region. Deep Sea Res. Part II-Top. Stud. Oceanogr. 55, 2225–2244. doi: 10.1016/j.dsr2.2008.05.024
Søreide, J. E., Leu, E., Berge, J., Graeve, M., and Falk-Petersen, S. (2010). Timing of blooms, algal food quality and Calanus glacialis reproduction and growth in a changing Arctic. Glob. Change Biol. 16, 3154–3163.
Steemann Nielsen, E. (1952). The use of radioactive (14C) for measuring organic production in the sea. J. Cons. Int. Explor. Mer. 18, 117–140. doi: 10.1093/icesjms/18.2.117
Straile, D. (1997). Gross growth efficiencies of protozoan and metazoan zooplankton and their dependence on food concentration, predator-prey weight ratio, and taxonomic group. Limnol. Oceanogr. 42, 1375–1385. doi: 10.4319/lo.1997.42.6.1375
Svensen, C., and Kiørboe, T. (2000). Remote prey detection in Oithona similis: hydromechanical versus chemical cues. J. Plankton Res. 22, 1155–1166. doi: 10.1093/plankt/22.6.1155
Svensen, C., Seuthe, L., Vasilyeva, Y., Pasternak, A., and Hansen, E. (2011). Zooplankton distribution across fram strait in autumn: are small copepods and protozooplankton important? Prog. Oceanogr. 91, 534–544. doi: 10.1016/j.pocean.2011.08.001
Svensen, C., and Vernet, M. (2016). Production of dissolved organic carbon by Oithona nana (Copepoda: Cyclopoida) grazing on two species of dinoflagellates. Mar. Biol. 163:237.
Tremblay, M. J., and Roff, J. C. (1983). Production estimates for scotian shelf copepods based on mass specific P/B ratios. Can. J. Fish. Aqu. Sci. 40, 749–753. doi: 10.1139/f83-097
Varpe, O., Jorgensen, C., Tarling, G. A., and Fiksen, O. (2009). The adaptive value of energy storage and capital breeding in seasonal environments. Oikos 118, 363–370. doi: 10.1111/j.1600-0706.2008.17036.x
Vernet, M., Matrai, P. A., and Andreassen, I. (1998). Synthesis of particulate and extracellular carbon by phytoplankton at the marginal ice zone in the Barents Sea. J. Geophys. Res. Oceans 103, 1023–1037. doi: 10.1029/97jc02288
Keywords: copepods, copepod nauplii, Calanus spp., Oithona similis, microzooplankton, food web, Arctic
Citation: Svensen C, Halvorsen E, Vernet M, Franzè G, Dmoch K, Lavrentyev PJ and Kwasniewski S (2019) Zooplankton Communities Associated With New and Regenerated Primary Production in the Atlantic Inflow North of Svalbard. Front. Mar. Sci. 6:293. doi: 10.3389/fmars.2019.00293
Received: 03 January 2019; Accepted: 20 May 2019;
Published: 05 June 2019.
Edited by:
Christos Dimitrios Arvanitidis, Hellenic Centre for Marine Research (HCMR), GreeceReviewed by:
Constantin Frangoulis, Hellenic Center for Marine Research, GreeceCopyright © 2019 Svensen, Halvorsen, Vernet, Franzè, Dmoch, Lavrentyev and Kwasniewski. This is an open-access article distributed under the terms of the Creative Commons Attribution License (CC BY). The use, distribution or reproduction in other forums is permitted, provided the original author(s) and the copyright owner(s) are credited and that the original publication in this journal is cited, in accordance with accepted academic practice. No use, distribution or reproduction is permitted which does not comply with these terms.
*Correspondence: Camilla Svensen, Q2FtaWxsYS5zdmVuc2VuQHVpdC5ubw==
†Present address: Gayantonia Franzè, Institute of Marine Research, Flødevigen, Norway
Disclaimer: All claims expressed in this article are solely those of the authors and do not necessarily represent those of their affiliated organizations, or those of the publisher, the editors and the reviewers. Any product that may be evaluated in this article or claim that may be made by its manufacturer is not guaranteed or endorsed by the publisher.
Research integrity at Frontiers
Learn more about the work of our research integrity team to safeguard the quality of each article we publish.