- 1Southern California Coastal Ocean Observing System, Scripps Institution of Oceanography, La Jolla, CA, United States
- 2Institute of Marine Sciences (CSIC), Barcelona, Spain
- 3Department of Ocean Sciences, University of California, Santa Cruz, Santa Cruz, CA, United States
- 4Marine Institute, Galway, Ireland
- 5Alaska Ocean Observing System, Anchorage, AK, United States
- 6Northwest Association of Networked Ocean Observing Systems, University of Washington, Seattle, WA, United States
- 7Environmental and Fisheries Sciences Division, Northwest Fisheries Science Center, National Marine Fisheries Service, National Oceanic and Atmospheric Administration, Seattle, WA, United States
- 8Great Lakes Observing System, Ann Arbor, MI, United States
- 9Great Lakes Environmental Research Laboratory, National Oceanic and Atmospheric Administration, Ann Arbor, MI, United States
- 10Northeastern Regional Association of Coastal Ocean Observing Systems, Portsmouth, NH, United States
- 11Gulf of Mexico Coastal Ocean Observing System, College Station, TX, United States
- 12Florida Fish and Wildlife Conservation Commission, St. Petersburg, FL, United States
- 13Caribbean Coastal Ocean Observing System, University of Puerto Rico, Mayagüez, Puerto Rico, PR, United States
Harmful algal blooms (HABs) produce local impacts in nearly all freshwater and marine systems. They are a problem that occurs globally requiring an integrated and coordinated scientific understanding, leading to regional responses and solutions. Given that these natural phenomena will never be completely eliminated, an improved scientific understanding of HAB dynamics coupled with monitoring and ocean observations, facilitates new prediction and prevention strategies. Regional efforts are underway worldwide to create state-of-the-art HAB monitoring and forecasting tools, vulnerability assessments, and observing networks. In the United States, these include Alaska, Pacific Northwest, California, Gulf of Mexico, Gulf of Maine, Great Lakes, and the United States Caribbean islands. This paper examines several regional programs in the United States, European Union, and Asia and concludes that there is no one-size-fits-all approach. At the same time, successful programs require strong coordination with stakeholders and institutional sustainability to maintain and reinforce them with new automating technologies, wherever possible, ensuring integration of modeling efforts with multiple regional to national programs. Recommendations for scaling up to a global observing system for HABs can be summarized as follows: (1) advance and improve cost-effective and sustainable HAB forecast systems that address the HAB-risk warning requirements of key end-users at global and regional levels; (2) design programs that leverage and expand regional HAB observing systems to evaluate emerging technologies for Essential Ocean Variables (EOVs) and Essential Biodiversity Variables (EBVs) in order to support interregional technology comparisons and regional networks of observing capabilities; (3) fill the essential need for sustained, preferably automated, near real-time information from nearshore and offshore sites situated in HAB transport pathways to provide improved, advanced HAB warnings; (4) merge ecological knowledge and models with existing Earth System Modeling Frameworks to enhance end-to-end capabilities in forecasting and scenario-building; (5) provide seasonal to decadal forecasts to allow governments to plan, adapt to a changing marine environment, and ensure coastal industries are supported and sustained in the years ahead; and (6) support implementation of the recent calls for action by the United Nations Decade 2010 Sustainable Development Goals (SDGs) to develop indicators that are relevant to an effective and global HAB early warning system.
Introduction
Commonly known in many parts of the world as floraciones algales nocivas (FANs), prolifération d’algues nuisibles (PANs), or harmful algal blooms (HABs), potentially noxious algae growth in coastal environments worldwide is characterized by appearing without warning and often lingering long past expectation (see Supplementary Table S1 for a list of abbreviations in Supplementary Material). The number of different micro- and even macro-algae responsible for causing HABs is remarkable, which adds to the difficulty in identifying universal causes and mechanisms driving both the initiation and termination of HABs in marine and freshwater environments. Complete prevention or mitigation of HABs may never be realistically achieved given the complex ecology typical of this broad collective that includes plant-like organisms and cyanobacteria. When human pollution or activities are a known cause or contributing pressure (Anderson et al., 2002), however, preventive measures may be possible (Anderson et al., 2015). For example, the regulation of human-derived nutrient inputs can benefit areas where HABs are prevalent (e.g., Ehlers, 1994; Glibert and Burford, 2017). International regulations also prevent contaminated ballast water exchange in sensitive marine regions and aim to diminish transport of organisms across regions (International Maritime Organization [IMO], 2003; Burkholder et al., 2007). For most scientists and policy-makers engaged in the HAB problem, however, the focus has shifted from prevention to mitigation and adaptation through frequent monitoring of causative organisms and their toxins using innovative technologies, short-term prediction, and strong stakeholder participation that alleviates the strain on ecosystems, public health, and local-to-regional economies and reduces the exposure to humans and wildlife (Anderson, 2009).
There is also a growing recognition that the increase in global mariculture and aquaculture, necessary for protein food supply to humanity, is bringing the potential risk of HABs into focus (Anderson, 2009). Declining wild fish stocks are creating a need for more efficient farm production methods. Indeed, a central theme of the European Commission (EC) Common Fisheries Policy is to improve aquaculture outputs. HAB toxins can contaminate farmed finfish and shellfish stocks, just as they do wild populations, leading to a heightened risk of seafood-borne illnesses when consumed by humans, with subsequent regulatory closures of recreational and commercial harvest. Aquaculture facilities also experience hypoxic events from high-biomass algal blooms that lead to high mortality of enclosed finfish. Shellfish production volumes have seen many peaks and troughs over the past 30 years, with declines potentially coinciding with HAB events accompanied by prolonged shellfish growing area closures (e.g., Cusack et al., 2016). The latest available European bivalve shellfish aquaculture production figures show a yield of 612,579 tons in 2016 with an estimated value of 1,111 million USD (Fisheries and Aquaculture Information and Statistics Branch - 02/08/2018)1. With this increased dependence on aquaculture comes significant food safety concerns. The focus on food quality (e.g., through food hygiene legislation to guarantee safe food) is acknowledged by industries who capitalize on the perception that seafood is very safe due to the high-quality regulations in place and use this as a marketing tool for their products. Seafood consumers often judge HAB disruptions as a signal of unreliability and look to industry competitors for future product supply. While fishery closures and recalls from HAB disruptions protect consumers, they also tend to be reactive since the timing of HAB and biotoxin events is often unpredictable days to weeks in advance. An effective HAB warning system should have components that are tailored to aquaculture to allow producers to change their husbandry practices, such as avoiding the handling of shellfish, proactively relocating stocks, harvesting shellfish earlier or canceling a delivery in order to minimize losses and waste due to contaminated stock, or, by intensifying harvest prior to a HAB event.
Harmful algal bloom risk can be difficult to quantify because HABs are characterized by an extremely diverse set of processes and impacts, all with significant societal relevance that warrants a decisive and strategic response from the ocean observing community. International co-operative research has proven decisive in identifying and institutionalizing tools to improve the understanding of HAB dynamics and design mitigation strategies. Indeed, the international programs GEOHAB (2000–2013) and GlobalHAB (established in 2016 to run until 20252), both sponsored by SCOR (Scientific Committee on Oceanic Research) and the Intergovernmental Oceanographic Commission (IOC) of the United Nations Educational, Scientific and Cultural Organization (UNESCO) foster research on HABs across ecosystems that share common features, comparing key species involved and the oceanographic processes that influence their population dynamics (GEOHAB, 2001, 2005, 2006, 2008, 2010a,b; Kudela et al., 2017). Under GEOHAB and GlobalHAB, the international community defined priority goals, objectives, and scientific road maps that constitute the basis for HAB research and management at local and regional scales. Importantly, these programs also interface with other trans-national and international efforts such as the Global Ocean Observing System (GOOS), Group on Earth Observations Biodiversity Observation Network (GEO BON), Marine Biodiversity Observation Network (MBON), and GEO/Blue Planet to implement HAB-specific efforts within these coordinated networks.
With the adoption of the UN list of Sustainable Development Goals (SDGs) as a global rubric for structuring future applied science objectives (G7, 2018. Paris Accord), the mandate for sustained ocean observing systems is crystallizing around these 17 central themes of societal benefit. For ocean observing systems with HAB monitoring programs, there is a pre-existing culture of focusing science outcomes and decision-making products around a set of well-defined stakeholder requirements. In other words, the monitoring of HABs and their toxins has fundamentally been a science-driven activity but with strong application to private industry, management, and policy decisions. The ocean observing community now recognizes the transcendent role that HAB monitoring plays in bridging ocean observing to ocean policy and to the many overlapping indicators specified by the SDGs. These targets and indicators fall within the themes of food security (SDG 2), human health (SDG 3), water supply (SDG 6), sustainability (SDG 11), climate change (SDG 13), and aquatic ecosystems (SDG 14). HABs impact each of these sectors: food security via contamination of seafood or direct mortality of fish stocks, human health via seafood contamination, water contamination, and reduction in air quality, water supply via poisoning of drinking reservoirs and general degradation of water quality, our ability to sustain resilient coasts, climate-related hazards in the aquatic zone, and the overall functioning and services that aquatic ecosystems provide. Improvement in seafood production also supports recommendations from the United Nations Environment Program (UNEP), which has voiced concerns that as the global population increases toward a predicted 9.1 billion people by 2050, the western preference for diets rich in meat and dairy products is unsustainable. Additionally, when it comes to food and economic security, marine aquaculture is vital to regional micro-economies as well as national economies.
This contribution aims to illustrate a variety of regional solutions to HAB observation and prediction as they fit within the context of the global problem. The paper does not attempt a comprehensive literature review on global HABs (c.f. Glibert et al., 2018) and does not purport to cover all the important matters pertinent to this vast and complicated subject, e.g., in situ bloom-prevention strategies (cf. Anderson et al., 2015, 2017). A common theme across regional observing systems is the engagement with relevant stakeholders and policy makers. Stakeholder outreach, in this context, does not simply refer to rudimentary display of summary data and analysis, but rather a tight feedback loop that generates iterative improvements on data and products and innovative communication strategies for impactful decision-making. The regional perspectives provided below demonstrate the utility of state-of-the-art, real-time observational capabilities, predictive models, and citizen science for meaningful academic-private-government partnerships that benefit society and are often tailored to a specific stakeholder audience. Moreover, our intent is not to document all HAB monitoring programs globally (c.f. Anderson et al., 2001), but rather to highlight key examples to identify commonalities and best practices. First, the paper highlights some key regional partnerships in the United States that enable effective HAB monitoring, followed by an overview of HAB monitoring and forecasting programs in the EU and Asia. The paper closes with a summary of lessons learned and future directions for integrating HAB monitoring and prediction into a global ocean observing framework.
Regional Case Studies
The United States Integrated Ocean Observing System (U.S. IOOS)
Ocean observing systems have been a proposed solution to long-term, sustained HAB monitoring for several decades (Anderson, 2008; Babin et al., 2008; Jochens et al., 2010; Anderson et al., 2012). In collaboration with many federal agencies - the National Aeronautics and Space Administration (NASA) and the National Oceanic and Atmospheric Administration offices, such as United States IOOS, the National Centers for Coastal Ocean Science (NCCOS), and Ocean and Atmospheric Research (OAR) – are funding regional programs in the United States that support HAB monitoring programs as part of a broader network of ocean observing in Alaska, the Pacific Northwest, California, Gulf of Mexico, Atlantic Northeast, Great Lakes, and the United States Caribbean islands. A major component driving the success of transitioning these HAB monitoring and forecasting tools from research to operations is the role that the IOOS Regional Associations play in transferring knowledge from academic, tribal, and industry partners to routine and sustained operations within the United States IOOS enterprise, often in partnership with state agencies. This section highlights many IOOS-funded activities but does not cover all regional programs and funding agencies in the United States that deal with HABs. Two important themes that emerge from the United States IOOS case studies are first, that each region has developed HAB programs that work well for that region–there is no single United States HAB observing system. Second, with few exceptions, these HAB monitoring and forecasting efforts are a part of the larger Ocean Observing framework, leveraging infrastructure, data, and personnel, and public benefit by addressing HABs as one of many important coastal ocean issues.
Alaska
Harmful algal blooms have been documented in coastal Alaska since the late 1700s, including those that can cause paralytic shellfish poisoning (PSP). Despite the risks of PSP, coastal Alaskans have always depended on wild shellfish for subsistence and recreational seafood harvests. The state currently tests only commercial shellfish products in Alaska using the mouse bioassay, but there is no routine state testing of non-commercial harvests. The Southeast Alaska Tribal Ocean Research (SEATOR) uses the receptor binding assay to determine paralytic shellfish toxins (PSTs) in shellfish tissue for subsistence harvesters. PSTs are considered unsafe by the U.S. Federal Department of Agriculture (FDA) if above the regulatory limit of 80 μg per 100 g of tissue. To reduce risk to subsistence and recreational shellfish harvesters, a number of regional monitoring programs have emerged to provide HAB-related information to harvesters. In 2017, representatives from these groups joined with research institutions and state and federal agencies in 2017 to form the Alaska Harmful Algal Bloom Network (AHAB). The goal of AHAB is to provide a statewide approach to HAB awareness, research, monitoring and response in Alaska.
The SEATOR is a consortium of 16 Tribal governments in southeast and southcentral Alaska, as well as academic, governmental, and non-profit partners. The SEATOR cornerstone partnership formed around the increasing threats of HABs and PSP in order to reduce the risks to traditional harvesters and increase year-round access to safe, wild shellfish. SEATOR partners monitor one or more community harvesting sites weekly by collecting and analyzing phytoplankton samples, filtering water samples to test for particulate toxins, and shipping bi-weekly shellfish samples to the Sitka Tribe of Alaska’s Environmental Research Lab for PSP toxin analysis using the Receptor Binding Assay (RBC, AOAC Method 2011.27). Shellfish toxicity results are sent to partners, state regulators, and researchers immediately after testing. Any shellfish with toxin levels above the FDA’s regulatory limit trigger public service announcements on local media stations and on SEATOR web pages. With weekly “eyes on the water” and shellfish results within 48 h of collection, SEATOR partners can establish subsistence management plans, reduce the risks to their citizens, and increase participation in traditional shellfish harvesting. All SEATOR data are made publicly available on the SEATOR website3 and the AHAB network site hosted by the Alaska Ocean Observing System (AOOS) and are used by resource managers and subsistence harvesters. The SEATOR partnership’s success suggests that while Alaska’s long coastlines and dispersed populations provide unique challenges for effective HAB and PSP monitoring, community-based sampling combined with close, regional partnerships can offer an effective way to reduce the human health risks from HABs.
While the SEATOR partnership was initially only focused on PSP-risk mitigation, partners’ weekly phytoplankton and environmental observations have allowed the group to respond to emerging HAB threats in Alaska as well. SEATOR partners have observed blooms of Pseudo-nitzschia, the phytoplankton responsible for Amnesic Shellfish Poisoning (ASP), more than 30 times since the group’s formation. When high Pseudo-nitzschia concentrations are observed, the Environmental Research Lab is able to test water and shellfish samples for domoic acid (DA) in addition to PSP toxins using an enzyme-linked immunosorbent assay (ELISA). Luckily, DA has not yet been detected at levels threatening to human health, although it has been detected in marine mammals (Lefebvre et al., 2016), highlighting the potential for future impacts. Given the pervasiveness of DA toxicity in Washington and Oregon and the high participation rates in traditional shellfish harvesting in Southeast Alaska, the SEATOR partnership’s proactive monitoring approach to an emerging toxin is warranted.
With Alaska’s extensive coastline and a significant population of subsistence users, expanding capacity for HABs sampling, testing and event response is imperative. Alaska is already seeing the benefits from collaborative efforts through the Alaska HAB Network, as well as technology transfer and lessons learned from other regions.
Pacific Northwest
The Pacific Northwest has a long history of human interaction with HABs as passed down through Native American oral history (Horner et al., 1997). The first recorded incident was four illnesses and one death among Capt. George Vancouver’s HMS Discovery crew in 1793 likely from PSP after eating shellfish in what is now British Columbia, Canada waters (Quale, 1966; Kao, 1993; Lewitus et al., 2012). Reported deaths from PSP occurred in Washington in 1942 and in Oregon in 1933 (Lewitus et al., 2012). Today, the most concerning HAB for human health and the regional economy is the threat of PSP from Alexandrium, Diarrhetic Shellfish Poisoning (DSP) from Dinophysis, and ASP from Pseudo-nitzschia. Blooms of Alexandrium and Dinophysis primarily occur in the inland coastal waters of Puget Sound, whereas blooms of Pseudo-nitzschia typically occur on the outer Washington-Oregon coast (Moore et al., 2009; Trainer et al., 2009a,b, Trainer et al., 2013; Lewitus et al., 2012). A combination of monitoring approaches, including state-funded programs, citizen science programs and advanced, automated technology such as the Environmental Sample Processor (ESP), as well as forecasts are used to provide early warning of impending HABs.
State monitoring of both recreationally and commercially harvested shellfish for marine biotoxins began in Washington in the 1950s (Nishitani and Chew, 1988). Since then, PSP shellfish toxicity has increased in frequency, magnitude, and geographic scope, and shellfish harvesting closures are now a regular occurrence in almost all of Puget Sound (Rensel, 1993; Trainer et al., 2003; Lewitus et al., 2012). Harvest closures related to ASP first occurred during 1991 and were widespread throughout Washington, Oregon, and northern California (Wekell et al., 1994). Pseudo-nitzschia blooms have warranted numerous subsequent closures along the coast and associated estuaries, some lasting for over one year (Trainer et al., 2012). In contrast to PSP, only a few ASP closures have occurred in Puget Sound (Trainer et al., 2003). The first documented cases of DSP in the Pacific Northwest (and the United States, for that matter), occurred more recently in 2011 when three people fell ill after consuming mussels they harvested locally in Sequim Bay, Washington (Trainer et al., 2013).
The first line of defense to protect local communities from the threat of various HABs in Puget Sound is provided by a citizen science monitoring program called SoundToxins4. SoundToxins is a partnership of shellfish growers, fish farmers, Native American tribes, environmental learning centers, local health jurisdictions, colleges, and volunteers. Participants are provided equipment and training to collect water samples and identify key HAB species, including Alexandrium, Pseudo-nitzschia, Dinophysis, and Heterosigma, at 28 locations near their growing areas or near their homes. The information is entered into a centralized database that alerts the Washington State Department of Health to sites where HAB species are identified and/or where their abundance is increasing so that they can identify and prioritize additional shellfish toxin analyses.
On the outer Washington coast, coordinated monitoring at six beaches by the Olympic Region Harmful Algal Blooms (ORHAB) program provides early warning of HABs of Pseudo-nitzschia (Trainer and Suddleson, 2005). ORHAB is a partnership of academic, federal, tribal, and state researchers and managers. The program was initially funded for 5 years by the National Oceanic and Atmospheric Administration (NOAA) Monitoring and Event Response to Harmful Algal Blooms (MERHAB) program but was successfully transitioned to an operational monitoring program funded by Washington State through a surcharge on recreational shell fishing licenses. The ORHAB program uses a simple combination of monitoring the abundance and toxicity of Pseudo-nitzschia; when cell concentrations exceed a size-dependent threshold (30,000 cells per L for large cells and 1 million cells per L for small cells), toxin testing of seawater particulates (e.g., filtered phytoplankton cells) is conducted. Like SoundToxins, the Washington State Department of Health uses ORHAB data to identify and prioritize additional shellfish samples for toxin analysis.
Beach monitoring does not determine whether a toxic bloom of Pseudo-nitzschia is lurking just off the coast. This is because ORHAB sampling is conducted at beaches, while the primary sources of toxic cells are offshore retentive oceanographic features, such as the seasonal Juan de Fuca eddy located off the Juan de Fuca Strait of the northern Washington coast (Trainer et al., 2009a). Retentive circulation, relatively low grazing rates compared to growth rates, and both macro- and micro-nutrient stress can contribute to the abundance and toxicity of Pseudo-nitzschia in the Juan de Fuca eddy, making it an initiation site for toxic HABs (Olson et al., 2008; Trainer et al., 2009a,b; MacFayden and Hickey, 2010). Toxic cells can escape from the eddy and be transported to the Washington coast during storm events when downwelling-favorable winds drive onshore flow (Figure 1). Immediately to the south-southeast of the Juan de Fuca eddy is the Northwest Enhanced Moored Observatory (NEMO), a real-time mooring system that is owned and operated by the University of Washington and the Northwest Association of Networked Ocean Observing Systems (NANOOS). In 2016, with funding from the NOAA IOOS Ocean Technology Transfer program, NEMO was enhanced with an advanced, remote, autonomous, near real-time HAB biosensor called the Environmental Sample Processor (ESP). The ESP uses sensitive and specific molecular assays to quantitatively detect Pseudo-nitzschia and domoic acid (DA) using sandwich hybridization assay and competitive enzyme-linked immunosorbent assay, respectively (Doucette et al., 2009; Scholin et al., 2009). The results of the assays are captured in a photograph that is relayed via telemetry in near real-time. The entire process, from sample collection through results delivery, takes as little as 3 h. The ESP is deployed on NEMO during high risk seasons when blooms could interfere with shellfish harvest (i.e., spring and fall). The data from the ESP are served by the NANOOS Data Visualization System (NVS) and the “Real-time HABs” application5 that NANOOS developed. This website incorporates contextual data and other data products to enhance interpretation and understanding of the ESP data (e.g., 1–3-day advection forecasts illustrating horizontal water transport calculated from current meter data) by state and tribal resource managers and other interested parties.
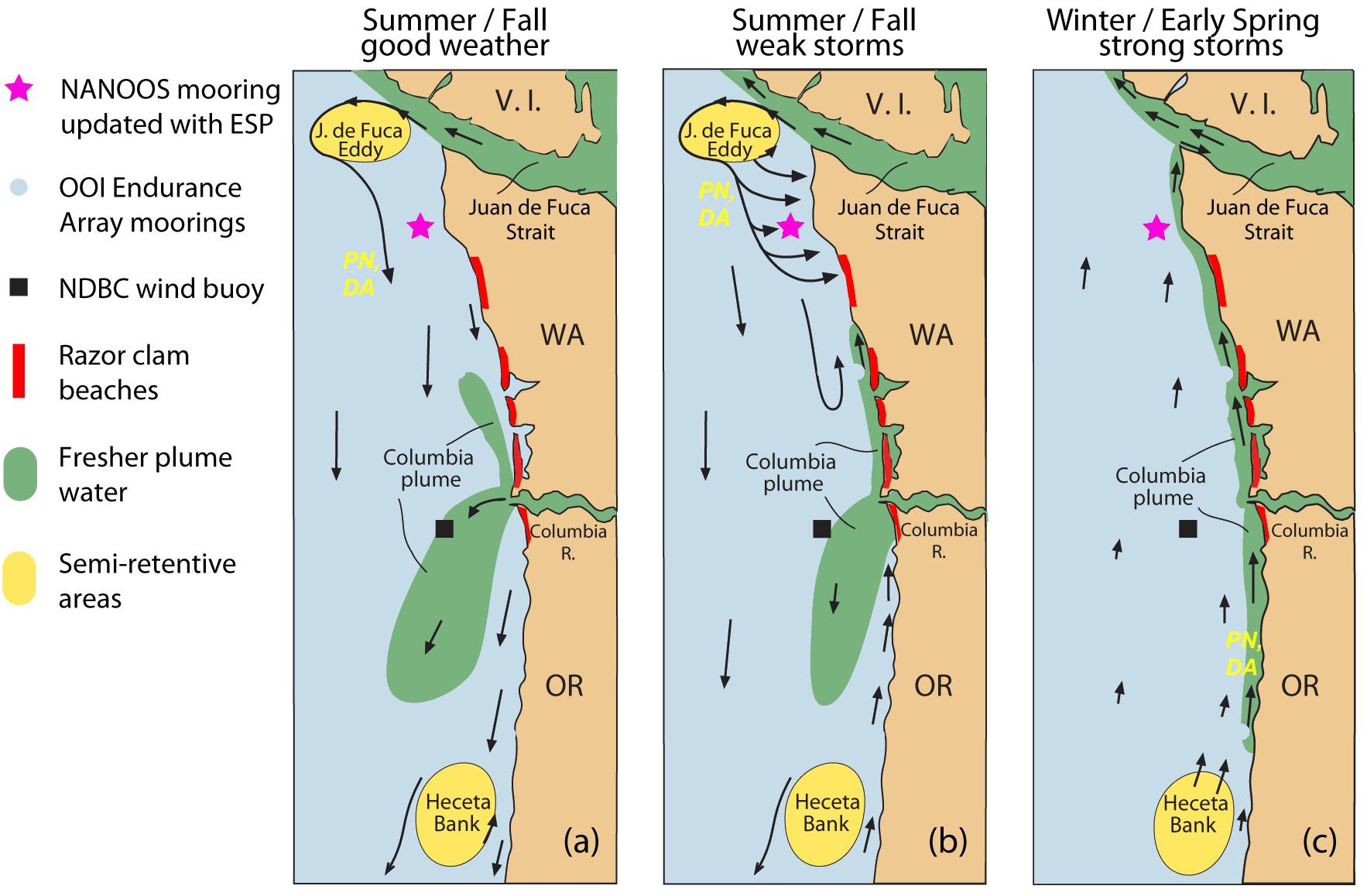
Figure 1. Schematic diagram of the outer Washington coast showing the locations of semi-retentive oceanographic features that are known to be HAB initiation sites (i.e., the Juan de Fuca eddy and Heceta Bank), ocean observing platforms, razor clamming beaches, and the transport pathways during (a) summer/fall good weather, (b) summer/fall weak storms, and (c) winter/early spring strong storms. PN and DA are Pseudo-nitzschia and domoic acid, respectively, and indicate the pathways that toxic HABs can take from HAB initiation sites to coastal beaches. Figure modified from Hickey et al. (2013).
When available, near real-time ESP observations of Pseudo-nitzschia and DA inform a forecast product called the Pacific Northwest HAB Bulletin that currently is being transitioned from a research- to an operational product. Once operational, the most current PNW HAB Bulletin will be distributed to managers and other partners on the NANOOS website where archived Bulletins now can be viewed6. This product was originally developed through a partnership among NOAA Northwest Fisheries Science Center, the University of Washington, and the Centers for Disease Control and has since been funded by the NOAA MERHAB program as a collaboration with multiple partners and is now distributed to managers. The forecast is grounded in a mechanistic understanding of Pseudo-nitzschia bloom dynamics in the Pacific Northwest that has accumulated over two decades of research (Brown et al., 2012). ORHAB data and oceanographic data from NANOOS and other partners describing the environmental conditions that are known to support toxic bloom development in the eddy and bring toxic cells onshore to coastal beaches are synthesized by local experts to forecast HAB risk. The level of risk is communicated using a “traffic light” approach, where a green symbol indicates low risk and a red symbol indicates high risk. Placing the ESP in the pathway of toxic HAB transport to coastal beaches complements beach monitoring conducted as part of ORHAB and vastly improves the ability to forecast HABs via the Pacific Northwest HAB Bulletin.
Harmful algal blooms forecasting efforts in the Pacific Northwest are a premier example of how collaborative research and monitoring forms the foundation for valuable risk assessments of coastal hazards. Sustaining a system that allows for the integration of advanced technology and near real-time forecasts will allow for local tribes and state agencies to be proactive in their monitoring and management of coastal resources.
California
The leading HAB issue in California is Pseudo-nitzschia and the threat of ASP from DA via recreationally and commercially harvested molluscan and crustacean shellfish. These blooms occur annually and cause devastating impacts to local economies through fishery closures (McCabe et al., 2016) and, unlike in the Pacific Northwest, commonly lead to unusual mortality events (UMEs) in seabirds and marine mammals (e.g., Lefebvre et al., 1999; Scholin et al., 2000). Historically, ASP has been managed by state agencies: California Department of Public Health (CDPH), California Department of Fish and Wildlife (CDFW), and the Office of Environmental Health Hazard Assessment (OEHHA). Rigorous and routine academic involvement in monitoring for HABs began in 2008 with the advent of the California Harmful Algal Bloom Monitoring and Alert Program (Cal-HABMAP) that conducts weekly nearshore surveys at major piers and harbors of eight critical HAB taxa (e.g., Alexandrium spp, Akashiwo sanguinea) via light microscopy and the DA toxin via ELISA and LC-MS (Kudela et al., 2015). The program builds on NOAA MERHAB support and is sustained with support from the Southern California Coastal Ocean Observing System (SCCOOS) and the Central and Northern California Ocean Observing System (CeNCOOS). Immediate, qualitative results are reported through an email listserv to managers and interested stakeholders with periodic guidance from a standing steering committee comprised of state, federal, and academic partners. The quantitative and curated data are shared in a SCCOOS public archive. These data are progressively being made Darwin-Core compliant before being stored within the NOAA Environmental Research Division’s Data Access Program (ERDDAP) for eventual ingest by the global Ocean Biogeographic Information System (OBIS) and by the United States IOOS Environmental Sensor Map7. The latter is true for all United States IOOS Regional Association data sets.
In response to the need for a predictive HAB capability in California, the California Harmful Algae Risk Mapping (C-HARM) System was developed to inform when and where toxic blooms occur to better inform management decisions. The NASA, NOAA National Centers for Coastal Ocean Science (NCCOS), and CA Ocean Protection Council-funded C-HARM project successfully generated and validated routine nowcast and forecast products in a research-to-operations demonstration of predictions of toxigenic Pseudo-nitzschia blooms and DA along the central California coast. Statistical ecological models are built from mechanistic understanding of blooms and driven in near real-time by information from hydrodynamic model simulations (Regional Ocean Model System) with three-dimensional variational data assimilation, satellite imagery, and community (Cal-HABMAP)/marine mammal observations (Figure 2; Anderson et al., 2016). Data that are routinely assimilated into the hydrodynamic model that are SCCOOS and CeNCOOS-supported are: 1) operational, autonomous, underwater glider data (most are Spray gliders8 and 2) daily high-frequency radar (HFR) measurements of surface currents (62 radar in CA9). Optical parameters required by the ecological models are retrieved from daily 1-km MODIS-Aqua satellite data and enhanced using Data Interpolating Empirical Orthogonal Functions (DINEOF) (Beckers and Rixen, 2003; Figure 2).
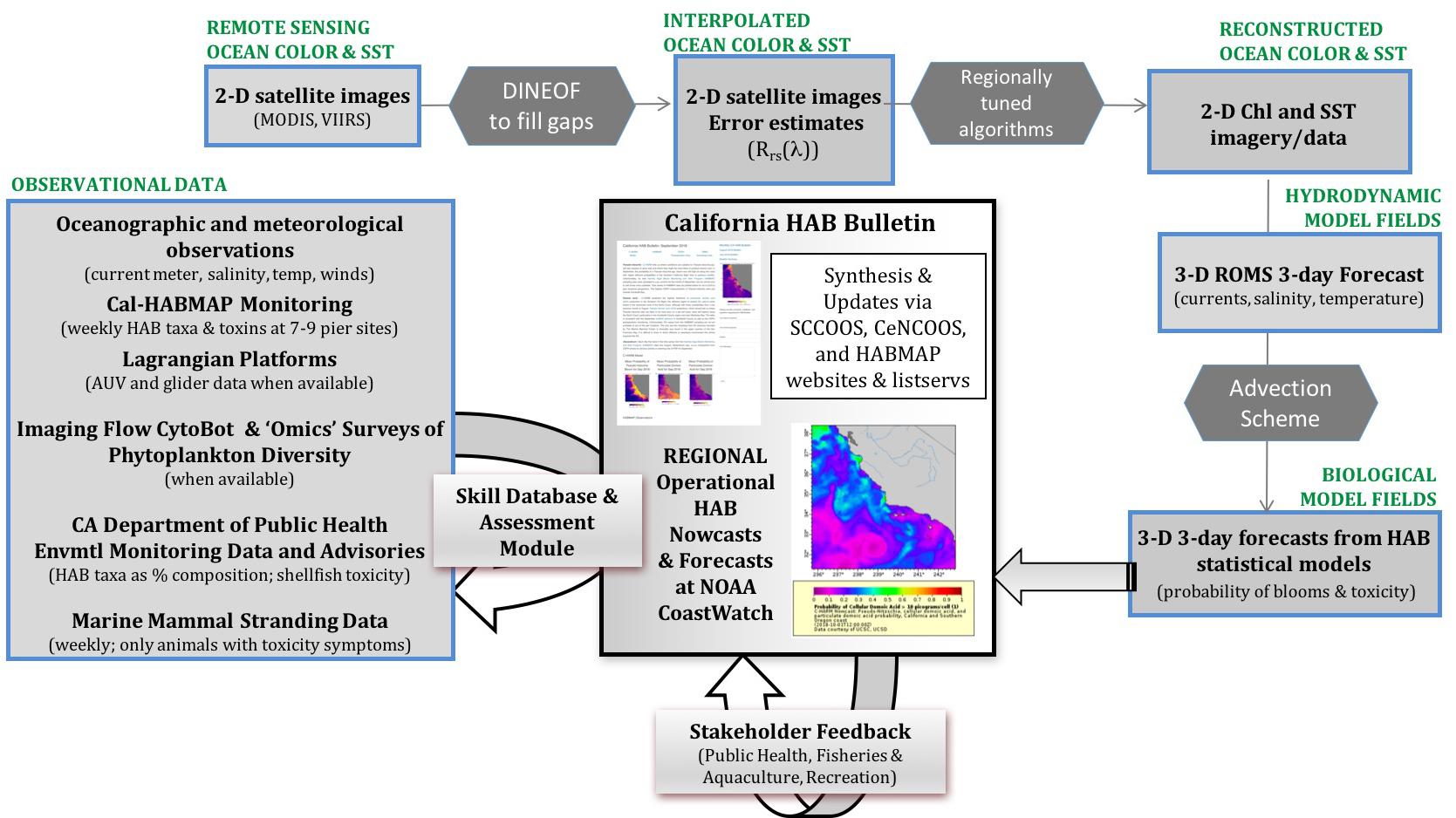
Figure 2. An integrative schematic of the in situ data sets, remote sensing imagery, and model output that support production of the C-HARM system for blooms and domoic acid events. Stakeholder engagement is conducted via the monthly CA HAB Bulletin. Modified from Anderson et al. (2016).
With the recent transition to operations of C-HARM at NOAA CoastWatch, this joint academic-government contribution will hopefully aid natural resource managers with early warnings of unusual mortality events of marine mammals, DA risk in wild fish stocks, and the need for more targeted sampling at commercial aquaculture sites. Pixel-level time series of model output are accessible via the CeNCOOS data portal, and all nowcast and forecasts can be easily visualized on the ERDDAP. Model validation is performed relative to the nearshore Cal-HABMAP sampling but would be greatly enhanced if offshore data were available to compare with the 3-km horizontal grid scale. Commercial fishing associations, marine mammal rescue groups, and public health managers provide frequent feedback on the utility of HABMAP and C-HARM and are engaged in the production of a California HAB Bulletin that synthesizes C-HARM predictions, HABMAP observations, marine mammal stranding records for DA toxicosis, and CDPH phytoplankton monitoring results as a monthly retrospective to stakeholders and to the public (Figure 3). The Bulletin is distributed via an electronic mailing list and publicly displayed on the SCCOOS10 and HABMAP11 websites.
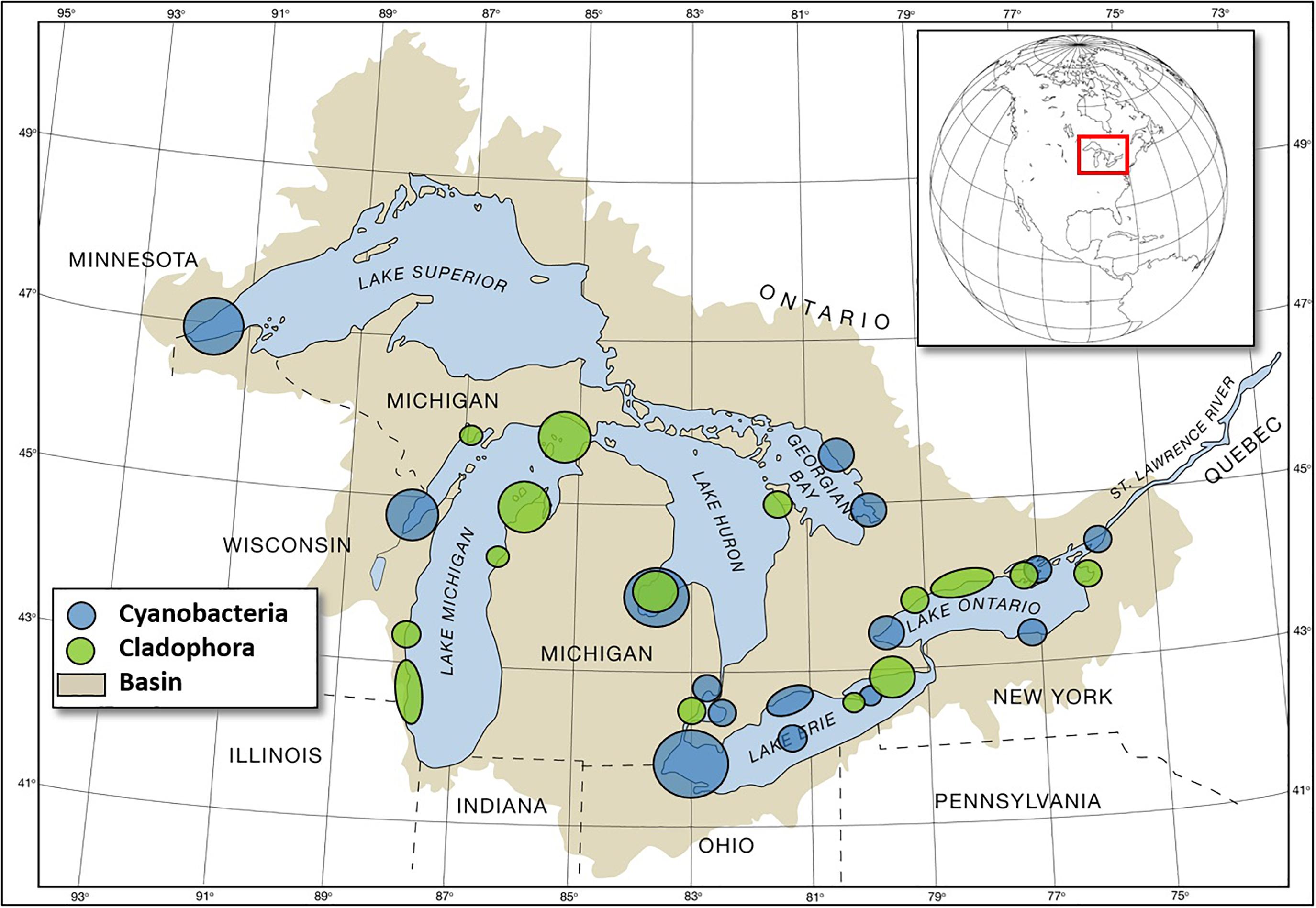
Figure 3. Map of reported cyanobacterial and Cladophora HAB locations in the Great Lakes basin. (Graphic from National Science, and Technology Council [US] (2017); courtesy of GLERL/OAR/NOAA).
Integration of the Imaging Flow CytoBot (IFCB; Sosik and Olson, 2007; essentially an underwater microscope capable of collection and automated classification of water samples every ∼20 min) into the California observational repertoire has allowed monitoring of high-frequency variability in phytoplankton biodiversity at pier sites and as part of the shipboard time series (Kudela et al., unpublished data; Cloern, 2018), and may lead to the ability to detect HABs at their inception. Genetic surveys of Pseudo-nitzschia populations in Monterey Bay are conducted in tandem with deployments of the ESP in Monterey Bay on both moored and autonomous glider platforms (Scholin et al., 2009; Ryan et al., 2011), providing essential molecular information for understanding the subtle evolutionary variations driving toxic DA events (Bowers et al., 2016). We imagine that a new generation of small, easily deployed and even free-drifting swarms of autonomous sensors (Jaffe et al., 2017) for moieties like dissolved toxins and/or a network of moored and autonomous instruments like the IFCB and ESP will enable true early warning of blooms in the California Current System. As mentioned, a major observing gap is in the offshore environment where HAB data are critical for properly validating the moderate resolution C-HARM model output and for tracking offshore initiation of blooms. Another knowledge gap is a quantitative understanding of the transfer of DA from phytoplankton to higher trophic levels such that accurate predictions of food web impacts can be delivered to stakeholders.
Ultimately, the key to continued success of the California response to HABs is (1) strong communication between the many partners, (2) a better understanding of offshore bloom initiation, coastal-to-nearshore connectivity, and food web transfer of toxins to produce more accurate forecasts, and (3) continued investment by the state to ensure that rapid alerts are issued to the fishing and aquaculture communities as well as the public.
The Gulf of Mexico
The five US states bordering the northern Gulf of Mexico, Texas, Louisiana, Mississippi, Alabama, and Florida have all experienced HABs in their coastal waters. Karenia brevis is the most prevalent species in the Gulf of Mexico and is responsible for annual blooms that not only contaminate shellfish but also cause massive fish, marine mammal, and sea turtle mortalities. K. brevis produces a suite of neurotoxins, brevetoxins, that are not only released into the water but, when subjected to wind and wave action, are converted to marine aerosols (Pierce et al., 2005; Cheng et al., 2005). When inhaled, these airborne toxins cause respiratory distress in people, especially those who have asthma and/or other underlying lung diseases (Fleming et al., 2007; Kirkpatrick et al., 2011). Observations of K. brevis blooms are acquired primarily through traditional means, such as grab samples and microscopic enumeration. In recent years, new technology, such as the IFCB, has provided in situ monitoring off Texas (Campbell et al., 2013), and the Optical Phytoplankton Discriminator (“a.k.a. BreveBuster”) has been deployed on both fixed platforms and autonomous underwater gliders in southwest Florida (Shapiro et al., 2015).
Current regulations mandate commercial shellfish harvest closures if K. brevis reaches 5000 cells per L, and it is the only HAB which has regulatory action tied to cell counts rather than toxin measurements. Heil and Steidinger (2009) published a summary of the monitoring for K. brevis in the Eastern Gulf of Mexico. The Florida Department of Agriculture is now the agency responsible for regulating shellfish harvest areas, while the Florida Fish and Wildlife Conservation Commission (FWC) is responsible for monitoring marine HABs in the state. They collate and analyze samples from numerous field partners, including citizen volunteers, and produce a weekly to bi-weekly status reports and hosts and interactive mapping tool12. The University of South Florida issues remote sensing products and forecasts of ocean currents to help stakeholders track K. brevis and associated impacts. Since 2004, NOAA has been producing the first operational HAB Bulletin weekly for the Gulf of Mexico13 (Stumpf et al., 2009). Texas Parks and Wildlife posts status reports only when levels above background (1,000 cells/L) have been detected14. In Alabama, the monitoring is conducted by the Alabama Department of Public Health15. In Mississippi, the Department of Marine Resources monitors for K. brevis but does not post routine reports16. The Louisiana Department of Health’s Molluscan Shellfish Program samples for K. brevis based on reports from surrounding states (TX and MS)17. Coordination across state lines occurs when a bloom covers multiple state waters or there is an unusual event warranting assistance for accurate species identification and biotoxin measurements. Coordination between the United States and Mexico for K. brevis has been via the United States Environmental Protection Agency Gulf of Mexico Program (Soto et al., 2012). The collaboration provided capacity building, technology transfer, and joint research efforts between the two countries.
Citizen science programs are emerging as an effective approach for improving spatial and temporal coverage of HAB observations that directly support models and forecasts. In Texas, a group of Master Naturalists known as the Red Tide Rangers assist the state with sampling during a bloom event. On the west coast of Florida, lifeguard and park rangers file reports from a smartphone daily to provide qualitative information that a beach goer might be interested in – from the presence or absence of dead fish on the beach, to rip currents and wind speed and direction (Kirkpatrick et al., 2008). This program was implemented in 2006 and currently has 33 reporting sites. Two other ongoing projects also employ citizen science. The Nucleic Acid Sequence Based Amplification (NASBA) project trains volunteers to process water samples through a hand-held, battery-operated sensor that uses isothermal amplification coupled with fluorometry to detect and quantify K. brevis RNA using species-specific molecular beacons (Casper et al., 2007). The data are uploaded to a portal at the Gulf of Mexico Coastal Ocean Observing System (GCOOS) that estimates a cell count based on the RNA amplification profile relative to standards instead of the amount of RNA detected. A second project trains volunteers to process water samples using a microscope connected to a smartphone. Instead of counting cells, the volunteer takes a video of the sample. The video is then uploaded to a separate GCOOS portal, and deep learning software uses machine learning to automatically estimate a cell count from the video. The goal of this innovative sampling strategy is to reduce technical expertise/knowledge required by traditional microscopy and provide cell counts at a higher spatial and temporal scale than previously possible (Hardison et al., in prep). Daily input of data supports high-frequency forecasts of aerosolized toxins at beaches in three-hour time intervals. Beach goers can then decide where and when to visit beaches in order to minimize exposure to the toxic aerosols18.
Due to the skill required for microscopic cell enumeration and the level of training required for accurate quantitation, stakeholders in the Gulf of Mexico are exploring new approaches for HAB observations. Some of these involve automation such as the ICFB and OPD, and some require employing citizen scientists to improve the spatial and temporal resolution of observation. Continuing to improve collaboration across state lines and international borders is also needed.
Gulf of Maine
The economic impact of HABs in the Gulf of Maine can be severe; a single fishery closure from a HAB threat in Massachusetts resulted in a loss of $18 million in 2005 (Jin et al., 2008). Alexandrium fundyense and associated PSP has historically been the focal HAB threat to human health in the Gulf of Maine, which is bordered by the United States states of Maine, New Hampshire, and Massachusetts, as well as by the Canadian Provinces of New Brunswick and Nova Scotia. Research monitoring efforts use ship-board surveys of cells in the water column as well as mapping of benthic cysts (Anderson et al., 2005, 2014) and more recently automated measurements with the ESP and IFCB (Brosnahan et al., 2015) to track bloom risk and severity. Coupled physical-biological models that combine the HAB population dynamics and hydrodynamics have facilitated hindcast and process-oriented studies (McGillicuddyJr., Anderson et al., 2005; He et al., 2008; Li et al., 2009). An annual cyst map is an important initial condition for the coupled biological-physical models that are also used for seasonal to sub-seasonal forecasts and to examine the effects of water mass anomalies when combined with observations (McGillicuddyJr., Townsend et al., 2011). The forecast configuration that uses ROMS is currently being transitioned into operations within the NOAA Center for Operational Oceanographic Products and Services (CO-OPS). More recently, blooms of Pseudo-nitzschia have led to unprecedented shellfish closures in Maine and Rhode Island, but not in Massachusetts, due to detection of ASP toxins.
Despite significant progress in understanding and managing HABs in the Gulf of Maine, many challenges remain. The three US states that border the Gulf of Maine all have monitoring activities focused on phytoplankton monitoring and toxin levels in shellfish. Information from these state monitoring activities, research activities, and the NOAA forecasts are shared via a controlled electronic mailing list. In addition to the state shellfish and phytoplankton monitoring, much of the work being funded is connected to research grants or rapid response projects. While there are routine oceanographic observations capable of discerning water masses in the region as part of the Northeastern Regional Association of Coastal Ocean Observing Systems (NERACOOS), there are no routine HAB observations to validate the model currently being transitioned to operational status at NOAA.
During a recent stakeholder workshop, the need for these observations and higher spatial resolution modeling along the coast was highlighted. The concept of a regional IFCB network for HAB early warning and better integration of all HAB data at a regional scale were also thought to have great potential.
United States Laurentian Great Lakes
Great Lakes HABs in freshwater, represented mostly by an array of cyanobacteria and submerged aquatic vegetation that disrupt ecosystems, damage local economies, and impact drinking water supplies. The Great Lakes case study is focused on the development of a Cyano-HAB observational capacity and forecasts by the NOAA National Ocean Service and OAR. However, comparable observational and forecasting development efforts are currently underway by the EPA, NOAA, United States Geological Survey (United States GS), and university partners to address the impact of the submerged aquatic vegetation Cladophora (Figure 3).
Great Lakes Cyano-HABs include Microcystis, Dolichospermum, Aphanizomenon, Planktothrix, and Lyngbya (Figure 3; National Science, and Technology Council [US], 2017). Observing and forecast systems have been developed for Lake Erie and are in development for other areas including Saginaw Bay on Lake Huron and Green Bay on Lake Michigan. The Lake Erie forecasts and observing systems were developed in response to a highly toxic bloom event in 2014 that resulted in the shutdown of the Toledo, OH drinking water intake (Steffen et al., 2017).
Forecast systems on Lake Erie addressing toxic Microcystis blooms include an operational forecast bulletin and a seasonal HAB ensemble forecast (Wynne and Stumpf, 2015). The seasonal ensemble forecast combines forecast products from NOAA, industry, and university partners and relies on satellite remote sensing time series and phosphorus loading data from the Maumee River (Stow et al., 2015; Wynne and Stumpf, 2015). Daily to weekly forecast bulletins, weekly sampling, the HAB Tracker19, real-time buoys (Ruberg et al., 2007), and hyperspectral flyovers20 provide a combination of environmental information decision support tools for regional managers. Forecast bulletins21 produced by the NOAA CO-OPS, NCCOS, and the Great Lakes Environmental Research Laboratory (GLERL) employ satellite remote sensing and coastal circulation to generate a five-day HAB outlook combined with bloom toxicity results obtained from weekly sampling and the deployment of near real-time ESPs (Scholin et al., 2009; Ryan et al., 2011). Real-time buoys provide hourly observations22 of parameters such as winds, waves, phycocyanin (a characteristic pigment of cyanobacteria), chlorophyll, and nutrients to provide awareness of HAB development and to distinguish wind-driven resuspension of nutrient inputs from river contributions. Weekly flyovers of drinking water intakes using a hyperspectral imaging sensor (Vander Woude et al., 2019) provide observations in the nearshore and below the cloud layer that usually interferes with satellite remote sensing retrievals. Many of these observations are accessed through the Great Lakes Observing System (GLOS) HAB Data Portal23.
Great Lakes HABs observations are becoming increasingly automated as two additional Monterey Bay Aquarium Research Institute (MBARI) Second Generation ESPs will be deployed in Lake Erie with the real-time capacity to detect microcystin. With the successful demonstration of the MBARI Third Generation (3G) ESP autonomous underwater vehicle (AUV) during the 2018 HAB season with NCCOS, OAR, and the Cooperative Institute for Great Lakes Research (CIGLR) partners, further demonstrations of the 3G AUV system are planned for 2019 testing of real-time saxitoxin and microcystin sensors.
This combination of forecast and observational tools currently provides the awareness that was lacking when HAB toxins impacted the Toledo water intake in 2014. Information is now available to drinking water managers and the public providing advanced warning of changing lake conditions. This information is valuable for planning drinking water treatment as well as coastal recreational activities. Future research is now focused on the reliable detection and forecasting of HAB toxicity.
The United States Caribbean Islands
The Caribbean region is mainly affected by two significant HABs: “golden tides” caused by the accumulation of the macrophyte Sargassum in coastal zones and ciguatera fish poisoning, endemic to tropical areas, due to the consumption of fish contaminated by ciguatoxins synthesized by unicellular benthic microalgae (see below). These are very different types of HABs that require specialized observing approaches.
Pelagic Sargassum constitutes a unique habitat for fishes and invertebrates. For this reason, the Sargasso Sea, an area where these macroalgae naturally proliferate was identified as an Ecologically or Biologically Significant Area (EBSA) by the Convention on Biological Diversity24. Sargassum is transported by ocean currents to coastal zones, and in the last 15 years, in particular in 2014–2015 (Schell et al., 2015; Hu et al., 2016), massive accumulations (100 kg wet wt/m2) have been documented in bays and beaches of the Gulf of Mexico, Caribbean Islands (as well as in West African Atlantic coasts). Sargassum beachings cause economic losses to coastal fisheries and tourism, alter the dynamics of the shoreline ecosystems, and the hydrogen sulfide produced by the seaweed decomposition can also affect human health (Dabor et al., 2018; Langin, 2018). These negative impacts have fostered research to understand the factors involved in Sargassum bloom dynamics. An improved understanding of the spatial distribution (from open sea to coastal zones) in biomass, including seasonality, inter-annual variability, and long-term trends is achieved thanks to recent advances in remote sensing of Sargassum using Landsat, MODIS, and MERIS. Two systems, SEAS (Webster and Linton, 2013) and Satellite-based Sargassum Watch System (SaWS) (Wang and Hu, 2016, 2017), are now operational. The Sargassum Outlook monthly bulletin25 offers a quick assessment of the magnitude of the Sargassum blooms since 2011 and strongly suggest that Sargassum concentrations during 2018 have exceeded the 2015 event (Figure 4). Observations have revealed the existence of a recurrent “Sargassum belt” extending from western Africa to the Caribbean Sea and Gulf of Mexico (Franks et al., 2016; Putnam et al., 2018) that has potentially created a “new Sargasso Sea” (Gower et al., 2013). CARICOOS, the Caribbean Coastal Ocean Observing System, publishes daily imagery of Floating Algae Index in the region (Franks et al., 2016)26 issued by the Optical Oceanography Laboratory at the University of South Florida. An ongoing effort led by H. Hoarty at Rutgers University and supported by CARICOOS (Prakash et al., 2018) utilizes surface current data derived from high-frequency radar (HFR) coupled to satellite imagery to forecast Sargassum arrivals into the CARICOOS region.
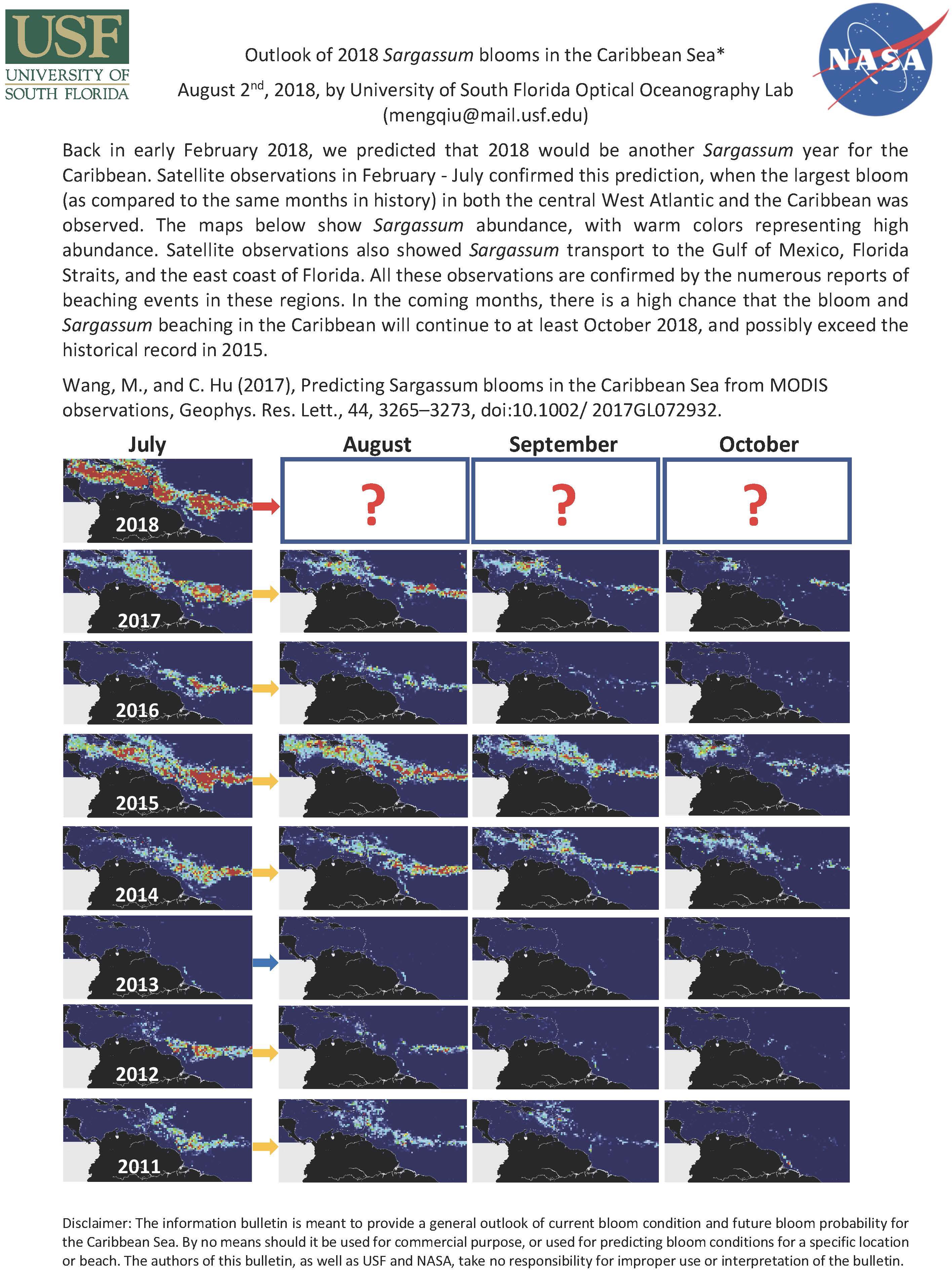
Figure 4. University of South Florida Sargassum Outlook monthly bulletin for Sargassum abundance in the Caribbean from SaWS (Satellite-based Sargassum Watch System).
Ciguatera fish poisoning (CFP) is caused by the ingestion of fish (e.g., Friedman et al., 2017 and references therein) or shellfish (Darius et al., 2017) contaminated with neurotoxins (ciguatoxins and/or maitotoxins) produced by the benthic dinoflagellates in the genera Gambierdiscus and Fukuyoa. CFP is the most frequently reported, non-bacterial illness endemic in tropical regions and produces a complex array of acute gastrointestinal, neurological/neuropsychological, and cardiovascular symptoms that can become chronic after repeated exposure to these toxins. Recent improvements in sampling, taxonomic characterization, and sophisticated toxin detection indicate that CFP-producing species may be spreading from tropical to temperate latitudes due to global warming (Tester et al., 2010; Laza-Martínez et al., 2016; Rodríguez et al., 2017; Nishimura et al., 2018). Phenological changes may also be occurring in CFP-prevalent areas such as the Caribbean Sea (Tosteson, 2004; Nakada et al., 2018). Protecting human health requires efficient monitoring of the benthic species and their toxins, seafood, and improved epidemiology surveillance.
New approaches, such as the artificial substrate sampling method (Tester et al., 2014), have been successful in detecting new benthic species worldwide. The challenge is to prevent CFP in local populations whose main protein source is seafood, and one possible solution would be the banning of commercial sales of species that have been consistently linked to poisoning events, such as barracuda (Reglamento de Pesca de Puerto Rico, 200427). Recent studies suggest that the spatial distribution of toxic fish is defined by environmental gradients in grazing (Loeffler et al., 2018), wave energy (Loeffler et al., 2015), and temperature. These results suggest the possibility of establishing guidelines for “no take” zones as a risk management strategy. CARICOOS acquires environmental data, including wave observations and models, which has been key for identifying conditions linked to ciguatoxin concentration in commercially harvested fishes in the Virgin Islands (Figure 5).
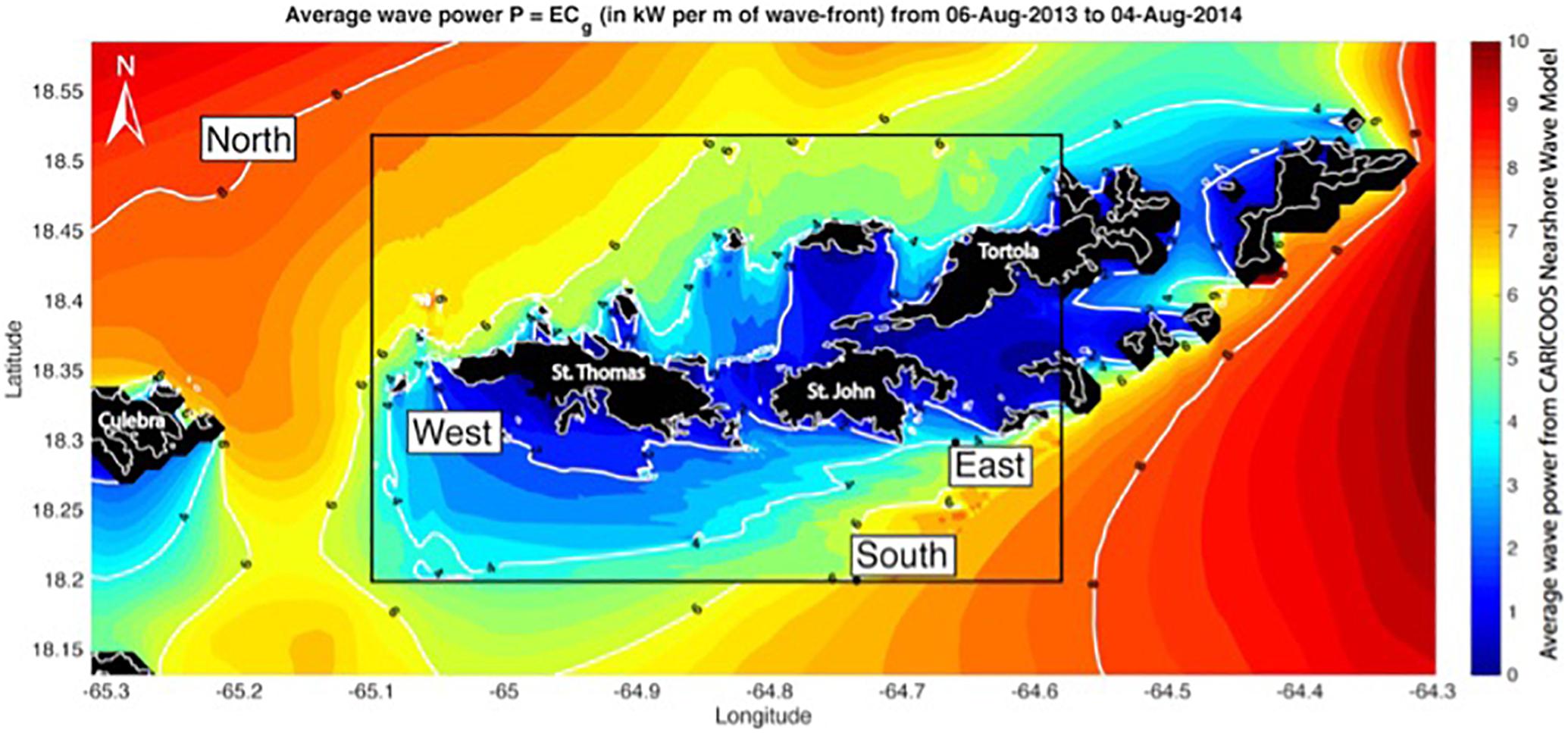
Figure 5. Average wave power per unit wave crest in the United States Virgin Islands computed using model output from the CARICOOS Nearshore Wave Model. Site locations are within the white boxes (Loeffler et al., 2018).
Precise toxin detection and quantification is possible by LC-MS in accredited laboratories and by fluorescence-based receptor binding assay (RBA-F), Hardison et al., 2016), an alternative method used to screen fish samples for ciguatoxins in facilities that are not certified to use isotopes. However, the development of affordable, reliable, and fast detection methods available to the general population and medical community is fundamental. The protection of human health also requires efficient diagnosis and reporting. The annual prevalence of CFP worldwide has been estimated to be around 50,000–100,000 cases, but these values could represent only 20% of actual cases, due to misdiagnosis and under-reporting (Gatti et al., 2018). Thus, in addition to monitoring Gambierdiscus and its toxins, efficient public health surveillance is mandatory. A successful approach has been implemented in French Polynesia after 20 years of effort (Morin et al., 2016; Gatti et al., 2018), making this region a reference for other areas of the world. Since 2007, the multidisciplinary and coordinated research between the Institut Louis Malardé (ILM) and the Public Health Directorate of French Polynesia have facilitated assessing the current CFP status on different archipelagos, provided a quantitative estimate of the health-related costs imputed to this illness, established outreach activities to document the risk perception and describe associated avoidance strategies among tourists, and professional and non-professional fishermen and, identified novel vectors of ciguatera poisoning.
In summary, intrinsically different organisms, macroalgal Sargassum and microscopic Gambierdiscus, threaten human health and fragile economies in these tropical areas. In this context Sargassum and Gambierdiscus are fundamental ecological variables that can structure coordinated regional and observing systems in the Caribbean and Gulf of Mexico. CARICOOS through satellite observing and dissemination tools is proving suitable as an early warning system for the detection of massive Sargassum beachings along Caribbean coasts. In coordination with other research technologies, CARICOOS can contribute to understanding Sargassum biology and bloom dynamics and will help to design mitigation strategies on the massive biomass accumulations in coastal areas. Finally, effective monitoring of benthic dinoflagellates and ciguatoxins is a main challenge for the tropical areas and require efforts on new technologies. Here, international cooperation in the development and implementation of cost-effective sampling and probes will be decisive for protection of human health in economically fragile areas.
Asia
Harmful algal blooms in Asia have long been recognized as a serious threat in coastal ecosystems, fisheries, and public health. The best documented impacts are associated with “red tide” outbreaks of Noctiluca, Trichodesmium, Heterocapsa, and Cochlodinium (now Margalefidinium) (GEOHAB, 2010b; c.f. Red Tides in Korea special issue, Harmful Algae 30, 2013), although massive green tides, such as blooms of the macroalgae Ulva ( = Enteromorpha) prolifera and Cladophora species as well as the green variant of the dinoflagellate Noctiluca scintillans, are also prevalent, particularly in the Yellow Sea (GEOHAB, 2010b; Liu and Zhou, 2018). Since the 2000s, other harmful organisms have caused massive fish kills and economic disruption in regions where large-scale HAB events associated with these organisms were rare or unreported (c.f. Furuya et al., 2018). For example, Karenia mikimotoi has been identified with increasing frequency in China, Japan, Korea, Hong Kong, and Singapore (Okaichi, 2004; Qi et al., 2004; Leong et al., 2015), while the dinoflagellates Akashiwo sanguinea, Gonyaulax polygramma, Prorocentrum spp., and Scrippsiella trochoidea, as well as the diatoms Pseudo-nitzschia spp. and Chaetoceros spp., have emerged as HABs (c.f. Furuya et al., 2018). Known HAB organisms have also expanded to new regions, with (for example) Karlodinium australe causing massive fish kills in Johor Strait Malaysia in 2014 (Lim et al., 2014) followed by even more damaging blooms in subsequent years (Teng et al., 2016).
Direct toxicity in shellfish, finfish, and humans is also well documented, with more than 2,000 PSP cases reported in Southeast Asia, approximately 10% of which caused fatalities (Furuya et al., 2018), while other lipophilic toxins (okadaic acid, azaspiracid, pectenotoxin, yessotoxins, gymnodimine, and spirolides) and DA are also present (c.f. Furuya et al., 2018). As with other regions globally, benthic HABs including Coolia spp. and Gambierdiscus spp. are also emerging as potential threats.
Given the long history of HABs in Asia, many local monitoring programs have been in effect for decades, gradually developing into national and regional efforts. For example, a massive Margalefidinium polykrikoides bloom in Korean coastal waters in 1995 led the National Fisheries Research and Development Institute (NFRDI) to develop a national (South Korea) monitoring program that coordinates more than 30 local offices (Lee et al., 2013). Similar efforts to standardize research, monitoring, and prediction at a national level were implemented under the auspices of the Chinese Ecology and Oceanography of Harmful Algal Blooms (CEOHAB) and Ecology and Oceanography of Harmful Algal Blooms in the Philippines (PhilHABS) programs.
Beyond these local and national efforts, in response to the recognition of regional HAB threats, a regional plan has emerged for HAB research focused on cooperation in Asia. This developed out of two meetings (Tokyo, Japan, 2007. and Nha Tang, Vietnam, 2008) held in coordination with IOC/WESTPAC and the IOC HabViet Program, culminating in the GEOHAB Asia Regional Comparative Program (GEOHAB, 2010b). The meeting report gave an overview of Asian HAB problems and provided a framework for guiding priorities for research. The need to develop a comparative, international program on HABs in Asia was particularly compelling because Asia (1) is characterized by the highest production of aquaculture fish and shellfish globally, and thus the greatest impacts from HABs on these resources; (2) has a diversity of harmful syndromes (documented briefly above); (3) is experiencing an apparent trend of increasing HABs throughout the region and an increasing trend toward regional eutrophication linked to HABs.
Today there are multiple regional coordinating groups in Asia, including IOC/WESTPAC-HAB (formed in 1989), The Northwest Pacific Action Plan (NOWPAP), a part of the Regional Seas Programme of the United Nations Environment Programme (UNEP), adopted in 1994, the North Pacific Marine Science Organization (PICES) section on HABs, formed in 2003, and the Targeted HAB Species in the East Asia Waters (EASTHAB) established in 2004 (GEOHAB, 2010b). All of these coordinating bodies support standardization of best practices across the region, training and capacity building, and dissemination of scientific knowledge. A key lesson learned from this successful approach is that successful programs recognize and develop interfaces among all actors in the region for their mutual benefit, with flexibility regarding implementation at the local, national, and regional levels.
As with other regions, multiple approaches have been taken to address the HAB problem in Asia. In addition to coordination and capacity building, many countries and regions have implemented integrated monitoring and modeling systems for both freshwater and marine HABs (e.g., PICES, 2014; Jeong et al., 2015; Srivastava et al., 2015; Furuya et al., 2018; Yñiguez et al., 2018; Yu et al., 2018), often relying heavily on satellite remote sensing (e.g., Ahn and Shanmugam, 2006; Ye et al., 2011; Lee et al., 2013) for high biomass blooms. Many of these programs continue to innovate as new technologies become available. In Korea, for example, monitoring programs are evaluating the use of acoustics (Kang et al., 2016), molecular methods such as digital PCR (Lee et al., 2017), unmanned aerial vehicles (Oh et al., 2016), and new satellite platforms such as the Geostationary Ocean Color Imager (GOCI) coupled to numerical models to provide real-time decision-support tools for managers (Kim et al., 2017).
Asia is also a region where more proactive, mitigative measures have been refined, particularly the use of coagulants for high-biomass blooms, but also various biological and chemical methods (c.f. Yu et al., 2018). For clay flocculation, the basic principle is that charge differences between the flocculating agent and cell membranes causes adsorption via ionic bonding, and the algal cells sink from the water column. Natural (Park et al., 2013) and modified clays (Yu et al., 2017) are the prime mitigation techniques in South Korea and China, respectively, and have been used elsewhere, including freshwater systems (Li and Pan, 2013). Other mitigation used in Asia and elsewhere includes addition of chemicals, ozonation, electrolysis, and acoustic disruption [reviewed by Anderson et al. (2017)]. Biological control has also been proposed in Asia. Jeong et al. (2003) have proposed using heterotrophic dinoflagellates to control HAB events, while Imai et al. (2006) and Onishi et al. (2014) have identified plant- or macroalgae-associated naturally occurring bacteria that have lytic effects on a variety of HAB organisms, including Heterosigma akashiwo, Karenia mikimotoi, Alexandrium tamarense, while Shi et al. (2018) isolated a highly algicidal bacterial strain naturally occurring in a bloom of Prorocentrum donghaiense, leading to the proposed use of algicidal bacteria as a direct mitigation strategy (Meyer et al., 2017).
While these methods, particularly clay flocculation, have proven effective for targeted mitigation, there is also a strong need for continued research on the economic and ecological impacts of HABs, on observation and prediction, as well as on control of land-based pollution and coastal eutrophication to reduce the rapid proliferation of HABs in the region (Glibert, 2014; Yu et al., 2018).
The European Union (EU)
Throughout the 1990s, an early HAB warning system was identified as a key industry need to help farms plan seafood harvesting schedules in order to avoid severe financial losses after a series of prolonged closures in shellfish production areas. A reliable HAB warning system would give producers a chance to change their husbandry practices, e.g., avoid handling shellfish, proactively relocate stocks, harvest shellfish earlier or cancel a delivery in order to minimize losses and waste due to contaminated stock, or, increase harvesting prior to a HAB event. Scientists, industry, and regulators began to discuss how this accumulated knowledge could help assess the likely risk of HABs.
In the early 2000s, a number of research projects were initiated (e.g., the internationally funded HABWATCH, the European fifth and seventh Framework/Commission-funded HABES, HABBUOY, HABIT, MIDTAL, NEMEDA, and Irish BOHAB project) to investigate HAB transport, growth and physiology, biophysical interactions, and algal mortality in EU shellfish areas. The projects focused on enhancing the understanding of the environmental factors and oceanographic phenomena that influence HAB-shellfish toxic pathways, including the role of thin layers, horizontal advection, physical forcing mechanisms such as eddies, gyres, frontal systems, bottom density fronts, upwelling and downwelling events, seasonally formed bottom density fronts and associated jets, alongshore winds and wind exchange events (e.g., Raine et al., 2010; Raine, 2014). These scientific studies were also instrumental in the development and testing new technologies and methodologies (e.g., Lunven et al., 2005, 2012). New conceptual models were formulated using the observed, regional, physical forcing mechanisms and local circulation patterns, and knowledge of biophysical mechanisms that influence the distribution and cross shelf transport of HABs into aquaculture bays (Aleynik et al., 2016; Pinto et al., 2016). By 2008, it was clear that an integrated approach was needed to provide near real-time and forecast information services for the aquaculture industry. Armed with an improved understanding of HAB ecology, European scientists were in a position to exploit research outputs of past projects and work toward building a near real-time predictive HAB capacity, supported by the now well-established national phytoplankton and biotoxin databases.
In 2013, an EU-funded project, ASIMUTH (Applied Simulations and Integrated Modeling for the Understanding of Toxic and HABS), led by Ireland with partners from Portugal, Spain, France and Scotland, focused on HABs in NE Atlantic European shelf and coastal waters. The project endeavored to develop forecasting capabilities to warn of impending HABs (Davidson et al., 2016). Steps to achieve this included a series of scientific and technical objectives to enable the use of numerical modeling of physical – biological interactions to help forecast toxic shellfish events, fish mortalities, and ecological disruption from HABs. Ireland automated an operational service to provide a three-day prediction of water movements in the vicinity of Bantry Bay off the SW coast of Ireland, historical and current HAB and biotoxin data, and satellite-derived data products to produce a bulletin with expert interpretation of the likely HAB occurrence in the days ahead. A gap identified, as with many regions previously discussed, was that offshore observatories that provide near real-time information on HABs are missing. However, the ASIMUTH approach successfully demonstrated the power of integrating information and data products from multiple disciplines (remote sensing, physics, biogeochemistry, biology and ecosystems) to create a risk analysis/forecasting downstream service to assist farm management decisions to mitigate the potential negative HAB impacts (e.g., Cusack et al., 2016; Maguire et al., 2016; Ruiz-Villarreal et al., 2016). Data used to create the downstream service are available through the Copernicus Marine Environmental Monitoring Services (CMEMS), national biotoxin and phytoplankton monitoring programs, and from satellite providers (e.g., NASA and ESA data processed by IFREMER).
Active EU research projects such as CoCliME28 (Co-development of Climate services for adaptation to changing Marine Ecosystems co-funded by ERA4CS JPI-climate), PRIMROSE (Predicting Risk and Impact of Harmful Events on the Aquaculture Sector funded by Interreg Atlantic Area) and Alertox-Net29 (Atlantic Area Network for introduction of Innovative Toxicity Alert Systems for safer seafood products funded by Interreg Atlantic Area) foster interdisciplinary and transnational cooperation. Their efforts aim to improve the predictive capabilities of HAB events, enhance HAB management and monitoring practices, and support decision-makers through a co-development approach to clearly identifying stakeholder needs (Figure 6).
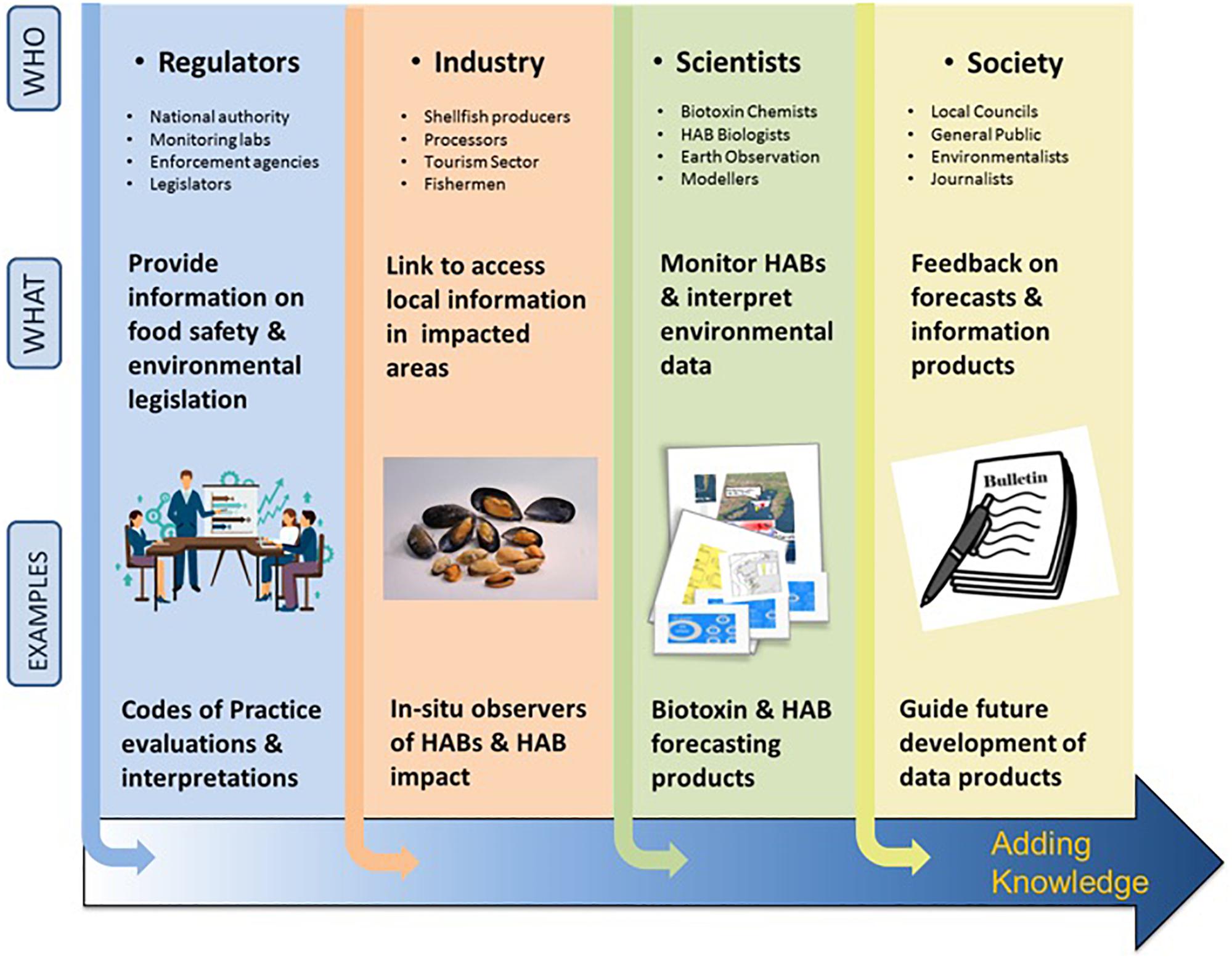
Figure 6. HAB value chain: application of knowledge along the pathway to forecasting bulletin production from different sectors, regulators, industry, scientists and society and examples of the knowledge they provide. “Users” of the products along the chain are vital as they provide expertise to determine the HAB forecast and other data-derived products (https://www.coclime.eu/project-overview/co-development-processes).
CoCliME focuses on the potential future climate-driven shifts of HABs, marine biotoxins, pathogens and marine microbial biodiversity that have direct impacts on human health (food-borne poisoning and water-quality related health disorders), economic prosperity (fisheries, aquaculture, tourism) and social well-being (recreation). Europe’s coastal and shelf seas are the target regions for the CoCliME case studies; areas where the greatest impact is felt from harmful microorganisms on populations and commercial sectors of both water quality and seafood safety. The overall CoCliME aim is to co-develop and co-produce bespoke, proof-of-concept or prototype marine ecosystem climate services. The transdisciplinary team includes natural and social scientists, decision makers, and users of climate services that dynamically interact to identify common and priority climate change-related vulnerabilities and solutions. CoCliME plans to focus on local issues in six European focus areas (case studies include Atlantic Ireland, Atlantic French, Baltic, Black Sea, Mediterranean and North Sea/Norwegian Sea). Current activities include historic data analysis to identify any dependencies between HAB events and known environmental factors related to climate change, which will help to estimate the likelihood of future HAB events when coupled with numerical model climate projection outputs. Climate service prototypes are being co-developed with stakeholders in industry, public health officials, and policy makers in each case study region to ensure maximum impact. This close engagement between the project teams allows the users to help guide the scientific work; for example, determining the time scales they are interested in for future climate projections. A transferable framework for climate services development is needed globally to support informed decisions relevant to climate change-related ecological and socio-economic impacts in coastal regions. The CoCliME results will feed into mechanisms such as the UN Sustainable Development Goals, EU Marine Strategy Framework Directive (MSFD), Marine Spatial Planning (MSP), national monitoring and reporting requirements, and climate adaptation planning to ensure the protection and sustainable use of Europe’s marine and coastal ecosystems for future generations.
The aforementioned ASIMUTH project developed the methodology for producing HAB risk alerts to aquaculture and continues to publish them weekly with some partner countries. Continuing the development of this theme, the project PRIMROSE was initiated in late 2016 among ten Atlantic area partners from five EU member states. It aims to develop improvements to these alerts using operational oceanographic forecasts, downscaled regional hydrodynamic models, novel satellite data, HAB / toxin monitoring data, and expert evaluation (Leadbetter et al., 2018). PRIMROSE will upgrade this service to a regional scale and provide mesoscale trans-national HABs and microbial risk information and knowledge exchange with the user community. The project has commenced to develop close involvement/co-development with industry partners throughout the project to ensure maximum impact of the project outcomes. New information coming from the Marine Strategy Framework and Water Framework Directives monitoring programs and from a new generation of sensors aboard the Sentinel suite of satellites, deployed using drone technology and aboard buoys in marine locations will be utilized for the alert system. Other new features will include:
• The existing system will be expanded into new areas and new species (S and NW Spain, Canary Islands, Shetlands)
• Forecasting of shellfish bacterial contamination to account for microbial risk (e.g., E. coli, Norovirus, Vibrio) will be developed
• The potential for mitigation will be explored
• A Climate / Environmental change 10 year + product to evaluate future risk from harmful effects will be developed
• Policy makers, risk regulators, food safety authorities and the industry will be included
Alertox-Net, which also commenced in late 2016 among 14 EU partners, has a principal objective to facilitate market delivery of safer marine food products by putting urgently needed innovative toxicity alert systems in the value chain. In this project, the main toxins targeted are emerging toxins, non-regulated marine toxins that have proliferated in the Atlantic Area as a consequence of climate change and which are harmful toward public health. The system consists of cost-effective, easy-to-understand, detection and alert methods, which will facilitate adoption by industry. Furthermore, Alertox-net will recommend a regulatory framework addressed to authorities regarding emerging toxins, including tetrodotoxins, palytoxins, and cyclic imines. Moreover, the alert system will be developed for toxins that are currently regulated so that industries which are not adapted, e.g., they are not aware of the toxins and methods, can benefit from better protected products for their consumers (Figure 7).
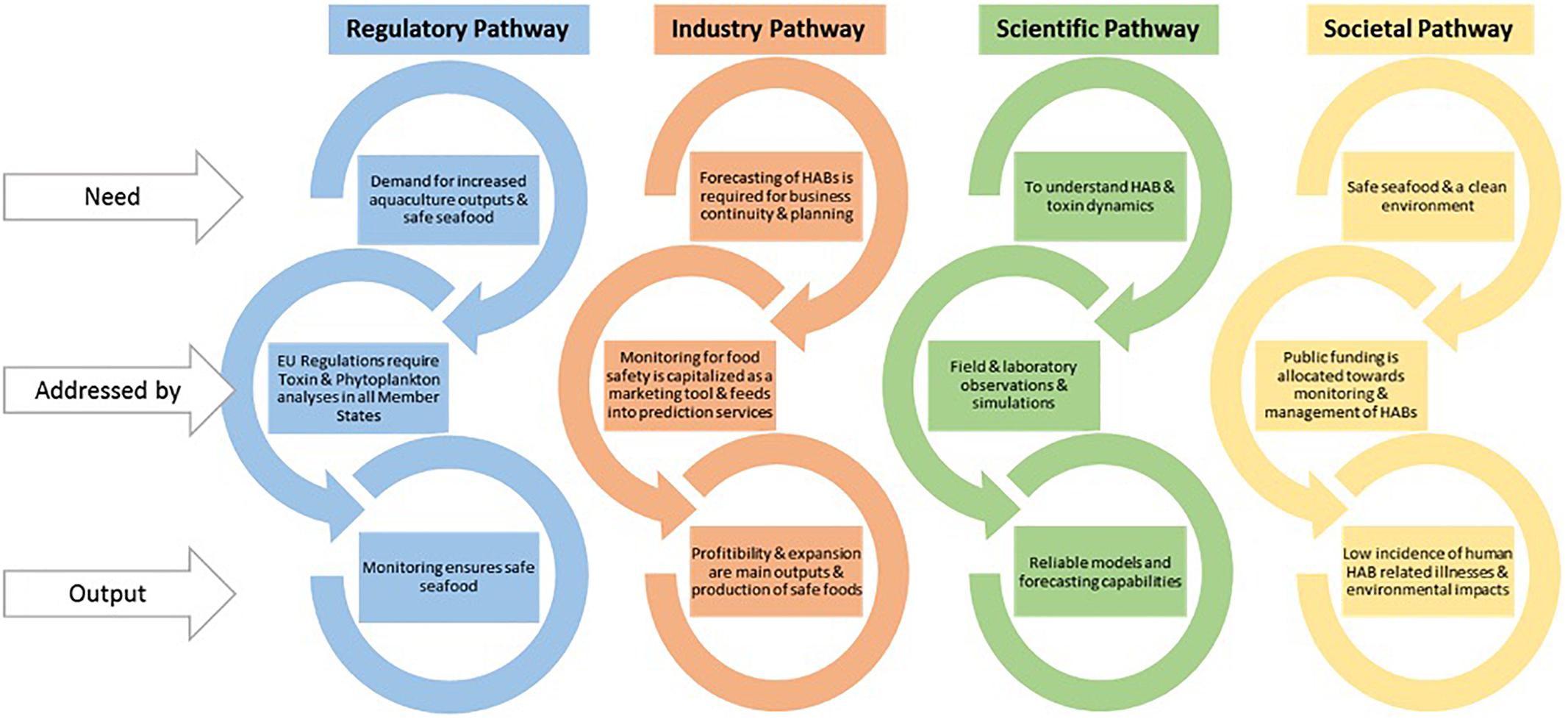
Figure 7. Different sectors have different priorities for HAB and shellfish toxin forecasting in the EU (https://www.coclime.eu/project-overview/co-development-processes).
In the Mediterranean, a recent threat deserves attention: the proliferation of the benthic dinoflagellate Ostreopsis. Since the late 1990s, the tropical genus Ostreopsis has been increasingly detected in many beaches of the Mediterranean Sea, reaching high cell abundances in some areas (Figure 8). Ostreopsis cf. ovata benthic HABs have been linked to massive mortalities of benthic fauna and mild respiratory irritations in people exposed to marine aerosols (Vila et al., 2016. and references there in). The genus also produces potent toxins (palytoxins and analogues) that were associated with dramatic seafood poisonings in tropical latitudes (Randall, 2005). Despite the toxins having been found in marine fauna in the Mediterranean, seafood-borne poisonings have not yet been reported in this area. So far, Ostreopsis blooms have only caused touristic impacts in the Mediterranean (Funari et al., 2015). However, the toxins have been rarely detected in the air (Ciminiello et al., 2014), while the presence of cell debris in the aerosol were confirmed by combining Scanning Electron Microscope and PCR (Casabianca et al., 2013). The recurrence of these events has stimulated coordinated research in the area, in particular through the RAMOGE Accord30 and national and EU-funded projects. Multidisciplinary approaches involve scientists, public health and environmental authorities, and affected citizens. Common sampling, monitoring (Mangialajo et al., 2017), communication and outreach activities, including citizen science are also underway to design prevention strategies before Ostreopsis blooms constitute a major problem in this highly anthropized area.
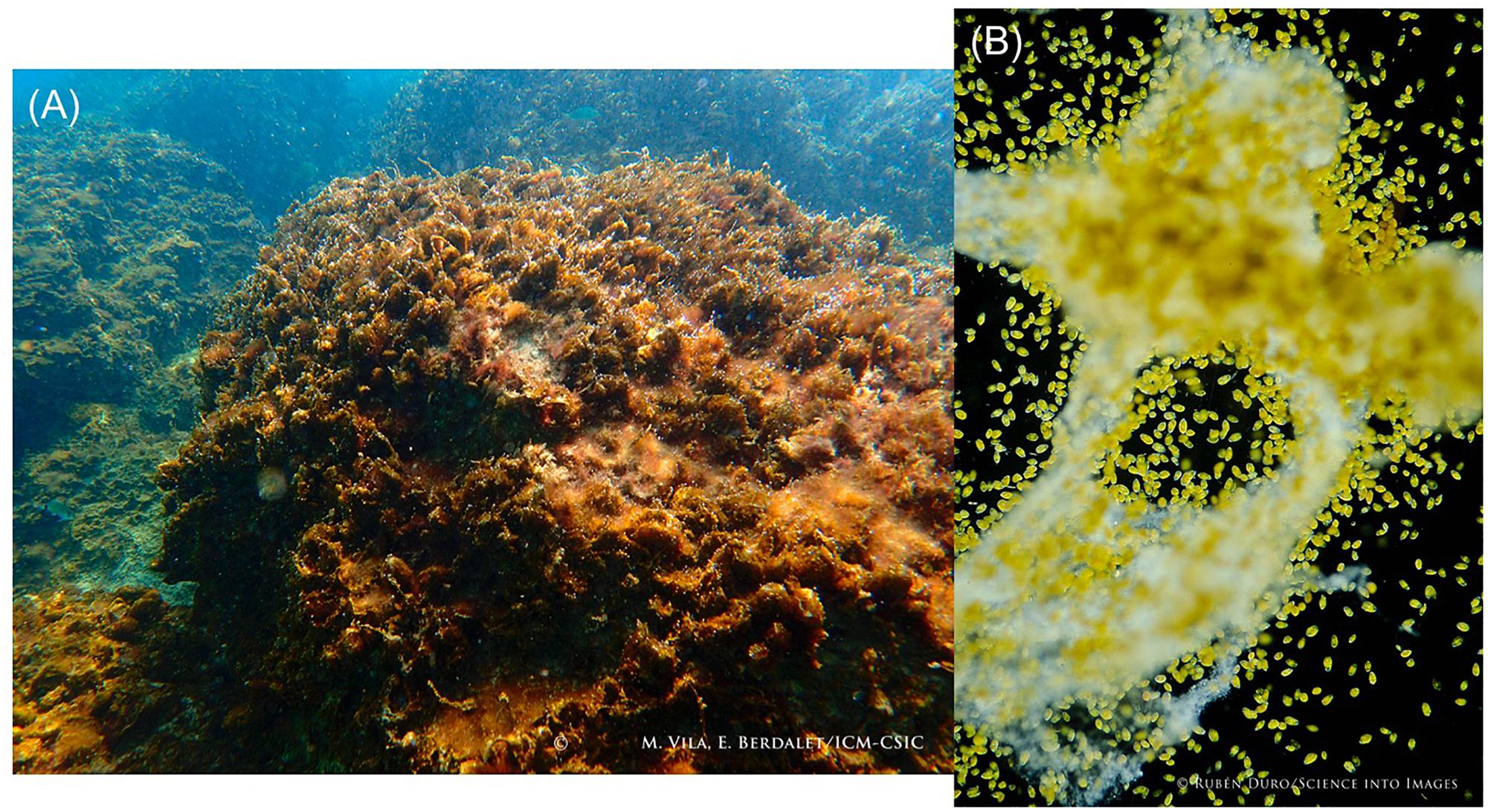
Figure 8. Bloom of the benthic dinoflagellate Ostreopsis cf. ovata in the NW Mediterranean coast. (A) Mucilaginous biofilm covering the macroalgae (photo credit: M. Vila and E. Berdalet). (B) Microscopic image of Ostreopsis cells and the mucus produced to attach to the surfaces (magnification 4x) (photo credit: @RUBEN DURO/SCIENCE INTO IMAGES).
Years of coordinated projects and improvements to regulatory monitoring programs in Europe have proven effective for protecting human health from seafood poisoning. It is now time for progress in ecological modeling and climate projections to ascertain future trends of HABs in the European Seas due to the combined anthropogenic pressures and climate heating and to design mitigation strategies. Today, emerging blooms that cause mild respiratory irritation and biotoxins that cause potential seafood poisoning (associated with benthic Ostreopsis blooms) constitute a new challenge that requires an adaptive monitoring design to include the benthos. In all cases, communication with the general public, stakeholders, and policymakers is becoming urgent and is the best tool to address future strategies. Multiple ocean observatories should be identified within the very distinct marine ecosystems of the EU that can help support both hindcast and forecast models.
Synthesis of Regional Case Studies
Recent progress in several areas of technology, coupled with advances in our knowledge of biological behavior, such as HAB life history and oceanographic processes, has pointed toward a potential transformation in the way that we currently monitor, manage, and understand HABs. Rapid progress in bio-optics is providing hyperspectral signatures of water bodies associated with various HABs (Babin et al., 2005; Blondeau-Patissier et al., 2014; Muller-Karger et al., 2018a). Developments in imaging flow cytometry and particle size analysis have enabled better automation of bloom identification (e.g., Campbell et al., 2013). Progress in molecular biology has led to the development of next-generation molecular tools (Kudela et al., 2010). New technologies and modeling capabilities combined with more traditional biogeochemical methods will no doubt improve our understanding of HAB and seafood toxicity dynamics. Indeed, many of these technologies and methods for studying algae, primarily plankton, are described in detail by Boss et al., 2019 (in this Special Issue). The focus of the present contribution has been on regional programs where many of these newer technologies, such as the ESP and IFCB, are being evaluated for long-term, stakeholder-relevant HAB monitoring and mitigation, with the caveat that automated instruments may not be appropriate everywhere. Cross-cutting features of the regional legacies, approaches, and programs can be summarized as follows:
(1) Each region is unique, and we cannot develop a one-size-fits-all-approach.
(2) At the same time, successful programs include strong buy-in and coordination with end-users and stakeholders, including citizen scientists.
(3) Technology is automating monitoring in many regional programs, but these measurements and models must be institutionalized to maintain continuity.
(4) Integrated modeling approaches that provide co-benefits for multiple resource monitoring efforts or are integrated into regional and national programs are the most stable in the long-term.
In order to improve existing HAB monitoring and prediction efforts, there is a critical requirement to continue to foster international cooperation and interdisciplinary research, to increase operational capabilities to capture HAB events in real-time, and to increase the spatial and vertical resolution of models. The projects highlighted in this community paper are all good examples of blended technology projects with public and private consortium members. As technologies continue to advance, it is essential that working groups and collaborative projects are established to facilitate effective interdisciplinary communications. Interaction with, and feedback from, stakeholders is essential for a successful HAB surveillance and forecasting system. The feedback loop ensures end-user information products are continually refined and developed as user needs increase and change with time. Finally, over the next 10 years, a continued long-term commitment and support from governing bodies at the local, regional, and international levels will play a significant role in determining if more robust automated HAB forecasting services of value are delivered (Table 1).
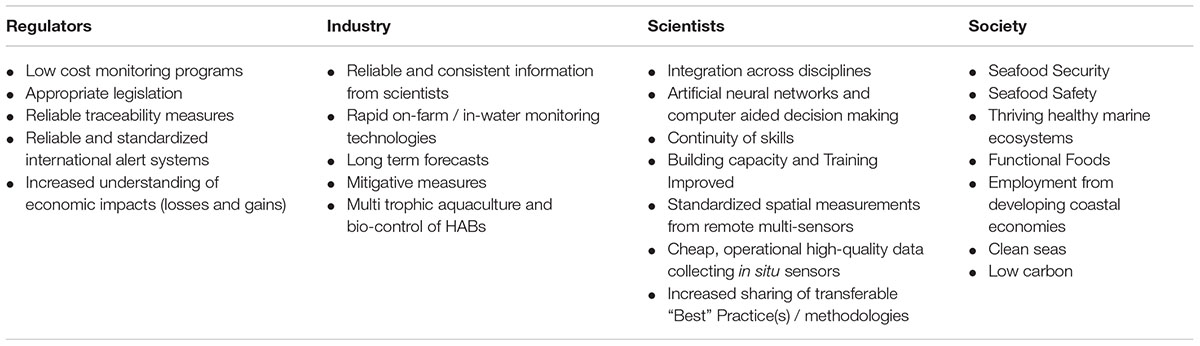
Table 1. Future needs of different sectors in the European Union (also found at www.coclime.eu/node/132).
Scaling Up: Future Directions for Global Observing of HABs
A critical, strategic tool for addressing HABs as a global community is the common language that is forming in the Global Ocean Observing System (GOOS) around essential oceanographic variables (EOVs) and in the Group on Earth Observations (Pereira et al., 2013) around essential biodiversity variables (EBVs) (Navarro et al., 2017; Muller-Karger et al., 2018a,b). While more refinement of terms is needed, attaining a fundamental and universal lexicon of terms is relevant to the distribution, management, and discoverability of plankton datasets and HAB information. From there, a standardized set of indicators will lead to a more cohesive assessment of global trends and impacts from HABs and interacting environmental pressures, such as sea level rise, ocean acidification, and coastal hazards. Looking ahead to the next decade, policy directives will need to identify and describe the appropriate phytoplankton community and HAB-related ecosystem indicators that integrate across SDGs and are relevant to an effective early warning system.
GOOS recently organized a workshop to identify a common set of goals for implementing a global and sustainable, multi-disciplinary plankton observing capability (Miloslavich et al., 2018b). The central themes surrounding the workshop recommendations resonate with the case studies described in this paper: (1) communication with stakeholders, (2) accessibility of affordable, real-time automated imagers for in situ plankton detection and quantification, (3) and availability of standardized data sets that reside in major, global biodiversity and event databases, such as OBIS, the Harmful Algae Event Database (HAEDAT), and the Global Biodiversity Information Facility (GBIF) (Miloslavich et al., 2018a). The latter topic of data standardization, archive, and discoverability is woven into the regional HAB monitoring vignettes presented here, whereby stakeholder engagement and database management form a tight feedback loop. For a global HAB observing system that is dependent on globally defined SDG-level indicators to be fully operative, however, seemingly mundane issues such as data management “best practices” become ever more important. Indeed, ecological forecasting and trend analyses, such as those captured by Intergovernmental Science-Policy Platform on Biodiversity and Ecosystem Services (IPBES) assessments (Ferrier et al., 2016) or used to support a growing Blue Economy, strongly rely on the interoperability of geospatial data and compatibility across user interfaces for biogeographic and biodiversity data repositories.
Recommendations for a Global HAB Observing System
In an effort to encourage the development of an integrative global ocean observing system optimized for HABs and building on the GOOS framework for ocean observing (UNESCO, 2012), we contribute these specific objectives that are inspired by the regional case studies presented in this paper.
Deliver an observing system that is fit for purpose
• Advance and improve cost-effective and sustainable HAB forecast systems that address the HAB-risk warning requirements of key end-users at global and regional levels.
• Fill the essential need for sustained, and preferably automated, near real-time information from nearshore and offshore sites situated in HAB transport pathways to provide improved, advanced HAB warnings.
• Increase communication between regions to enable knowledge-sharing of best practices.
Apply a systems approach for sustained global ocean observing
• Incorporate available Earth Observations into monitoring and predictive efforts, including blended model-satellite products and data-assimilative model systems.
• Merge ecological knowledge and models with existing Earth System Modeling Frameworks to enhance end-to-end capabilities in forecasting and scenario-building.
• Work toward providing seasonal to decadal forecasts to allow governments to plan and adapt to a changing marine environment while ensuring coastal industries are supported and sustained in the years ahead.
Recognize and develop interfaces among all actors in the Framework for their mutual benefit
• Identify societal priorities with respect to the HAB problem, e.g., public health, food security, clean drinking water, aquaculture, sustainable fishing, tourism and recreation.
• Address critical stakeholder needs while striving to attain and sustain climate-quality data sets for scientific understanding of long-term trends in HABs.
• Build interoperable user interfaces such that complementary biological and environmental datasets can be accessed by user groups.
Provide the basis for and promote transformation of observational data organized in EOVs into information (syntheses, analyses, assessments, forecasts, projections, and scenarios) that serves a wide range of science and societal needs and enables good management of the human relationship with the ocean.
• Form programs with robust communication channels for stakeholders and partners.
• Support implementation of the recent calls for action by the UN 2010 SDGs to develop global indicators that remain relevant to early-warning systems at the regional scale.
• Design programs that leverage regional HAB observing systems to evaluate emerging technologies for EOVs and EBVs in order to support interregional technology comparisons and regional networks of observing capabilities.
• Facilitate dialogue on HABs within all relevant global initiatives, e.g., GOOS, GlobalHAB, Future Earth, GEO, and the International Ocean-Color Coordinating Group, to name just a few.
• Challenge policy-makers to incorporate goals for HAB mitigation that cut across SDG themes and promote maximal societal benefit.
Author Contributions
CA contributed to the overall writing and framework of the manuscript, with significant contributions from EOR, CC, EB, RK, and JS. Regional contributions were written by DD and MM (Alaska), SM and JN (Pacific Northwest), CA and RK (California), BK and KH (Gulf of Mexico), JM (Gulf of Maine), SR and KP (Great Lakes), EB and JM (Caribbean Islands), EOR, CC, EB, and JS (European Union), and RK (Asia). All authors contributed to the manuscript revision and have read and approved the submitted abstract.
Funding
This paper contributes to the implementation of the objectives of the SCOR and IOC/UNESCO GlobalHAB Program. The AHAB Network is partially funded through the AOOS with support from NOAA (Grant No. NA16NOS0120027, entitled, “Implementation and Development of Regional Coastal Ocean Observing System: Alaska Ocean Observing System.” Funding for Southeast Alaska Tribal Ocean Research is supported by funding from the EPA General Assistance Program (GA-01J13701-1), the NOAA Saltonstall-Kennedy Grant Program (Grant No. NA17NMF4270238) and ECOHAB grants, and the BIA Tribal Resilience Program (Grant No. 1800-0002). Funding for Pacific Northwest United States HAB research, monitoring and forecasting came from many sources. Multiple projects, including SoundToxins, ORHAB, and the PNW HAB Bulletin, have been supported by the NOAA NOS NCCOS national competitive HAB programs: ECOHAB, and MERHAB. Barbara Hickey, Ryan McCabe, and Vera Trainer are acknowledged for major contributions to those efforts and overall leadership. SM and JN acknowledge United States IOOS Ocean Technology Transition award “Operational Ecological Forecasting of Harmful Algal Blooms in the Pacific Northwest using an Environmental Sample Processor (ESP)” (NA14NOS0120149) to UW and SM for the ESP monitoring, and the NOAA NOS United States IOOS regional association funding for monitoring and forecasting efforts by NANOOS through “Sustaining NANOOS, the Pacific Northwest component of the United States IOOS” (NA16NOS0120019) to JN. SM was supported by NOAA Fisheries’ Northwest Fisheries Science Center. Funding for the California Harmful Algae Risk Mapping (C-HARM) system is provided through a grant from NASA (Grant No. 80NSSC17K0049) and sustained support NOAA National Environmental Satellite, Data, and Information Service (NESDIS) and the NOS NCCOS. Sustained distribution and maintenance of the California HAB Bulletin and support of HABMAP management is provided by NOAA Award “Integrated Ocean Observing System Implementation: Southern California Coastal Ocean Observing System (SCCOOS)” (NA16NOS0120022). Long-term monitoring, including implementation of the IFCB technology, has been supported by the NOAA Ecology and Oceanography of Harmful Algal Blooms (ECOHAB; NA11NOS4780030), Monitoring and Event Response for HABs (MERHAB; NA04NOS4780239), the Marine Sensor Technology Transition Program (NA14NOS0120148), and the CeNCOOS partnership: Ocean Information for Decision Makers (NA16NOS0120021). Funding for the Gulf of Mexico “Every Beach, Every Day” Forecast product and HABscope is provided through a grant from NASA (Grant No. NNH15AB23I), internal support from NOAA, the Florida Fish and Wildlife Conservation Commission, and the NOAA Award for Continued Development of a Gulf of Mexico Coastal Ocean Observing System (Grant No. NA16NOS0120018). The Great Lakes HABs observations and forecasts are partially funded through the Great Lakes Restoration Initiative (Grant No. GLRI DW-013-9249280), with sustained support from NOAA OAR Great Lakes Environmental Research Laboratory and the NOS IOOS (Grant No. NA16NOS0120025), NCCOS, and the Center for Operational Oceanographic Products and Services (CO-OPS). NOAA NOS United States IOOS supports the development of the regional Sargassum forecast system for the CARICOOS region through “CARICOOS: Enhancing Coastal Intelligence in the United States Caribbean” (NA16NOS0120026). European Union – Research activities described in this article were funded through the Interreg Atlantic Area programme projects PRIMROSE (Grant No. EAPA_182/2016) and Alertox-Net (Grant No. EAPA_317/2016), the AtlantOS project under the European Union’s Horizon 2020 Research and Innovation Program (Grant No. 633211), and project CoCliME an ERA4CS Network (ERA-NET) initiated by JPI Climate, and funded by EPA (IE), ANR (FR), BMBF (DE), UEFISCDI (RO), RCN (NO) and FORMAS (SE), with co-funding by the European Union (Grant No. 690462).
Conflict of Interest Statement
The authors declare that the research was conducted in the absence of any commercial or financial relationships that could be construed as a potential conflict of interest.
Supplementary Material
The Supplementary Material for this article can be found online at: https://www.frontiersin.org/articles/10.3389/fmars.2019.00250/full#supplementary-material
Footnotes
- ^www.fao.org
- ^www.globalhab.info
- ^http://www.seator.org/
- ^www.soundtoxins.org
- ^http://www.nanoos.org/products/real-time_habs/home.php
- ^http://www.nanoos.org/products/habs/forecasts/bulletins.php
- ^https://sensors.ioos.us/
- ^https://spraydata.ucsd.edu/
- ^http://www.sccoos.org/data/hfrnet/
- ^http://sccoos.org/california-hab-bulletin/
- ^http://www.habmap.info/
- ^http://myfwc.com/research/redtide/statewide/
- ^https://tidesandcurrents.noaa.gov/hab/gomx.html
- ^https://tpwd.texas.gov/landwater/water/environconcerns/hab/redtide/status.phtml
- ^https://www.alabamapublichealth.gov/news/2018/11/21b.html
- ^https://www.facebook.com/MississippiDMR/posts/red-tide-update-for-the-gulf-coast/874997942608667/
- ^http://ldh.la.gov/index.cfm/page/629/n/210
- ^https://habscope.gcoos.org/forecasts
- ^https://www.glerl.noaa.gov/res/HABs_and_Hypoxia/habTracker.html
- ^https://www.glerl.noaa.gov/res/HABs_and_Hypoxia/airSatelliteMon.html
- ^https://tidesandcurrents.noaa.gov/hab/lakeerie_bulletins/bulletin_current.pdf
- ^https://www.glerl.noaa.gov/metdata/
- ^http://habs.glos.us/map/
- ^https://www.cbd.int
- ^https://optics.marine.usf.edu/projects/SaWS.html
- ^https://www.caricoos.org/oceans/observation/modis_aqua/ECARIBE/afai
- ^http://app.estado.gobierno.pr/ReglamentosOnLine/Reglamentos/6768.pdf
- ^www.coclime.eu
- ^https://www.alertox-net.eu/
- ^http://www.ramoge.org/fr/default.aspx
References
Ahn, Y. H., and Shanmugam, P. (2006). Detecting the red tide algal blooms from satellite ocean color observations in optically complex Northeast-Asia coastal waters. Remote Sens. Environ. 103, 419–437. doi: 10.1016/j.rse.2006.04.007
Aleynik, D., Dale, A. C., Porter, M., and Davidson, K. (2016). A high resolution hydrodynamics model system suitable for novel harmful algal bloom modelling in areas of complex coastline and topography. Harmful Algae 53, 102–117. doi: 10.1016/j.hal.2015.11.012
Anderson, C. R., Kudela, R. M., Kahru, M., Chao, Y., Rosenfeld, L. K., Bahr, F. L., et al. (2016). Initial skill assessment of the California harmful algae risk mapping (C-HARM) system. Harmful Algae 59, 1–18. doi: 10.1016/j.hal.2016.08.006
Anderson, C. R., Moore, S. K., Tomlinson, M. C., Silke, J., and Cusack, C. K. (2015). “Living with harmful algal blooms in a changing world: strategies for modeling and mitigating their effects in coastal marine ecosystems,” in Coastal and Marine Hazards, Risks, and Disasters, eds J. T. Ellis and D. J. Sherman (Amsterdam: Elsevier), 495–561. doi: 10.1016/b978-0-12-396483-0.00017-0
Anderson, C. R., Sellner, K. G., and Anderson, D. M. (2017). “Bloom prevention and control,” in Harmful Algal Blooms (HABs) and Desalination: A Guide to Impacts, Monitoring, and Management, eds D. M. Anderson, F. E. Boerlage, and M. B. Dixon (Paris: UNESCO), 205–222.
Anderson, D. M. (2008). “Harmful algal blooms and ocean observing systems: needs, present status and future potential,” in Fisheries for Global Welfare and Environment, eds K. Tsukamoto, T. Kawamura, T. Takeuchi, T. D. Beard Jr., and M. J. Kaiser (Tokyo: Terrapub), 317–334.
Anderson, D. M. (2009). Approaches to monitoring, control and management of harmful algal blooms (HABs). Ocean Coast. Manag. 52, 342–347. doi: 10.1016/j.ocecoaman.2009.04.006
Anderson, D. M., Andersen, P., Bricelj, V. M., Cullen, J. J., and Rensel, J. E. (2001). Monitoring and Management Strategies for Harmful Algal Blooms in Coastal Waters. Paris: Intergovernmental Oceanographic Commission.
Anderson, D. M., Cembella, A. D., and Hallegraeff, G. M. (2012). Progress in understanding harmful algal blooms: paradigm shifts and new technologies for research, monitoring, and management. Annu. Rev. Mar. Sci. 4, 143–176. doi: 10.1146/annurev-marine-120308-081121
Anderson, D. M., Glibert, P. M., and Burkholder, J. M. (2002). Harmful algal blooms and eutrophication: nutrient sources, composition, and consequences. Estuaries 25, 704–726. doi: 10.1007/bf02804901
Anderson, D. M., Keafer, B. A., Kleindinst, J. L., McGillicuddy, D. J. Jr., Martin, J. L., Norton, K., et al. (2014). Alexandrium fundyense cysts in the Gulf of Maine: long-term time series of abundance and distribution, and linkages to past and future blooms. Deep-Sea Res. II 103, 6–26. doi: 10.1016/j.dsr2.2013.10.002
Anderson, D. M., Keafer, B. A., McGillicuddy, D. J. Jr., Mickelson, M. J., Keay, K. E., Libby, P. S., et al. (2005). Initial observations of the 2005 Alexandrium fundyense bloom in southern New England: general patterns and mechanisms. Deep Sea Res. Part II Top. Stud. Oceanogr. 52, 2856–2876. doi: 10.1016/j.dsr2.2005.09.004
Babin, M., Cullen, J. C., Roesler, C. S., Donaghay, P. L., Doucette, G. J., Kahru, M., et al. (2005). New approaches and technologies for observing harmful algal blooms. Oceanography 18, 210–227. doi: 10.5670/oceanog.2005.55
Babin, M., Roesler, C. S., and Cullen, J. J. (eds). (2008). Real-Time Coastal Observing Systems for Marine Ecosystem Dynamics and Harmful Algal Blooms: Theory, Instrumentation and Modelling. Oceanographic Methodology Series, Paris: UNESCO, 830.
Beckers, J. M., and Rixen, M. (2003). EOF calculations and data filling from incomplete oceanographic datasets. J. Atmos. Ocean. Technol. 20, 1839–1856. doi: 10.1175/1520-0426(2003)020
Blondeau-Patissier, D., Gower, J. F., Dekker, A. G., Phinn, S. R., and Brando, V. E. (2014). A review of ocean color remote sensing methods and statistical techniques for the detection, mapping and analysis of phytoplankton blooms in coastal and open oceans. Prog. Oceanogr. 123, 123–144. doi: 10.1016/j.pocean.2013.12.008
Boss, E., Lombard, F., Stemmann, L., Miloslavich, P., Schulz, J., Romagnan, J.-B., et al. (2019). Globally consistent quantitative observations of planktonic ecosystems. Front. Mar. Sci. 6:196. doi: 10.3389/fmars.2019.00196
Bowers, H. A., Marin, R. III, Birch, J. M., Scholin, C. A., and Doucette, G. J. (2016). Recovery and identification of Pseudo-nitzschia (Bacillariophyceae) frustules from natural samples acquired using the environmental sample processor. J. Phycol. 52, 135–140. doi: 10.1111/jpy.12369
Brosnahan, M. L., Velo-Suárez, L., Ralston, D. K., Fox, S. E., Sehein, T. R., Shalapyonok, A., et al. (2015). Rapid growth and concerted sexual transitions by a bloom of the harmful dinoflagellate Alexandrium fundyense (Dinophyceae). Limnol. Oceanogr. 60, 2059–2078. doi: 10.1002/lno.10155
Brown, C. W., Green, D., Hickey, B. M., Jacobs, J. M., Lanerolle, L. W. J., Moore, S., et al. (2012). “Towards operational forecasts of algal blooms and pathogens,” in Environmental Tracking for Public Health Surveillance, eds S. A. Morain and A. M. Budge (Boca Raton, FL: CRC Press), 345–368. doi: 10.1201/b12680-13
Burkholder, J. M., Hallegraeff, G. M., Melia, G., Cohen, A., Bowers, H. A., Oldach, D. W., et al. (2007). Phytoplankton and bacterial assemblages in ballast water of US military ships as a function of port of origin, voyage time, and ocean exchange practices. Harmful Algae 6, 486–518. doi: 10.1016/j.hal.2006.11.006
Campbell, L., Henrichs, D. W., Olson, R. J., and Sosik, H. M. (2013). Continuous automated imaging-in-flow cytometry for detection and early warning of Karenia brevis blooms in the Gulf of Mexico. Environ. Sci. Pollut. Res. 20, 6896–6902. doi: 10.1007/s11356-012-1437-4
Casabianca, S., Casabianca, A., Riobó, P., Franco, J. M., Vila, M., and Penna, A. (2013). Quantification of the toxic dinoflagellate Ostreopsis spp. by qPCR assay in marine aerosol. Environ. Sci. Technol. 47, 3788–3795. doi: 10.1021/es305018s
Casper, E. T., Patterson, S. S., Bhanushali, P., Farmer, A., Smith, M., Fries, D. P., et al. (2007). A handheld NASBA analyzer for the field detection and quantification of Karenia brevis. Harmful Algae 6, 112–118. doi: 10.1016/j.hal.2006.11.001
Cheng, Y. S., Zhou, Y., Irvin, C. M., Pierce, R. H., Naar, J., Backer, L. C., et al. (2005). Characterization of marine aerosol for assessment of human exposure to brevetoxins. Environ. Health Perspect. 113, 638–643. doi: 10.1289/ehp.7496
Ciminiello, P., Dell’Aversano, C., Dello Lacovo, E., Fattorusso, E., Forino, M., and Tartaglione, L. (2014). First finding of Ostreopsis cf. ovata toxins in marine aerosols. Environ. Sci. Technol. 48, 3532–3540. doi: 10.1021/es405617d
Cloern, J. E. (2018). Why large cells dominate estuarine phytoplankton. Limnol. Oceanogr. 63, S392–S409.
Cusack, C., Dabrowski, T., Lyons, K., Berry, A., Westbrook, G., Salas, R., et al. (2016). Harmful algal bloom forecast system for SW Ireland. Part II: are operational oceanographic models useful in a HAB warning system? Harmful Algae 53, 86–101. doi: 10.1016/j.hal.2015.11.013
Dabor, R., Ruddy, V., Rémi, N., Rishika, B., Papa, G., and Jonathan, F. (2018). Sargassum seaweed on Caribbean islands: an international public health concern. Lancet 392:2691. doi: 10.1016/s0140-6736(18)32777-6
Darius, H. T., Roué, M., Sibat, M., Viallon, J., Gatti, C. M., Vandersea, M. W., et al. (2017). Tectus niloticus (Tegulidae, Gastropod) as a novel vector of ciguatera poisoning: detection of Pacific ciguatoxins in toxic samples from Nuku Hiva Island (French Polynesia). Toxins 10:E2. doi: 10.3390/toxins10010002
Davidson, K., Anderson, D. M., Mateus, M., Reguera, B., Silke, B., Sourissueau, S., et al. (2016). Forecasting the risk of harmful algal blooms: preface to the asimuth special issue. Harmful Algae 53, 1–7. doi: 10.1016/j.hal.2015.11.005
Doucette, G. J., Mikulski, C. M., Jones, K. L., King, K. L., Greenfield, D. I., Marin, R. III, et al. (2009). Remote, sub-surface detection of the algal toxin domoic acid onboard the environmental sample processor: assay development and field trials. Harmful Algae 8, 880–888. doi: 10.1016/j.hal.2009.04.006
Ehlers, P. (1994). The baltic sea area: convention on the protection of the marine environment of the baltic sea area (Helsinki Convention) of 1974 and the revised convention of 1992. Mar. Poll. Bull. 29, 617–621. doi: 10.1016/0025-326x(94)90697-1
Ferrier, S., Ninan, K. N., Leadley, P., Alkemade, R., Acosta, L. A., Akçakaya, H. R., et al. (2016). The Methodological Assessment Report on Scenarios and Models of Biodiversity and Ecosystem Services. Bonn: Secretariat of the Intergovernmental Platform for Biodiversity and Ecosystem Services.
Fleming, L. E., Kirkpatrick, B., Backer, L. C., Bean, J. A., Wanner, A., Reich, A., et al. (2007). Aerosolized red-tide toxins (brevetoxins) and asthma. Chest 131, 187–194. doi: 10.1378/chest.06-1830
Franks, J. S., Johnson, D. R., and Ko, D. S. (2016). Pelagic Sargassum in the Tropical North Atlantic. Gulf Caribb. Res. 27, SC6–SC11.
Friedman, M. A., Frenandez, M., Backer, L. C., Dickey, R. W., Bernstein, J., Schrank, K., et al. (2017). An updated review of ciguatera fish poisoning: clinical, epidemiological, environmental, and public health management. Mar. Drugs 15:72. doi: 10.3390/md15030072
Funari, E., Testai, E., and Maganelli, M. (2015). Ostreopsis cf. ovata blooms in coastal water: italian guidelines to assess and manage the risk associated to bathing waters and recreational activities. Harmful Algae 50, 45–56. doi: 10.1016/j.hal.2015.10.008
Furuya, K., Mitsunori, I., Po Teen, L., Songhui, L., Chui-Pin, L., Azanza, R. V., et al. (2018). “Overview of harmful algal blooms in Asia,” in Global Ecology and Oceanography of Harmful Algal Blooms, eds P. Glibert, E. Berdalet, M. Burford, G. Pitcher, and M. Zhou (Cham: Springer), 289–308. doi: 10.1007/978-3-319-70069-4_14
G7 (2018). Charlevoix Blueprint for Healthy Oceans, Seas and ResilientCommunities in 2018. The Charlevoix G7 Summit Communique 7pp. Availableat: https://g7.gc.ca/wp-content/uploads/2018/06/HealthyOceansSeasResilientCoastalCommunities.pdf (accessed May 11, 2019).
Gatti, C. M. I., Lonati, D., Darius, H. T., Zancan, A., Roueì, M., Schicchi, A., et al. (2018). Tectus niloticus (Tegulidae, Gastropod) as a novel vector of ciguatera poisoning: clinical characterization and follow-up of a mass poisoning event in Nuku Hiva Island (French Polynesia). Toxins 10:102. doi: 10.3390/toxins10030102
GEOHAB (2001). Global Ecology and Oceanography of Harmful Algal Blooms,” in Science Plan, eds P. Glibert and G. Pitcher (Paris: SCOR), 87.
GEOHAB (2005). “Global Ecology and Oceanography of Harmful Algal Blooms,” in GEOHAB Core Research Project: HABs in Upwelling Systems, eds G. Pitcher, T. Moita, V. Trainer, R. Kudela, F. Figuieras, and T. Probyn (Paris: IOC), 88.
GEOHAB (2006). Global Ecology and Oceanography of Harmful Algal Blooms: Eutrophication and HABs. Paris: IOC, 74.
GEOHAB (2008). “Global Ecology and Oceanography of Harmful Algal Blooms,” in GEOHAB Core Research Project: HABs in Stratified Systems, eds P. Gentien, B. Reguera, and H. Yamazaki (Paris: IOC), 59.
GEOHAB (2010a). “Global Ecology and Oceanography of Harmful Algal Blooms,” in GEOHAB Core Research Project: HABs in Fjords and Coastal Embayments, eds A. Cembella, L. Guzmán, S. Roy, and J. Diogène (Paris: IOC), 57.
GEOHAB (2010b). “Global Ecology and Oceanography of Harmful Algal Blooms,” in Harmful Algal Blooms in Asia, eds K. Furuya, P. M. Glibert, and M. Zhou (Paris: IOC), 68.
Glibert, P. M. (2014). Harmful algal blooms in asia: an insidious and escalating water pollution phenomenon with effects on ecological and human health. ASIANetwork exchange. J. Asian Stud. Lib. Arts 21, 52–68. doi: 10.16995/ane.46
Glibert, P. M., Berdalet, E., Burfort, M., Pitcher, G., and Zhou, M. (eds) (2018). Global Ecology and Oceanography of Harmful Algal Blooms. Cham: Springer International Publishing AG.
Glibert, P. M., and Burford, M. A. (2017). Globally changing nutrient loads and harmful algal blooms: recent advances, new paradigms, and continuing challenges. Oceanography 30, 58–69. doi: 10.5670/oceanog.2017.110
Gower, J., Young, E., and King, S. (2013). Satellite images suggest a new Sargassum source region in 2011. Remote Sens. Lett. 4, 764–773. doi: 10.1080/2150704X.2013.796433
Hardison, D. R., Holland, W. C., McCall, J. R., Bourdelais, A. J., Baden, D. G., Darius, H. T., et al. (2016). Fluorescent receptor binding assay for detecting ciguatoxins in fish. PLoS One 11:e0153348. doi: 10.1371/journal.pone.0153348
He, R., McGillicuddy, D. J., Keafer, B. A., and Anderson, D. M. (2008). Historic 2005 toxic bloom of Alexandrium fundyense in the western Gulf of Maine: 2. Coupled biophysical numerical modeling. J. Geophys. Res. Oceans 113:C07040. doi: 10.1029/2007JC004602
Heil, C. A., and Steidinger, K. A. (2009). Monitoring, management, and mitigation of Karenia blooms in the eastern Gulf of Mexico. Harmful Algae 8, 611–617. doi: 10.1016/j.hal.2008.11.006
Hickey, B. M., Trainer, V. L., Kosro, P. M., Adams, N. G., Connolly, T. P., Kachel, N. B., et al. (2013). A springtime source of toxic Pseudo-nitzschia cells on razor clam beaches in the Pacific Northwest. Harmful Algae 25, 1–14. doi: 10.1016/j.hal.2013.01.006
Horner, R. A., Garrison, D. L., and Plumley, F. G. (1997). Harmful algal blooms and red tide problems on the US west coast. Limnol. Oceanogr. 42, 1076–1088. doi: 10.4319/lo.1997.42.5_part_2.1076
Hu, C., Murch, B., Barnes, B. B., Wang, M., Marechal, J., Franks, J., et al. (2016). Sargassum watch warns of incoming seaweed. EOS Trans. Am. Am. Geophys. Union 97, 10–15. doi: 10.1029/2016EO058355
Imai, I., Fujimaru, D., Nishigaki, T., Kurosaki, M., and Sugita, H. (2006). Algicidal bacteria isolated from the surface of seaweeds from the coast of Osaka Bay in the Seto Inland Sea, Japan. Afr. J. Mar. Sci. 28, 319–323. doi: 10.2989/18142320609504170
International Maritime Organization [IMO] (2003). International Convention for the Control and Management of Ships’ Ballast Water and Sediments. International Conference on Ballast Water Management for Ships. Available at: http://globallast.imo.org/index.asp?page=mepc.htm (accessed May 11, 2019).
Jaffe, J. S., Franks, P. J., Roberts, P. L., Mirza, D., Schurgers, C., Kastner, R., et al. (2017). A swarm of autonomous miniature underwater robot drifters for exploring submesoscale ocean dynamics. Nat. Commun. 8:14189. doi: 10.1038/ncomms14189
Jeong, H. J., Kim, J. S., Yoo, Y. D., Kim, S. T., Kim, T. H., Park, M. G., et al. (2003). Feeding by the heterotrophic dinoflagellate Oxyrrhis marina on the red-tide raphidophyte Heterosigma akashiwo: a potential biological method to control red tides using mass-cultured grazers. J. Eukaryot. Microbiol. 50, 274–282. doi: 10.1111/j.1550-7408.2003.tb00134.x
Jeong, H. J., Lim, A. S., Franks, P. J. S., Lee, K. H., Kim, J. H., Kang, N. S., et al. (2015). A hierarchy of conceptual models of red-tide generation: nutrition, behavior, and biological interactions. Harmful Algae 47, 97–115. doi: 10.1016/j.hal.2015.06.004
Jin, D., Thunberg, E., and Hoagland, P. (2008). Economic impact of the 2005 red tide event on commercial shellfish fisheries in New England. Ocean Coast. Manag. 51, 420–429. doi: 10.1016/j.ocecoaman.2008.01.004
Jochens, A. E., Malone, T. C., Stumpf, R. P., Hickey, B. M., Carter, M., Morrison, R., et al. (2010). Integrated ocean observing system in support of forecasting harmful algal blooms. Mar. Technol. Soc. J. 44, 99–121. doi: 10.4031/mtsj.44.6.16
Kang, D., Kim, H., Jung, S., Kim, M., and Kim, B. K. (2016). Development and field application of early warning system for harmful algal blooms (red-tide) early warning using ultrasound wave. Korea J. Acoust. Soc. Am. 140, 3242–3242. doi: 10.1121/1.4970253
Kao, C. Y. (1993). “Paralytic shellfish poisoning,” in Algal Toxins in Seafood and Drinking Water, ed. I. R. Falconer (London: Academic Press Ltd), 75–86.
Kim, T. W., Kim, J. H., Song, M. S., and Yun, H. S. (2017). Convergence technique study on red tide prediction in the littoral sea. J. Coast. Res. 79, 254–258. doi: 10.2112/si79-052.1
Kirkpatrick, B., Currier, R., Nierenberg, K., Reich, A., Backer, L. C., Stumpf, R., et al. (2008). Florida red tide and human health: a pilot beach conditions reporting system to minimize human exposure. Sci. Total Environ. 402, 1–8. doi: 10.1016/j.scitotenv.2008.03.032
Kirkpatrick, B., Fleming, L. E., Bean, J. A., Nierenberg, K., Backer, L. C., Cheng, Y. S., et al. (2011). Aerosolized red tide toxins (brevetoxins) and asthma: continued health effects after 1 h beach exposure. Harmful Algae 10, 138–143. doi: 10.1016/j.hal.2010.08.005
Kudela, R. M., Berdalet, E., Enevoldsen, H., Pitcher, G., Raine, R., and Urban, E. (2017). GEOHAB–the global ecology and oceanography of harmful algal blooms program: motivation, goals, and legacy. Oceanography 30, 12–21. doi: 10.5670/oceanog.2017.106
Kudela, R. M., Bickel, A., Carter, M. L., Howard, M. D., and Rosenfeld, L. (2015). “The monitoring of harmful algal blooms through ocean observing: the development of the California Harmful Algal Bloom Monitoring and Alert Program,” in Coastal Ocean Observing Systems, eds Y. Liu, H. Kerkering, and R. H. Weisberg (Amsterdam: Elsevier), 58–75. doi: 10.1016/b978-0-12-802022-7.00005-5
Kudela, R. M., Howard, M. D. A., Jenkins, B. D., Miller, P. E., and Smith, G. J. (2010). Using the molecular toolbox to compare harmful algal blooms in upwelling systems. Prog. Oceanogr. 85, 108–121. doi: 10.1016/j.pocean.2010.02.007
Langin, K. (2018). Mysterious masses of seaweed assault Caribbean islands. Science 1157–1158. doi: 10.1126/science.aau4441
Laza-Martínez, A., David, H., Riobó, P., Miguel, I., and Orive, E. (2016). Characterization of a strain of Fukuyoa paulensis (Dinophyceae) from the Western Mediterranean Sea. J. Eukaryot. Microbiol. 63, 481–497. doi: 10.1111/jeu.12292
Leadbetter, A., Silke, J., and Cusack, C. (2018). Creating a Weekly Harmful Algal Bloom Bulletin. Galway: A Best Practice Description Document Marine Institute, 59.
Lee, C. K., Park, T. G., Park, Y. T., and Lim, W. A. (2013). Monitoring and trends in harmful algal blooms and red tides in Korean coastal waters, with emphasis on Cochlodinium polykrikoides. Harmful Algae 30,S3–S14.
Lee, H. G., Kim, H. M., Min, J., Kim, K., Park, M. G., Jeong, H. J., et al. (2017). An advanced tool, droplet digital PCR (ddPCR), for absolute quantification of the red-tide dinoflagellate, Cochlodinium polykrikoides Margalef (Dinophyceae). Algae 32, 189–197. doi: 10.4490/algae.2017.32.9.10
Lefebvre, K. A., Powell, C. L., Busman, M., Doucette, G. J., Moeller, P. D. R., Silver, J. B., et al. (1999). Detection of domoic acid in northern anchovies and California sea lions associated with an unusual mortality event. Nat. Toxins 7, 85–92. doi: 10.1002/(sici)1522-7189(199905/06)7
Lefebvre, K. A., Quakenbush, L., Frame, E., Huntington, K. B., Sheffield, G., Stimmelmayr, R., et al. (2016). Prevalence of algal toxins in Alaskan marine mammals foraging in a changing arctic and subarctic environment. Harmful Algae 55, 13–24. doi: 10.1016/j.hal.2016.01.007
Leong, S., Yew, C., Peng, L. L., Moon, C. S., Kit, J. K. W., and Ming, S. T. L. (2015). Three new records of dinoflagellates in Singapore’s coastal waters, with observations on environmental conditions associated with microalgal growth in the Johor Straits. Raffles Bull. Zool. (Suppl. 31), 24–36.
Lewitus, A. J., Horner, R. A., Caron, D. A., Garcia-Mendoza, E., Hickey, B. M., Hunter, M., et al. (2012). Harmful algal blooms along the North American west coast region: history, trends, causes, and impacts. Harmful Algae 19, 133–159. doi: 10.1016/j.hal.2012.06.009
Li, L., and Pan, G. A. (2013). universal method for flocculating harmful algal blooms in marine and fresh waters using modified sand. Environ. Sci. Tech. 47, 4555–4562. doi: 10.1021/es305234d
Li, Y., He, R., McGillicuddy, D. J., Anderson, D. M., and Keafer, B. A. (2009). Investigation of the 2006 Alexandrium fundyense bloom in the Gulf of Maine: in situ observations and numerical modeling. Cont. Shelf Res. 29, 2069–2082. doi: 10.1016/j.csr.2009.07.012
Lim, H. C., Leaw, C. P., Tan, T. H., Kon, N. F., Yek, L. H., and Hii, K. S. (2014). A bloom of Karlodinium australe (Gymnodiniales, Dinophyceae) associated with mass mortality of cage-cultured fishes in West Johor Strait, Malaysia. Harmful Algae 40, 51–62. doi: 10.1016/j.hal.2014.10.005
Liu, D., and Zhou, M. (2018). “Green tides of the Yellow Sea: massive free-floating blooms of Ulva prolifera,” in Global Ecology and Oceanography of Harmful Algal Blooms, eds P. Glibert, E. Berdalet, M. Burford, G. Pitcher, and M. Zhou (Cham: Springer), 317–326. doi: 10.1007/978-3-319-70069-4_16
Loeffler, C. R., Richlen, M. L., Brandt, M. E., and Smith, T. B. (2015). Effects of grazing, nutrients, and depth on the ciguatera-causing dinoflagellate Gambierdiscus in the U.S. Virgin Islands. Mar. Ecol. Prog. Ser. 531, 91–104. doi: 10.3354/meps11310
Loeffler, C. R., Robertson, A., Flores Quintana, H. A., Silander, M. C., Smith, T. B., and Olsen, D. (2018). Ciguatoxin prevalence in 4 commercial fish species along an oceanic exposure gradient in the U.S. Virgin Islands. Environ. Toxicol. Chem. 37, 1852–1863. doi: 10.1002/etc.4137
Lunven, M., Guillaud, J. F., Youenou, A., Crassous, M. P., Berric, R., Le Gall, E., et al. (2005). Nutrient and phytoplankton distribution in the Loire River plume (Bay of Biscay, France) resolved by a new Fine Scale Sampler. Estuar Coast. Shelf Sci. 17, 94–108. doi: 10.1016/j.ecss.2005.06.001
Lunven, M., Landeira, J. M., Lehaître, M., Siano, R., Podeur, C., Danielou, M. M., et al. (2012). In situ video and fluorescence analysis (VFA) of marine particles: applications to phytoplankton ecological studies. Limnol. Oceanogr. Meth. 10, 807–823. doi: 10.4319/lom.2012.10.807
MacFayden, A., and Hickey, B. M. (2010). Generation and evolution of a topographically linked, mesoscale eddy under steady and variable wind-forcing. Cont. Shelf Res. 30, 1387–1402. doi: 10.1016/j.csr.2010.04.001
Maguire, J. A., Cusack, C., Ruiz-Villarreal, M., Silke, J., McElligott, D., and Davidson, K. (2016). Applied simulations and integrated modelling for the understanding of toxic and harmful algal blooms (ASIMUTH): integrated HAB forecast systems for Europe’s Atlantic Arc. Harmful Algae 53, 160–166. doi: 10.1016/j.hal.2015.11.006
Mangialajo, L., Fricke, A., Perez-Gutierrez, G., Catania, D., Jauzein, C., and Lemée, R. (2017). Benthic dinoflagellate integrator (BEDI): a new method for the quantification of benthic harmful algal blooms. Harmful Algae 64, 1–10. doi: 10.1016/j.hal.2017.03.002
McCabe, R. M., Hickey, B. M., Kudela, R. M., Lefebvre, K. A., Adams, N. G., Bill, B. D., et al. (2016). An unprecedented coastwide toxic algal bloom linked to anomalous ocean conditions. Geophys. Res. Lett. 43, 10366–10376.
McGillicuddy, D. J. Jr., Anderson, D. M., Lynch, D. R., and Townsend, D. W. (2005). Mechanisms regulating large-scale seasonal fluctuations in Alexandrium fundyense populations in the Gulf of Maine: results from a physical–biological model. Deep Sea Res. Part II Top. Stud. Oceanogr. 52, 2698–2714. doi: 10.1016/j.dsr2.2005.06.021
McGillicuddy, D. J. Jr., Townsend, D. W., He, R., Keafer, B. A., Kleindinst, J. L., Li, Y., et al. (2011). Suppression of the 2010 Alexandrium fundyense bloom by changes in physical, biological, and chemical properties of the Gulf of Maine. Limnol. Oceanogr. 56, 2411–2426. doi: 10.4319/lo.2011.56.6.2411
Meyer, N., Bigalke, A., Kaulfuß, A., and Pohnert, G. (2017). Strategies and ecological roles of algicidal bacteria. FEMS Microbiol. Rev. 41, 880–899. doi: 10.1093/femsre/fux029
Miloslavich, P., Bax, N. J., Simmons, S. E., Klein, E., Appeltans, W., Aburto-Oropeza, O., et al. (2018a). Essential ocean variables for global sustained observations of biodiversity and ecosystem changes. Glob. Change Biol. 24, 2416–2433. doi: 10.1111/gcb.14108
Miloslavich, P., Pearlman, F., Pearlman, J., Muller-Karger, F., Appeltans, W., Batten, S., et al. (2018b). “Implementation of global, sustained, and multidisciplinary observations of plankton communities,” in Plankton-mob Workshop Proceedings, Santa Cruz.
Moore, S. K., Mantua, N. J., Hickey, B. M., and Trainer, V. L. (2009). Recent trends in paralytic shellfish toxins in Puget Sound, relationships to climate, and capacity for prediction of toxic events. Harmful Algae 8, 463–477. doi: 10.1016/j.hal.2008.10.003
Morin, E., Gatti, C., Bambridge, T., and Chinain, M. (2016). Ciguatera fish poisoning: incidence, health costs and risk perception on Moorea Island (Society archipelago, French Polynesia). Harmful Algae 60, 1–10. doi: 10.1016/j.hal.2016.10.003
Muller-Karger, F. E., Hestir, E., Ade, C., Turpie, K., Roberts, D. A., Siegel, D., et al. (2018a). Satellite sensor requirements for monitoring essential biodiversity variables of coastal ecosystems. Ecol. Appl. 28, 749–760. doi: 10.1002/eap.1682
Muller-Karger, F. E., Miloslavich, P., Bax, N. J., Simmons, S., Costello, M. J., Sousa Pinto, I., et al. (2018b). Advancing marine biological observations and data requirements of the complementary Essential Ocean Variables (EOVs) and Essential Biodiversity Variables (EBVs) frameworks. Front. Mar. Sci. 5:211. doi: 10.3389/fmars.2018.00211
Nakada, M., Hatayama, Y., Ishikawa, A., Ajisaka, T., Sawayama, S., and Imai, I. (2018). Seasonal distribution of Gambierdiscus spp. in Wakasa Bay, the Sea of Japan, and antagonistic relationships with epiphytic pennate diatoms. Harmful Algae 76, 58–65. doi: 10.1016/j.hal.2018.05.002nta
National Science, and Technology Council [US] (2017). Harmful Algal Blooms and Hypoxia in the Great Lakes Research Plan and Action Strategy: An Interagency Report. Available at: https://purl.fdlp.gov/GPO/gpo89660 (accessed May 11, 2019).
Navarro, L. M., Fernández, N., Guerra, C., Guralnick, R., Kissling, W. D., Londoño, M. C., et al. (2017). Monitoring biodiversity change through effective global coordination. Curr. Opin. Environ. Sustain. 29, 158–169. doi: 10.1016/j.cosust.2018.02.005
Nishimura, T., Tawong, W., Sakanari, H., Ikegami, T., Uehara, K., Inokuchi, D., et al. (2018). Abundance and seasonal population dynamics of the potentially ciguatera-causing dinoflagellate Gambierdiscus in Japanese coastal areas between 2007 and 2013. Plankton Benthos Res. 13, 46–58. doi: 10.3800/pbr.13.46
Nishitani, L., and Chew, K. (1988). PSP toxins in the Pacific coast states: monitoring programs and effects on bivalve industries. J. Shellfish Res. 7, 653–669.
Oh, S. Y., Kim, D. H., and Yoon, H. J. (2016). Application of unmanned aerial image application red tide monitoring on the aquaculture fields in the coastal waters of the South Sea, Korea. Korean J. Remote Sens. 32, 87–96. doi: 10.7780/kjrs.2016.32.2.2
Olson, M. B., Lessard, E. J., Cochlan, W. P., and Trainer, V. L. (2008). Intrinsic growth and phytoplankton grazing on toxigenic Pseudo-nitzschia spp. Diatoms from the coastal Northeast Pacific. Limnol. Oceanogr. 53, 1352–1368. doi: 10.4319/lo.2008.53.4.1352
Onishi, Y., Mohri, Y., Tuji, A., Ohgi, K., Yamaguchi, A., and Imai, I. (2014). The seagrass Zostera marina harbors growth inhibiting bacteria against the toxic dinoflagellate Alexandrium tamarense. Fish. Sci. 80, 353–362. doi: 10.1007/s12562-013-0688-4
Park, T. G., Lim, W. A., Park, Y. T., Lee, C. K., and Jeong, H. J. (2013). Economic impact, management and mitigation of red tides in Korea. Harmful Algae 30, S131–S143.
Pereira, H. M., Ferrier, S., Walters, M., Geller, G. N., Jongman, R. H. G., Scholes, R. J., et al. (2013). Essential biodiversity variables. Science 339,277–278.
PICES (2014). Proceedings of the Workshop on Economic Impacts of Harmful Algal Blooms on Fisheries and Aquaculture, eds V. L. Trainer and T. Yoshida (Sidney: PICES).
Pierce, R. H., Henry, M. S., Blum, P. C., Hamel, S. L., Kirkpatrick, B., Cheng, Y. S., et al. (2005). Brevetoxin composition in water and marine aerosol along a Florida beach: assessing potential human exposure to marine biotoxins. Harmful Algae 4, 965–972. doi: 10.1016/j.hal.2004.11.004
Pinto, L., Mateus, M., and Silva, A. (2016). Modeling the transport pathways of harmful algal blooms in the Iberian coast. Harmful Algae 53, 8–16. doi: 10.1016/j.hal.2015.12.001
Prakash, D., Dicopolous, J., Roarty, H., Morell, J., Canals, M., and Evans, C. (2018). Development of Sargassum seaweed tracking tools. Oceans2018, 1–6.
Putnam, N. F., Goni, G. J., Gramer, L. J., Hu, C., Johns, E. M., Trinanes, J., et al. (2018). Simulating transport pathways of pelagic Sargassum from the equatorial atlantic into the Caribbean Sea. Prog. Oceanogr. 165, 205–214. doi: 10.1016/j.pocean.2018.06.009
Qi, Y., Chen, J., Wang, Z., Xu, N., Wang, Y., Shen, P., et al. (2004). “Some observations on harmful algal bloom (HAB) events along the coast of Guangdong, southern China in 1998,” in Asian Pacific Phycology in the 21st Century: Prospects and Challenges. Developments in Hydrobiology, ed. P. O. Ang (Dordrecht: Springer), 173.
Quale, D. B. (1966). Paralytic Shellfish Poisoning – Safe Shellfish. Nanaimo, B. C: Fisheries Research Board of Canada Biological Station.
Raine, R. (2014). A review of the biophysical interactions relevant to the promotion of HABs in stratified systems: the case study of Ireland. Deep Sea Res. II 101, 21–31. doi: 10.1016/j.dsr2.2013.06.021
Raine, R., McDermott, G., Silke, J., Lyons, K., Nolan, G., and Cusack, C. (2010). A simple short range model for the prediction of harmful algal events in the bays of southwestern Ireland. J. Mar. Syst. 83, 150–157. doi: 10.1016/j.jmarsys.2010.05.001
Randall, J. E. (2005). Review of clupeotoxism, an often fatal illness from the consumption of clupeoid fishes. Pac. Sci. 59, 73–77. doi: 10.1353/psc.2005.0013
Rensel, J. (1993). Factors controlling paralytic shellfish poisoning (PSP) in Puget Sound, Washington. J. Shellfish Res. 12, 142–143.
Rodríguez, F., Fraga, S., Ramilo, I., Rial, P., Figueroa, R. I., Riobó, P., et al. (2017). Canary Islands (NE Atlantic) as a biodiversity ‘hotspot’ of Gambierdiscus: implications for future trends of ciguatera in the area. Harmful Algae 67, 131–143. doi: 10.1016/j.hal.2017.06.009
Ruberg, S., Brandt, S., Muzzi, R., Hawley, N., Leshkevich, G., Lane, J., et al. (2007). A wireless real-time coastal observation network. EOS Trans. 88, 285–286. doi: 10.1029/2007EO280001
Ruiz-Villarreal, M., García-García, L. M., Cobas, M., Díaz, P. A., and Regura, B. (2016). Modelling the hydrodynamic conditions associated with Dinophysis blooms in Galicia (NW Spain). Harmful Algae 53, 40–52. doi: 10.1016/j.hal.2015.12.003
Ryan, J., Greenfield, D., Marin, R. III, Preston, C., Roman, B., Jensen, S., et al. (2011). Harmful phytoplankton ecology studies using an autonomous molecular analytical and ocean observing network. Limnol. Oceanogr. 56, 1255–1272. doi: 10.4319/lo.2011.56.4.1255
Schell, J. M., Goodwin, D. S., and Siuda, A. N. S. (2015). Recent Sargassum inundation events in the caribbean: shipboard observations reveal dominance of a previously rare form. Oceanography 29, 8–10. doi: 10.5670/oceanog.2015.70
Scholin, C. A., Doucette, G., Jensen, S., Roman, B., Pargett, D., Marin, R. III, et al. (2009). Remote detection of marine microbes, small invertebrates, harmful algae, and biotoxins using the Environmental Sample Processor (ESP). Oceanography 22, 158–167. doi: 10.5670/oceanog.2009.46
Scholin, C. A., Gulland, F., Doucette, G. J., Benson, S., Busman, M., Chavez, F. P., et al. (2000). Mortality of sea lions along the central California coast linked to a toxic diatom bloom. Nature 403, 80–84. doi: 10.1038/47481
Shapiro, J., Dixon, L. K., Schofield, O. M., Kirkpatrick, B., and Kirkpatrick, G. J. (2015). “New sensors for ocean observing: the optical phytoplankton discriminator,” in Coastal Ocean Observing Systems, eds Y. Liu, H. Kerkering, and R. H. Weisberg (Boston, MA: Academic Press), 326–350. doi: 10.1016/b978-0-12-802022-7.00018-3
Shi, X., Liu, L., Li, Y., Xiao, Y., Ding, G., Lin, S., et al. (2018). Isolation of an algicidal bacterium and its effects against the harmful-algal-bloom dinoflagellate Prorocentrum donghaiense (Dinophyceae). Harmful Algae 80, 72–79. doi: 10.1016/j.hal.2018.09.003
Sosik, H. M., and Olson, R. J. (2007). Automated taxonomic classification of phytoplankton sampled with imaging-in-flow cytometry. Limnol Oceanogr Methods 5, 204–216. doi: 10.4319/lom.2007.5.204
Soto, I., Chuanmin, H., Karen, S., Frank, M. K., Jennifer, C., Jennifer, W., et al. (2012). Binational collaboration to study Gulf of Mexico’s harmful algae. EOS Trans. Am. Geophys. Union 5, 49–50. doi: 10.1029/2012eo050002
Srivastava, P. K., Islam, T., Gupta, M., Petropoulos, G., and Dai, Q. (2015). WRF dynamical downscaling and bias correction schemes for NCEP estimated hydro-meteorological variables. Water Resour. Manag. 29, 2267–2284. doi: 10.1007/s11269-015-0940-z
Steffen, M. M., Davis, T. W., McKay, R. M. L., Bullerjahn, G. S., Krausfeldt, L. E., Stough, J. M., et al. (2017). Ecophysiological examination of the Lake Erie microcystis bloom in 2014: linkages between biology and the water supply shutdown of Toledo, OH. Environ. Sci. Technol. 51, 6745–6755. doi: 10.1021/acs.est.7b00856
Stow, C. A., Cha, Y., Johnson, L. T., Confesor, R., and Richards, R. P. (2015). Long-term and seasonal trend decomposition of maumee river nutrient inputs to western Lake Erie. Environ. Sci. Technol. 49, 3392–3400. doi: 10.1021/es5062648
Stumpf, R. P., Tomlinson, M. C., Calkins, J. A., Kirkpatrick, B., Fisher, K., Nierenberg, K., et al. (2009). Skill assessment for an operational algal bloom forecast system. J. Mar. Syst. 76, 151–161. doi: 10.1016/j.jmarsys.2008.05.016
Teng, S. T., Leaw, C. P., Lau, W. L. S., et al. (2016). Recurrence of the harmful dinoflagellate Karlodinium australe along the Johor Strait. Harmful Algal News 52:5.
Tester, P. A., Feldman, R. L., Nau, A. W., Kibler, S. R., and Litaker, R. W. (2010). Ciguatera fish poisoning and sea surface temperatures in the Caribbean Sea and the West Indies. Toxicon 56, 698–710. doi: 10.1016/j.toxicon.2010.02.026
Tester, P. A., Kibler, S. R., Holland, W. C., Usup, G., Vandersea, M. W., Leaw, C. P., et al. (2014). Sampling harmful benthic dinoflagellates: comparison of artificial and natural substrate methods. Harmful Algae 39, 8–25. doi: 10.1016/j.hal.2014.06.009
Trainer, V. L., Bates, S. S., Lundholm, N., Thessen, A. E., Cochlan, W. P., Adams, N. G., et al. (2012). Pseudo-nitzschia physiological ecology, phylogeny, toxicity, monitoring and impacts on ecosystem health. Harmful Algae 14, 271–300. doi: 10.1016/j.hal.2011.10.025
Trainer, V. L., Eberhart, B. T. L., Wekell, J. C., Adams, N. G., Hanson, L., Cox, F., et al. (2003). Paralytic shellfish toxins in Puget Sound, Washington state. J. Shellfish Res. 22, 213–223. doi: 10.1111/j.1529-8817.2012.01175.x
Trainer, V. L., Hickey, B. M., Lessard, E. J., Cochlan, W. P., Trick, C. G., Wells, M. L., et al. (2009a). Variability of Pseudo-nitzschia and domoic acid in the Juan de Fuca eddy region and its adjacent shelves. Limnol. Oceanogr. 54, 289–308. doi: 10.4319/lo.2009.54.1.0289
Trainer, V. L., Wells, M. L., Cochlan, W. P., Trick, C. G., Bill, B. D., Baugh, K. A., et al. (2009b). An ecological study of a massive bloom of toxigenic Pseudo-nitzschia cuspidata off the Washington State coast. Limnol. Oceanogr. 54, 1461–1474. doi: 10.4319/lo.2009.54.5.1461
Trainer, V. L., Moore, L., Bill, B. D., Adams, N. G., Harrington, N., Borchert, J., et al. (2013). Diarrhetic shellfish toxins and other polyether toxins of human health concern in Washington State. Mar. Drugs 11, 1815–1835. doi: 10.3390/md11061815
Trainer, V. L., and Suddleson, M. (2005). Monitoring approaches for early warning of domoic acid events in Washington State. Oceanography 18, 228–237. doi: 10.5670/oceanog.2005.56
UNESCO (2012). A Framework for Ocean Observing. By the Task Team for an Integrated Framework for Sustained Ocean Observing. Paris: UNESCO, doi: 10.5270/OceanObs09-FOO
Vander Woude, A., Ruberg, S., Johengen, T., Miller, R., and Stuart, D. (2019). Spatial and temporal scales of variability of cyanobacteria harmful algal blooms from NOAA GLERL airborne hyperspectral imagery. J. Great Lakes Res. doi: 10.1016/j.jglr.2019.02.006
Vila, M., Abós-Herràndiz, R., Isern-Fontanet, J., Àlvarez, J., and Berdalet, E. (2016). Establishing the link between Ostreopsis cf. ovata blooms and human health impacts using ecology and epidemiology. Sci. Mar. 80, 107–115. doi: 10.3989/scimar.04395.08a
Wang, M., and Hu, C. (2016). Mapping and quantifying Sargassum distribution and coverage in the Central West Atlantic using MODIS observations. Remote Sens. Environ. 183, 356–367. doi: 10.1016/j.rse.2016.04.019
Wang, M., and Hu, C. (2017). Predicting Sargassum blooms in the Caribbean Sea from MODIS observations. Geophys. Res. Lett. 44, 3265–3273. doi: 10.1002/2017GL072932
Webster, R. K., and Linton, T. (2013). Development and implementation of Sargassum early advisory system (SEAS). Shore Beach 81:3.
Wekell, J. C., Gauglitz Jr., E. J., Bamett, H. J., Hatfield, C. L., Simons, D., and Ayres, D. (1994). Occurrence of domoic acid in washington state razor clams (Siliqua patula) during 1991–1993. Nat. Toxins 2, 197–205. doi: 10.1002/nt.2620020408
Wynne, T. T., and Stumpf, R. P. (2015). Spatial and temporal patterns in the seasonal distribution of toxic cyanobacteria in western Lake Erie from2002–2014. Toxins 7, 1649–1663. doi: 10.3390/toxins7051649
Ye, N. H., Zhang, X. W., Mao, Y. Z., Liang, C. W., Xu, D., Zou, J., et al. (2011). ‘Green tides’ are overwhelming the coastline of our blue planet: taking the world’s largest example. Ecol. Res. 26:477. doi: 10.1007/s11284-011-0821-8
Yñiguez, A. T., Maister, J., Villanoy, C. L., Deauna, J. D., Peñaflor, E., Almo, A., et al. (2018). Insights into the dynamics of harmful algal blooms in a tropical estuary through an integrated hydrodynamic-Pyrodinium-shellfish model. Harmful Algae 80, 1–14. doi: 10.1016/j.hal.2018.08.010
Yu, R. C., Lü, S. H., and Liang, Y. B. (2018). “Harmful algal blooms in the coastal waters of China,” in Global Ecology and Oceanography of Harmful Algal Blooms, eds P. Glibert, E. Berdalet, M. Burford, G. Pitcher, and M. Zhou (Cham: Springer), 309–316. doi: 10.1007/978-3-319-70069-4_15
Keywords: biodiversity, phytoplankton, biotoxin, phycotoxin, ecological forecasting, early warning system, stakeholder engagement, earth system science
Citation: Anderson CR, Berdalet E, Kudela RM, Cusack CK, Silke J, O’Rourke E, Dugan D, McCammon M, Newton JA, Moore SK, Paige K, Ruberg S, Morrison JR, Kirkpatrick B, Hubbard K and Morell J (2019) Scaling Up From Regional Case Studies to a Global Harmful Algal Bloom Observing System. Front. Mar. Sci. 6:250. doi: 10.3389/fmars.2019.00250
Received: 12 November 2018; Accepted: 24 April 2019;
Published: 22 May 2019.
Edited by:
Frank Edgar Muller-Karger, University of South Florida, United StatesReviewed by:
Inia M. Soto Ramos, Texas A&M University Corpus Christi, United StatesDigna Tibisay Rueda-Roa, University of South Florida, United States
Copyright © 2019 Anderson, Berdalet, Kudela, Cusack, Silke, O’Rourke, Dugan, McCammon, Newton, Moore, Paige, Ruberg, Morrison, Kirkpatrick, Hubbard and Morell. This is an open-access article distributed under the terms of the Creative Commons Attribution License (CC BY). The use, distribution or reproduction in other forums is permitted, provided the original author(s) and the copyright owner(s) are credited and that the original publication in this journal is cited, in accordance with accepted academic practice. No use, distribution or reproduction is permitted which does not comply with these terms.
*Correspondence: Clarissa R. Anderson, Y2xyYW5kZXJAdWNzZC5lZHU=