- 1National Institute of Water and Atmospheric Research, Wellington, New Zealand
- 2Department of Physics, The University of Auckland, Auckland, New Zealand
- 3National Institute of Water and Atmospheric Research, Christchurch, New Zealand
We argue that there is a separation between studies of the biophysics of natural and “built” marine canopies. Here, by “built” we specifically refer to floating, suspended aquaculture canopies. These structures, combining support infrastructure and crop, exhibit several unique features relative to natural marine canopies, in that they take a particular species, suspend them in spatially structured, mono-cultured arrangement and then induce a systematic harvest cycle. This is in contrast to natural canopies that are irregular and variable in form, have natural recruitment and growth, and sustain some level of biodiversity and more exposed to climate extremes. We synthesize published work to identify the points of difference and similarity with natural canopy studies. This perspective article identifies four main themes relating to (i) key scales, (ii) structural configuration, (iii) connections between biology and physics and (iv) connecting natural and built canopy science. Despite clear differences between natural and built canopies, they have more in common than not and we suggest that both sub-fields would benefit from better connection across the divide.
Introduction
In the marine environment, natural canopies (kelp beds, seagrass meadows etc.) provide significant ecological value (Seitz et al., 2013). At the same time, “built” canopies provided by aquaculture installations have economic value (Troell et al., 2014). We argue that, in part because of these different high-level drivers, there is a separation in the marine canopy biophysics literature whereby it is rare to see studies make the connection between natural marine canopies and built aquaculture canopies. In this Perspective article we consider the nature of the two classes of canopy, explore key comparative themes and then make suggestions for how to build interconnections between the two sub-fields.
Natural canopies are irregular and variable in form, have natural recruitment and growth, and sustain some level of biodiversity. Studies on natural canopies tend to have objectives that are ecosystem function-centric, documenting ecosystem services like maintaining habitats, promoting biodiversity and sustaining pathways of nutrients and matter (e.g., Duarte, 2000). Aquaculture canopies are constructed, regular structures with a controlled mono-culture life-cycle (recruitment and harvesting) and an associated diverse transient fouling community and associated motile fauna (Stevens et al., 2008). Restored natural canopies form an intersectional category (Fonseca and Fisher, 1986). Aquaculture-based studies have focused primarily on (i) how various natural processes affect crop production and (ii) the role of cultured stocks in controlling some coastal ecosystems; largely as a result of how their feeding activity interacts directly and indirectly with energy flow and nutrient cycling. Ecological studies on aquaculture systems have much in common with studies on natural canopies, except that they tend to have at least one anthropogenic objective (i.e., what is the local carrying capacity? e.g., Byron et al., 2011).
Despite these overarching differences, there is little to distinguish the two classes of canopies from hydrodynamic and even biophysical perspectives. Considering flow distortion, turbulence, waves and nutrient transport; natural and built marine canopies have more in common than not – so why are their respective literatures so distinct? This perspective article addresses several fundamental questions in the context similarities and differences between built and natural canopies. First, is the separation real? Assuming it is, what are the key differences in canopy-induced processes for determining feeding and scales of food depletion? Are the differing details of the canopy structural configuration important? Finally, in the two systems do biological aspects like productivity and physiology connect differently with physical processes like transport and turbulence?
Does the Separation Even Exist and Is There Benefit to Closing It?
A consideration of selected papers from the reference list supports the argument that a separation exists as it is rare to see an aquaculture hydrodynamics paper referenced in a natural canopy study, although the reverse is somewhat more common. Built canopies have key attributes that differ from natural canopies (Stevens et al., 2008; Klebert et al., 2013), prime amongst these is structural regularity (Figure 1). For example, a shellfish long-line tethers a sequence of buoys at the water surface and then suspends the shellfish crop beneath. Multiple long-lines are laid out at regular intervals in parallel, occasionally with gaps of open water between lines to improve flushing and/or navigation, to form a “farm.” Similarly, caged fish structures moor a sequence of cages side by side along with their operating infrastructure. The suspended cages or crop extend vertically anywhere from a few meters to nearly on the sea bed. The result, whatever the arrangement, is that a population finds itself constrained mid-water column, some distance from shore with its success depending on adequate food supply and waste removal. The literature on built canopies is clearly useful, in itself, for applied questions relating to crop production and environmental impact. However, some understanding can be transferred in either direction (see Table 1) when considering related processes in natural canopies. In other words, what discoveries from the built literature can aid in understanding natural canopies – and vice versa?
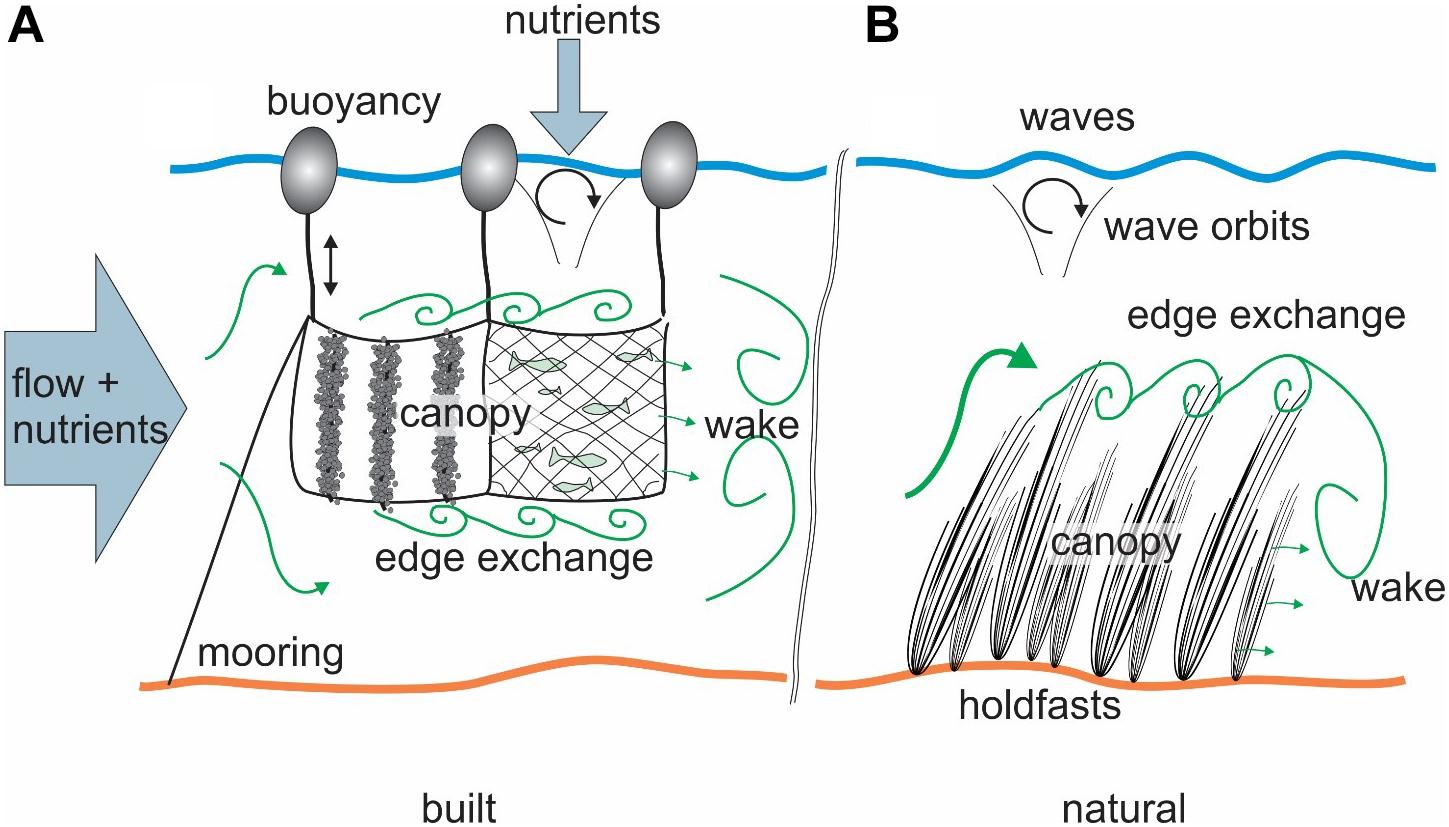
Figure 1. Comparative synthesis diagram showing (A) built (showing both suspended longline shellfish and fish cage examples) and (B) natural (submerged used here as an example) canopies suggesting that while there are similarities in generalities (flow deformation, wakes, shear layers, etc.) there are substantial differences in the details like mooring/holdfast, buoyancy and nutrient supply. The decaying wave orbits in both panels indicate the approximate direct influence of surface waves.
What are the Key Scales for Determining “Footprints”?
When considering either canopy system, one can identify several key scales. The outer boundary conditions are set by (1) the far-field “embayment and inner continental shelf” scale which relates to the regional oceanography and meteorology. Depending on the scale of the canopy, this can reasonably be carried out in the absence of consideration of the effect of the canopy. If, however, the canopy is extensive it may well affect the wider circulation (Plew, 2011b). (2) The canopy/farm-scale is that at which crop feeding and effect is strongly influenced by the canopy and so representation of the details of the canopy is required. Focusing on the immediate region around the canopy provides information about the conditions that the population must live with. This highlights a common challenge at the canopy scale trying to separate the “canopy effect” from background variability. (3) The crop/organism scale is where the feeding takes place (Figure 1). This is likely influenced by the canopy-scale modulation of far-field drivers and needs detailed representation of the canopy and flow variability including waves.
At the regional to large scale, while models can have a vertical dimension (Wu et al., 2017), if the canopy is represented at all, it is typical to represent canopy drag as an enhanced bottom friction in 2D models (Grant and Bacher, 2001; Plew, 2011a,b; Shields et al., 2017). This approximation precludes detailed interaction between canopy and water column stratification (Plew et al., 2006) which can influence where food comes from as well as the fate of impacted water. This remains a challenge for both sub-disciplines.
Focus at the scale of the plant or crop forming the canopy brings the benefit that this is the scale at which biophysical interactions (photosynthesis, feeding, nutrient absorption, etc.) take place (e.g., Ackerman and Nishizaki, 2004). It is instructive to see approaches to determining useful scaling for natural canopy physical effects, like the laboratory experiments of Rosman et al. (2010), consider different physical representations for different scales. In a similar way, numerical models of built canopies move from highly resolved representations at fine scale (Delaux et al., 2011) to coarse representation at wider scales (Plew, 2011a; O’Donncha et al., 2015; Zhao et al., 2017) but the need to represent the canopy in a meaningful way remains. The same decisions need to be made when representing natural canopies (e.g., Mullarney and Henderson, 2018; van Rooijen et al., 2018).
The boundaries of any canopy are critical to quantifying fluxes of material in and out of the canopy region – and are often a key point of difference between built and natural canopies (Figure 1). Consequently, flow instability due to velocity shear is a key flux in both natural (Ghisalberti and Nepf, 2006) and built (Plew et al., 2006) systems. A suspended canopy can potentially sustain such boundaries both above and below the canopy. Adequately determining the mixing at the boundaries of the canopy is fundamental to understanding the canopy biomechanics. This is borne out when examining canopy retention times at larger scales in both natural and built canopies where any attempt at a nutrient budget requires estimation of boundary fluxes (e.g., Nepf et al., 2007; Venayagamoorthy et al., 2011; Lin et al., 2016). In addition, the leading and training edges form critical points whereby accelerated flow around the canopy (Tseung et al., 2016) or the recirculation in the trailing edge (Liu et al., 2017) perform a key mixing role in the near-field.
Are the Details of the Structural Configuration Important?
It is important to consider if the details of the structural configuration have a bearing on the canopy effects – or if mixing or larger-scale variability dominate flows and transfer rates. Certainly, it has been long-established that substrate heterogeneity affects marine species (e.g., Menge, 1976). Shellfish long-line farming structures have a particular set of scales associated with their configuration (number of droppers, spacing, canopy size etc.). But this is not the only such arrangement to be found in built marine systems. Rafted shellfish structures tend to have quite dense aggregations of vertical lines beneath each raft (Newell and Richardson, 2014) but the rafts themselves are relatively well spaced to allow navigation and avoid line entanglement (Blanco et al., 1996). Fish cage installations take this to an extreme where several cages (more like flexible pens) are usually side by side and then the next installation is some distance away. A further complication with fish cages is they confine a free-swimming crop – sometimes in the tens of thousands per cage (He et al., 2018).
The ultimate cross-fertilization in canopy science is the improved understanding of the interaction of different types of canopies (O’Donncha et al., 2017). Abiological canopies are relevant in the discussion of built vs. natural canopies as they provide examples of flow evolution in the absence of any biological process. Marine renewable energy extraction arrays, while not common, are planned and in development in many places and offshore wind farms are already common (Broström, 2008). These would introduce what could be considered very large, sparse canopies. Wave energy devices, in particular, have much in common with early floating breakwaters which in turn were involved in some of the earliest canopy hydrodynamics studies (Elwany et al., 1995; Seymour, 1996). Non-canopy forming aquaculture such as on-bottom bivalve cultivation (e.g., Petersen et al., 2013; Saurel et al., 2013) provides extreme examples of structural configuration effects.
It is common for built canopies to be comprised of regular elements of crop (Figure 1) so that there are gaps in the canopy distribution affecting flow and in-out of canopy exchange (Stevens and Petersen, 2011). High resolution numerical simulations found flow behavior very sensitive to flow-canopy orientation (Delaux et al., 2011). Mixing rates will affect the influence of any heterogeneity (Abdolahpour et al., 2017). Similarly, the role of gaps in canopy-flow exchange is a well-studied aspect of terrestrial canopies (e.g., Bohrer et al., 2008). Despite this, there has been less examination of this facet in the natural canopy literature (except e.g., Rosman et al., 2007; Kregting et al., 2011; El Allaoui et al., 2015; Hamed et al., 2017). Related to this point, most built canopies (aquaculture) are relatively sparse compared to natural canopies, and their dimensions commonly insufficient for a fully developed canopy flow to occur (the transition length is a function of the density of the canopy, Tseung et al., 2016). It is also important to recognize that the structural regularity actually makes some of the built canopy-scale observations possible. For example, it would not be possible to tow an undulator through most natural macroalgal canopies, whereas it is possible to work in the channels between shellfish rafts and long lines (Cranford et al., 2014).
An example of where built canopy understanding can benefit from natural canopy work is the role of surface waves (Figure 1). The macroalgal literature continues to make substantial advances in understanding of the response of individuals and canopies to wave forcing (e.g., Denny et al., 1997; Mullarney and Henderson, 2018). While some open-ocean studies exist (Plew et al., 2005; Gentry et al., 2017), aquaculture typically takes place in sheltered waters, where it is implicit that the farms will only be exposed to fetch-limited (i.e., short wavelength) waves. The influence of a surface wave decays exponentially with depth to zero, at half the wavelength (which is short). A consequence is that in aquaculture studies it is unusual to consider the influence of waves upon shellfish growth and behavior. However, it is clear that, as for a buoyant macroalgal frond, suspended canopy or raft shellfish culture structures directly link the crop to the surface wave field through the buoyancy of the supporting elements (Stevens et al., 2007). Therefore, even short wavelength waves can induce vertical velocities at depth in built canopies. This results in a relative velocity between crop and water – potentially with a strong vertical component. Again, relative motion in suspended natural canopies has been explored (Stevens et al., 2001; Lowe et al., 2005), along with waves associated with the canopy itself (monami – Ghisalberti and Nepf, 2009). So, it is not remarkable that this could affect shellfish and even caged fish and is a topic where the built canopy science stands to gain from the experiences of the macroalgal biophysics literature.
Are the Connections Between Biological and Physical Processes Understood?
There are a range of connections between biology and physics – most of which will vary depending on canopy organism (e.g., Riisgård et al., 2011) and the in-canopy flow conditions. At the largest scale, the changing climate is central to natural canopy biophysical studies (e.g., Arias-Ortiz et al., 2018). Phenomenon like marine heat waves are impacting on both kinds of canopy and the associated ecosystems (Wernberg et al., 2016), but only the built canopy systems are in a position to rapidly adjust (Weatherdon et al., 2016).
Often these connections at the large scale, and the canopy differences, cascade to incorporate smaller scale interactions. For example, mussels generate feeding flows associated with their siphons (Riisgård et al., 2011), algae rely on diffusive processes (Hurd, 2000) and caged fish generate canopy-scale flows through their motility (Gansel et al., 2014; Johansson et al., 2014). The flow variability in the crop near-field suggests that any instantaneous snapshot of food depletion from a single point would fail to identify the effect of the feeding. This highlights the need when talking about scale to consider temporal scales also. For example, a “nutrient halo” whose scale is determined by time-averaging background turbulence and feeding rate as identified by Nielsen et al. (2016). Working at this crop-scale in natural (O’Riordan et al., 1995) and built (Plew et al., 2009) shellfish applications has a direct analogy with biophysics at the canopy-forming individual scale.
In a recent review of the magnitude and spatial extent of food depletion by bivalve aquaculture, Cranford (2018) emphasized the important role of the built canopy in limiting food depletion at all spatial scales. Canopy-induced flow reduction directly affects the degree of food depletion around individual mussel droppers as a result of the mussels re-filtering more of the same water instead of the feeding zone being sufficiently replenished by advection. This canopy effect at the scale of individual mussels ultimately limits the degree of depletion at canopy- and ecosystem-scales. Canopy-induced flow reduction has been shown to result in food depletion levels that are substantially lower than predicted by models that do not account for this physical effect (Cranford et al., 2014; Nielsen et al., 2016). Similar effects are seen in natural canopy studies (Boyle et al., 2004) but it would appear to be more difficult to achieve the depletion vs. distance measurements in a natural canopy as the crop elements are not so consistently spatially separated from one another.
Turbulence is a key quantity that influences transfer rates of material and energy both within, at the boundaries of, and in the background flow. One overarching question in all canopy mechanics is – “does the canopy generate more turbulence?” The answer is seemingly obvious – yes, due to the wakes of all the structural material in the water column. However, with the drag of the canopy and the slow-down of flow at the embayment scale (Plew et al., 2005), the system has the potential to produce less drag and wake at the individual element scale (Cranford et al., 2014). This is a demonstration of the multi-scale nature of flow-canopy interaction and seen equally in natural canopies (Ackerman and Okubo, 1993). Getting this balance right is fundamental to understanding mass fluxes in canopies (e.g., Pilditch et al., 2001; Strohmeier et al., 2005; Gaylord et al., 2007). Furthermore, the nature of the canopy edges has some bearing of how the wake develops as a more porous canopy with a relatively diffuse boundary will likely have a different wake than the abrupt end of a built canopy (Figure 1). The limited range of scales in built canopies lends itself to being more readily empirically quantified – the results of which are useful to both sub-disciplines.
Closing the Separation
Despite the various differences (Figure 1 and Table 1) in canopy structure between built and natural systems, they have much in common. Furthermore, we suggest that studies of a particular approach (field observation, numerical simulations, physical model) have more in common with each other, regardless of being built or natural canopies, compared to the differences between approaches – which are substantial. It is not uncommon to see that studies designed to explore natural canopy behavior, especially where the approach involves physical or numerical modeling often appears as if it would have at least as relevant to built canopies as it does with the target natural canopy (e.g., Rosman et al., 2007; Qiao et al., 2016). Conversely, because they are constructed from a limited set of scales, built canopy studies seek to reduce, as much as is possible, the level of complexity when exploring flow-canopy interactions.
It is clear that the flow of information should be bi-directional. While debatable, we suggest that the natural canopy studies approach a problem with a more open perspective(e.g., Gaylord et al., 2007; Fram et al., 2008) than aquaculture studies which might have some focused targets (e.g., Maar et al., 2010; Newell and Richardson, 2014). This is in-part, we believe, due to the differing overarching drivers (economic vs. ecosystem) as well as the only partially overlapping research communities.
This perspective article is a call to better connect between the two fields of endeavor – so how do we do this? Certainly, it requires a better awareness of the various literature threads from both sides and cross-over of application of results. A common language should be possible relating in-water mixing processes to the canopy and substrate and the associated feedbacks (Nepf, 1999). It is likely that the research communities do not overlap sufficiently, so targeted combined special sessions at conferences would be one avenue of fostering better integration. A valuable result of such sessions would be an integrated review article. Ultimately, studies that seamlessly integrate across the separation to get at the best representation of bio-mechanics stand to generate the greatest advances.
Author Contributions
CS and DP conceived the ideas. CS wrote the manuscript.
Funding
This work was supported by the National Institute for Water and Atmospheric Research (NIWA) Aquaculture Sustainability Program, the Danish Council for Strategic Research, research project “Production of Mussels: Mitigation and Feed for Husbandry (MuMiHus)” under grant agreement no. 09-066983 and the New Zealand Sustainable Seas National Science Challenge Project 4.2.2 Stressor Footprints.
Conflict of Interest Statement
The authors declare that the research was conducted in the absence of any commercial or financial relationships that could be construed as a potential conflict of interest.
Acknowledgments
We would like to thank Pernille Nielsen, Peter Cranford, Jens K. Petersen, Barb Hayden, Joe O’Callaghan, and Niall Broekhuizen for support and advice. We would also like to thank two reviewers and the editor for their input and suggestions.
References
Abdolahpour, M., Ghisalberti, M., Lavery, P., and McMahon, K. (2017). Vertical mixing in coastal canopies. Limnol. Oceanogr. 62, 26–42. doi: 10.1002/lno.10368
Ackerman, J. D., and Nishizaki, M. T. (2004). The effect of velocity on the suspension feeding and growth of the marine mussels Mytilus trossulus and M. californianus: implications for niche separation. J. Mar. Sys. 49, 195–208.
Ackerman, J. D., and Okubo, A. (1993). Reduced mixing in a marine macrophyte canopy. Funct. Ecol. 7, 305–309. doi: 10.3732/ajb.89.7.1119
Arias-Ortiz, A., Serrano, O., Masqué, P., Lavery, P. S., Mueller, U., Kendrick, G. A., et al. (2018). A marine heatwave drives massive losses from the world’s largest seagrass carbon stocks. Nat. Clim. Chang. 8:338. doi: 10.1038/s41558-018-0096-y
Blanco, J., Zapata, M., and Moroño, Á. (1996). Some aspects of the water flow through mussel rafts. Sci. Mar. 60, 275–282.
Bohrer, G., Katul, G. G., Nathan, R., Walko, R. L., and Avissar, R. (2008). Effects of canopy heterogeneity, seed abscission and inertia on wind-driven dispersal kernels of tree seeds. J. Ecol. 96, 569–580. doi: 10.1111/j.1365-2745.2008.01368.x
Boyle, K. A., Kamer, K., and Fong, P. (2004). Spatial and temporal patterns in sediment and water column nutrients in a eutrophic southern California estuary. Estuaries 27, 378–388. doi: 10.1007/bf02803530
Broström, G. (2008). On the influence of large wind farms on the upper ocean circulation. J. Mar. Syst. 74, 585–591. doi: 10.1016/j.jmarsys.2008.05.001
Byron, C., Bengtson, D., Costa-Pierce, B., and Calanni, J. (2011). Integrating science into management: ecological carrying capacity of bivalve shellfish aquaculture. Mar. Policy 35, 363–370. doi: 10.1016/j.marpol.2010.10.016
Cranford, P. J. (2018). “Magnitude and extent of water clarification services provided by bivalve suspension feeding,” in Goods and Services of Marine Bivalves, eds A. Smaal, J. Ferreira, J. Grant, J. Petersen, and Ø Strand (Cham: Springer).
Cranford, P. J., Duarte, P., Robinson, S. M., Fernández-Reiriz, M. J., and Labarta, U. (2014). Suspended particulate matter depletion and flow modification inside mussel (Mytilus galloprovincialis) culture rafts in the Ría de Betanzos, Spain. J. Exp. Mar. Biol. Ecol. 452, 70–81. doi: 10.1016/j.jembe.2013.12.005
Delaux, S., Stevens, C. L., and Popinet, S. (2011). High-resolution computational fluid dynamics modelling of suspended shellfish structures. Environ. Fluid Mech. 11, 405–425. doi: 10.1007/s10652-010-9183-y
Denny, M. W., Gaylord, B. P., and Cowen, E. A. (1997). Flow and flexibility II. The roles of size and shape in determining wave forces on the bull kelp Nereocystis luetkeana. J. Exp. Biol. 200, 3165–3183.
Duarte, C. M. (2000). Marine biodiversity and ecosystem services: an elusive link. J. Exp. Mar. Biol. Ecol. 250, 117–131. doi: 10.1016/s0022-0981(00)00194-5
El Allaoui, N., Serra, T., Soler, M., Colomer, J., Pujol, D., and Oldham, C. (2015). Modified hydrodynamics in canopies with longitudinal gaps exposed to oscillatory flows. J. Hydrol. 531, 840–849. doi: 10.1016/j.jhydrol.2015.10.041
Elwany, M. H. S., O’Rielly, W. C., Guza, R. T., and Flick, R. T. (1995). Effects of southern California kelp beds on waves. J. Waterway Port Coast. Ocean Eng. 121, 143–150. doi: 10.1061/(asce)0733-950x(1995)121:2(143)
Fonseca, M. S., and Fisher, J. S. (1986). A comparison of canopy friction and sediment movement between four species of seagrass with reference to their ecology and restoration. Mar. Ecol. Prog. Ser. 29, 15–22. doi: 10.3354/meps029015
Fram, J. P., Stewart, H. L., Brzezinski, M. A., Gaylord, B., Reed, D. C., Williams, S. L., et al. (2008). Physical pathways and utilization of nitrate supply to the giant kelp, Macrocystis pyrifera. Limnol. Oceanogr. 53, 1589–1603. doi: 10.4319/lo.2008.53.4.1589
Gansel, L. C., Rackebrandt, S., Oppedal, F., and McClimans, T. A. (2014). Flow fields inside stocked fish cages and the near environment. J. Offshore Mech. Arct. Eng. 136, 031201. doi: 10.1115/1.4027746
Gaylord, B., Rosman, J. H., Reed, D. C., Koseff, J. R., Fram, J., MacIntyre, S., et al. (2007). Spatial patterns of flow and their modification within and around a giant kelp forest. Limnol. Oceanogr. 52, 1838–1852. doi: 10.4319/lo.2007.52.5.1838
Gentry, R. R., Froehlich, H. E., Grimm, D., Kareiva, P., Parke, M., Rust, M., et al. (2017). Mapping the global potential for marine aquaculture. Nat. Ecol. Evol. 1:1317. doi: 10.1038/s41559-017-0257-9
Ghisalberti, M., and Nepf, H. (2006). The structure of the shear layer in flows over rigid and flexible canopies. Environ. Fluid Mech. 6, 277–301. doi: 10.1007/s10652-006-0002-4
Ghisalberti, M., and Nepf, H. (2009). Shallow flows over a permeable medium: the hydrodynamics of submerged aquatic canopies. Trans. Porous Media 78:309. doi: 10.1007/s11242-008-9305-x
Grant, J., and Bacher, C. (2001). A numerical model of flow modification induced by suspended aquaculture in a Chinese bay. Can. J. Fish. Aquat. Sci. 58, 1003–1011. doi: 10.1139/f01-027
Hamed, A. M., Sadowski, M. J., Nepf, H. M., and Chamorro, L. P. (2017). Impact of height heterogeneity on canopy turbulence. J. Fluid Mech. 813, 1176–1196. doi: 10.1017/jfm.2017.22
He, Z., Faltinsen, O. M., Fredheim, A., and Kristiansen, T. (2018). The influence of fish on the mooring loads of a floating net cage. J. Fluids Struct. 76, 384–395. doi: 10.1016/j.jfluidstructs.2017.10.016
Hurd, C. L. (2000). Water motion, marine macroalgal physiology, and production. J. Phycol. 36, 453–472. doi: 10.1046/j.1529-8817.2000.99139.x
Johansson, D., Laursen, F., Fernö, A., Fosseidengen, J. E., Klebert, P., Stien, L. H., et al. (2014). The interaction between water currents and salmon swimming behaviour in sea cages. PLoS One 9:e97635. doi: 10.1371/journal.pone.0097635
Klebert, P., Lader, P., Gansel, L., and Oppedal, F. (2013). Hydrodynamic interactions on net panel and aquaculture fish cages: a review. Ocean Eng. 58, 260–274. doi: 10.1016/j.oceaneng.2012.11.006
Kregting, L. T., Stevens, C. L., Cornelisen, C. D., Pilditch, C. A., and Hurd, C. L. (2011). Effects of a small-bladed macroalgal canopy on benthic boundary layer dynamics: Implications for nutrient transport. Aquat. Biol. 14, 41–56. doi: 10.3354/ab00369
Lin, J., Li, C., and Zhang, S. (2016). Hydrodynamic effect of a large offshore mussel suspended aquaculture farm. Aquaculture 451, 147–155. doi: 10.1016/j.aquaculture.2015.08.039
Liu, C., Hu, Z., Lei, J., and Nepf, H. (2017). Vortex structure and sediment deposition in the wake behind a finite patch of model submerged vegetation. J. Hydr. Eng. 144:04017065. doi: 10.1061/(asce)hy.1943-7900.0001408
Lowe, R. J., Koseff, J. R., Monismith, S. G., and Falter, J. L. (2005). ). Oscillatory flow through submerged canopies. Part 2. Canopy mass transfer. J. Geophys. Res. 110:C10017. doi: 10.1029/2004JC002789
Maar, M., Timmermann, K., Petersen, J. K., Gustafsson, K. E., and Storm, L. M. (2010). A model study of the regulation of blue mussels by nutrient loadings and water column stability in a shallow estuary, the Limfjorden. J. Sea Res. 64, 322–333. doi: 10.1016/j.seares.2010.04.007
Menge, B. A. (1976). Organization of the New England rocky intertidal community: role of predation, competition, and environmental heterogeneity. Ecol. Monogr. 46, 355–393. doi: 10.2307/1942563
Mullarney, J. C., and Henderson, S. M. (2018). “Flows within marine vegetation canopies,” in Advances in Coastal Hydraulics, eds V. Panchang and J. Kaihatu (Singapore: World Scientific), 1–46. doi: 10.1142/9789813231283_0001
Nepf, H., Ghisalberti, M., White, B., and Murphy, E. (2007). Retention time and dispersion associated with submerged aquatic canopies. Water Resourc. Res. 43, 1–10. doi: 10.1029/2006WR005362
Nepf, H. M. (1999). Drag, turbulence, and diffusion in flow through emergent vegetation. Water Resourc. Res. 35, 479–489. doi: 10.1016/j.watres.2008.10.027
Newell, C. R., and Richardson, J. (2014). The effects of ambient and aquaculture structure hydrodynamics on the food supply and demand of mussel rafts. J. Shellfish Res. 33, 257–272. doi: 10.2983/035.033.0125
Nielsen, P., Cranford, P. J., Maar, M., and Petersen, J. K. (2016). Magnitude, spatial scale and optimization of ecosystem services from a nutrient extraction mussel farm in the eutrophic Skive Fjord. Denmark. Aquac. Environ. Interact. 8, 311–329. doi: 10.3354/aei00175
O’Donncha, F., Hartnett, M., and Plew, D. R. (2015). Parameterizing suspended canopy effects in a three-dimensional hydrodynamic model. J. Hydr. Res. 53, 714–727. doi: 10.1080/00221686.2015.1093036
O’Donncha, F., James, S. C., and Ragnoli, E. (2017). Modelling study of the effects of suspended aquaculture installations on tidal stream generation in Cobscook Bay. Renew. Energy 102, 65–76. doi: 10.1016/j.renene.2016.10.024
O’Riordan, C. A., Monismith, S. G., and Koseff, J. R. (1995). The effect of bivalve excurrent jet dynamics on mass transfer in a benthic boundary layer. Limnol. Oceanogr. 40, 330–344. doi: 10.4319/lo.1995.40.2.0330
Petersen, J. K., Maar, M., Ysebaert, T., and Herman, P. M. (2013). Near-bed gradients in particles and nutrients above a mussel bed in the Limfjorden: influence of physical mixing and mussel filtration. Mar. Ecol. Prog. Ser. 490, 137–146. doi: 10.3354/meps10444
Pilditch, C. A., Grant, J., and Bryan, K. R. (2001). Seston supply to scallops in suspended culture. Can. J. Fish. Aquat. Sci. 58, 241–253. doi: 10.1139/f00-242
Plew, D. R. (2011a). Depth-averaged drag coefficient for modeling flow through suspended canopies. J. Hydr. Eng. 137, 234–247. doi: 10.1061/(asce)hy.1943-7900.0000300
Plew, D. R. (2011b). Shellfish farm-induced changes to tidal circulation in an embayment, and implications for seston depletion. Aquac. Environ. Interact. 1, 201–214. doi: 10.3354/aei00020
Plew, D. R., Enright, M. P., Nokes, R. I., and Dumas, J. K. (2009). Effect of mussel bio-pumping on the drag on and flow around a mussel crop rope. Aquac. Eng. 40, 55–61. doi: 10.1016/j.aquaeng.2008.12.003
Plew, D. R., Spigel, R. H., Stevens, C. L., Nokes, R. I., and Davidson, M. J. (2006). Stratified flow interactions with a suspended canopy. Environ. Fluid Mech. 6, 519–539. doi: 10.1007/s10652-006-9008-1
Plew, D. R., Stevens, C. L., Spigel, R. H., and Hartstein, N. D. (2005). Hydrodynamic implications of large offshore mussel farms. IEEE J. Ocean. Eng. 30, 95–108. doi: 10.1109/joe.2004.841387
Qiao, J. D., Delavan, S. K., Nokes, R. I., and Plew, D. R. (2016). Flow structure and turbulence characteristics downstream of a spanwise suspended linear array. Environ. Fluid Mech. 16, 1021–1041. doi: 10.1007/s10652-016-9465-0
Riisgård, H. U., Jørgensen, B. H., Lundgreen, K., Storti, F., Walther, J. H., Meyer, K. E., et al. (2011). The exhalant jet of mussels Mytilus edulis. Mar. Ecol. Progr. Ser. 437, 147–164. doi: 10.3354/meps09268
Rosman, J. H., Koseff, J. R., Monismith, S. G., and Grover, J. (2007). A field investigation into the effects of a kelp forest (Macrocystis pyrifera) on coastal hydrodynamics and transport. J. Geophys. Res. Oceans 112:C2. doi: 10.1029/2005JC003430
Rosman, J. H., Monismith, S. G., Denny, M. W., and Koseff, J. R. (2010). Currents and turbulence within a kelp forest (Macrocystis pyrifera): Insights from a dynamically scaled laboratory model. Limnol. Oceanogr. 55, 1145–1158. doi: 10.4319/lo.2010.55.3.1145
Saurel, C., Petersen, J. K., Wiles, P. J., and Kaiser, M. J. (2013). Turbulent mixing limits mussel feeding: direct estimates of feeding rate and vertical diffusivity. Mar. Ecol. Prog. Ser. 485, 105–121. doi: 10.3354/meps10309
Seitz, R. D., Wennhage, H., Bergström, U., Lipcius, R. N., and Ysebaert, T. (2013). Ecological value of coastal habitats for commercially and ecologically important species. ICES J. Mar. Sci. 71, 648–665. doi: 10.1093/icesjms/fst152
Seymour, R. J. (1996). Discussion of Effects of southern California kelp beds on waves. J. Waterway Port Coast. Ocean Eng. 122, 207–208. doi: 10.1061/(asce)0733-950x(1996)122:4(207)
Shields, F. D. Jr., Coulton, K. G., and Nepf, H. (2017). Representation of vegetation in two-dimensional hydrodynamic models. J. Hydr. Eng. 143:02517002. doi: 10.1061/(ASCE)HY.1943-7900.0001320
Stevens, C., Plew, D., Hartstein, N., and Fredriksson, D. (2008). The physics of open-water shellfish aquaculture. Aquac. Eng. 38, 145–160. doi: 10.1016/j.aquaeng.2008.01.006
Stevens, C. L., Hurd, C. L., and Smith, M. J. (2001). Water motion relative to subtidal kelp fronds. Limnol. Oceanogr. 46, 668–678. doi: 10.4319/lo.2001.46.3.0668
Stevens, C. L., and Petersen, J. K. (2011). Turbulent, stratified flow through a suspended shellfish canopy: implications for mussel farm design. Aquac. Environ. Interact. 2, 87–104. doi: 10.3354/aei00033
Stevens, C. L., Plew, D. R., Smith, M. J., and Fredriksson, D. W. (2007). Hydrodynamic forcing of long-line mussel farms: observations. J. Waterway Port Coast. Ocean Eng. 133, 192–199. doi: 10.1061/(asce)0733-950x(2007)133:3(192)
Strohmeier, T., Aure, J., Duinker, A., Castberg, T., Svardal, A., and Strand, O. (2005). Flow reduction, seston depletion, meat content and distribution of diarrhetic shellfish toxins in a long-line blue mussel (Mytilus edulis) farm. J. Shellfish Res. 24, 15–23. doi: 10.2983/0730-8000(2005)24
Troell, M., Naylor, R. L., Metian, M., Beveridge, M., Tyedmers, P. H., Folke, C., et al. (2014). Does aquaculture add resilience to the global food system? Proc. Natl. Acad. Sci. U.S.A. 111, 13257–13263. doi: 10.1073/pnas.1404067111
Tseung, H. L., Kikkert, G. A., and Plew, D. (2016). Hydrodynamics of suspended canopies with limited length and width. Environ. Fluid Mech. 16, 145–166. doi: 10.1007/s10652-015-9419-y
van Rooijen, A., Lowe, R., Ghisalberti, M., Conde-Frias, M., and Tan, L. (2018). Predicting current-induced drag in emergent and submerged aquatic vegetation canopies. Front. Mar. Sci. 5:449. doi: 10.3389/fmars.2018.00449
Venayagamoorthy, S. K., Ku, H., Fringer, O. B., Chiu, A., Naylor, R. L., and Koseff, J. R. (2011). Numerical modeling of aquaculture dissolved waste transport in a coastal embayment. Environ. Fluid Mech. 11, 329–352. doi: 10.1007/s10652-011-9209-0
Weatherdon, L. V., Magnan, A. K., Rogers, A. D., Sumaila, U. R., and Cheung, W. W. (2016). Observed and projected impacts of climate change on marine fisheries, aquaculture, coastal tourism, and human health: an update. Front. Mar. Sci. 3:48. doi: 10.3389/fmars.2016.00048
Wernberg, T., Bennett, S., Babcock, R. C., De Bettignies, T., Cure, K., Depczynski, M., et al. (2016). Climate-driven regime shift of a temperate marine ecosystem. Science 353, 169–172. doi: 10.1126/science.aad8745
Wu, Y., Hannah, C. G., O’Flaherty-Sproul, M., and Thupaki, P. (2017). Representing kelp forests in a tidal circulation model. J. Mar. Syst. 169, 73–86. doi: 10.1016/j.jmarsys.2016.12.007
Keywords: canopies, stratification, mixing, biophysics, flow-structure interactions
Citation: Stevens C and Plew D (2019) Bridging the Separation Between Studies of the Biophysics of Natural and Built Marine Canopies. Front. Mar. Sci. 6:217. doi: 10.3389/fmars.2019.00217
Received: 20 November 2018; Accepted: 08 April 2019;
Published: 24 April 2019.
Edited by:
Marco Ghisalberti, The University of Western Australia, AustraliaReviewed by:
Rodrigo Riera, Catholic University of the Most Holy Conception, ChileAndrew William Mackay Pomeroy, The University of Western Australia, Australia
Copyright © 2019 Stevens and Plew. This is an open-access article distributed under the terms of the Creative Commons Attribution License (CC BY). The use, distribution or reproduction in other forums is permitted, provided the original author(s) and the copyright owner(s) are credited and that the original publication in this journal is cited, in accordance with accepted academic practice. No use, distribution or reproduction is permitted which does not comply with these terms.
*Correspondence: Craig Stevens, Y3JhaWcuc3RldmVuc0BuaXdhLmNvLm56