- 1School of Biological Sciences, Victoria University of Wellington, Wellington, New Zealand
- 2Oceans Institute, Oceans Graduate School, and Australian Research Council Centre of Excellence for Coral Reef Studies, The University of Western Australia, Crawley, WA, Australia
- 3School of Environment and Science, and Australian Rivers Institute, Griffith University, Brisbane, QLD, Australia
- 4Laboratoire d'Océanographie de Villefranche, Sorbonne Université, CNRS-INSU, Villefranche-sur-Mer, France
Coralline algae are foundation species in many hard-bottom ecosystems acting as a settlement substrate, and binding together and even creating reefs in some locations. Ocean acidification is known to be a major threat to coralline algae. However, the effects of ocean warming are less certain. Here we bring multiple lines of evidence together to discuss the potential impacts of ocean warming on these ecologically crucial taxa. We use a meta-analysis of 40 responses within 14 different studies available which assessed the effects of increasing temperature on coralline algal calcification in laboratory experiments. We find a net negative impact of increasing temperature on coralline algal calcification at 5.2°C above ambient conditions. Conversely, negative effects are observed when temperature drops below 2.0°C from ambient conditions. We propose that some coralline algae will be more capable of both acclimatizing and locally adapting to increasing ocean temperatures over the coming decades. This is because many species possess short generation times, the ability to opportunistically rapidly utilize open space, and relatively high phenotypic plasticity. However, less resistant and resilient species will be those that are long-lived, those with long generation times, or with narrow thermal tolerances (e.g., tropical taxa living close to their thermal maxima). Additionally, ocean warming will occur simultaneously with ocean acidification, a potentially greater threat to coralline algae, which could also reduce any tolerance to ocean warming for many species. To maximize the potential to accurately determine how coralline algae will respond to future ocean warming and marine heatwaves, future research should use environmentally relevant temperature treatments, use appropriate acclimation times and follow best practices in experimental design.
Introduction
Marine climate change has been under increasing focus over the last two decades, particularly the dual threats of ocean acidification and warming (Gattuso et al., 2015; Riebesell and Gattuso, 2015). Coralline algae are one of the most crucial foundation taxa in the photic zone. They form extensive reefs in tropic regions, rhodolith beds (e.g., free living crustose coralline algae, or CCA) in warm-temperate to Arctic habitats (Foster, 2001), and also act to bind together substrates in traditional coral habitats and temperate habitats (Adey, 1978; Steneck, 1986). While in some tropical locations they only form caps over dead corals (“algal ridges”) (Adey, 1978), coralline algal reefs exist, including the 380 km2 Montgomery Reef comprised almost entirely of CCA (Heyward and Moore, 2009; Le Nohaïc et al., 2017). CCA also act as important settlement substrates for many invertebrates such as corals, sea urchins and abalone (Pearce and Scheibling, 1991; Heyward and Negri, 1999; Roberts, 2001; Tebben et al., 2015). Because of their crucial ecological roles throughout the oceans' photic zones, it is therefore of paramount interest to understand how their future ecological role will be altered.
Ocean warming is perceived as a great threat to key foundation species such as corals and kelp (Wernberg et al., 2016; Hughes et al., 2017), but we have relatively little understanding of its effects on coralline algae (Martone et al., 2010). Average sea surface temperatures will rise by 2.73°C by 2100 under Representative Concentration Pathway 8.5 (Gattuso et al., 2015), the scenario now considered most likely. Elevated temperatures from marine heat waves causes widespread coral bleaching resulting in high mortality of many coral species (Hughes et al., 2017, 2018), and can also cause loss of temperate kelp forests and gorgonian beds (Garrabou et al., 2009; Wernberg et al., 2013, 2016; Krumhansl et al., 2016). Because of increasing frequencies of marine heatwaves due to ocean warming (Oliver et al., 2018) there has been a rapid increase in research identifying the responses of some habitat-forming species such as corals, gorgonians, and kelp to elevated temperature (Wernberg et al., 2012; Hughes et al., 2017). For example, loss of the kelps Macrocystis pyrifera and Ecklonia radiata in Australia has caused irreversible ecological change to more depauperate communities (Johnson et al., 2011; Wernberg et al., 2013, 2016; Vergés et al., 2016). However, while coralline algae are ecologically crucial in most hard-bottom habitats within the photic zone, such as coral reefs and temperate kelp forests, there is a paucity of information detailing coralline algal responses to ocean warming. This in contrast to the intensive research investment in the impacts of ocean acidification. For example, we find 78 articles on the impacts of ocean acidification on coralline algal calcification, but only 14 on ocean warming or its interactive effects with acidification (see methods below).
Here we review the existing literature on coralline algal responses to experimental manipulations of temperature in isolation and examine the magnitude and direction of these responses. There has been immense research effort placed into synthesizing how the physiological effects of ocean acidification will manifest at ecological levels (Hall-Spencer et al., 2008; Fabricius et al., 2011; Ordoñez et al., 2014; Cornwall et al., 2017b), and why (Cornwall et al., 2017a; Barner et al., 2018). Hence, there have also been numerous reviews on the impacts of ocean acidification on coralline algae (Hurd et al., 2009; Nelson, 2009; Roleda and Hurd, 2012; Hofmann and Bischof, 2014; Mccoy and Kamenos, 2015). However, relatively little effort has been placed on synthesizing the impacts of ocean warming, and there is no dedicated review of evidence to date. Therefore, here we assess coralline algal response to changes in temperature in laboratory experiments using a meta-analysis approach, and then discuss their capacity for migration away from, acclimatization, or adaption to continued ocean warming into the Anthropocene. We also provide recommendations for future research on the topic. We focus our review on the response of calcification, as this process is critically important for maintaining growth (accretion) and structural complexity of tropical and temperate reefs, as well as rhodolith beds. It is also a measure of fitness and the most commonly measured response in eco-physiological studies (see below).
Response to Temperature in Laboratory Experiments
Meta-Analysis Methods
We assessed the literature and found 36 studies that assessed the effects of temperature on coralline algal physiology. Briefly: we narrowed these 36 studies down to 14 that assessed the effects of temperature on calcification. To be included, a study needed to use one of the two following methods to measure calcification: (1) Via calcium carbonate accretion using the buoyant weighing technique, (2) measure metabolic calcification via the total alkalinity anomaly, or calcium isotope techniques, when specimens were exposed to treatment conditions for more than 10 days, or (3) using changes in linear extension where different treatments were employed simultaneously. These studies are detailed in Supplementary Table 1. We assessed the independent effects of temperature at control pH levels for those studies that examined the interactive effects of ocean warming and acidification, because the goal of the present study was to look only at the effect of ocean warming. Calcification was assessed because of its ecological relevance, as mentioned above, but also because it was the most measured parameter in research assessing the response of coralline algae to manipulated temperatures (14 studies), with photosynthesis being a distant 2nd (6 studies). We log transformed mean responses of experimental and control treatments according to Hedges et al. (1999) to create mean log response ratios, then calculated variance from standard deviations, standard errors, or confidence intervals given in each study.
We then conducted a meta-analysis in R with the metaphor package that assessed the effects of (1) decreasing temperature relative to a control designated by the authors of each study, and (2) increasing temperature relative to the same control. Weighting was given to each study so the impacts of any one study with multiple temperature levels were controlled for in a mixed model linear regression meta-analysis. We also summarize the main findings of each publication, including those excluded from the meta-analysis (see Supplementary Material).
Meta-Analysis Results and Discussion
Not surprisingly, we found that both decreasing and increasing temperature had negative impacts on coralline algal calcification (Figure 1 and Table 1). What was unexpected though, was that the mean effect of elevated temperature on coralline algal calcification only manifests at 5.23°C above the temperatures that individual authors considered were representative of control or ambient temperature across both cool and warm temperate locations, and tropical coral reefs (Figure 1). The current “worse-case” representative concentration pathways (RCP 8.5) are predicted to result in mean increases in surface seawaters by 2.73°C by the year 2100 (Gattuso et al., 2015). This indicates coralline algal calcification may be relatively robust to ocean warming compared to other taxa, such as corals (Kornder et al., 2018). The comparatively greater decline in calcification in response to decreasing temperatures seems superficially surprising. However, these apparently greater responses could be due to the cumulative effects of three factors: (1) cold-stress reducing organism fitness as lower Δ temperatures; (2) metabolic depression, often resulting from decreasing temperature, and (3) the fact that precipitation is thermodynamically favored at higher temperatures (Mcculloch et al., 2012; Pörtner, 2012).
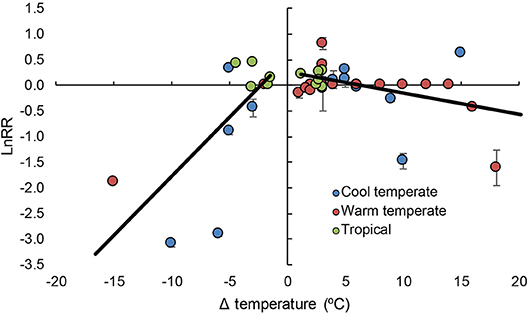
Figure 1. Log response ratios of coralline algal calcification to changes in temperature across different latitudinal regions in 14 studies. Error bars represent variance around each log response ratio, black lines represent linear regressions. See Table 1 for results. X intercept = −2.019°C and + 5.234°C.

Table 1. Model results for mixed model meta-analysis that assesses the effect of increasing and decreasing Δ temperature (°C) on log response ratios for coralline algae across 14 studies.
There are large gaps in the data used in this study. For example, most studies were conducted in Europe or the Pacific Ocean (see Figure 2), with no studies in the southern Atlantic Ocean, Indian Ocean, no polar research, nor any temperate studies in the southern hemisphere. There was also a disproportionate number of studies on CCA (82%) vs. articulate coralline algae (18%). Only one study examined the effects on juvenile coralline algae (Tanaka et al., 2017). However, there was a relatively good spread between depth ranges from intertidal (11% of studies) through to shallow subtidal (1–4 m: 36%), intermediate subtidal (5–8 m: 18%), and to deeper subtidal (10–35 m: 36%). There were more studies conducted during Summer (15) compared to Autumn and winter (8 each) and Spring (10).
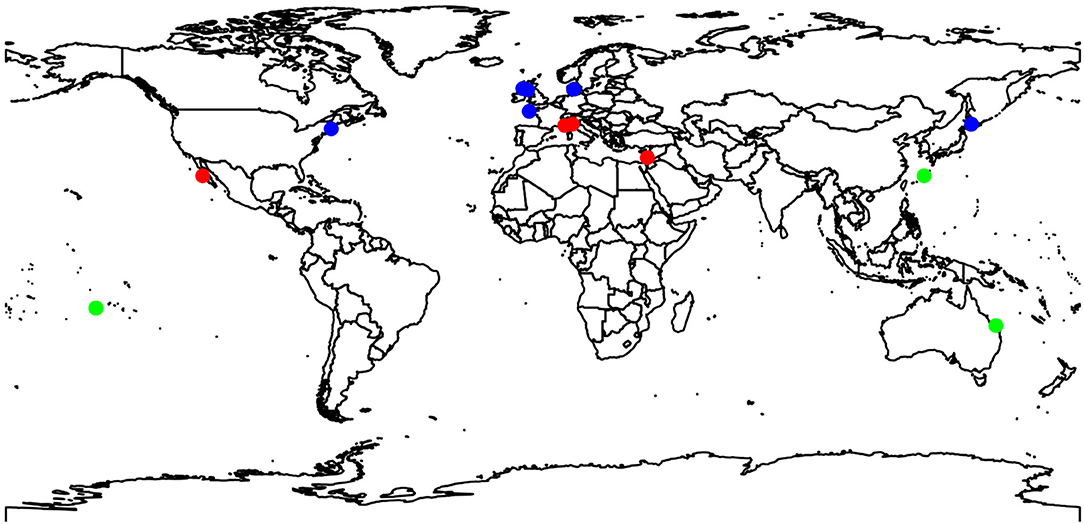
Figure 2. Map of collection sites of studies measuring responses to changing temperature assessed in Figure 1.
However, this does not mean that ocean warming will have limited impacts on all coralline algal species. Firstly, some species are evidentially more vulnerable than others (e.g., Porolithon onkodes in some studies; Tanaka et al., 2017). Secondly, there is evidence of some negative consequences of increasing temperatures that occur irrespective of impacts of calcification. For example, reproductive conceptacles of the sub-arctic Clathromorphum circumscriptum only reached maturity when temperatures where reduced, even though calcification and photosynthetic rates of this genus increases as temperature is elevated (Adey, 1973; Adey et al., 2013). Additionally, seasonal switching of articulate coralline algae from the upright (frondose) phase to the crust only has been suggested as one outcome of increasing temperatures (Guy-Haim et al., 2016). Thirdly, ocean acidification will occur in tandem with warming. The effects of acidification could exacerbate negative effects (Martin et al., 2013), or reverse (Anthony et al., 2008) any short-term benefits from elevated temperatures in some species. Though this has not been observed in all species (Comeau et al., 2016). Therefore, it must be noted that these other consequences of increasing temperature cannot be captured in meta-analyses.
Coralline Algal Bleaching
Bleaching, followed by necrosis and eventual death would be a severe consequence of ocean warming for coralline algae. Bleaching refers to the loss of photosynthetic pigments and may lead to necrosis in many cases (Martone et al., 2010). Loss of pigments leaves the skeletal material, causing the coralline algae to turn white. Macroalgal bleaching is different to coral bleaching, which generally refers to the rejection of endosymbiotic dinoflagellates by their hosts, and is likely caused by a build-up of reactive oxygen species, which results in the destruction of pigments caused by oxidative stress (Latham, 2008). Bleaching in coralline algae can also occur due to diseases (Vargas-Ángel, 2010; Quéré and Nugues, 2015). Once bleached, coralline algal calcification and, not surprisingly, photosynthetic rates drop dramatically. Coralline algae are notoriously difficult to grow for long periods of time. Therefore, bleaching even of coralline algae maintained in “control” conditions has occurred in past research attempting to determine the impacts of climate change on these taxa (Anthony et al., 2008; Martin and Gattuso, 2009; Martone et al., 2010; Diaz-Pulido et al., 2011; Cornwall et al., 2017a). However, we can make inferences regarding how the treatments may influence the survival of future populations in some instances when bleaching of controls has not occurred. Increases of 2–4°C beyond the seasonal maxima causes ~90% bleaching in the articulate Ellisolandia elongata in tanks with no water motion (Guy-Haim et al., 2016). Conversely, increasing temperature from 15 to 30°C had relatively little effect compared to that of increasing irradiance in Corallina tuberculosum (Martone et al., 2010). Martone et al. indicate this could be due to the low humidity used in their study, or the great variability in temperature encountered by intertidal C. tuberculosum in situ. As an example, tropical corals generally bleach and suffer mortality after exposure at 1–2°C above the maximum monthly mean for sustained periods of time, usually weeks or days depending on the temperature anomaly (Jokiel and Coles, 1990; Le Nohaïc et al., 2017; Hughes et al., 2018). Much further research is required to adequately assess the physiological mechanisms responsible and thresholds of coralline algal bleaching in response to elevated temperatures.
Capacity to Migrate, Acclimatize, or Adapt to Ocean Warming, or Die
The outcomes of future climate change will be dependent on the capacity of a species to adapt or acclimatize over multiple generations, rather than short-term responses measured in most laboratory research. This is particularly true for some coralline algal species that have short generation times compared to many other calcifying foundation species. Coralline algae have been reported as having negatively buoyant and non-motile spores, which would usually indicate short dispersal distances per generation (Santelices, 1990; Miklasz, 2012). However, there is likely considerable variability in dispersal capacities. For example, the time between settlement and reproduction can be extremely short: <6 weeks for Hydrolithon reinboldii, and spores of this species can be more neutrally buoyant than those reported in other species (Cornwall and Comeau, personal observation). Their capacity to disperse rapidly is also increased by their ability to colonize various substrates and raft to new localities. For example, rafting on pumice has been recorded previously (Bryan et al., 2012), and it is extremely likely that this occurs on other substrates such as kelp holdfasts and other drifting macroalgae that have large dispersal capacity (Fraser et al., 2018). Coralline algae are almost always the first colonizers to occupy new space on new substrate in many systems (Kroeker et al., 2013; Fabricius et al., 2015; Kennedy et al., 2017), demonstrating their ability to spread quickly. Their migration over large spatial scales could thus be relatively rapid. Over the time periods it will take for the effects of warming to manifest (i.e., the next few decades), it is likely that short-lived coralline algal populations could migrate from one biogeographic region to another. Therefore, some range contractions or extensions could occur rapidly, with cooler-affinity populations or species being replaced by warmer ones. Additionally, we would expect that coralline algae in tropical regions could begin to exploit new space formerly occupied by corals. This is supported by geological evidence that coralline algae were the dominant benthic calcifiers during warm geological times such as the Miocene which coincided with decreased coral abundance (Halfar and Mutti, 2005).
We also consider that it could be possible that some coralline algal populations are capable of locally adapting to changes in temperature over periods of time, while other calcifying benthic species, such as corals, will struggle to keep pace with warming. This will be particularly true if coralline algae can tolerate marine heatwave events, which is largely unknown, except for some contradictory results showing either increased or no change in mortality and no change in calcification rates after these events in Western Australia (Short et al., 2015; Cornwall et al., in preparation). In addition to factors mentioned above, their mixture of sexual and asexual reproduction gives them additional benefits. Species with both modes of reproduction have been classified as having the “best of both worlds” where asexual reproduction allows the rapid progression of mutations to build in the population, combined with periods where disadvantageous mutations are subsequently purged through sexual reproduction and recombination of alleles (Rengefors et al., 2017). It is likely coralline algal populations already possess large amounts of genotypic and phenotypic plasticity, which allows their continued survival in the vastly different microhabitats in which they settle. For example, the same populations settling in subtidal kelp forests vs. intertidal rockpools, or in the open vs. under canopies of kelp or coral, would encounter environmental conditions that vary greatly over small spatial scales (e.g., Morris and Taylor, 1983; Anthony and Hoegh-Guldberg, 2003; Cornwall et al., 2015).
Not all coralline algal species may respond similarly to warming. Those with extremely long generation times (Adey et al., 2013), and with narrow thermal ranges could have a reduced capacity to both acclimatize via transgenerational effects or adapt over multiple generations to future changes in ocean temperature (Boyd et al., 2016; Donelson et al., 2018). This is extremely likely for some populations of tropical coralline algae that specialize in living in thermally stable offshore reefs. Some species seemingly occupy large geographical ranges, and therefore encounter vastly different temperature regimes and possess different thermal tolerances (Colthart and Johansen, 1973). It is possible that species with broader thermal ranges might be able to maintain their presence in geographic regions within the lower latitudes and extend their future range into higher latitudes with increasing mean temperatures. However, more research is clearly required that assesses the thermal tolerances of a range of coralline algal species from across different biogeographical regions, supported by molecular identification to ensure that the same species is being compared (Gabrielson et al., 2018).
Future of Habitats Reliant on Coralline Algae
In coral reef ecosystems, coralline algae could opportunistically exploit space left by dead coral over the coming decades. Scleractinian corals are important competitors for space, but will be badly impacted by rising ocean temperatures and continued increased frequencies of marine heatwaves (Hughes et al., 2018). This will be exacerbated by the negative consequences of ocean acidification, which are predicted to reduce coral calcification by 15% by 2100 (Chan and Connolly, 2012). Based on this evidence, we predict a continual decline in coral cover, with increasing coralline algal cover across corals reefs, particularly those shallow reefs dominated by herbivorous fishes that could keep cyanobacterial and macroalgal turfs low in abundance (Mccook et al., 2001). However, coralline algae are particularly sensitive to ocean acidification, and its effects could cancel out any benefits of increasing bare space. If coralline algae are impacted by either ocean warming or acidification, it will likely further impact corals via reduced settlement (Webster et al., 2011; Fabricius Katharina et al., 2017). Additionally, it is unknown how coralline algae will respond to increasing frequencies of marine heatwaves, a subject outside of the bounds of this review that poses a considerable threat.
Ocean warming has been predicted to badly impact temperate and polar ecosystems occupied by coralline algae (Brodie et al., 2014). Warming could cause widespread loss of gorgonian communities in the Mediterranean (Garrabou et al., 2009), and in temperate and polar seaweed fucoid or kelp forests we will see a continued polewards drift in the extent of these brown seaweed beds and/or local extinctions (Johnson et al., 2011; Brodie et al., 2014; Martínez et al., 2018). Warm-water affinity coralline algae and those with wide geographical ranges would be expected to increase their range at the expense of cooler-affinity species. However, strong interactions with other species exist in these ecosystems, and there is limited understanding of how changes in these interactions could interplay with the direct effects of warming. For example, removal of kelp or other shade/scour-providing taxa that some coralline algal species rely on (Connell, 2003) could have larger negative impacts on coralline algae than warming itself. It would be useful if future assessments of tropicalisation (e.g., Wernberg et al., 2013, 2016) also includes assessments of range shifts and other effects on coralline algae. This will be hampered by poor knowledge of their current ranges in many parts of the world due to the difficulty of identifying many crustose coralline algae by morphology alone (Gabrielson et al., 2018). Additionally, future research should attempt to target how changing ecological interactions under warming alter coralline algal responses (Mccoy and Pfister, 2014).
How the structure of reefs and other biogenic habitats comprised of coralline algae would fare into the far future under increasing ocean warming when combined with ocean acidification poorly constrained. Dead coralline algal material comprised of high magnesium calcite is especially prone to dissolution than dead reefs made of coral aragonite (Ries, 2010). Though there are other more stable minerals such as dolomite that can occur in coralline algae, it is not present in all species, or populations (Nash et al., 2013, 2015; Diaz-Pulido et al., 2014). Therefore, dead reef material comprised of coralline algae could be more prone to dissolution that coral-dominated reefs. Not all live coralline reefs will be as vulnerable, it is becoming clear that certain coralline algal species have a greater capacity to resist changes in external seawater carbonate chemistry by modifying chemistry within the site of calcification (Cornwall et al., 2017a, 2018; Comeau et al., 2018). These responses can also be mediated by changes in irradiance, seawater nutrient concentrations, and water motion that act to either alter stress or energy levels, or to change pH at the surface of the coralline algae (Comeau et al., 2014; Cornwall et al., 2014; Johnson and Carpenter, 2018). The complex responses of coralline algae to multiple drivers, together with the variable outcomes to warming may complicate our ability to predict future coralline algal communities and reefs under ocean warming and acidification.
Recommended Protocols for Future Research
It is ideal to assess the responses of organisms to treatment conditions over biologically-relevant time frames and under realistic conditions. The robust response to elevated temperature here in some taxa was surprising, especially since many studies we examined employed rapid exposures of organism to treatment conditions without slow acclimation to treatment conditions. However, this apparently robust response could be due to the short durations that were also employed during laboratory experiments. This type of approach is appropriate for assessments of short-term physiological responses of intertidal organisms to rapid changes in temperature during different tidal periods for example, but is less representative to predictions of how subtidal organisms will fare under ocean warming, or even their responses to marine heatwaves in many instances (i.e., 5 days). Much of the research on coral responses to warming follow stricter protocols where experimental organisms are slowly acclimated to elevated temperatures over periods of weeks (see Kornder et al., 2018), rather than placing the organisms instantly into such treatments, as many of the coralline algal experiments did. Although one study we examined used biologically relevant time-scales (5 years) (Rodríguez-Prieto, 2016), no study to date has assessed responses beyond the F1 generation, which is more ecologically relevant for many coralline species that possess short generation times. Coralline algal research should begin to adopt protocols were individuals are slowly exposed to changes in temperature, experiments are conducted over long periods of time, in-depth temperature data from collection sites are given, and efforts are made to determine the capacity of temperature variability to influence responses to elevated temperatures (Table 2). Though, these guidelines present logistical challenges that could be difficult to overcome given time and financial constraints, this field of research will be significantly improved if these guidelines can be followed.
Research investigating the impacts of ocean warming on coralline algae needs to be based on relevant environmental records and conditions. Temperature ranges were usually provided as brief values in the methods or introduction in most studies we surveyed. Exposure to differing ranges in temperature could explain some disparity in the responses reviewed here (e.g., Martone et al., 2010). Temperature variability can be extreme on some shallow coralline algal reefs (e.g., 14°C daily, max 38°C, excluding marine heatwave data) (Gruber et al., 2017; Cornwall et al., 2018). Therefore, estimating ambient temperatures for many of the collection sites was difficult, and we had to usually rely on a single value provided by authors, without knowing the summer maximum monthly mean or daily variability at collections sites. In order to reconcile how marine heatwaves influence corals living in different thermal regimes, degree heating days were developed. This metric uses all positive temperature anomalies above the maximum monthly mean at the focal site (Maynard et al., 2008; Le Nohaïc et al., 2017). This value could be a useful comparative tool across studies, but appropriate temperature monitoring at collections sites are needed for it to be used.
Adequate water motion and simulating species-interactions are two other environmental factors to consider. Many coralline algal species often live in wave exposed habitats. Therefore, there is a requirement to provide adequate water motion in laboratory experiments in the future in order to measure accurate responses. For example, one study merely shook the experimental tank by hand once per day to provide water motion. This would be inadequate in providing realistic chemical conditions in the diffusion boundary layer, which could therefore affect recorded responses (Hurd et al., 2011; Cornwall et al., 2014). The presence of canopy-forming seaweed can further modify the light, pH and water velocity surrounding coralline algae (Connell, 2003; Cornwall et al., 2013, 2015), so emulating the conditions present in the field as best as possible is ideal.
Importantly, we currently do not understand how temperature variability within experimental set-ups, or the past thermal history to which organisms are exposed could influence responses. For example, Anthony et al. (2008) found no declines in calcification at 28–29°C for Porolithon onkodes under ambient mean seawater pH, whereas Tanaka et al. (2017) found sharp declines at 30°C which they attributed to the higher temperatures used in their study. However, Anthony et al. incorporated natural temperature variability and collected individuals from a naturally variable environment, whereas Tanaka et al. maintained the experimental temperature constantly at 30°C. Therefore, we cannot ascertain whether the two populations of P. onkodes would respond similarly to ocean warming in the field. Further, due to high speciation rates in the P. onkodes complex (Gabrielson et al., 2018), it is also likely that these two studies used different taxa. We also have limited understanding regarding how ocean warming will interact with other global and local stressors/drivers beyond the synergistic effects of ocean acidification. There are several past guidelines on this topic that also mirror some of our suggestions here in a more general sense (Wernberg et al., 2012; Cornwall and Hurd, 2016; Boyd et al., 2018).
Conclusions and Future Research
Our review has highlighted that some coralline algae are very likely to be both resistant and resilient (sensu Connell et al., 2016) to the impacts of ocean warming, while others are not, and may be particularly hard hit by the combination of ocean warming and acidification. Our meta-analysis would have lacked the statistical power to assess the impacts of ocean warming on any other physiological parameters. This indicates a greater need for more in-depth physiological examination of coralline algal responses to ocean warming in laboratory research, going beyond merely assessing the impacts on calcification rates. Responses of multiple generations of algae to climate change are limited to studies that have merely compared the responses of adults to that of their offspring under ocean acidification or increased pH variability (Roleda et al., 2015). Future studies assessing the impacts of warming need to consider that we need to determine the responses of multiple generations to warming due to the short generation times of many species. No research to date has assessed how changes in canopy-forming species that strongly influence coralline algal community structure could influence coralline algae indirectly under changing temperatures. There are also large gaps geographic gaps in the collection sites of coralline algae in the assessed studies, and a focus on crustose coralline algae: these need to be improved to gain greater understanding of patterns. Additionally, most reports of the biological impacts of marine heatwaves focus on charismatic species, indicating a further need for (1) specialist coralline algal biologists to take more active roles in assessing the impacts of marine heatwaves on coralline algae, and (2) for generalist scientists to gain a greater understanding of the importance of coralline algae in polar, temperate, and tropical nearshore hard-bottom ecosystems. However, there is no study to date which has assessed how this temperature variability influences the physiology of coralline algae, nor what role these habitats could play in harboring more temperature-tolerant populations of coralline algae. Further, there is little understanding of the variability in responses to thermal stress, particularly in populations along latitudinal gradients. Overall, we hope that these findings highlight an urgent need to further document and assess coralline algal responses to temperature, marine heatwaves, and future ocean warming. Furthermore, we should be looking toward improvements in current experimental practices, especially increasing experimental durations. Only once the fundamentals are achieved can we then start to focus on including multi-generational assessments of responses, research that will offer more realistic estimates regarding how coralline algal species will respond to future warming.
Author Contributions
CC and SC conceived the research. CC wrote the manuscript and analyzed the data with assistance from SC. CC and SC extracted all data from papers. All authors contributed significant ideas and discussion around these ideas. All authors edited the manuscript.
Funding
CC was supported by a Rutherford Discovery Fellowship granted by the Royal Society of New Zealand (RDF-VUW1701). SC was supported by an ARC DECRA (DE160100668). GD-P was supported by an Australian Research Council Discovery Grant (DP160103071).
Conflict of Interest Statement
The authors declare that the research was conducted in the absence of any commercial or financial relationships that could be construed as a potential conflict of interest.
The handling editor declared a shared affiliation, though no other collaboration, with several of the authors, CC and SC, at time of review.
Supplementary Material
The Supplementary Material for this article can be found online at: https://www.frontiersin.org/articles/10.3389/fmars.2019.00186/full#supplementary-material
References
Adey, W. H. (1973). Temperature control of reproduction and productivity in a subarctic coralline alga. Phycologia 12, 111–118. doi: 10.2216/i0031-8884-12-3-111.1
Adey, W. H. (1978). Algal ridges of the Caribbean sea and West Indies. Phycologia 17, 361–367. doi: 10.2216/i0031-8884-17-4-361.1
Adey, W. H., Halfar, J., and Williams, B. (2013). “Biological, phsyiological and ecological factors controlling carbonate production in an Arctic-Subarctic Climate Archive: the calcified coralline genus Clathromorphum Fosile,” in Smithsonian Institution Contributions to the Marine Sciences (Washington, DC).
Anthony, K. R. N., and Hoegh-Guldberg, O. (2003). Variation in coral photosynthesis, repsiration and growth characteristics in contrasting light microhabitats: an analogue to plants in forest gaps and understoreys? Funct. Ecol. 17, 246–259. doi: 10.1046/j.1365-2435.2003.00731.x
Anthony, K. R. N., Kline, D. I., Diaz-Pulido, G., Dove, S., and Hoegh-Guldberg, O. (2008). Ocean acidification causes bleaching and productivity loss in coral reef builders. Proc. Natl. Acad. Sci. U.S.A. 105, 17442–17446. doi: 10.1073/pnas.0804478105
Barner, A. K., Chan, F., Hettinger, A., Hacker, S. D., Marshall, K., and Menge, B. A. (2018). Generality in multispecies responses to ocean acidification revealed through multiple hypothesis testing. Glob. Change Biol. 24, 4464–4477. doi: 10.1111/gcb.14372
Boyd, P. W., Collins, S., Dupont, S., Fabricius, K., Gattuso, J.-P., Havenhand, J., et al. (2018). Experimental strategies to assess the biological ramifications of multiple drivers of global ocean change—A review. Glob. Change Biol. 24, 2239–2261. doi: 10.1111/gcb.14102
Boyd, P. W., Cornwall, C. E., Davison, A., Doney, S. C., Fourquez, M., Hurd, C. L., et al. (2016). Biological responses to environmental heterogeneity under future ocean conditions. Glob. Change Biol. 22, 2633–2650. doi: 10.1111/gcb.13287
Brodie, J., Wiiliamson, C. J., Smale, D. A., Kamenos, N. A., Mieszkowska, N., Santos, R., et al. (2014). The future of the northeast Atlantic benthic flora in a high CO2 world. Ecol. Evol. 4, 2787–2798. doi: 10.1002/ece3.1105
Bryan, S. E., Cook, A. G., Evans, J. P., Hebden, K., Hurrey, L., Colls, P., et al. (2012). Rapid, long-distance dispersal by pumice rafting. PLoS ONE 7:e40583. doi: 10.1371/journal.pone.0040583
Chan, N. C. S., and Connolly, S. R. (2012). Sensitivity of coral calcification to ocean acidification: a meta-analysis. Glob. Change Biol. 19, 282–290. doi: 10.1111/gcb.12011
Colthart, B. J., and Johansen, H. W. (1973). Growth rates of Corallina officinalis (Rhodophyta) at different temperatures. Mar. Biol. 18, 46–49. doi: 10.1007/BF00347919
Comeau, S., Carpenter, R. C., and Edmunds, P. J. (2014). Effects of irradiance on the response of the coral Acropora pulchra and the calcifying alga Hydrolithon reinboldii to temperature elevation and ocean acidification. J. Exp. Mar. Biol. Ecol. 453, 28–35. doi: 10.1016/j.jembe.2013.12.013
Comeau, S., Carpenter, R. C., Lantz, C. A., and Edmunds, P. J. (2016). Parameterization of the response of calcification to temperature and pCO2 in the coral Acropora pulchra and the alga Lithophyllum kotschyanum. Coral Reefs 35, 1–11. doi: 10.1007/s00338-016-1425-0
Comeau, S., Cornwall, C. E., Decarlo, T. M., Krieger, E., and Mcculloch, M. T. (2018). Similar controls on calcification under ocean acidification across unrelated coral reef taxa. Global Change Biol. 24, 4857–4868. doi: 10.1111/gcb.14379
Connell, S. D. (2003). The monopolization of understory habitat by subtidal encrusting coralline algae: a test of the combined effects of canopy-mediated light and sedimentation. Mar. Biol. 142, 1065–1071. doi: 10.1007/s00227-003-1021-z
Connell, S. D., Nimmo, D. G., Ghedini, G., Mac Nally, R., and Bennett, A. F. (2016). Ecological resistance - why mechanisms matter: a reply to sundstrom et al. Trends Ecol. Evol. 31, 413–414. doi: 10.1016/j.tree.2016.03.015
Cornwall, C. E., Boyd, P. W., Mcgraw, C. M., Hepburn, C. D., Pilditch, C. A., Morris, J. N., et al. (2014). Diffusion boundary layers ameliorate the negative effects of ocean acidification on the temperate coralline macroalga Arthrocardia corymbosa. PLoS ONE 9:e97235. doi: 10.1371/journal.pone.0097235
Cornwall, C. E., Comeau, S., Decarlo, T. M., Moore, B., D'alexis, Q., and Mcculloch, M. T. (2018). Resistance of corals and coralline algae to ocean acidification: physiological control of calcification under natural pH variability. Proc. R. Soc. Biol. Sci. 285:2018116. doi: 10.1098/rspb.2018.1168
Cornwall, C. E., Comeau, S., and Mcculloch, M. T. (2017a). Coralline algae elevate pH at the site of calcification under ocean acidification. Glob. Change Biol. 23, 4245–4256. doi: 10.1111/gcb.13673
Cornwall, C. E., Hepburn, C. D., Mcgraw, C. M., Currie, K. I., Pilditch, C. A., Hunter, K. A., et al. (2013). Diurnal fluctuations in seawater pH influence the response of a calcifying macroalga to ocean acidification. Proc. R. Soc. B. Biol. Sci. 280:20132201. doi: 10.1098/rspb.2013.2201
Cornwall, C. E., and Hurd, C. L. (2016). Experimental design in ocean acidification research: problems and solutions. ICES J. Mar. Sci. 73, 572–581. doi: 10.1093/icesjms/fsv118
Cornwall, C. E., Pilditch, C. A., Hepburn, C. D., and Hurd, C. L. (2015). Canopy macroalgae influence understorey corallines' metabolic control of near-surface pH and oxygen concentration. Mar. Ecol. Prog. Ser. 525, 81–95. doi: 10.3354/meps11190
Cornwall, C. E., Revill, A. T., Hall-Spencer, J. M., Milazzo, M., Raven, J. A., and Hurd, C. L. (2017b). Inorganic carbon physiology underpins macroalgal responses to elevated CO2. Sci. Rep. 7:46297. doi: 10.1038/srep46297
Diaz-Pulido, G., Anthony, K. R. N., Kline, D. I., Dove, S., and Hoegh-Guldberg, O. (2011). Interactions between ocean acidification and warming on the mortality and dissolution of coralline algae. J. Phycol. 48, 32–39. doi: 10.1111/j.1529-8817.2011.01084.x
Diaz-Pulido, G., Nash, M. C., Anthony, K. R. N., Bender, D., Opdyke, B. N., Reyes-Nivia, C., et al. (2014). Greenhouse conditions induce mineralogical changes and dolomite accumulation in coralline algae on tropical reefs. Nat. Commun. 5:3310. doi: 10.1038/ncomms4310
Donelson, J. M., Salinas, S., Munday, P. L., and Shama, L. N. S. (2018). Transgenerational plasticity and climate change experiments: where do we go from here? Glob. Change Biol. 24, 13–34. doi: 10.1111/gcb.13903
Fabricius Katharina, E., Noonan Sam, H. C., Abrego, D., Harrington, L., and De'ath, G. (2017). Low recruitment due to altered settlement substrata as primary constraint for coral communities under ocean acidification. Proc. R. Soc. B. Biol. Sci. 284:20171536. doi: 10.1098/rspb.2017.1536
Fabricius, K., Kluibenschedl, A., Harrington, L., Noonan, S., and De'ath, G. (2015). In situ changes of tropical crustose coralline algae along carbon dioxide gradients. Sci. Rep. 5:9537. doi: 10.1038/srep09537
Fabricius, K., Langdon, C., Uthicke, S., Humphrey, C., Noonan, S., De'ath, G., et al. (2011). Losers and winners in coral reefs acclimatized to elevated carbon dioxide concentrations. Nat. Clim. Change 1, 165–169. doi: 10.1038/nclimate1122
Foster, M. S. (2001). Rhodoliths: between rocks and soft places. J. Phycol. 37, 659–667. doi: 10.1046/j.1529-8817.2001.00195.x
Fraser, C. I., Morrison, A. K., Hogg, A. M., Macaya, E. C., Van Sebille, E., Ryan, P. G., et al. (2018). Antarctica's ecological isolation will be broken by storm-driven dispersal and warming. Nat. Clim. Change 8, 704–708. doi: 10.1038/s41558-018-0209-7
Gabrielson, P. W., Hughey, J. R., and Diaz-Pulido, G. (2018). Genomics reveals abundant speciation in the coral reef building alga Porolithon onkodes (Corallinales, Rhodophyta). J. Phycol. 54, 429–434. doi: 10.1111/jpy.12761
Garrabou, J., Coma, R., Bensoussan, N., Bally, M., Chevaldonné, P., Cigliano, M., et al. (2009). Mass mortality in Northwestern Mediterranean rocky benthic communities: effects of the 2003 heat wave. Glob. Change Biol. 15, 1090–1103. doi: 10.1111/j.1365-2486.2008.01823.x
Gattuso, J. P., Magnan, A., Billé, R., Cheung, W. W. L., Howes, E. L., Joos, F., et al. (2015). Contrasting futures for ocean and society from different anthropogenic CO2 emissions scenarios. Science 349:aac4722. doi: 10.1126/science.aac4722
Gruber, R. K., Lowe, R. J., and Falter, J. L. (2017). Metabolism of a tide-dominated reef platform subject to extreme diel temperature and oxygen variations. Limnol. Oceanogr. 62, 1701–1717. doi: 10.1002/lno.10527
Guy-Haim, T., Silverman, J., Raddatz, S., Wahl, M., Israel, A., and Rilov, G. (2016). The carbon turnover response to thermal stress of a dominant coralline alga on the fast warming Levant coast. Limnol. Oceanogr. 61, 1120–1133. doi: 10.1002/lno.10279
Halfar, J., and Mutti, M. (2005). Global dominance of coralline red-algal facies: a response to Miocene oceanographic events. Geology 33, 481–484. doi: 10.1130/G21462.1
Hall-Spencer, J. M., Rodolfo-Metalpa, R., Martin, S., Ransome, E., Fine, M., Turner, S. M., et al. (2008). Volcanic carbon dioxide vents show ecosystem effects of ocean acidification. Nature 454, 96–99. doi: 10.1038/nature07051
Hedges, L. V., Gurevitch, J., and Curtis, P. S. (1999). The meta-analysis of response ratios in experimental ecology. Ecology 80, 1150–1156. doi: 10.1890/0012-9658(1999)080[1150:TMAORR]2.0.CO;2
Heyward, A., and Negri, A. (1999). Natural inducers for coral larval metamorphosis. Coral Reefs 18, 273–279. doi: 10.1007/s003380050193
Heyward, A. J., and Moore, C. (2009). Benthic Habitat Characterisation of Montgomery Reef: Assessing the Distribution and Relative Abudnance of Dominant Benthic Communities. Report for AIMS.
Hofmann, L. C., and Bischof, K. (2014). Ocean acidification effects on calcifying macroalgae. Aquat. Biol. 22, 261–279. doi: 10.3354/ab00581
Hughes, T. P., Anderson, K. D., Connolly, S. R., Heron, S. F., Kerry, J. T., Lough, J. M., et al. (2018). Spatial and temporal patterns of mass bleaching of corals in the Anthropocene. Science 359, 80–83. doi: 10.1126/science.aan8048
Hughes, T. P., Barnes, M. L., Bellwood, D. R., Cinner, J. E., Cumming, G. S., Jackson, J. B. C., et al. (2017). Coral reefs in the Anthropocene. Nature 546:82. doi: 10.1038/nature22901
Hurd, C. L., Cornwall, C. E., Currie, K. I., Hepburn, C. D., Mcgraw, C. M., Hunter, K. A., et al. (2011). Metabolically-induced pH fluctuations by some coastal calcifiers exceed projected 22nd century ocean acidification: a mechanism for differential susceptibility? Glob. Change Biol. 17, 3254–3262. doi: 10.1111/j.1365-2486.2011.02473.x
Hurd, C. L., Hepburn, C. D., Currie, K. I., Raven, J. A., and Hunter, K. A. (2009). Testing methods of ocean acidification on algal metabolism: consideration for experimental designs. J. Phycol. 45, 1236–1251. doi: 10.1111/j.1529-8817.2009.00768.x
Johnson, C. R., Banks, S. C., Barrett, N. S., Cazassus, F., Dunstan, P. K., Edgar, G. J., et al. (2011). Climate change cascades: shifts in oceanography, species' ranges and subtidal marine community dynamics in eastern Tasmania. J. Exp. Mar. Biol. Ecol. 400, 17–32. doi: 10.1016/j.jembe.2011.02.032
Johnson, M. D., and Carpenter, R. C. (2018). Nitrogen enrichment offsets direct negative effects of ocean acidification on a reef-building crustose coralline alga. Biol. Lett. 14:20180371. doi: 10.1098/rsbl.2018.0371
Jokiel, P. L., and Coles, S. L. (1990). Response of Hawaiian and other Indo-Pacific reef corals to elevated temperature. Coral Reefs 8, 155–162. doi: 10.1007/BF00265006
Kennedy, E. V., Ordoñez, A., Lewis, B. E., and Diaz-Pulido, G. (2017). Comparison of recruitment tile materials for monitoring coralline algae responses to a changing climate. Mar. Ecol. Prog. Ser. 569, 129–144. doi: 10.3354/meps12076
Kornder, N. A., Riegl, B. M., and Figueiredo, J. (2018). Thresholds and drivers of coral calcification responses to climate change. Glob. Change Biol. 24, 5084–5095. doi: 10.1111/gcb.14431
Kroeker, K. J., Micheli, F., and Gambi, M. C. (2013). Ocean acidification causes ecosystem shifts via altered competitive interactions. Nat. Clim. Change 3, 156–159. doi: 10.1038/nclimate1680
Krumhansl, K. A., Okamoto, D. K., Rassweiler, A., Novak, M., Bolton, J. J., Cavanaugh, K. C., et al. (2016). Global patterns of kelp forest change over the past half-century. Proc. Natl. Acad. Sci. U.S.A. 113, 13785–13790. doi: 10.1073/pnas.1606102113
Latham, H. (2008). Temperature stress-induced bleaching of the coralline alga Corallina officinalis: a role for the enzyme bromoperoxidase. Biosci. Horizons 1, 104–113. doi: 10.1093/biohorizons/hzn016
Le Nohaïc, M., Ross, C. L., Cornwall, C. E., Comeau, S., Lowe, R., Mcculloch, M. T., et al. (2017). Marine heatwave causes unprecedented regional mass bleaching of thermally resistant corals in northwestern Australia. Sci. Rep. 7:14999. doi: 10.1038/s41598-017-14794-y
Martin, S., Cohu, S., Vignot, C., Zimmerman, G., and Gattuso, J. P. (2013). One-year experiment on the physiological response of the Mediterranean crustose coralline alga, Lithophyllum cabiochae, to elevated pCO2 and temperature. Ecol. Evol. 3, 676–693. doi: 10.1002/ece3.475
Martin, S., and Gattuso, J. P. (2009). Response of Mediterranean coralline algae to ocean acidification and elevated temperature. Glob. Change Biol. 15, 2089–2100. doi: 10.1111/j.1365-2486.2009.01874.x
Martínez, B., Radford, B., Thomsen, M. S., Connell, S. D., Carreño, F., Bradshaw, C. J. A., et al. (2018). Distribution models predict large contractions of habitat-forming seaweeds in response to ocean warming. Diver. Distrib. 24, 1350–1366. doi: 10.1111/ddi.12767
Martone, P. T., Alyono, M., and Stites, S. (2010). Bleaching of an intertidal coralline alga: untangling the effects of light, temperature, and desiccation. Mar. Ecol. Prog. Ser. 416, 57–67. doi: 10.3354/meps08782
Maynard, J. A., Turner, P. J., Anthony, K. R. N., Baird, A. H., Berkelmans, R., Eakin, C. M., et al. (2008). ReefTemp: an interactive monitoring system for coral bleaching using high-resolution SST and improved stress predictors. Geophys. Res. Lett. 35:5. doi: 10.1029/2007GL032175
Mccook, L. J., Jompa, J., and Diaz-Pulido, G. (2001). Competition between corals and algae on coral reefs: a review of evidence and mechanisms. Coral Reefs 19, 400–417. doi: 10.1007/s003380000129
Mccoy, S. J., and Kamenos, N. A. (2015). Coralline algae (Rhodophyta) in a changing world: intergrating ecological, physiological, and geochemical responses to global change. J. Phycol. 51, 6–24. doi: 10.1111/jpy.12262
Mccoy, S. J., and Pfister, C. A. (2014). Historical comparisons reveal altered competitive interactions in a guild of crustose coralline algae. Ecol. Lett. 17, 475–483. doi: 10.1111/ele.12247
Mcculloch, M. T., Falter, J., Trotter, J., and Montagna, P. (2012). Coral resilience to ocean acidification and global warming through pH up-regulation. Nat. Clim. Change 2, 623–627. doi: 10.1038/nclimate1473
Miklasz, K. A. (2012). Physical Contrainsts on the Size and Shape on Microalgae. Ph.D. Stanford University.
Morris, S., and Taylor, A. C. (1983). Diurnal and seasonal variation in physico-chemical conditions within intertidal rock pools. Estuar. Coast. Shelf Sci. 17, 339–355. doi: 10.1016/0272-7714(83)90026-4
Nash, M., Uthicke, S., Negri, A., and Cantin, N. (2015). Ocean acidification does not affect magnesium composition or dolomite formation in living crustose coralline algae, Porolithon onkodes in an experimental system. Biogeosciences 12, 5247–5260. doi: 10.5194/bg-12-5247-2015
Nash, M. C., Opdyke, N. N., Troitzsch, U., Russell, B. D., Adey, W. H., Kato, A., et al. (2013). Dolomite-rich coralline algae in reefs resist dissoltuion in acidified conditions. Nat. Clim. Change 3, 268–272. doi: 10.1038/nclimate1760
Nelson, W. A. (2009). Calcified macroalgae—critical to coastal ecosystems and vulnerable to change: a review. Mar. Freshw. Res. 60, 787–801. doi: 10.1071/MF08335
Oliver, E. C. J., Donat, M. G., Burrows, M. T., Moore, P. J., Smale, D. A., Alexander, L. V., et al. (2018). Longer and more frequent marine heatwaves over the past century. Nat. Commun. 9:1324. doi: 10.1038/s41467-018-03732-9
Ordoñez, A., Doropoulos, C., and Diaz-Pulido, G. (2014). Effects of ocean acidification on population dynamics and community structure of crustose coralline algae. Biol. Bull. 226, 255–268. doi: 10.1086/BBLv226n3p255
Pearce, C. M., and Scheibling, R. E. (1991). Effect of macroalgae, microbial films, and conspecifics on the induction of metamorphosis of the green sea urchin Strongylocentrotus droebachiensis (Müller). J. Exp. Mar. Biol. Ecol. 147, 147–162. doi: 10.1016/0022-0981(91)90179-Z
Pörtner, H. O. (2012). Integrating climate-related stressor effects on marine organisms: unifying principles linking molecule to ecosystem-level changes. Mar. Ecol. Prog. Ser. 470, 273–290. doi: 10.3354/meps10123
Quéré, G., and Nugues, M. M. (2015). Coralline algae disease reduces survival and settlement success of coral planulae in laboratory experiments. Coral Reefs 34, 863–870. doi: 10.1007/s00338-015-1292-0
Rengefors, K., Kremp, A., Reusch, T. B. H., and Wood, M. (2017). Genetic diversity and evolution in eukaryotic phytoplankton: revelations from population genetic studies. J. Plankton Res. 39, 165–179. doi: 10.1093/plankt/fbw098
Riebesell, U., and Gattuso, J.-P. (2015). Lessons learned from ocean acidification research. Nat. Clim. Change 5, 12–14. doi: 10.1038/nclimate2456
Ries, J. B. (2010). Geological and experimental evidence for secular variation in seawater Mg/Ca (calcite-aragonite seas) and its effects on marine biological calcification. Biogeosciences 7, 2795–2849. doi: 10.5194/bg-7-2795-2010
Roberts, R. D. (2001). A review of settlement cues for larval abalone (Haliotis spp.). J. Shell. Res. 20, 571–586.
Rodríguez-Prieto, C. (2016). Light and temperature requirements for survival, growth and reproduction of the crustose coralline Lithophyllum stictaeforme from the Mediterranean Sea. Bot. Mar. 59, 95–104. doi: 10.1515/bot-2015-0070
Roleda, M. Y., Cornwall, C. E., Feng, Y., Mcgraw, C. M., Smith, A. M., and Hurd, C. L. (2015). Effect of ocean acidification and pH fluctuations on the growth and development of coralline algal recruits, and an associated benthic algal assemblage. PLoS ONE 10:e0140394. doi: 10.1371/journal.pone.0140394
Roleda, M. Y., and Hurd, C. L. (2012). “Seaweed responses to ocean acidification,” in Seaweed Biology, eds. C. Wiencke and K. Bischof (Heidelberg: Springer), 407–431.
Santelices, B. (1990). Patterns of reproduction, dispersal and recruitment in seaweeds. Oceanogr. Mar. Biol. Annu. Rev. 28, 177–276.
Short, J., Foster, T., Falter, J., Kendrick, G. A., and Mcculloch, M. T. (2015). Crustose coralline algal growth, calcification and mortality following a marine heatwave in Western Australia. Cont. Shelf Res. 106, 38–44. doi: 10.1016/j.csr.2015.07.003
Steneck, R. S. (1986). The ecology of coralline algal crusts: convergent patterns and adaptive strategies. Annu. Rev. Ecol. Syst. 17, 273–303. doi: 10.1146/annurev.es.17.110186.001421
Tanaka, Y., Suzuki, A., and Sakai, K. (2017). Effects of elevated seawater temperature and phosphate enrichment on the crustose coralline alga Porolithon onkodes (Rhodophyta). Phycol. Res. 65, 51–57. doi: 10.1111/pre.12152
Tebben, J., Motti, C. A., Siboni, N., Tapiolas, D. M., Negri, A. P., Schupp, P. J., et al. (2015). Chemical mediation of coral larval settlement by crustose coralline algae. Sci. Rep. 5:10803. doi: 10.1038/srep10803
Vargas-Ángel, B. (2010). Crustose coralline algal diseases in the U.S.-Affiliated Pacific Islands. Coral Reefs 29, 943–956. doi: 10.1007/s00338-010-0646-x
Vergés, A., Doropoulos, C., Malcolm, H. A., Skye, M., Garcia-Piz,á, M., Marzinelli, E. M., et al. (2016). Long-term empirical evidence of ocean warming leading to tropicalization of fish communities, increased herbivory, and loss of kelp. Proc. Natl. Acad. Sci. U.S.A. 113, 13791–13796. doi: 10.1073/pnas.1610725113
Webster, N. S., Soo, R., Cobb, R., and Negri, A. P. (2011). Elevated seawater temperature causes a microbial shift on crustose coralline algae with implications for the recruitment of coral larvae. ISME J. 5, 759–770. doi: 10.1038/ismej.2010.152
Wernberg, T., Bennett, S., Babcock, R. C., De Bettignies, T., Cure, K., Depczynski, M., et al. (2016). Climate-driven regime shift of a temperate marine ecosystem. Science 353, 169–172. doi: 10.1126/science.aad8745
Wernberg, T., Smale, D. A., and Thomsen, M. S. (2012). A decade of climate change experiments on marine organisms: procedures, patterns and problems. Glob. Change Biol. 18, 1491–1498. doi: 10.1111/j.1365-2486.2012.02656.x
Keywords: ocean warming, coralline algae, meta-analysis, guidelines, kelp forests, coral reefs, coralligenous
Citation: Cornwall CE, Diaz-Pulido G and Comeau S (2019) Impacts of Ocean Warming on Coralline Algal Calcification: Meta-Analysis, Knowledge Gaps, and Key Recommendations for Future Research. Front. Mar. Sci. 6:186. doi: 10.3389/fmars.2019.00186
Received: 04 October 2018; Accepted: 25 March 2019;
Published: 06 May 2019.
Edited by:
Thomas Wernberg, University of Western Australia, AustraliaReviewed by:
Sophie J. McCoy, Florida State University, United StatesJacqueline L. Padilla-Gamino, University of Washington, United States
Copyright © 2019 Cornwall, Diaz-Pulido and Comeau. This is an open-access article distributed under the terms of the Creative Commons Attribution License (CC BY). The use, distribution or reproduction in other forums is permitted, provided the original author(s) and the copyright owner(s) are credited and that the original publication in this journal is cited, in accordance with accepted academic practice. No use, distribution or reproduction is permitted which does not comply with these terms.
*Correspondence: Christopher E. Cornwall, Y2hyaXN0b3BoZXIuY29ybndhbGxAdnV3LmFjLm56