- Department of Earth System Science, University of California, Irvine, Irvine, CA, United States
The cupric ion (Cu2+) plays a dual role as both nutrient and toxicant to freshwater and marine phytoplankton, functioning in multiple photochemical processes, as well as reactive oxygen species (ROS) production. This duality has been investigated through a variety of methods to determine the consequences of natural and anthropogenic copper introduction to algal ecosystem composition. Studies conducted over the past few decades have described the growth responses of many unique organisms to copper availability. Such observations are critical for describing the global distributions of major phytoplankton species in terms of global trace metal abundance; however, highly variable experimental practices impede direct inter-study comparison. The aim of this systematic review is to summarize the available data regarding the effects of copper concentration on diverse marine phytoplankton growth rates. Through extensive literary comparison, 143 studies were systematically reviewed, and data on copper concentrations and growth rates reported were extracted. From the data available, we conclude that trends in phytoplankton sensitivity to copper are mainly driven by a single study. We discuss the obstacles to inter-study comparison and detail both the concurring and conflicting results to date, with an emphasis on taxonomic trends and methodologies employed. Finally, we present the first copper sensitivity measurements for marine unicellular diazotrophs using three representative strains of the unicellular nitrogen-fixing cyanobacterium, Crocosphaera watsonii.
Introduction
The toxicity of copper (Cu) to algal growth was widely recognized and utilized to prevent nuisance algal blooms even before the twentieth century. Moore and Kellerman (1904) reviewed the application of copper sulfate as an algicide and attempted to prescribe optimum application protocols that would avoid harmful impacts on fish populations. This practice had apparent success, but the effectiveness was inconsistent (Maloney and Palmer, 1956). Subsequent work revealed that organic and synthetic chelating agents could modulate the toxicity of Cu to phytoplankton (Morgan and Lackey, 1958; Fitzgerald and Faust, 1963), and the dependence of nutritive and toxic responses on free cupric ion molarity ([Cu2+]), which could be systematically altered by chelating agent addition, was later demonstrated (Manahan and Smith, 1973; Sunda, 1976).
Since then, the role of Cu in both limitation and toxicity of photosynthetic microalgae has been investigated from myriad perspectives. In the following 3 sections, we briefly review the biogeochemical cycling of Cu as well as its physiological relevance. We then preface our distillation of the surveyed literature with a brief discussion of the obstacles encountered when attempting to compare 50 years of publications on a common scale. We emphasize that roughly half the studies assessed reported total Cu content in natural seawater media, which, without added chelator or analytic measurement, cannot offer insight into the relationship between ionic Cu and marine phytoplankton growth.
Copper Sources, Sinks, and Cycling
The effect of Cu on marine biota largely depends on the amount of free copper (Cu2+) the receiving waters accumulate. This is determined by the flux of total Cu, its relative solubility, and the concentration of Cu-binding ligands. Of the major natural Cu sources to the ocean, wind-blown mineral dust is the largest single component (Nriagu, 1979, 1989), providing seasonal micronutrient pulses to regions often limited by such resources (Duce et al., 1991). These fluxes are geographically confined, with the largest accumulation observed in regions directly under the main transport pathway (Prospero, 1999). Emissions resulting from natural and prescribed fires rank second among non-anthropogenic Cu aerosol production, and they are largely dependent on location, with some biomes showing negligible Cu release (Sanhueza, 1996) and others being major regional sources (Maenhaut et al., 1996).
Nearly 10 times greater than natural sources (Hong et al., 1996), anthropogenic emissions of Cu are dominated by fossil fuel combustion (Nriagu, 1989) and automotive brake wear. Approximately 50% of particulates generated from the forced deceleration of automotive vehicles is released as aerosol (Sanders et al., 2003; Schauer et al., 2006). As a continent, the total contribution of brake wear to western European atmospheric Cu emissions is roughly 50% (Denier van der Gon et al., 2007). In certain areas of the ocean, such as the western North Pacific and western North Atlantic, Cu deposition has increased more than four-fold in the present day compared to the pre-industrial era (Paytan et al., 2009).
Of the total Cu that is deposited onto the ocean surface, only a fraction is soluble (entering the dissolved pool), with solubility in natural vs. anthropogenic aerosols differing by orders of magnitude. Sholkovitz et al. (2010) reported the fractional solubility of Cu in the Sargasso Sea to be below 8% in Saharan dust, yet as high as 100% in anthropogenic aerosols. As such, it is possible that Cu concentrations toxic to marine phytoplankton are confined to areas impacted by heavy anthropogenic emissions (Sholkovitz et al., 2012). Despite numerous studies regarding trace metal content of aerosols sampled locally (Duce et al., 1991; Artaxo et al., 1999; Chen et al., 2008; Trapp et al., 2010), there are few measurements of actual deposition rates. It is, therefore, likely that large-scale characterization of Cu distributions will be driven by in situ measurements of dissolved concentrations, as is the goal of the GEOTRACES campaign (http://www.geotraces.org/), which aims to quantify “the supply, removal, internal cycling, chemical form and distribution of essential micronutrients” at a global scale.
The spatial resolution of the GEOTRACES transects allows unprecedented potential to understand regional Cu toxicity in terms of its chemical speciation. Of fundamental importance to this understanding is that Cu-induced growth inhibition and/or limitation is a function of [Cu2+], rather than total dissolved Cu concentration (Sunda, 1976). This parameter is typically reported as pCu, the negative logarithm of the free cupric ion activity. It has long been recognized that the presence of synthetic chelators, such as ethylene diamine tetra acetic acid (EDTA) and nitrilotriacetic acid (NTA), generally improves phytoplankton growth by buffering the free aquated ion concentration (Erickson et al., 1970; Sunda, 1976). Similarly, bioavailability of Cu in situ is controlled by organic ligands (Bruland et al., 1991). In many regions, up to 99% of total Cu is complexed by organic ligands well beyond the mixed layer (Coale and Bruland, 1988, 1990; Blake et al., 2004; Shank et al., 2004; Moffett and Dupont, 2007; Buck et al., 2012; Stockdale et al., 2015). These compounds act as a natural trace metal buffering system; where ligand concentration exceeds total Cu concentration, the system avoids potentially toxic conditions, and where ligand concentration is exceeded by Cu concentration, the system can approach the [Cu2+] toxicity thresholds of certain organisms (Brand et al., 1986; Buck et al., 2012). Relatively little is known about the chemical structure of organic ligands in the marine environment (Schwarzenbach et al., 2003). The key parameter in describing complexation of metals with these ligands, however, is well studied. Stability constants are thermodynamically unique measures of the interaction strength between ion and ligand. Although organic ligands likely exist as a continuum of stability constants, they are partitioned into class 1 and class 2 pools, with class 1 being a generally smaller pool of strong ligands, and class 2 being a much larger and weaker group. Consequently, small increases in total dissolved Cu can affect [Cu2+] strongly when speciation is dominated by the class 1 pool (Moffett, 1995). It is most important, however, whether total dissolved Cu exceeds ligand concentrations or not. The production of organic substances characteristic of both ligand pools is observed in several organisms, with chelation strength being correlated to the Cu sensitivity exhibited by respective species (Moffett et al., 1997; Croot et al., 2000). Because the only known sink for these ligands is photochemical degradation occurring over days, the “buffering capacity” of organic ligands to prevent rises in [Cu2+] may be altered by changes in vertical mixing (Moffett et al., 1990).
Ecological and Physiological Relevance of Copper
Cu2+ is fundamental in biochemical pathways in marine phytoplankton. In denitrifying prokaryotes, Cu is essential to the nitrous-oxide reductase enzyme, which is responsible for the conversion of nitrous oxide to dinitrogen gas—the completion of the nitrogen cycle (Rasmussen et al., 2000). In Cu nitrite reductase, which catalyzes the reduction of nitrite to nitric oxide, Cu serves as the necessary cofactor; this is in contrast to the iron (Fe) assimilatory nitrite reductase that converts nitrite to ammonium (Li et al., 2015). Cu is also present in amine oxidases, functioning in organic nitrogen assimilation, and in ferroxidases, functioning in Fe acquisition (Palenik et al., 1989; La Fontaine et al., 2002; Maldonado et al., 2006). More generally, Cu facilitates electron transport in both NADH dehydrogenase and the widely-encoded plastocyanin, which replaces cytochrome c in some organisms experiencing Fe-limitation and completes the transfer of electrons to the terminal acceptor (Ho and Krogmann, 1984; De la Rosa et al., 2002). Cu itself serves to mitigate oxidative stress through Cu/Zn superoxide dismutase (SOD) (Chadd et al., 1996), and Cu occupies both centers of the electron transport chain's terminal enzyme, cytochrome c oxidase, one of which constitutes the site of oxygen reduction and helps in establishing the transmembrane proton motive force responsible for ATP synthesis (Osman and Cavet, 2008; Huertas et al., 2014).
The ability of Cu to cycle between oxidation states makes it an ideal cofactor in redox processes; however, it also allows Cu to catalyze the production of reactive oxygen species through the metal catalyzed Haber-Weiss reaction (below; Haber and Weiss, 1934; Halliwell and Gutteridge, 1984).
The resulting production of hydroxl radicals leads to severe lipid, protein, and DNA damage (Kehrer, 2000). Additionally, the Irving-Williams series predicts an alternate route of Cu toxicity attributed to the high capacity of Cu to bind organic compounds, meaning that Cu can displace other metals from their associated binding sites within metalloproteins (Macomber and Imlay, 2009; Chillappagari et al., 2010; Tottey et al., 2012). Replacement of Fe by Cu in Fe-S clusters of essential enzymes was recently proposed to be the main form of Cu toxicity in the freshwater Synechocystis sp. PCC 6803 (Giner-Lamia et al., 2014). Various methods of Cu detoxification/tolerance are documented and include: changes in cell fluidity (Rocha et al., 2016), changes in cell size (Erickson, 1972), deliberate cellular accumulation of competing metals (Hong-Hermesdorf et al., 2014), production of intra- and extracellular metal chelators (Gekeler et al., 1988; Lombardi and Vieira, 2000; Pistocchi et al., 2000), and SOD upregulation (Liping and Binghui, 2008).
Challenges in Cross-Study Comparison
The heterogeneity of natural and even artificial seawater is such that pCu is the only common scale on which to compare observations. We encountered several barriers to direct inter-study comparison mostly regarding insufficient information to calculate pCu. A recent review by Leal et al. (2016) concisely summarized methodological discrepancies confounding cross-study comparison of Cu ecotoxicological studies. These include: labware used, labware cleaning protocols, stock solution preparation, reported Cu concentrations, and analytical techniques for determination of dissolved Cu. Maienthal and Becker (1976) detailed the potential for error arising from such inconsistencies. The desired concentrations of trace metals in Cu ecotoxicology studies are typically quite low, and the impurities present in chemical reagents used to prepare culturing media can easily introduce substantial contamination (Morel et al., 1979). Likewise, natural seawater contains unknown concentrations of trace metals and dissolved organic matter (Armstrong et al., 1966).
When these issues are not accounted for in experimental design, it is not possible to compute the speciation of Cu, and there is virtually no capacity for inter-study comparison. Because of this, a seemingly rich literature of Cu ecotoxicology studies spanning 50+ years is largely reduced to anecdotal observation which, although valuable, cannot improve our quantitative understanding on the role of Cu2+ limitation/toxicity in microalgae. Below we describe the methods used to classify Cu toxicity studies for the inter-comparison. When we discuss replicability, we refer to the ability to confidently recreate the experimental conditions under which a set of observations was collected.
Methods
Search Strategy
This section details our compilation of the published observations on marine phytoplankton growth rates as a function of Cu2+ availability. Studies reporting on Cu inhibition of phytoplankton growth (until March 2018) were searched in Google Scholar. The keyword criteria used in the search for peer reviewed literature were titles containing the word “copper” and at least one of: “phytoplankton,” “microalgae,” “photosynthesis,” or “fixation.” This initial search returned 671 results, many of which were regarding terrestrial vegetation. Additionally, the citations appearing on google scholar for two seminal publications in the field of Cu ecotoxicology were searched. These are (1) the dissertation of William Sunda (1976), which first demonstrated a systematic decline in the growth rate of marine phytoplankton with increasing [Cu2+] and has been cited by 1,070 publications, and (2) Brand et al. (1986), which remains the largest single demonstration of phytoplankton growth as a function of [Cu2+] and has been cited by 502 publications. References from relevant publications were also hand searched. The criterion for inclusion of articles in this review was that the study performed some type of Cu toxicity dose-response assay on cultured or field phytoplankton populations with regard to cellular division rates. The total pool of published literature was then partitioned into two categories: studies reporting either calculated or analytically measured Cu speciation, and those which only reported total nominal Cu additions (Figure 1). Those studies in the latter category were then split into two additional pools based on whether the described methodologies permitted post hoc calculation of pCu. For those 99 studies which could not be converted to pCu units, the major qualitative results are presented in Supplementary Table 1, along with the reason for exclusion. Otherwise, the chemical speciation of the growth medium used in a given study was determined using the chemical equilibrium modeling software MINEQL+ version 5.0 (Schecher and McAvoy, 1992) as described below.
Calculation of pCu in MINEQL+
MINEQL+ is a widely used chemical equilibrium modeling program in microalgal studies where aqueous speciation is controlled. The details of the program can be found in Morel and Herring (1993) and Schecher and McAvoy (1992). Of 44 surveyed studies: 32% used MINEQL+ to calculate pCu, 20% calculated pCu manually or using some allometric equation, 43% required post hoc calculation (discussed below), and 5% measured pCu analytically. Because any calculation of pCu is dependent on input data for reaction equilibria and stability constants, it is uncertain how comparable reported pCu values are, as these inputs were not typically available. Additionally, it is at the discretion of the experimenter to prescribe the system: pH, pCO2, precipitation allowances, ionic strength, and temperature. We note that of the publications reviewed here, these parameters are unspecified in either the main or supplementary texts for nearly all studies reporting calculated pCu.
As stated earlier, our literature search was first separated into studies reporting results in terms of Cu2+ and those reporting results in terms of total Cu. Of the later, [Cu2+] was calculated in MINEQL+ by inputting the reported media compositions using consistent assumptions as indicated in Table 1. Activity was estimated as in Sunda (1976).
For each study, total component concentrations were inputted along with ionic strength (typically constant at 0.7 M for seawater-type media), pH, and temperature at which cells were grown. Because of the typically high concentrations of EDTA employed and the short timescale of toxicity experiments, precipitation of dissolved solids was not allowed (“not considered”).
Experimental Design for Cu2+ Toxicity Assay Using C. Watsonii
C. Watsonii strains WH0003, WH0005, and WH0402 were maintained in a modified version of the artificial seawater culture medium, AQUIL (Price et al., 1989), with alterations described below (Table 2). Fe and Cu were added as a pre-mixed metal-EDTA complex at a 1:1.05 molar ratio, while the total molarity of other acidified trace metals (Co, Mn, Mo, Zn) was adjusted to maintain constant free ion availability between treatments as those reported in Table 2. Nalgene 250 mL PC bottles were used to culture cells and were soaked in Citranox labware detergent for 1 week, rinsed three times with Milli-Q water, and soaked in 10% trace metal grade HCl for one week before being rinsed three times with Milli-Q and once with the respective media being used (Fitzwater et al., 1982). Care was taken to avoid issues with “bottle memory” by establishing a specific set of labware for each pCu tested.
The artificial sea water (ASW) base of the media formulation was prepared from reagent grade salts according to Harrison et al. (1980) and was sterilized by 0.2 um filtration and microwave treatment. This was followed by filtration through Chelex® 100 chelating ion exchange resin according to the procedure of Price et al. (1989), followed by an additional microwave sterilization. Macronutrient stocks were sterilized following the same protocol (except for microwave treatment), with all manipulations executed in a plastic AirClean® 6000 laminar flow hood.
Cells were grown in an Isotemp™ growth incubator at 26.5° on a 12-12 light/dark cycle at 65 μmol photons m−2 sec−1 and were gently mixed daily. Prior to experimental treatments, cells were maintained in exponential growth for at least 5 generations in pCu 15.95 media either with or without fixed nitrogen before being inoculated into media that had been allowed to equilibrate for at least 48 h but was no older than 1 week. From these pCu 15.95 control treatments, exponentially growing cells were inoculated into 10 treatments spanning 4 orders of magnitude, from potentially limiting to toxic (pCu 15.95 to pCu 11.65). The chemical composition of our control medium is reported in Table 2.
The other 9 treatments were maintained at identical ionic metal concentrations as that reported in Table 2, although the total dissolved concentrations were varied slightly to do so. We monitored growth in our C. watsonii cultures at pCu: 15.35, 13.75, 12.95, 12.65, 12.45, 12.35, 12.15, 11.95, and 11.65 with and without added nitrogen sources (376 μM NaNO3 or 50 μM NH4Cl).
C. watsonii was inoculated at low cellular density, in triplicate, into respective treatments during mid-exponential phase, and growth rates were calculated from the natural logarithm of raw fluorescence (RFU obtained using the Chl-a in vivo module, Trilogy® Turner designs) over 96 h. Previous experiments showed a linear relationship between raw fluorescence and cells mL−1 measured by flow cytometry (Supplementary Figure 1).
Analysis of variance (ANOVA) was used to detect statistically significant differences in mean growth rates between treatments (pCu and nitrogen-sources) using a p < 0.05. This was followed by Tukey's Honest Significant Difference test using an alpha of 0.05 to identify specific treatments with significant differences in observed mean growth rates.
Results
Diazotrophic Cyanobacteria (Figures 2, 3)
N2-fixing cyanobacteria perform the ecological service of reducing inert nitrogen gas into bio-available ammonia. In the oligotrophic marine environment, large fractions of export production are supported by several diazotroph taxa, with the contribution of unicellular genera discovered relatively recently (Zehr et al., 2001).
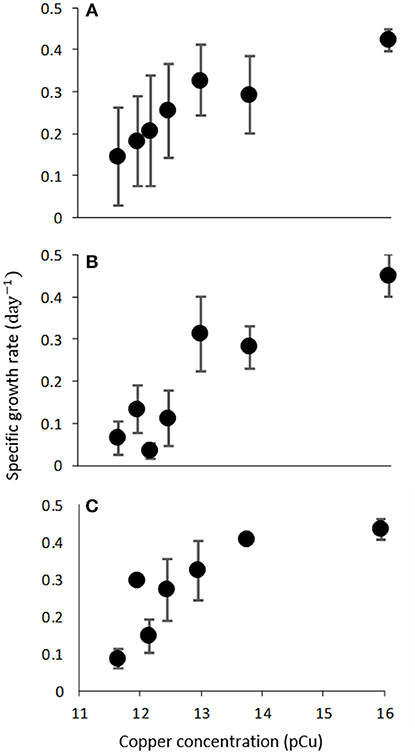
Figure 2. Growth rate (d−1) of C. watsonii WH0003 (A), WH0005 (B), and WH0402 (C) as a function of increasing pCu. Points represent averages of triplicate treatments ± standard error.
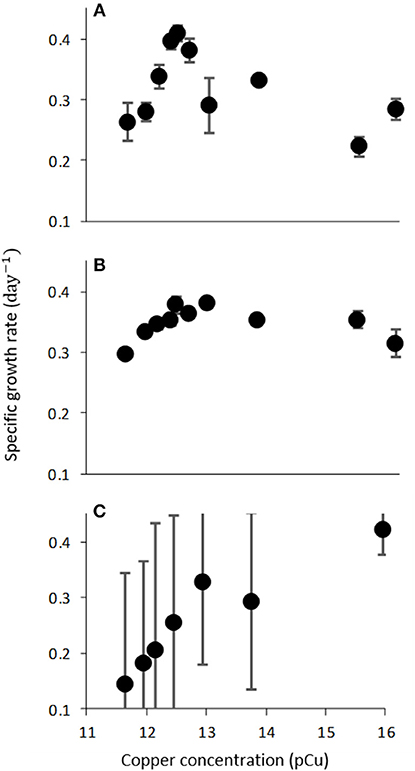
Figure 3. Growth rate (d−1) of C. watsonii WH0003 as function of increasing pCu when grown with varied nitrogen sources; (A) 376 μM NaNO3, (B) 50 μM NH4Cl, and (C) N2-fixation. Points represent averages of triplicate treatments ± standard error.
Very few published studies have investigated Cu toxicity in this environmentally important group. Rueter et al. (1979) reported drastic declines in 14C-fixation of the filamentous diazotroph, Trichodesmium, in response to pCu 10, and Horne and Goldman (1974) monitored suppression of N2-fixation in a eutrophic lake following Cu addition; however, to the best of our knowledge, there are no studies using the unicellular marine species.
We observed consistent growth rates between C. watsonii isolates WH0003, WH0005, and WH0402 grown in nitrogen-free artificial seawater media from pCu 15.95 to pCu 11.65. Maximum division rates agree with previous work (Webb et al., 2009) and remain relatively constant between our control (pCu 15.95) and pCu 13.75 for those cells relying solely on nitrogen-fixation (Figure 2). At pCu 12.15, mean growth rates in all strains were reduced by at least 50%, and at the highest cupric ion molarity tested, mean growth rates are reduced by more than 75%. Thus, C. watsonii appears highly sensitive to cupric ion availability, with sensitivity rivaling that of tested Prochlorococcus isolates (Mann et al., 2002). Statistically significant differences between pCu treatments are summarized in Table 3.
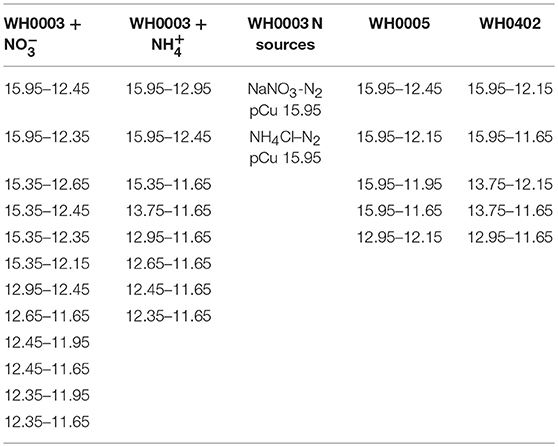
Table 3. Summary of treatments (pCu or nitrogen source) between which statistically significant differences in observed mean growth rate were found according to Tukey's HSD.
The observed sensitivity of C. watsonii growth rates appeared to be reduced by fixed nitrogen supplementation (Figure 3), although differences in mean growth rates at toxic pCu were not found to be significant. C. watsonii WH0003 was selected for additional investigation of Cu sensitivity when supplemented with nitrate and ammonium. All other variables held constant, nitrate and ammonium availability yielded higher mean growth rates at pCu values which otherwise became inhibiting in nitrogen-free media. Interestingly, an exception to this trend was observed at the highest pCu tested. At pCu 15.95, we observed significantly reduced growth rates in the treatments supplied with fixed nitrogen, relative to that of the nitrogen-fixing treatment. Therefore, C. watsonii WH0003 maintains higher growth when co-limited by Cu and nitrogen, than when limited by Cu alone (Table 3).
Meta-Analysis of the Surveyed Literature
From the initial 2,243 search results, 143 relevant publications were identified. Of these, 44 permitted inclusion (Figure 1, Supplementary Table 2). The data extracted from these publications is shown in Figure 4. Below, we discuss the quantitative trends identified through our meta-analysis, with a focus on taxonomic responses. We found that a majority of data on phytoplankton growth as a function of pCu is contained in the single study of Brand et al. (1986).
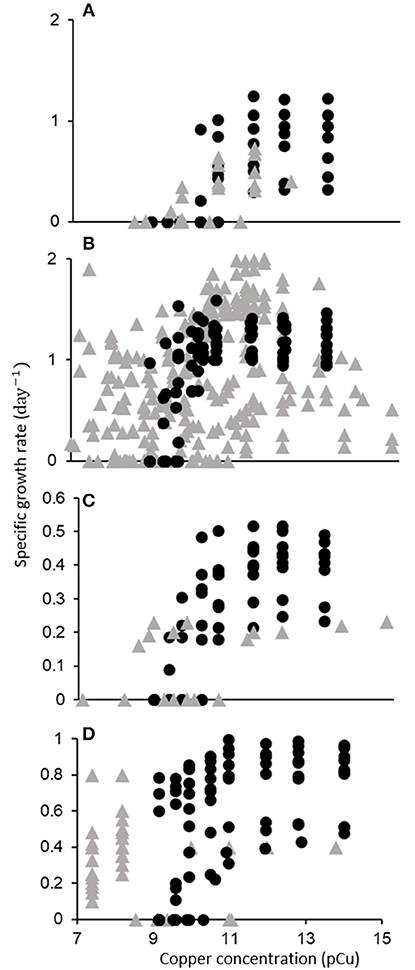
Figure 4. Growth rate (d−1) of major phytoplankton functional types as a function of pCu. Points represent single data points (one growth rate at one pCu) gathered from 44 publications. Data is categorized taxonomically: (A) Cyanobacteria, (B) Diatoms, (C) Dinoflagellates, and (D) Coccolithophores. Filled circles correspond to data from Brand et al. (1986), and shaded triangles correspond to data extracted from all other publications.
Picocyanobacteria (Figure 4A)
Of the seven Synechococcus isolates tested by Brand et al. (1986): two were found to be completely inhibited at pCu 11, three at pCu 10.5, and two at pCu 10. In a more recent study, four additional marine Synechococcus isolates showed significantly diminished growth at pCu 12 and 11 for open-ocean strains, but pCu 10 for coastal ecotypes (Stuart et al., 2009). Although one of the two most sensitive isolates tested by Brand et al. (1986) was an open-ocean strain (WH7805), they did not observe a consistent disparity between neritic and oceanic species. Increased resilience to Cu toxicity in coastal Synechococcus, relative to that of oceanic isolates, was also observed by Debelius et al. (2010), and coastal cyanobacteria exhibited higher sensitivity to Cu additions than picoeukaryotic open-ocean species, supporting the taxonomic trends first published by Brand et al. (1986). Miao et al. (2005) present one of very few investigations of a previously tested isolate by tracking the growth response of Synechococcus bacillaris (CCMP 1333) to increasing Cu2+. This same isolate was used in Brand et al. (1986), yet they report drastically different growth responses. Brand et al. (1986) observed complete cessation of growth by pCu 10.97, yet Miao et al. (2005) report 60% of control growth rates at pCu 10.7 and measurable growth at pCu 10.3. This represents nearly a one order of magnitude discrepancy in pCu sensitivity. Major differences between these studies include seawater sterilization procedures (autoclave vs. filter), seawater chelation (to remove background metal contaminants), macronutrient additions (ten times higher nitrate and four times higher phosphate) and growth temperature and irradiance. We believe that similar disagreement would arise for any species tested using such largely different procedures, as discussed below.
Cu-induced growth inhibition has also been studied in both coastal and open ocean cyanobacterial communities in situ (Gustavson and Wängberg, 1995; Moffett et al., 1997; Mann et al., 2002; Broise and Palenik, 2007; Debelius et al., 2009, 2010, 2011; Paytan et al., 2009; Stuart et al., 2013, 2017). Although the exact origin and structure of metal-binding ligands remains uncertain, there is evidence that cyanobacteria are major sources of class 1 ligands (Moffett et al., 1990; Moffett and Brand, 1996; Croot et al., 2000; Wiramanaden et al., 2008) and may drive the seasonality of Cu speciation in coastal environments (Croot, 2003). Conversely, Cu additions can alter cyanobacterial community composition through selective toxicity, and it appears that natural inputs indeed produce a realized niche-partitioning (Moffett et al., 1997; Mann et al., 2002; Stuart et al., 2013, 2017).
Diatoms (Figure 4B)
Literature on Cu toxicity as a function of pCu among diatoms begins with the dissertation of William Sunda. Sunda (1976) reported growth of Thalassiosira pseudonana (CCMP 1335) at the extreme pCu value of 8.4. Of 6 T. pseudonana isolates tested by Gavis et al. (1981), however, none were able to grow at pCu 8.5, although CCMP 1335 grew at half a division per day at pCu 8.7. Specific growth rates at pCu values between 14 and 15 are in remarkable agreement between Brand et al. (1986) and Annett et al. (2008) who tested the same isolate (CCMP 1335) using natural and artificial seawater, respectively. Davis et al. (2006) observed a rapid genomic response to elevated Cu conditions in the same T. pseudonana isolate. In natural seawater f/2 media, they identified 11 novel, Cu-induced genes, most of which indicated specificity in response. Using a “natural” saltwater composition with added EDTA, we calculated a pCu of approximately 9.8 that corresponds to a reduction in growth rate by more than 50% in Davis et al. (2006)—in agreement with Sunda (1976); Gavis et al. (1981); and Brand et al. (1986). Both T. weissflogii and T. oceanica isolates were strongly (about 50% of control) growth-inhibited near pCu 9.2 as well (Brand et al., 1986; Miao et al., 2005). Miao et al. (2005) and Tang et al. (2005) reported measurable growth (roughly 0.15 d−1) of T. weissflogii isolates at extreme pCu values of 7.7 and 7.6 in natural and artificial seawater, respectively. Also, both Tang et al. (2005) and Chang and Reinfelder (2000) reported fairly constant growth in T. weissflogii (CCMP 1336) between pCu 14.8 to 9.5, although exact methodology differed. Morel et al. (1978) reported on growth responses to elevated Cu2+ in another centric diatom, Skeletonema costatum. Calculated Cu2+ molarities indicated measurable growth up to pCu 8.8, and Brand et al. (1986) confirmed high Cu2+ tolerance among Skeletonema costatum, with specific growth rates in reasonable agreement. Skeletonema costatum isolated elsewhere displayed a similar Cu toxicity threshold below pCu 8.8, with completely inhibited growth at pCu 8.5 (Metaxas and Lewis, 1991). The diatom species Ditylum brightwellii, however, has been reported to be growth-inhibited across a large range of pCu thresholds from as low as 8.5 (Rijstenbil and Gerringa, 2002) to as high as 9.75 (Bentley-Mowat and Reid, 1977; Brand et al., 1986).
To our knowledge, there are no published accounts of in situ diatom growth responses to varying pCu. There are, however, many documented accounts of divergent responses between diatoms and other taxonomic groups in response to reagent and aerosol Cu additions (Hollibaugh et al., 1980; Anderson et al., 1984; Gustavson et al., 1999; Paytan et al., 2009; Henriquez-Castillo et al., 2015; Wang F. J. et al., 2017; Mahowald et al., 2018).
Dinoflagellates (Figure 4C)
As with the previous functional types, Brand et al. (1986) completed the largest survey of dinoflagellate sensitivity to cupric ion availability. They found this group to be generally more sensitive than coccolithophores, but less so than cyanobacteria. Several Peridinium, Prorocentrum, and Thoracosphaera species displayed completely suppressed growth around pCu 10.5. Prior to this, Schenck (1984) documented complete inhibition of Gonyaulax tamarensis at pCu 11, and Pistocchi et al. (1997) later confirmed this sensitivity, noting complete inhibition of Prorocentrum micans and Gonyaulax polyedra at pCu 10.7. Anderson and Morel (1978), however, found that pCu 9.7 was necessary to reduce motility of a coastal Gonyaulax tamarensis isolate. We cannot know if cell division ceased with motility. In Gymnodinium sp. and Gonyaulax tamarensis, Cu sensitivity may be due to relatively high intracellular accumulation and lower extracellular polysaccharide release (Schenck and Hull, 1985; Pistocchi et al., 2000).
To our knowledge, there are no published accounts of dinoflagellate responses to Cu addition in situ that can be discussed in terms of pCu. As a group, dinoflagellates have received the least attention of the four taxonomic groups discussed here.
Coccolithophores (Figure 4D)
Coccolithophores display intermediate sensitivity to elevated pCu relative to cyanobacterial and diatom species. Brand et al. (1986) observed consistent responses between multiple Emiliania huxleyi isolates, with all strains maintaining daily division at pCu as high as 9.58. One of these (CCMP 373) also maintained constant division rates in the artificial seawater medium, AQUIL, between pCu 13.8 and 10 (Dupont and Ahner, 2005). Coccolith morphology and volume in E. huxleyi appears to be sensitive to trace metal availability even while consistent growth rates are maintained (Faucher et al., 2017). The largest sample of isolates tested to date are reported in Echeveste et al. (2018), who monitored the responses of 17 isolates grown in modified AQUIL (EDTA was withheld). We calculate an average pCu of 8.8 to reduce growth rates by half from their reported media composition. However, as Cu speciation in AQUIL sans EDTA is likely to be influenced by excreted ligands, which increased with increasing Cu availability in all isolates examined, the calculated value of 8.8 is uncertain. Similarly, we calculate a pCu of 9.7 to prevent growth in natural seawater cultures of E. huxleyi (CCMP 379) reported by Leal et al. (1999).
In situ observation and incubations involving coccolithophores are few; on the west coast of Norway, Muller et al. (2003) conducted mesocosm experiments during an E. huxleyi bloom and saw consistent reductions in labile Cu to pCu 10.7, at which point ligand release ceased. Hoffmann et al. (2012) noted declines in E. huxleyi numbers following volcanic ash exposure, while that of T. pseudonana increased. Meng et al. (2016), however, recorded stimulatory effects on coccolithophore abundance by aerosol addition to the East China Sea. Hoffmann et al. (2012) measured solubilized trace metal concentrations following addition of ash to seawater, with Cu and Fe content ranging from 1 to 11 nM and 2 to 83 nM per gram of ash, respectively. Meng et al. (2016), however, only reported total mass of metals contained in aerosol samples.
Discussion
This systematic review highlights that most studies on Cu toxicity in marine phytoplankton do not facilitate discussion in terms of pCu. As such, the dominant trend yielded by efforts to describe Cu toxicity between diverse phytoplankton, as that presented here, remains the taxonomic partitioning reported in Brand et al. (1986), where cyanobacteria—particularly Prochlorococcus and Crocosphaera watsonii—are most sensitive, dinoflagellate and coccolithophore species display higher tolerance, and diatom species possess the highest tolerance observed. There exists large variability within these groupings and even between replicated studies, however. We found no further trends in Cu toxicity from the perspectives of microalgal biogeography, finer taxonomic division (e.g., pennate vs. centric diatoms), or cell size and surface area to volume ratios that can be discerned from the available data. Qualitative observations gathered through this review are plentiful (Supplementary Table 1), and many of these studies support the taxonomic trend presented by Brand et al. (1986) (Paytan et al., 2009; Langlois et al., 2012; Mackey et al., 2012, 2017; Stuart et al., 2013; Meng et al., 2016; Wang F. J. et al., 2017).
Suggested Considerations for Future Work
Our literature survey highlighted issues confounding comparability among Cu ecotoxicology studies. Here we briefly discuss some considerations and suggestions to obtain high-quality data and improve replicability in Cu toxicity investigations with regard to three broad issues.
Cu2+ Toxicity Studies Should Account for Trace Metal Speciation
Sunda et al. (2005) presented a detailed discussion on the preparation, composition, and manipulation of chemically defined culture media. We recommend this be read before any attempt to investigate Cu toxicity in photosynthetic microalgae. Of the 99 studies which could not be compared here, 60 were excluded from our quantitative comparison because they were conducted in natural seawater without synthetic chelating agent addition. Therefore, accounting for trace metal speciation is the most overlooked consideration in Cu toxicity investigations, and we stress once more that it is imperative to a replicable study.
Cu2+ Toxicity Studies Should Avoid Batch Culture Protocols and High Cell Densities
Lombardi and Maldonado (2011) found that significantly higher pCu levels were required to halve growth in batch vs. semi-continuous cultures of Phaeocystis cordata. This discrepancy was likely due to accumulation of organic metabolite exudates in the batch cultures, which has been addressed before (Saifullah, 1978; Lombardi and Vieira, 2000; Wiramanaden et al., 2008; Debelius et al., 2009). Their data reinforce that trace metal ecophysiology results obtained using batch cultures should be viewed with caution, particularly when cell densities greatly exceed environmental levels (Vasseur et al., 1988; Moreno-Garrido et al., 2000; Franklin et al., 2002; Debelius et al., 2009). Lower cell densities, in conjunction with semi-continuous or similar culturing methods, reduce accumulation of organic exudates, changes in pH, and required nutrient amendments, all of which may confound observations and replicability.
Macronutrient and Micronutrient Availability Should be Considered
Nitrogen and phosphorus availability altered Cu sensitivity in Ditylum brightwellii, Thalassiosira pseudonana, and Phaeodactylum tricornutum (Rijstenbil and Wijnholds, 1991; Rijstenbil et al., 1998; Wang D. et al., 2017), and mediation of Cu toxicity by high silicic acid concentrations was observed in T. pseudonana and Skeletonema costatum (Morel et al., 1978; Rueter et al., 1981). Likewise, the availability of other trace metals can ameliorate Cu toxicity. Increases in dissolved zinc and manganese reduced sensitivity to added Cu in situ (Braek et al., 1976; Jensen et al., 1976; Kazumi et al., 1987; Coale, 1991) and in vivo (Sunda and Huntsman, 1983, 1998), and both Stauber and Florence (1985) and Maldonado et al. (2002) documented reductions in Cu sensitivity following Fe-enrichment. Alternatively, Cu toxicity was exacerbated by increases in cadmium, zinc, and lead (Bartlett and Rabe, 1973; Khan and Saifullah, 1984, 1986), and Cu availability alters Fe-quotas and uptake in various isolates and even heterogeneous field populations (Peers et al., 2005; Wells et al., 2005; Maldonado et al., 2006; Guo et al., 2012; Biswas et al., 2013; Lelong et al., 2013; Semeniuk et al., 2016). The relatively short timescale of Cu toxicity experiments allows for many experimental treatments. We recommend that nutrient interactions be considered and ideally tested in experimental design.
Cu Toxicity in C. watsonii
Multiple laboratory studies report the growth of C. watsonii to be limited by synergisms between multiple resources (Dyhrman and Haley, 2006; Moisander et al., 2010; Garcia et al., 2013, 2015; Jacq et al., 2014) Most recently, Jacq et al. (2014) and Garcia et al. (2015) observed the size and growth rate of WH8501 and WH0003 cells to be reduced by half in Fe-limited conditions. In the latter strain, however, decreases in cell volume were only achieved when Fe and phosphorus were co-limiting. In fact, cellular growth rates of WH0003 were substantially increased in Fe-deficient media when phosphorus concentrations were low, relative to Fe-replete, high and low phosphorus treatments (Garcia et al., 2015).
We anticipated a reduced Cu sensitivity of WH0003 in the presence of fixed nitrogen as a result of lowered nitrogenase demand. In the presence of high amounts of fixed nitrogen, C. watsonii does not have to rely solely on dinitrogen reduction to meet its nitrogen quota. This is important because the nitrogenase enzyme contains multiple Fe-S clusters which are prone to disruption by high intracellular Cu2+. In WH0003, 10 μM ammonium reduced nitrogen-fixation rates by 83% (Dekaezemacker and Bonnet, 2011). Therefore, fixed nitrogen availability may mollify Cu toxicity and support higher growth in C. watsonii, though further work is needed to confirm a relationship between the two resources, as nitrogen source was not found to significantly alter Cu toxicity in WH0003.
Conclusions
The consistent responses reported within a small subset of the studies discussed above are encouraging, and the changes in natural community composition following Cu addition suggest that selective pressure does occur. We argue, however, that much remains to be learned about the influence of Cu toxicity on marine phytoplankton biogeography and biogeochemical cycling, despite a seemingly extensive literature. The characterization of diverse species' responses to Cu2+ availability warrants further investigation. Future work should take care to ensure that observations are replicable. Through careful selection of test organisms and deliberate control of media composition, we believe more robust relationships may emerge.
Author Contributions
JL and LL substantial contributions to the conception or design of the work and analysis/collection of experimental data. JL and KM interpretation of data and drafting of work or revising it critically, as well as final approval of version to be published.
Funding
This material is based upon work supported by the National Science Foundation Graduate Research Fellowship Program under Grant No. DGE-1321846 to JL and a Simons Early Career Award in Marine Microbial Ecology and Evolution to KM.
Conflict of Interest Statement
The authors declare that the research was conducted in the absence of any commercial or financial relationships that could be construed as a potential conflict of interest.
Supplementary Material
The Supplementary Material for this article can be found online at: https://www.frontiersin.org/articles/10.3389/fmars.2018.00511/full#supplementary-material
Supplementary Figure 1. Robust linear relationship between raw fluorescence (RFU) and cell counts (cells/mL) for C. Watsonii strains used.
References
Anderson, D. M., Lively, J. S., and Vaccaro, R. F. (1984). Copper complexation during spring phytoplankton blooms in coastal waters. J. Mar. Res. 42, 677–695. doi: 10.1357/002224084788506013
Anderson, D. M., and Morel, F. M. M. (1978). Copper sensitivity of Gonyaulax tamarensis. Limnol. Oceanogr. 23, 283–295. doi: 10.4319/lo.1978.23.2.0283
Annett, A. L., Lapi, S., Ruth, T. J., and Maldonado, M. T. (2008). The effects of Cu and Fe availability on the growth and Cu: C ratios of marine diatoms. Limnol. Oceanogr. 53, 2451–2461. doi: 10.4319/lo.2008.53.6.2451
Armstrong, F. A. J., Williams, P. M., and Strickland, J. D. H. (1966). Photo-oxidation of organic matter in sea water by ultra-violet radiation, analytical and other applications. Nature 211, 481–483. doi: 10.1038/211481a0
Artaxo, P., Oyola, P., and Martinez, R. (1999). Aerosol composition and source apportionment in Santiago de Chile. Nucl. Instrum. Meth. B 150, 409–416. doi: 10.1016/S0168-583X(98)01078-7
Bartlett, L., and Rabe, F. W. (1973). Effects of copper, zinc, and cadmium on Selanastrum capricornutum. Water Res. 8, 179–185. doi: 10.1016/0043-1354(74)90041-4
Bentley-Mowat, J. A., and Reid, S. M. (1977). Survival of marine phytoplankton in high concentrations of heavy metals, and uptake of copper. J. Exp. Mar. Biol. Ecol. 26, 249–264. doi: 10.1016/0022-0981(77)90085-5
Biswas, H., Bandyopadhyay, D., and Waite, A. (2013). Copper addition helps alleviate iron stress in a coastal diatom: response of Chaetoceros gracilis from the Bay of Bengal to experimental Cu and Fe addition. Mar. Chem. 157, 224–232. doi: 10.1016/j.marchem.2013.10.006
Blake, A. C., Chadwick, D. B., Zirino, A., and Rivera-Duarte, I. (2004). Spatial and temporal variations in copper speciation in San Diego Bay. Estuaries 27, 437–447. doi: 10.1007/BF02803536
Braek, G. S., Jensen, A., and Mohus, A. (1976). Heavy metal tolerance of marine phytoplankton. III. Combined effects of copper and zinc ions on cultures of four common species. J. Exp. Mar. Biol. Ecol. 25, 37–50. doi: 10.1016/0022-0981(76)90074-5
Brand, L. E., Sunda, W. G., and Guillard, R. R. (1986). Reduction of marine phytoplankton reproduction rates by copper and cadmium. J. Exp. Mar. Biol. Ecol. 96, 225–250. doi: 10.1016/0022-0981(86)90205-4
Broise, D. D., and Palenik, B. P. (2007). Immerse in situ microcosms: a tool for the assessment of pollution impact on phytoplankton. J. Exp. Mar. Biol. Ecol. 341, 274–281. doi: 10.1016/j.jembe.2006.10.045
Bruland, K. W., Donat, J. R., and Hutchins, D. A. (1991). Interactive influences of bioactive trace metals on biological production in oceanic waters. Limnol. Oceanogr. 36, 1555–1577. doi: 10.4319/lo.1991.36.8.1555
Buck, K. N., Moffett, J., Barbeau, K. A., Bundy, R. M., Kondo, Y., and Wu, J. (2012). The organic complexation of iron and copper: an intercomparison of competitive ligand exchange–adsorptive cathodic stripping voltammetry (CLE-ACSV) techniques. Limnol. Oceanogr. Methods 10, 496–515. doi: 10.4319/lom.2012.10.496
Chadd, H. E., Newman, J., Mann, N. H., and Carr, N. G. (1996). Identification of iron superoxide dismutase and a copper/zinc superoxide dismutase enzyme activity within the marine cyanobacterium Synechococcus sp. WH 7803. FEMS Microbiol. Lett. 138, 161–165. doi: 10.1016/0378-1097(96)00096-1
Chang, S. I., and Reinfelder, J. R. (2000). Bioaccumulation, subcellular distribution, and trophic transfer of copper in a coastal marine diatom. Environ. Sci. Technol. 34, 4931–4935. doi: 10.1021/es001213r
Chen, Y., Paytan, A., Chase, Z., Measures, C., Beck, A. J., Sañudo-Wilhelmy, S. A., et al. (2008). Sources and fluxes of atmospheric trace elements to the Gulf of Aqaba, Red Sea. J. Geophys. Res.-Atmos. 113:110. doi: 10.1029/2007JD009110
Chen, Y. B., Zehr, J. P., and Mellon, M. (1996). Growth and nitrogen fixation of the diazotrophic filamentous nonheterocystous cyanobacterium Trichodesmium sp. IMS 101 in defined media: evidence for a circadian rhythm. J. Phycol. 32, 916–923. doi: 10.1111/j.0022-3646.1996.00916.x
Chillappagari, S., Seubert, A., Trip, H., Kuipers, O. P., Marahiel, M. A., and Miethke, M. (2010). Copper stress affects iron homeostasis by destabilizing iron-sulfur cluster formation in Bacillus subtilis. J. Bacteriol. 192, 2512–2524. doi: 10.1128/JB.00058-10
Coale, K. H. (1991). Effects of iron, manganese, copper, and zinc enrichments on productivity and biomass in the subarctic Pacific. Limnol. Oceanogr. 36, 1851–1864. doi: 10.4319/lo.1991.36.8.1851
Coale, K. H., and Bruland, K. W. (1988). Copper complexation in the Northeast Pacific. Limnol. Oceanogr. 33, 1084–1101. doi: 10.4319/lo.1988.33.5.1084
Coale, K. H., and Bruland, K. W. (1990). Spatial and temporal variability in copper complexation in the North Pacific. Deep-Sea Res. 37, 317–336. doi: 10.1016/0198-0149(90)90130-N
Croot, P. L. (2003). Seasonal cycle of copper speciation in Gullmar Fjord, Sweden. Limnol. Oceanogr. 48, 764–776. doi: 10.4319/lo.2003.48.2.0764
Croot, P. L., Moffett, J. W., and Brand, L. E. (2000). Production of extracellular Cu complexing ligands by eukaryotic phytoplankton in response to Cu stress Limnol. Oceanogr. 45, 619–627. doi: 10.4319/lo.2000.45.3.0619
Davis, A. K., Hildebrand, M., and Palenik, B. (2006). Gene expression induced by copper stress in the diatom Thalassiosira pseudonana. Eukaryot. Cell 5, 1157–1168. doi: 10.1128/EC.00042-06
De la Rosa, M. A., Navarro, J. A., Diaz-Quintana, A., De la Cerda, B., Molina-Heredia, F. P., Balme, A., et al. (2002). An evolutionary analysis of the reaction mechanisms of photosystem 1 reduction by cytochrome c6 and plastocyanin. Bioelectrochemistry 55, 41–45. doi: 10.1016/S1567-5394(01)00136-0
Debelius, B., Forja, J. M., DelValls, Á., and Lubián, L. M. (2010). Toxic effect of copper on marine picophytoplankton populations isolated from different geographic locations. Sci. Mar. 74, 133–141. doi: 10.3989/scimar.2010.74s1133
Debelius, B., Forja, J. M., DelValls, T. A., and Lubián, L. M. (2009). Toxicity of copper in natural marine picoplankton populations. Ecotoxicology 18, 1095–1103. doi: 10.1007/s10646-009-0377-3
Debelius, B., Forja, J. M., and Lubian, L. M. (2011). Toxicity of copper, nickel and zinc to Synechococcus populations from the strait of Gibraltar. J. Mar. Syst. 88, 113–119. doi: 10.1016/j.jmarsys.2011.02.009
Dekaezemacker, J., and Bonnet, S. (2011). Sensitivity of N2 fixation to combined nitrogen forms in two strains of the marine diazotroph Crocosphaera watsonii (Cyanobacteria). Mar. Ecol. Prog. Ser. 438, 33–46. doi: 10.3354/meps09297
Denier van der Gon, H. A. C., Hulskotte, J. H. J., Visschedijk, A. J. H., and Schaap, M. (2007). A revised estimate of copper emissions from road transport in UNECE-Europe and its impact on predicted copper concentrations. Atmos Environ. 41, 8697–8710. doi: 10.1016/j.atmosenv.2007.07.033
Duce, R. A., Liss, P. S., Merrill, J. T., Atlas, E. L., Buat-Menard, P., Hicks, B. B., et al. (1991). The atmospheric input of trace species to the world ocean, Global Biogeochem. Cy. 5, 193–259. doi: 10.1029/91GB01778
Dupont, C. L., and Ahner, B. A. (2005). Effects of copper, cadmium, and zinc on the production and exudation of thiols by Emiliania huxleyi. Limnol. Oceanogr. 50, 508–515. doi: 10.2307/3597725
Dyhrman, S. T., and Haley, S. T. (2006). Phosphorus scavenging in the unicellular marine diazotroph Crocosphaera watsonii. Appl. Environ. Microbiol. 72, 1452–1458. doi: 10.1128/AEM.72.2.1452-1458.2006
Echeveste, P., Croot, P., and von Dassow, P. (2018). Differences in the sensitivity to Cu and ligand production of coastal vs offshore strains of Emiliania huxleyi. Sci. Total Environ. 625, 1673–1680. doi: 10.1016/j.scitotenv.2017.10.050
Erickson, S. J. (1972). Toxicity of copper to Thalassiosira pseudonana in unenriched inshore seawater. J. Phycol. 8, 318–323. doi: 10.1111/j.1529-8817.1972.tb04045.x
Erickson, S. J., Lackie, N., and Maloney, T. E. (1970). A screening technique for estimating copper toxicity to estuarine phytoplankton. J. Water Pollut. Con. F. 42, R270–R278.
Faucher, G., Hoffmann, L., Bach, L. T., Bottini, C., Erba, E., and Riebesell, U. (2017). Impact of trace metal concentrations on coccolithophore growth and morphology: laboratory simulations of Cretaceous stress. Biogeosciences 14, 3603–3613. doi: 10.5194/bg-14-3603-2017
Fitzgerald, G. P., and Faust, S. L. (1963). Factors affecting the algicidal and algistatic properties of copper. Appl. Microbiol. 11, 345–351.
Fitzwater, S. E., Knauer, G. A., and Martin, J. H. (1982). Metal contamination and its effect on primary production estimates. Limnol. Oceanogr. 27, 544–551. doi: 10.4319/lo.1982.27.3.0544
Franklin, N. M., Stauber, J. L., Apte, S. C., and Lim, R. P. (2002). Effect of initial cell density on the bioavailability and toxicity of copper in microalgal bioassays. Environ. Toxicol. Chem. 21, 742–751. doi: 10.1002/etc.5620210409
Garcia, N. S., Fu, F., Sedwick, P. N., and Hutchins, D. A. (2015). Iron deficiency increases growth and nitrogen-fixation rates of phosphorus-deficient marine cyanobacteria. ISME J. 9:238. doi: 10.1038/ismej.2014.104
Garcia, N. S., Fu, F.-X., Breene, C. L., Yu, E. K., Bernhardt, P. W., Mulholland, M. R., et al. (2013). Combined effects of CO2 and light on large and small isolates of the unicellular N 2 -fixing cyanobacterium Crocosphaera watsonii from the western tropical Atlantic Ocean. Eur. J. Phycol. 48, 128–139. doi: 10.1080/09670262.2013.773383
Gavis, J. R., GuiIlard, R. L., and Woodward, B. L. (1981). Cupric ion activity and the growth of phytoplankton clones isolated from different marine environments. Mar. Res. 39, 315–333.
Gekeler, W., Grill, E., Winnacker, E.-L., and Zenk, M. H. (1988). Algae sequester heavy metals via synthesis of phytochelatin complexes. Arch. Microbiol. 150, 197–202. doi: 10.1007/BF00425162
Giner-Lamia, J., López-Maury, L., and Florencio, F. J. (2014). Global transcriptional profiles of the copper responses in the Cyanobacterium Synechocystis sp. PCC 6803. PLoS ONE 9:e108912. doi: 10.1371/journal.pone.0108912
Guo, J., Lapi, S., Ruth, T. J., and Maldonado, M. T. (2012). The effects of iron and copper availability on the copper stoichiometry of marine phytoplankton 1: Cu quotas of marine phytoplankton. J. Phycol. 48, 312–325. doi: 10.1111/j.1529-8817.2012.01133.x
Gustavson, K., Petersen, S., Pedersen, B., Stuer-Lauridsen, F., Pedersen, S., and Wängberg, S. (1999). Pollution-Induced Community Tolerance (PICT) in coastal phytoplankton communities exposure to copper. Hydrobiologia 416, 125–138. doi: 10.1023/A:1003811419842
Gustavson, K., and Wängberg, S. (1995). Tolerance induction and succession in microalgae communities exposed to copper and atrazine. Aquat. Toxicol. 32, 283–302. doi: 10.1016/0166-445X(95)00002-L
Haber, F., and Weiss, J. (1934). The catalytic decomposition of hydrogen peroxide by iron salts. P. R. Soc. Lond. 147, 332–351. doi: 10.1098/rspa.1934.0221
Halliwell, B., and Gutteridge, J. M. C. (1984). Oxygen toxicity, oxygen radicals, transition metals and disease. Biochem. J. 219, 1–14. doi: 10.1042/bj2190001
Harrison, P. J., Waters, R. E., and Taylor, F. J. R. (1980). A broad spectrum artificial seawater medium for coastal and open ocean phytoplankton. J. Phycol. 16, 28–35. doi: 10.1111/j.0022-3646.1980.00028.x
Henriquez-Castillo, C., Rodríguez-Marconi, S., Rubio, F., Trefault, N., Andrade, S., and De la Iglesia, R. (2015). Eukaryotic pico-phytoplankton community response to copper enrichment in a metal perturbed coastal environment. Phycol. Res. 63, 189–196. doi: 10.1111/pre.12087
Ho, K. K., and Krogmann, D. W. (1984). Electron donors to P700 in cyanobacteria and algae: an instance of unusual genetic variability. Biochim. Biophys. Acta 766, 310–316. doi: 10.1016/0005-2728(84)90246-9
Hoffmann, L., Breitbarth, E., Ardelan, M., Duggen, S., Olgun, N., Hassellöv, M., et al. (2012). Influence of trace metal release from volcanic ash on growth of Thalassiosira pseudonana and Emiliania huxleyi. Mar. Chem. 132–133, 28–33. doi: 10.1016/j.marchem.2012.02.003
Hollibaugh, J. T., Seibert, D. L. R., and Thomas, W. H. (1980). A comparison of the acute toxicities of ten heavy metals to phytoplankton from Saanich inlet, B.C., Canada. Estuar. Coast Mar. Sci. 10, 93–105. doi: 10.1016/S0302-3524(80)80052-1
Hong, S., Candelone, J., Soutif, M., and Boutron, C. F. (1996). A reconstruction of changes in copper production and copper emissions to the atmosphere during the past 7000 years. Sci. Total Environ. 188, 183–193. doi: 10.1016/0048-9697(96)05171-6
Hong-Hermesdorf, A., Miethke, M., Gallaher, S. D., Kropat, J., Dodani, S. C., Chan, J., et al. (2014). Subcellular metal imaging identifies dynamic sites of Cu accumulation in Chlamydomonas. Nat. Chem. Biol. 10, 1034–1042. doi: 10.1038/nchembio.1662
Horne, A. J., and Goldman, C. R. (1974). Suppression of nitrogen fixation by blue-green algae in a eutrophic lake with trace additions of copper. Science 184, 409–411. doi: 10.1126/science.183.4123.409
Huertas, M., López-Maury, L., Giner-Lamia, J., Sánchez-Riego, A., and Florencio, F. (2014). Metals in Cyanobacteria: analysis of the copper, nickel, cobalt and arsenic homeostasis mechanisms. Life 4, 865–886. doi: 10.3390/life4040865
Jacq, V., Ridame, C., L'Helguen, S., Kaczmar, F., and Saliot, A. (2014). Response of the unicellular diazotrophic Cyanobacterium Crocosphaera watsonii to iron limitation. PLoS ONE 9:e86749. doi: 10.1371/journal.pone.0086749
Jensen, A., Rystad, B., and Melsom, S. (1976). Heavy metal tolerance of marine phytoplankton II. Copper tolerance of three species in dialysis and batch cultures. J. Exp. Mar. Biol. Ecol. 22, 249–256. doi: 10.1016/0022-0981(76)90004-6
Kazumi, J., Zorkin, N., and Lewis, A. G. (1987). The effect of manganese-copper interactions on growth of a diatom in water from a manganese-rich British Columbia fjord. Estuar. Coast. Shelf. S. 25, 337–346. doi: 10.1016/0272-7714(87)90076-X
Kehrer, J. P. (2000). The Haber–Weiss reaction and mechanisms of toxicity. Toxicology 149, 43–50. doi: 10.1016/S0300-483X(00)00231-6
Khan, S. H., and Saifullah, S. M. (1984). Toxic effects of copper and lead on a tropical marine blue-green phytoplankter, Oscillatoria thiebautii. Pak. J. Bot. 16, 267–274.
Khan, S. H., and Saifullah, S. M. (1986). Bioassay studies of phytoplankton of coastal waters of Karachi in relation to heavy metal pollution: 1. Effect of copper and lead on Skeletonema costatum. Pak. J. Bot. 18, 137–145.
La Fontaine, S., Quinn, J. M., Nakamoto, S. S., Page, M. D., Gohre, V., Moseley, J. L., et al. (2002). Copper-dependent iron assimilation pathway in the model photosynthetic eukaryote Chlamydomonas reinhardtii. Eukaryotic Cell 1, 736–757. doi: 10.1128/EC.1.5.736-757.2002
Langlois, R., Mills, M., Ridame, C., Croot, P., and LaRoche, J. (2012). Diazotrophic bacteria respond to Saharan dust additions. Mar. Ecol. Prog. Ser. 470, 1–14. doi: 10.3354/meps10109
Leal, M. F. C., Vasconcelos, M., and van den Berg, C. M. (1999). Copper-induced release of complexing ligands similar to thiols by Emiliania huxleyi in seawater cultures. Limnol. Oceanogr. 44, 1750–1762. doi: 10.4319/lo.1999.44.7.1750
Leal, P. P., Hurd, C. L., Sander, S. G., Armstrong, E., and Roleda, M. Y. (2016). Copper ecotoxicology of marine algae: a methodological appraisal. Chem. Ecol. 32, 786–800. doi: 10.1080/02757540.2016.1177520
Lelong, A., Bucciarelli, E., Hégaret, H., and Soudant, P. (2013). Iron and copper limitations differently affect growth rates and photosynthetic and physiological parameters of the marine diatom Pseudo-nitzschia delicatissima. Limnol. Oceanogr. 58, 613–623. doi: 10.4319/lo.2013.58.2.0613
Li, Y., Hodak, M., and Bernholc, J. (2015). Enzymatic mechanism of copper-containing nitrite reductase. Biochemistry 54, 1233–1242. doi: 10.1021/bi5007767
Liping, W., and Binghui, Z. (2008). Toxic effects of fluoranthene and copper on marine diatom Phaeodactylum tricornutum. J. Environ. Sci. 20, 1363–1372. doi: 10.1016/S1001-0742(08)62234-2
Lombardi, A. T., and Maldonado, M. T. (2011). The effects of copper on the photosynthetic response of Phaeocystis cordata. Photosynth. Res. 108, 77–87. doi: 10.1007/s11120-011-9655-z
Lombardi, A. T., and Vieira, A. A. H. (2000). Copper complexation by Cyanophyta and Chlorophyta exudates. Phycologia 39:2, 118–125. doi: 10.2216/i0031-8884-39-2-118.1
Mackey, K. R. M., Buck, K. N., Casey, J. R., Cid, A., Lomas, M. W., Sohrin, Y., et al. (2012). Phytoplankton responses to atmospheric metal deposition in the coastal and open-ocean Sargasso Sea. Front. Microbiol. 3:359. doi: 10.3389/fmicb.2012.00359
Mackey, K. R. M., Kavanaugh, M., Wang, F., Chen, Y., Liu, F., Glover, D., et al. (2017). Atmospheric and fluvial nutrients fuel algal blooms in the East China Sea. Front. Mar. Sci. 4:2. doi: 10.3389/fmars.2017.00002
Macomber, L., and Imlay, J. A. (2009). The iron-sulfur clusters of dehydratases are primary intracellular targets of copper toxicity. P. Natl. Acad. Sci. U.S.A. 106, 8344–8349. doi: 10.1073/pnas.0812808106
Maenhaut, W., Salma, I., Cafmeyer, J., Annegarn, H. J., and Andreae, M. O. (1996). Regional atmospheric aerosol composition and sources in the eastern Transvaal, South Africa, and impact of biomass burning. J. Geophys. Res. 101, 23631–23650. doi: 10.1029/95JD02930
Mahowald, N. M., Hamilton, D. S., Mackey, K. R. M., Moore, J. K., Baker, A. R., Scanza, R. A., et al. (2018). Aerosol trace metal deposition dissolution and impacts on marine microorganisms and biogeochemistry. Nat. Commun. 9:2614. doi: 10.1038/s41467-018-04970-7
Maienthal, E., and Becker, D. (1976). A survey of current literature on sampling, sample handling, and long term storage for environmental materials. Natl. Bur. Stand. Tech. Note. 1976:929. doi: 10.6028/NBS.TN.929
Maldonado, M. T., Allen, A. E., Chong, J. S., Lin, K., Leus, D., Karpenko, N., et al. (2006). Copper-dependent iron transport in coastal and oceanic diatoms. Limnol. Oceanogr. 51, 1729–1743. doi: 10.4319/lo.2006.51.4.1729
Maldonado, M. T., Hughes, M. P., Rue, E. L., and Wells, M. L. (2002). The effect of Fe and Cu on growth and domoic acid production by Pseudo-nitzschia multiseries and Pseudo-nitzschia australis. Limnol. Oceanogr. 47, 515–526. doi: 10.4319/lo.2002.47.2.0515
Maloney, T. E., and Palmer, C. M. (1956). Toxicity of six chemical compounds to thirty cultures of algae. Wat. Sewage Works 103, 509–513.
Manahan, S. E., and Smith, M. J. (1973). Copper micronutrient requirement for algae. Envir. Sci. Tech. 7, 829–833. doi: 10.1021/es60081a013
Mann, E. L., Ahlgren, N., Moffett, J. W., and Chisholm, S. W. (2002). Copper toxicity and cyanobacteria ecology in the Sargasso Sea. Limnol. Oceanogr. 47, 976–988. doi: 10.4319/lo.2002.47.4.0976
Meng, X., Chen, Y., Wang, B., Ma, Q. W., and Wang, F. J. (2016). Responses of phytoplankton community to the input of different aerosols in the East China Sea: different aerosols change algae diversity. Geophys. Res. Lett. 43, 7081–7088. doi: 10.1002/2016GL069068
Metaxas, A., and Lewis, A. G. (1991). Copper tolerance of Skeletonema costatum and Nitzschia thermalis. Aquat. Toxicol. 19, 265–280. doi: 10.1016/0166-445X(91)90052-B
Miao, A.-J., Wang, W.-X., and Juneau, P. (2005). Comparison of Cd, Cu, and Zn toxic effects on four marine phytoplankton by pulse-amplitude-modulated fluorometry. Environ. Toxicol. Chem. 24, 2603–2611. doi: 10.1897/05-009R.1
Moffett, J. W. (1995). Temporal and spatial variability of copper complexation by strong chelators in the Sargasso Sea. Deep-Sea Res. Pt. I 42, 1273–1295. doi: 10.1016/0967-0637(95)00060-J
Moffett, J. W., and Brand, L. E. (1996). Production of strong, extracellular Cu chelators by marine cyanobacteria in response to Cu stress. Limnol. Oceanogr. 41, 388–395. doi: 10.4319/lo.1996.41.3.0388
Moffett, J. W., Brand, L. E., Croot, P. L., and Barbeau, K. A. (1997). Cu speciation and cyanobacterial distribution in harbors subject to anthropogenic Cu inputs. Limnol. Oceanogr. 42, 789–799. doi: 10.4319/lo.1997.42.5.0789
Moffett, J. W., and Dupont, C. (2007). Cu complexation by organic ligands in the sub-arctic NW Pacific and Bering Sea. Deep-Sea Res. Pt. I 54, 586–595. doi: 10.1016/j.dsr.2006.12.013
Moffett, J. W., Zika, R. G., and Brand, L. (1990). Distribution and potential sources and sinks of copper chelators in the Sargasso Sea. Deep-Sea Res. 31, 27–36. doi: 10.1016/0198-0149(90)90027-S
Moisander, P. H., Beinart, R. A., Hewson, I., White, A. E., Johnson, K. S., Carlson, C. A., et al. (2010). Unicellular cyanobacterial distributions broaden the oceanic N2 fixation domain. Science 327, 1512–1514. doi: 10.1126/science.1185468
Moore, G. T., and Kellerman, K. F. (1904). A method of destroying or preventing the growth of algae and certain pathogenic bacteria in water supplies. U.S. Dept. Agri. Bur. Plant Ind. Bull. 64, 1–44.
Morel, F. M. M., and Herring, J. G. (1993). Principles and Applications of Aquatic Chemistry. New York, NY: John Wiley and Sons, Inc.
Morel, F. M. M., Rueter, J. G., Anderson, D. M., and Guillard, R. R. L. (1979). AQUIL: a chemically defined phytoplankton culture medium for trace metal studies. J. Phycol. 15, 135–141. doi: 10.1111/j.1529-8817.1979.tb02976.x
Morel, N. M. L., Rueter, J. G., and Morel, F. M. M. (1978). Copper toxicity to Skeletonema costatum (Bacillariophyceae). J. Phycol. 14, 43–48. doi: 10.1111/j.1529-8817.1978.tb00629.x
Moreno-Garrido, I., Lubián, L. M., and Soares, A. M. V. M. (2000). Influence of cellular density on determination of EC50 in microalgal growth inhibition tests. Ecotox. Environ. Safety 47, 112–116. doi: 10.1006/eesa.2000.1953
Morgan, G. B., and Lackey, J. B. (1958). B.O.D. Determinations in wastes containing chelated copper or chromium. Sew. Ind. Wastes. 30, 283–286.
Muller, F. L., Jacquet, S., and Wilson, W. H. (2003). Biological factors regulating the chemical speciation of Cu, Zn, and Mn under different nutrient regimes in a marine mesocosm experiment. Limnol. Oceanogr. 48, 2289–2302. doi: 10.4319/lo.2003.48.6.2289
Nriagu, J. O. (1979). Global inventory of natural and anthropogenic emissions of trace metals to the atmosphere. Nature 279:409. doi: 10.1038/279409a0
Nriagu, J. O. (1989). A global assessment of natural sources of atmospheric trace metals. Nature 338:47. doi: 10.1038/338047a0
Osman, D., and Cavet, J. S. (2008). Copper homeostasis in bacteria. Adv. Appl. Microbiol. 65, 217–247. doi: 10.1016/S0065-2164(08)00608-4
Palenik, B., Kieber, D. J., and Morel, F. M. M. (1989). Dissolved organic nitrogen use by phytoplankton: the role of cell-surface enzymes. Biol. Oceanogr. 6, 347–354. doi: 10.1080/01965581.1988.10749536
Paytan, A., Mackey, K. R. M., Chen, Y., Lima, I. D., Doney, S. C., Mahowald, N., et al. (2009). Toxicity of atmospheric aerosols on marine phytoplankton. P. Natl. Acad. Sci. 106, 4601–4605. doi: 10.1073/pnas.0811486106
Peers, G., Quesnel, S.-A., and Price, N. M. (2005). Copper requirements for iron acquisition and growth of coastal and oceanic diatoms. Limnol. Oceanogr. 4, 1149–1158. doi: 10.4319/lo.2005.50.4.1149
Pistocchi, R., Guerrini, F., Balboni, V., and Boni, L. (1997). Copper toxicity and carbohydrate production in the microalgae Cylindrotheca fusiformis and Gymnodinium sp. Eur. J. Phycol. 32, 125–132. doi: 10.1080/09670269710001737049
Pistocchi, R., Mormile, M. A., Guerrini, F., Isani, G., and Boni, L. (2000). Increased production of extra- and intracellular metal-ligands in phytoplankton exposed to copper and cadmium. J. Appl. Phycol. 12, 469–477. doi: 10.1023/A:1008162812651
Price, N. M., Harrison, G. I., Hering, J. G., Hudson, R. J., Nirel, P. M., Palenik, B., et al. (1989). Preparation and chemistry of the artificial algal culture medium Aquil. Biol. Oceanogr. 6, 443–461. doi: 10.1080/01965581.1988.10749544
Prospero, J. M. (1999). Long-term measurements of the transport of African mineral dust to the southeastern United States: Implications for regional air quality. J. Geophys. Res. 104, 15917–15927. doi: 10.1029/1999JD900072
Rasmussen, T., Berks, B. C., Sanders-Loehr, J., Dooley, D. M., Zumft, W. G., and Thomson, A. J. (2000). The catalytic center in nitrous oxide reductase, Cuz, is a copper-sulfide cluster. Biochem 39, 12753–12756. doi: 10.1021/bi001811i
Rijstenbil, J. W., Dehairs, F., Ehrlich, R., and Wijnholds, J. A. (1998). Effect of the nitrogen status on copper accumulation and pools of metal-binding peptides in the planktonic diatom Thalassiosira pseudonana. Aquat. Toxicol. 42, 187–209. doi: 10.1016/S0166-445X(97)00091-X
Rijstenbil, J. W., and Gerringa, L. J. A. (2002). Interactions of algal ligands, metal complexation and availability, and cell responses of the diatom Ditylum brightwellii with a gradual increase in copper. Aquat. Toxicol. 56, 115–131. doi: 10.1016/S0166-445X(01)00188-6
Rijstenbil, J. W., and Wijnholds, J. A. (1991). Copper toxicity and adaptation in the marine diatom Ditylum brightwellii. Comp. Biochem. Phys. C 100, 147–150. doi: 10.1016/0742-8413(91)90142-G
Rocha, G. S., Parrish, C. C., Lombardi, A. T., da, G. G., and Melão, M. (2016). Copper affects biochemical and physiological responses of Selenastrum gracile (Reinsch). Ecotoxicology 25, 1468–1477. doi: 10.1007/s10646-016-1698-7
Rueter, J. G., Chisholm, S. W., and Morel, F. M. (1981). Effects of copper toxicity on silicic acid uptake and growth in Thalassiosira Pseudonana. J. Phycol. 17, 270–278. doi: 10.1111/j.1529-8817.1981.tb00850.x
Rueter, J. G., McCarthy, J. J., and Carpenter, E. J. (1979). The toxic effect of copper on Oscillatoria (Trichodesmium) theibautii. Limnol. Oceanogr. 24, 558–562. doi: 10.4319/lo.1979.24.3.0558
Saifullah, S. M. (1978). Inhibitory effects of copper on marine dinoflagellates. Mar. Biol. 44, 299–308. doi: 10.1007/BF00390893
Sanders, P. G., Xu, N., Dalka, T. M., and Maricq, M. M. (2003). Airborne brake wear debris: size distributions, composition, and a comparison of dynamometer and vehicle tests. Environ. Sci. Tech. 37, 4060–4069. doi: 10.1021/es034145s
Sanhueza, E. (1996). “Effects of vegetation burning on the atmospheric chemistry of the Venezuelan Savanna,” in Biomass Burning and Global Change, ed J. S. Levine (Cambridge, MA: MIT Press), 122–125.
Schauer, J. J., Lough, G. C., Shafer, M. M., Christensen, W. F., Arndt, M. F., DeMinter, J. T., et al. (2006). Characterization of metals emitted from motor vehicles. Res. Rep. Health Eff. Inst. 133, 77–88.
Schecher, W. D., and McAvoy, D. C. (1992). MINEQL+: a software environment for chemical equilibrium modeling. Comput. Environ. Urban Syst. 16, 65–76. doi: 10.1016/0198-9715(92)90053-T
Schenck, R. (1984). Copper deficiency and toxicity in Gonyaulax tamarensis. Mar. Biol. Lett. 5, 13–19.
Schenck, R., and Hull, R. J. (1985). Copper uptake and efflux in Gonyaulax tamarensis (Dinophyceae). Bot. Mar. 28, 275–282. doi: 10.1515/botm.1985.28.7.275
Schwarzenbach, R. P., Gschwend, P. M., and Imboden, D. M. (2003). Environmental Organic Chemistry. New York, NY: John Wiley and Sons Inc.
Semeniuk, D. M., Taylor, R. L., Bundy, R. M., Johnson, W. K., Cullen, J. T., Robert, M., et al. (2016). Iron-copper interactions in iron-limited phytoplankton in the northeast subarctic Pacific Ocean: Fe-Cu interactions in HNLC phytoplankton Limnol. Oceanogr. 61, 279–297. doi: 10.1002/lno.10210
Shank, G. C., Skrabal, S. A., Whitehead, R. F., and Kieber, R. J. (2004). Strong copper complexation in an organic-rich estuary: the importance of allochthonous dissolved organic matter. Mar. Chem. 88, 21–39. doi: 10.1016/j.marchem.2004.03.001
Sholkovitz, E. R., Sedwick, P. N., and Church, T. M. (2010). On the fractional solubility of copper in marine aerosols: toxicity of aeolian copper revisited. Geophys. Res. Lett. 37:44817. doi: 10.1029/2010GL044817
Sholkovitz, E. R., Sedwick, P. N., Church, T. M., Baker, A. R., and Powell, C. F. (2012). Fractional solubility of aerosol iron: synthesis of a global scale data set. Geochim. Cosmochim. Acta 89, 173–189. doi: 10.1016/j.gca.2012.04.022
Stauber, J. L., and Florence, T. M. (1985). The influence of iron on copper toxicity to the marine diatom, Nitzschia closterium. Aquat. Toxicol. 6, 297–305. doi: 10.1016/0166-445X(85)90025-6
Stockdale, A., Tipping, E., and Lofts, S. (2015). Dissolved trace metal speciation in estuarine and coastal waters: comparison of WHAM/Model VII predictions with analytical results. Environ. Toxicol. Chem. 34, 53–63. doi: 10.1002/etc.2789
Stuart, R. K., Brahamsha, B., Busby, K., and Palenik, B. (2013). Genomic island genes in a coastal marine Synechococcus strain confer enhanced tolerance to copper and oxidative stress. ISME J. 7:1139. doi: 10.1038/ismej.2012.175
Stuart, R. K., Bundy, R., Buck, K., Ghassemain, M., Barbeau, K., and Palenik, B. (2017). Copper toxicity response influences mesotrophic Synechococcus community structure: Marine Synechococcus copper response. Environ. Microbiol. 19, 756–769. doi: 10.1111/1462-2920.13630
Stuart, R. K., Dupont, C. L., Johnson, D. A., Paulsen, I. T., and Palenik, B. (2009). Coastal strains of marine Synechococcus species exhibit increased tolerance to copper shock and a distinctive transcriptional response relative to those of open-ocean strains. Appl. Environ. Microb. 75, 5047–5057. doi: 10.1128/AEM.00271-09
Sunda, W. (1976). The Relationship Between Cupric Ion Activity and the Toxicity of Copper to Phytoplankton. [Dissertation]. Cambridge (MA): Massachusetts Institute of Technology. doi: 10.1575/1912/1275
Sunda, W. G., and Huntsman, S. A. (1983). Effect of competitive interactions between manganese and copper on cellular manganese and growth in estuarine and oceanic species of the diatom Thalassiosira. Limnol. Oceanogr. 28, 924–934. doi: 10.4319/lo.1983.28.5.0924
Sunda, W. G., and Huntsman, S. A. (1998). Interactive effects of external manganese, the toxic metals copper and zinc, and light in controlling cellular manganese and growth in a coastal diatom. Limnol. Oceanogr. 43, 1467–1475. doi: 10.4319/lo.1998.43.7.1467
Sunda, W. G., Price, N. M., and Morel, F. M. M. (2005). “Trace metal ion buffers and their use in culture studies,” in Algal Culturing Techniques, ed. R. A. Anderson (New York, NY: Elsevier), 35–64.
Tang, D., Shafer, M. M., Karner, D. A., and Armstrong, D. E. (2005). Response of nonprotein thiols to copper stress and extracellular release of glutathione in the diatom Thalassiosira weissflogii. Limnol. Oceanogr. 50, 516–525. doi: 10.4319/lo.2005.50.2.0516
Tottey, S., Patterson, C. J., Banci, L., Bertini, I., Felli, I. C., Pavelkova, A., et al. (2012). Cyanobacterial metallochaperone inhibits deleterious side reactions of copper. P. Natl. Acad. Sci. 109, 95–100. doi: 10.1073/pnas.1117515109
Trapp, J. M., Millero, F. J., and Prospero, J. M. (2010). Trends in the solubility of iron in dust-dominated aerosols in the equatorial Atlantic trade winds: importance of iron speciation and sources. Geochem. Geophy. Geosy. 11:3. doi: 10.1029/2009GC002651
Vasseur, P., Pandard, P., and Burnel, D. (1988). Influence of some experimental factors on metal toxicity to Selenastrum capricornutum. Toxic Assess 3, 331–343. doi: 10.1002/tox.2540030308
Wang, D., Xia, W., Kumar, K. S., and Gao, K. (2017). Increasing copper alters cellular elemental composition (P and Mo) of marine diatom. Ecol. Evol. 7, 3362–3371. doi: 10.1002/ece3.2890
Wang, F. J., Chen, Y., Guo, Z. G., Gao, H. W., Mackey, K. R., Yao, X. H., et al. (2017). Combined effects of iron and copper from atmospheric dry deposition on ocean productivity: aerosol Fe and Cu affect productivity. Geophys. Res. Lett. 44:5. doi: 10.1002/2016GL072349
Webb, E. A., Ehrenreich, I. M., Brown, S. L., Valois, F. W., and Waterbury, J. B. (2009). Phenotypic and genotypic characterization of multiple strains of the diazotrophic cyanobacterium, Crocosphaera watsonii, isolated from the open ocean. Environ. Microbiol. 11, 338–348. doi: 10.1111/j.1462-2920.2008.01771.x
Wells, M. L., Trick, C. G., Cochlan, W. P., Hughes, M. P., and Trainer, V. L. (2005). Domoic acid: the synergy of iron, copper, and the toxicity of diatoms. Limnol. Oceanogr. 50, 1908–1917. doi: 10.4319/lo.2005.50.6.1908
Wiramanaden, C. I. E., Cullen, J. T., Ross, A. R. S., and Orians, K. J. (2008). Cyanobacterial copper-binding ligands isolated from artificial seawater cultures. Mar. Chem. 110, 28–41. doi: 10.1016/j.marchem.2008.02.003
Keywords: copper, toxicity, phytoplankton, speciation, Crocosphaera watsonii
Citation: Lopez JS, Lee L and Mackey KRM (2019) The Toxicity of Copper to Crocosphaera watsonii and Other Marine Phytoplankton: A Systematic Review. Front. Mar. Sci. 5:511. doi: 10.3389/fmars.2018.00511
Received: 17 May 2018; Accepted: 21 December 2018;
Published: 24 January 2019.
Edited by:
Antonio Tovar-Sanchez, Spanish National Research Council (CSIC), SpainReviewed by:
Benjamin Twining, Bigelow Laboratory For Ocean Sciences, United StatesHajime Obata, The University of Tokyo, Japan
Copyright © 2019 Lopez, Lee and Mackey. This is an open-access article distributed under the terms of the Creative Commons Attribution License (CC BY). The use, distribution or reproduction in other forums is permitted, provided the original author(s) and the copyright owner(s) are credited and that the original publication in this journal is cited, in accordance with accepted academic practice. No use, distribution or reproduction is permitted which does not comply with these terms.
*Correspondence: Johann S. Lopez, am9oYW5ubEB1Y2kuZWR1