- 1Department of Biology, The College of New Jersey, Ewing, NJ, United States
- 2Department of Physics and Astronomy, Colgate University, Hamilton, NY, United States
- 3Marine Science and Conservation, Duke University Marine Laboratory, Beaufort, NC, United States
Barnacles are dominant members of marine intertidal communities. Their success depends on firm attachment provided by their proteinaceous adhesive and protection imparted by their calcified shell plates. Little is known about how variations in the environment affect adhesion and shell formation processes in barnacles. Increased levels of atmospheric CO2 have led to a reduction in the pH of ocean waters (i.e., ocean acidification), a trend that is expected to continue into the future. Here, we assessed if a reduction in seawater pH, at levels predicted within the next 200 years, would alter physiology, adhesion, and shell formation in the cosmopolitan barnacle Amphibalanus (=Balanus) amphitrite. Juvenile barnacles, settled on silicone substrates, were exposed to one of three static levels of pHT, 8.01, 7.78, or 7.50, for 13 weeks. We found that barnacles were robust to reduced pH, with no effect of pH on physiological metrics (mortality, tissue mass, and presence of eggs). Likewise, adhesive properties (adhesion strength and adhesive plaque gross morphology) were not affected by reduced pH. Shell formation, however, was affected by seawater pH. Shell mass and base plate area were higher in barnacles exposed to reduced pH; barnacles grown at pHT 8.01 exhibited approximately 30% lower shell mass and 20% smaller base plate area as compared to those at pHT 7.50 or 7.78. Enhanced growth at reduced pH appears to be driven by the increased size of the calcite crystals that comprise the shell. Despite enhanced growth, mechanical properties of the base plate (but not the parietal plates) were compromised at the lowest pH level. Barnacle base plates at pHT 7.50 broke more easily and crack propagation, measured through microhardness testing, was significantly affected by seawater pH. Other shell metrics (plate thickness, relative crystallinity, and atomic disorder) were not affected by seawater pH. Hence, a reduction in pH resulted in larger barnacles but with base plates that would crack more readily. It is yet to be determined if such changes would alter the survival of A. amphitrite in the field, but changes in the abundance of this ecologically dominant species would undoubtedly affect the composition of biofouling communities.
Introduction
Barnacles are dominant members of marine biofouling communities throughout much of the world’s oceans. They settle and tenaciously adhere to nearly any inert surface in the marine environment, including ship hulls and maritime facilities, and once established can serve as a substrate for less tenacious species. This results in a tremendous cost burden for Naval and maritime industries in the form of coating application, cleaning and maintenance, as well as lost operational time (Callow and Callow, 2011; Schultz et al., 2011).
The success of Balanomorph barnacles depends on the firm attachment provided by their secreted adhesive and the protection imparted by their heavily calcified outer shell plates. Adult barnacles adhere using a largely proteinaceous glue which forms adhesive bonds with surfaces and cures (Walker, 1972; Naldrett, 1993; Kamino et al., 2000; Kamino, 2008). The glue is comprised of at least ten major proteins, which are thought to play differing but specific roles in the adhesion process, including displacement of water from the substratum, integrating the cement with the base plate, adsorption to the substratum, assembly, and curing (Kamino, 2016; So et al., 2016). Shell calcification in juvenile barnacles occurs soon after metamorphosis (LeFurgey et al., 1995). In the genus Amphibalanus, barnacles are protected by six parietal (i.e., lateral) shell plates that sit atop a calcified basal plate (Pitombo, 2004). Shell plates are composed of calcite, held within a matrix of chitin, acidic proteins, and sulfate-rich polymers (Fernandez et al., 2002; Rodriguez-Navarro et al., 2006; Khalifa et al., 2011). The base plate, and a narrow uncalcified growth region, are glued to the substrate. The calcified base plate is composed of layered structures, with grain size of calcite crystallites increasing with distance from the glue layer (Lewis et al., 2014; De Gregorio et al., 2015).
Although we are beginning to understand the biochemical mechanisms involved in barnacle adhesion and shell formation, relatively little is known about how variations in the environment, for example in seawater pH, affect barnacle adhesion and shell formation. Daily, monthly, and seasonal fluctuations in pH have been well-documented in coastal and intertidal habitats and are typically attributed to cycles of photosynthesis and respiration (Truchot and Duhamel-Jouve, 1980; Morris and Taylor, 1983; Wootton et al., 2008; Moulin et al., 2011; Baumann et al., 2015). Assessing the effects of seawater pH is particularly relevant given current and predicted changes in the pH of ocean waters, a process known as ocean acidification (OA). Resulting from the absorption of CO2 by the world’s oceans, the pH of global surface waters has decreased by 0.1 pH units since the industrial revolution and is projected to drop a further 0.3 – 0.5 pH units by the year 2100 (Caldeira and Wickett, 2003; Doney et al., 2009). Such changes will be more extreme in coastal regions, due to a decreased buffering capacity of coastal waters and biological CO2 production (Waldbusser et al., 2011; Baumann et al., 2015). Effects of reduced seawater pH or associated changes in carbonate chemistry (i.e., reduced calcium carbonate saturation states) have previously been found to affect adhesion and shell formation in other marine invertebrates. For example, in marine mussels reduced pH led to diminished attachment strength and changes in the expression of proteins that comprise the byssus and adhesive plaques (O’Donnell et al., 2013; Zhao et al., 2017). Alterations in calcification, growth, and shell properties resulting from decreased pH or calcium carbonate saturation states have been documented in a broad range of calcifying organisms (Kroeker et al., 2010; Kroeker et al., 2013).
Given the general sensitivity of protein conformation to pH, and evidence of a reduction in marine mussel attachment with reduced pH, we hypothesized that the barnacle adhesive system is sensitive to seawater pH. Specifically, we predicted that barnacles grown under different levels of pH would vary in: (1) adhesive strength, and (2) gross morphology of the adhesive plaque (i.e., whether the adhesive plaque was thin and transparent, or thick and opaque when grown on silicone: Berglin and Gatenholm, 2003; Wiegemann and Watermann, 2003; Holm et al., 2005). The effect of reduced pH (to pHNBS 7.4) on barnacle adhesion strength was tested previously in the barnacle Amphibalanus (=Balanus) amphitrite by McDonald et al. (2009), but in this experiment, barnacles were grown on glass and all barnacles broke upon removal. Therefore, force recordings reflected mechanical properties of the shell rather than adhesive properties per se. Here, we assessed if pH affects barnacle adhesion strength when barnacles were grown on silicone coatings and measured following the ASTM “Standard test method for measurement of barnacle adhesion strength in shear” (ASTM International, 2005). In this test, barnacles whose shells break during removal are excluded from analysis, and therefore force measurements are solely dependent on properties of the adhesive bond with the substrate and cohesive properties of the glue itself.
Interestingly, McDonald et al. (2009) observed an overall increase in exoskeleton calcification at pHNBS 7.4 as compared to the ambient level of 8.2, despite weakened mechanical strength of the parietal plates in barnacles exposed to low pH. We predicted that alterations in the shell formation and maintenance processes in barnacles would occur even under more moderately reduced seawater pH. Specifically, we tested the shell size, mass, and plate thickness of barnacles exposed to seawater at pHT (total H+ concentration scale) levels of 8.01, 7.78, and 7.50. These values are common targets in OA studies and approximate the current average for oceanic surface waters (8.01) and predicted global averages for oceanic surface waters in the years ∼2100 and ∼2200, respectively (Caldeira and Wickett, 2003; Doney et al., 2009). Although oceanic pH is a dynamic parameter, which can be influenced by a large number of biotic and abiotic factors (Riebesell et al., 2011), pHT values of 8.01, 7.78 and 7.50 roughly correspond to current atmospheric pCO2 levels (∼400 μatm), twice the current level (∼800 μatm), and four times the current level (∼1600 μatm), respectively. Shell mechanical properties for barnacles grown at these pH levels were assessed in both the base and parietal plates using microhardness testing. Further, using a combination of SEM and FTIR spectroscopy, we tested if alterations in calcification or mechanical properties are driven by changes in ultrastructure, composition, crystallinity, or atomic disorder of the shell plates.
To address these predictions, we exposed juvenile barnacles, Amphibalanus (=Balanus) amphitrite, to one of three static pHT levels (8.01, 7.78, or 7.50) for a total of 13 weeks. A. amphitrite is a cosmopolitan intertidal species, inhabiting tropical and semi-tropical waters, and both larvae and adults of this species have been found to tolerate reduced pH (McDonald et al., 2009). Therefore, in addition to the adhesion and shell formation metrics described, we also assessed if pH affects the general physiology of A. amphitrite. This was done by monitoring mortality throughout the exposure period and assessing tissue mass and the presence of eggs at the conclusion of the experiment. Overall, the goal of this work is to provide a comprehensive assessment of the effect of seawater pH on A. amphitrite; alterations in the presence or abundance of this common species could alter the composition of intertidal and biofouling communities.
Materials and Methods
Animal Collection, Larval Culture, and Experimental Exposure
Barnacle larvae were reared from field-collected adult barnacles following the methods of Rittschof et al. (1984, 1992, 2008). Barnacle cyprid larvae were settled on T2 silicone-coated glass panels (15.2 × 7.6 cm) on July 22, 2015. At 11 days post-settlement, barnacles were shipped overnight mail to The College of New Jersey (TCNJ; Ewing, NJ, United States) and placed individually in 1 L plastic bins filled with Artificial Seawater (Instant Ocean, mixed to a salinity of 35).
Panels with juvenile barnacles were randomly assigned to one of three target pHT treatments, 8.01, 7.78, or 7.50, with a total of 8 panels per pH treatment. Replicate panels were split evenly between two, replicate 5 gallon glass aquaria per pH treatment. Panels were placed into their assigned pH treatment on August 19, 2015 (26 days post-settlement). To prevent accumulation of organic matter in tanks and to enhance feeding, panels were removed from the aquaria once a day, 6 days a week, for feeding. Panels were placed individually in 1 L plastic bins (12.5 × 10.5 × 10.5 cm) that had been filled with seawater from the specific aquarium from which that panel had been taken. A dense solution of brine shrimp (Artemia sp.) was distributed evenly among bins using a serological pipette and barnacles fed for approximately an hour per day. Additional detail on feeding can be found in the Supplementary Material. The experimental exposure lasted for 13 weeks (91 days).
Adjustment and Monitoring of Seawater Conditions
Experimental exposures at TCNJ were run in artificial seawater (Instant Ocean), mixed to a salinity of 35. Since Instant Ocean is formulated with total alkalinity (TA) above what is found in natural seawater (SW), TA was reduced to ∼2200 μmol kg-1 SW by addition of 12 M HCl (Lunden et al., 2014). The value of ∼2200 μmol kg-1 SW was chosen to approximate typical TA values in Beaufort, NC where the barnacle broodstock was collected. The TA of artificial seawater was measured following SOP 3b (Dickson et al., 2007) on an automated titrator (Hanna Instruments, HI902) with 0.1 M HCl (Fluke #35335, certified volumetric) as a titrant, and values were checked against certified reference material from the Dickson Laboratory (Scripps Institution of Oceanography, La Jolla, CA, United States). All TA samples were run at least in duplicate.
Target pH and temperature levels were achieved using an automated aquarium control system (Apex AquaController, Neptune Systems), which functioned as both a pHstat and thermostat. Temperature was held at 25°C for all aquaria. Each aquarium was equipped with a temperature probe (Neptune Systems, Extended Life Temperature Probe) and a 50-watt submersible aquarium heater (Aqueon 06105 Pro). Each tank was also equipped with a pH probe (Neptune Systems, Lab Grade pH Probe). Seawater pH was brought to the set point for each aquarium (8.01, 7.78, or 7.50) by addition of pure CO2 gas (AirGas, food grade) or CO2-free air. Water within each aquarium was continuously circulated using an 80 GPH (gallons per hour) submersible aquarium pump (Patuoxun 80 GPH Submersible Pump).
Seawater conditions (salinity, pHT, and temperature) were measured 6 days per week using a handheld multiparameter meter (YSI, Professional Plus). A summary of seawater conditions is provided in Table 1 and reflects multiparameter meter readings. TA was measured weekly as described above. To ensure consistency of temperature and pH monitoring among replicate aquaria, the aquarium control system was calibrated against the multiparameter meter readings. Additional detail on the experimental exposure and monitoring seawater conditions can be found in the Supplementary Material.
Adhesion and Growth Assessments
Adhesion strength in shear (critical shear stress) was measured in all barnacles after 13 weeks exposure. Adhesion testing followed ASTM D 5618-94 (ASTM International, 2005). Barnacles were removed from panels using a hand-held digital force gauge (Shimpo, FGE-5X). Force values were discarded if damage to the barnacle shell or to the silicone panel occurred during removal. To enable determination of base plate area, base plate diameter was measured on each barnacle in two dimensions (along and perpendicular to the operculum) using a digital caliper. Removal force values were normalized to base plate area. The height of each barnacle was also measured with a digital caliper as the perpendicular distance from the bottom of the base plate to the top of the highest parietal plate. Lastly, the presence or absence of gummy, opaque glue (as described in Berglin and Gatenholm, 2003; Wiegemann and Watermann, 2003; Holm et al., 2005) was recorded for each barnacle.
Following assessments, barnacles from each panel were placed in shallow glass finger bowls with seawater taken from the aquarium in which that panel had been held. Within 72 h of removal, barnacles were individually removed from water and dissected to remove the soft body from the shell plates. Opercular plates were removed from the soft tissue and were not included in subsequent analyses. Dissected barnacle bodies were placed individually on pre-weighed pieces of weigh paper, allowed to dry for 48 h at room temperature and then dried in a vacuum oven at 45°C, 25 in. Hg. Dried barnacle bodies were weighed individually on an analytical balance with 0.02 mg precision (Metler-Toledo, XSE105DU) to determine tissue mass. During dissections, the presence or absence of eggs within the mantle cavity was recorded. Eggs, if present, were removed from the mantle cavity but were not included in tissue mass measurements. Remaining barnacle shells (base and all parietal plates) were then cleaned thoroughly with water to ensure all tissue had been removed. Shells were dried overnight at room temperature, then dried in a vacuum oven at 45°C, 25 in. Hg, for 24 h, and then weighed individually on an analytical balance with 0.02 mg precision. Visibly damaged shells were not included in shell mass measurements.
Structural and Mechanical Assessments
All barnacle shells were inspected after shell mass measurements using a stereomicroscope (Leica, S8Apo) and any damage to the base plate that had not been observed by eye (e.g., micro-scale cracks or holes) was recorded. Two undamaged barnacle shells per panel (16 per pH treatment) were then randomly selected for structural and micromechanical assessments. These assessments required embedding and polishing of the shell as shown in Figure 1 and as described in the Supplementary Material.
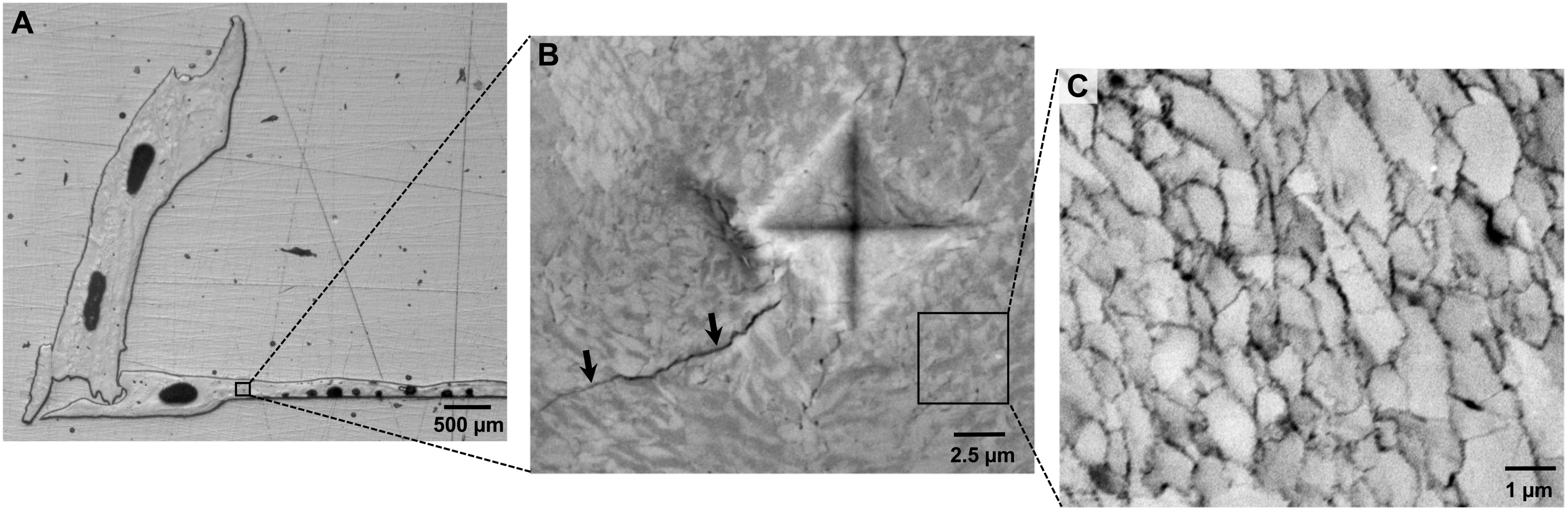
FIGURE 1. (A) Assessments of barnacle shell properties were conducted on polished cross-sections of individual barnacle shells, which exposed both the base and parietal plates. (B) Mechanical testing was conducted using microindentation, which results in a diamond shaped indent and induces crack formation (arrows). Image was taken under polarized light, revealing organization of calcite crystals. (C) Shell ultrastructure was assessed using SEM. Shells are comprised of a cobblestone-like network of calcite crystallites, the area of which can be measured using image analysis software.
Thickness of barnacle shell plates was measured on polished shell cross-sections using an upright reflected light microscope (Zeiss AxioScope.A1) equipped with a digital camera (Zeiss Axiocam 105 color). Images were taken of the entire shell cross-section at 2.5 X magnification. Typically, this required 2–4 images per sample, depending on the size of the barnacle. Using the camera’s analysis software (Zeiss Zen 2), a 100 × 100 μm grid was placed on each image. Thickness of the base or parietal plate was measured at each point the grid crossed the plate, resulting in about 40 measurements per shell plate. For the base plate, replicate measurements were averaged to determine the mean base plate thickness for each sample. At least two separate parietal plates were visible in each sample, and measurements were taken within all plates. Replicate measurements were combined among parietal plates, and values were averaged to determine the mean parietal plate thickness for each sample.
Micromechanical properties were quantified using a microindentation hardness tester (Mitutoyo HM-200). Indents were made at 20 g load, 5 s dwell time. Testing was conducted in the base and one of the parietal plates, with 10 replicate indents made in each plate. For the base plate, 5 indents were made on each side of the plate, spaced about 200 μm apart, starting at approximately 500 μm from the distal edge of the plate on each of the two sides (Figure 1A). Replicate indents were typically made throughout the length of a single parietal plate. Individual indents were measured directly on the hardness tester at 50 X magnification in two dimensions, and Vickers microhardness values were automatically calculated. An image of each indent was taken on the hardness tester using a digital microscope camera (Moticam 2.0MP), which enabled quantification of crack propagation (Figure 1B). Crack length was determined as the radius of a circle emanating from the center of the indent and encompassing all visible cracks. Replicate measurements within a shell plate were averaged to determine the mean microhardness and crack propagation for each shell plate.
SEM Imaging, Elemental Analysis, and Calcite Crystal Area Assessment
After micromechanical testing, SEM imaging was conducted on all polished shell cross-sections. Imaging was conducted on uncoated samples, at low vacuum (50 Pa), in back-scattered electron mode on a field emission SEM (Hitachi America, SU5000). An accelerating voltage of 15 kV was used at a working distance of approximately 8 μm. For each sample, images were taken within two separate regions of the base plate, and two regions of one of the parietal plates. In all cases, images were taken in close proximity (typically within 100 μm) to the indents made during micromechanical testing. Images were taken at 1,000 and 5,000X magnification.
Elemental analysis was conducted on all samples at 1,000X magnification using an EDAX EDS (energy dispersive X-ray spectroscopy) detector (AMTEK Materials Analysis Division, Octane Plus). Imaging conditions resulted in a count rate of 5,000–10,000 counts per second. For each region, a total of five point spectra were taken across the region. Replicate spectra were averaged within and between the two regions per plate, to determine the mean elemental composition for each shell plate.
Individual calcite crystals were readily resolved at 5000X magnification (Figure 1C). The area of individual calcite crystals was determined on SEM images using image analysis software (ImageJ, Ver. 1.49). For each image, a total of 15 different calcite crystals were randomly selected for area determination. For selection, a 2 μm2 grid was first placed on the image. Pairs of random numbers were generated using a random number generator, and each pair was used as coordinates to identify a specific calcite crystal on the image. The perimeter of each identified crystal was traced by hand using the polygon tool in ImageJ and area within the traced region was automatically determined. One SEM image per shell plate was assessed, and replicate area measurements within the image were averaged to provide a mean crystal area for each shell plate.
FTIR Analysis
FTIR spectroscopy was used to assess: (1) the polymorph of calcium carbonate present in barnacle shells; (2) relative crystallinity of shells and; (3) atomic disorder of shells. Spectra were collected for polymorph and relative crystallinity determination using a PerkinElmer Spectrum Two spectrometer and for atomic disorder using a PerkinElmer Spectrum 100 spectrometer. In all cases, individual barnacle shells that had been cleaned of soft tissue were powdered using a mortar and pestle. For these assessments, the base and all parietal plates were included for each sample, but the opercular plates had been removed. Powdered sample was placed directly on the instrument’s ATR (attenuated total reflectance) crystal and compressed to a uniform force with a built-in anvil. Spectra were taken at 4 wavenumbers resolution, with 32 scans per sample. Spectra were normalized and baseline corrected within the 600–2000 cm-1 region. The ratio of ν2 to ν4 peak absorbance was used as a measure of crystallinity of the shells (Beniash et al., 1997). For atomic disorder assessments, each sample was ground into a coarse powder for the first spectrum. Following the first spectrum the sample was then ground with the mortar and pestle to make a slightly finer powder, and a second spectrum was acquired. This process was carried out 5–8 times, depending on how much the spectrum changed after each subsequent grind. Each spectrum was ATR corrected and background removed before peak height measurements were acquired following Regev et al. (2010).
Statistical Analysis
Statistical analyses were conducted using SPSS (V. 23, IBM Analytics). Categorical data (mortality, presence of eggs, expression of gummy glue) were assessed using chi-square tests. All other data were assessed using one-way ANOVA (analysis of variance) followed by Tukey HSD post hoc testing. Prior to analyses, outliers were calculated for all metrics as values greater than three times the interquartile range above or below the third or first quartile, respectively, and were removed from the dataset. Following removal of outliers, assumptions of normality and equal variance were assessed using Shapiro–Wilk and Levene tests, respectively, and data were log transformed if necessary to meet these assumptions. If assumptions of normality or equal variance could not be met after log transforming data, a non-parametric Kruskal–Wallis test was used in place of the parametric ANOVA. In all cases, individual barnacles within a pH treatment were pooled among panels and tanks and treated as individual replicates. Testing for both panel and tank effects was conducted for metrics yielding a significant response to pH. No significant panel or tank effects were found.
Results
Seawater Chemistry
A summary of seawater chemistry over the 13 week exposure is provided in Table 1. pH targets were met in all treatments throughout the duration of the exposure. As expected, pCO2 increased with decreasing pH. Seawater remained supersaturated with respect to calcite for all treatments. Total alkalinity tended to be higher in pH 7.50 aquaria as compared to those at pH 7.78 and 8.01.
Physiology
Mortality of barnacles was low and variable throughout the experimental exposure and was not significantly influenced by pH treatment (chi-square: p > 0.05). Cumulative mortality was 21.0, 25.3, and 14.7% for the 7.50, 7.78, and 8.01 pH treatments, respectively. The value for the 8.01 treatment excludes a single panel in which 85% of barnacles died during the third week of the exposure. The reason for this die-off is unknown, but given that this level of mortality was not observed in any of the other panels, it is unlikely that this was driven by pH treatment.
Barnacles were dissected at the conclusion of the experiment, enabling quantification of soft body tissue mass and identification of eggs within the mantle cavity. The effect of pH treatment on tissue dry mass was not significant (Tables 2, 3). At the conclusion of the experiment, nearly all barnacles had eggs within the mantle cavity (96.4, 95.2, and 94.6% of barnacles in the 7.50, 7.78, and 8.01 pH treatments, respectively); the proportion of ovigerous barnacles was not affected by pH treatment (chi-square: p > 0.05).
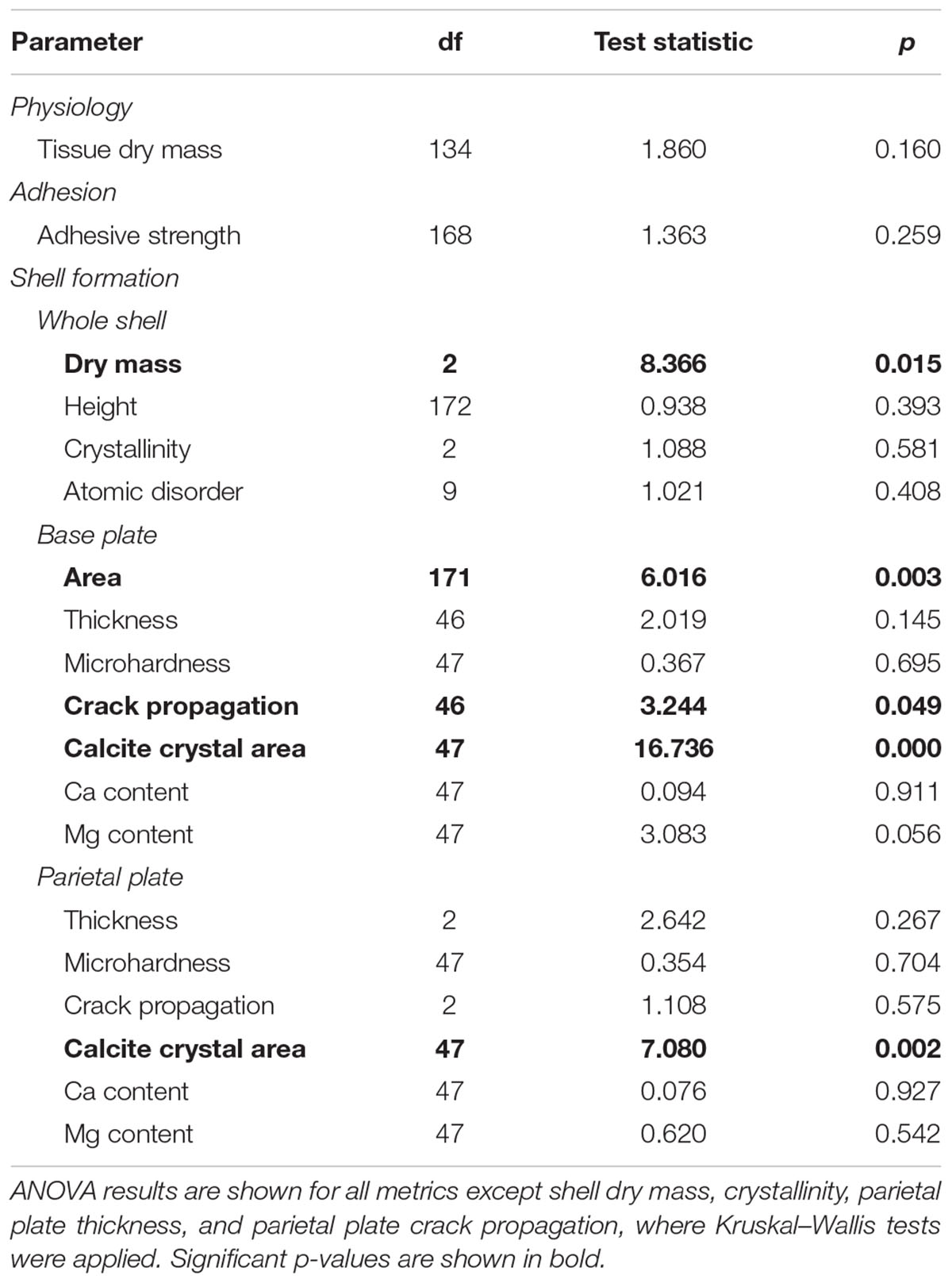
TABLE 2. Assessment of the effects of seawater pH on physiology, adhesion, and shell formation in the barnacle Amphibalanus amphitrite.
Adhesion
Adhesion strength, measured as critical shear force, was assessed after the 13 week exposure. Adhesion strength was not affected by pH treatment (Tables 2, 3). Likewise, the proportion of barnacles expressing opaque, gummy glue was not affected by pH treatment (chi-square: p > 0.05). Gummy glue was found on the base of most barnacles, with 72.9, 80.8, and 62.9% of barnacles exhibiting gummy glue in the 7.50, 7.78, and 8.01 pH treatments, respectively.
Shell Formation
Assessments of shell growth and materials properties were conducted following adhesion assays. Exposure pH was found to significantly affect barnacle shell mass and the area of the base plate (Tables 2, 3 and Supplementary Figure S1). Barnacles grown at pH 8.01 exhibited approximately 30% lower shell mass and 20% smaller base plate area as compared to barnacles grown at pH 7.50 or 7.78. Height of the barnacles, measured from the base plate to the highest parietal plate, was not affected by exposure pH (Tables 2, 3). Shell thickness was measured in both the base and parietal plates on polished shell cross-sections (see Figure 1A). Exposure pH did not significantly influence thickness in either the base or parietal plates. SEM imaging of polished cross-sections revealed a cobblestone like composite of calcite crystals (Figure 1C). Exposure pH was found to significantly alter calcite crystal area in both the base and parietal plates (Tables 2, 3 an Supplementary Figure S1). In the base plate, calcite crystals were approximately 95% smaller in barnacles grown at pH 8.01 as compared to barnacles grown at pH 7.50 or 7.78. The difference in calcite crystal area was less pronounced in the parietal plates, but on average calcite crystals were 35 and 23% smaller in barnacles grown at pH 8.01 as compared to barnacles grown at pH 7.50 or 7.78, respectively.
Qualitative assessments of barnacle base plates following adhesion testing and dissection suggested that damage to the base plate (identified by the presence of cracks or holes) occurred more often in barnacles exposed to pH 7.50 as compared to those exposed to pH 7.78 or 8.01. Base plate damage was identified in 38.6% of barnacles grown at pH 7.50 as compared to 21.3 and 18.8% at pH 7.78 and 8.01, respectively. Rigorous assessments of micromechanical properties were conducted on polished shell cross-sections. Mechanical testing revealed that microhardness was not affected by exposure pH in the base or parietal plates (Tables 2, 3). A significant overall effect of pH on crack propagation, however, was observed when tested in the base plate (Table 2 and Supplementary Figure S1). Within the base plate, cracks radiating from indents were, on average, 28% longer in barnacles exposed to pH 7.50 as compared to those at pH 8.01 (Figure 2; Tukey HSD: p = 0.058). Crack propagation was not affected by exposure pH when tested in the parietal plates.
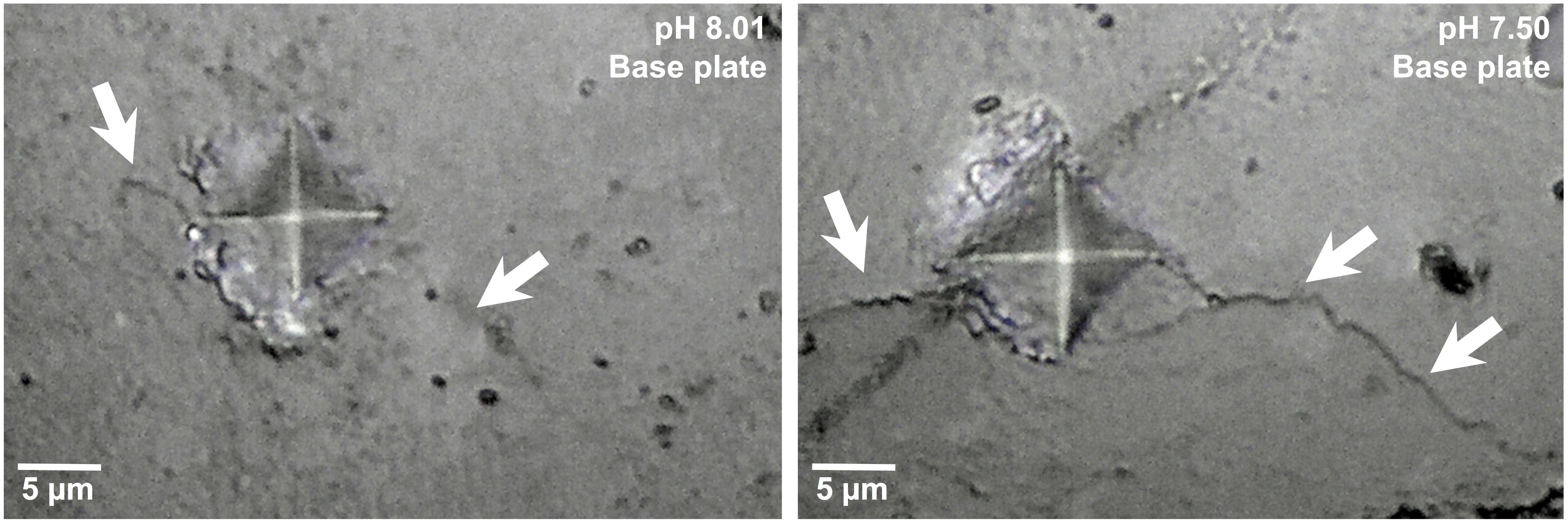
FIGURE 2. Indents and cracks (arrows) resulting from mechanical testing within the base plate of a barnacle (A. amphitrite) held as pH 8.01 (left) and 7.50 (right).
FTIR spectroscopy was used to assess the polymorph of calcium carbonate present in barnacle shells, as well as their relative crystallinity and atomic disorder. Spectroscopy was conducted on powdered shell samples and both base and all parietal plates were included in each sample. At all pH levels, identified peaks were characteristic of calcite with no other polymorphs of calcium carbonate present (Figure 3). Relative crystallinity of the shells (assessed as the ratio of the ν2 to ν4 absorbance) was not affected by pH (Tables 2, 3). Atomic disorder, assessed through FTIR grinding curves, varied among samples and was not significantly affected by pH (Tables 2, 3).
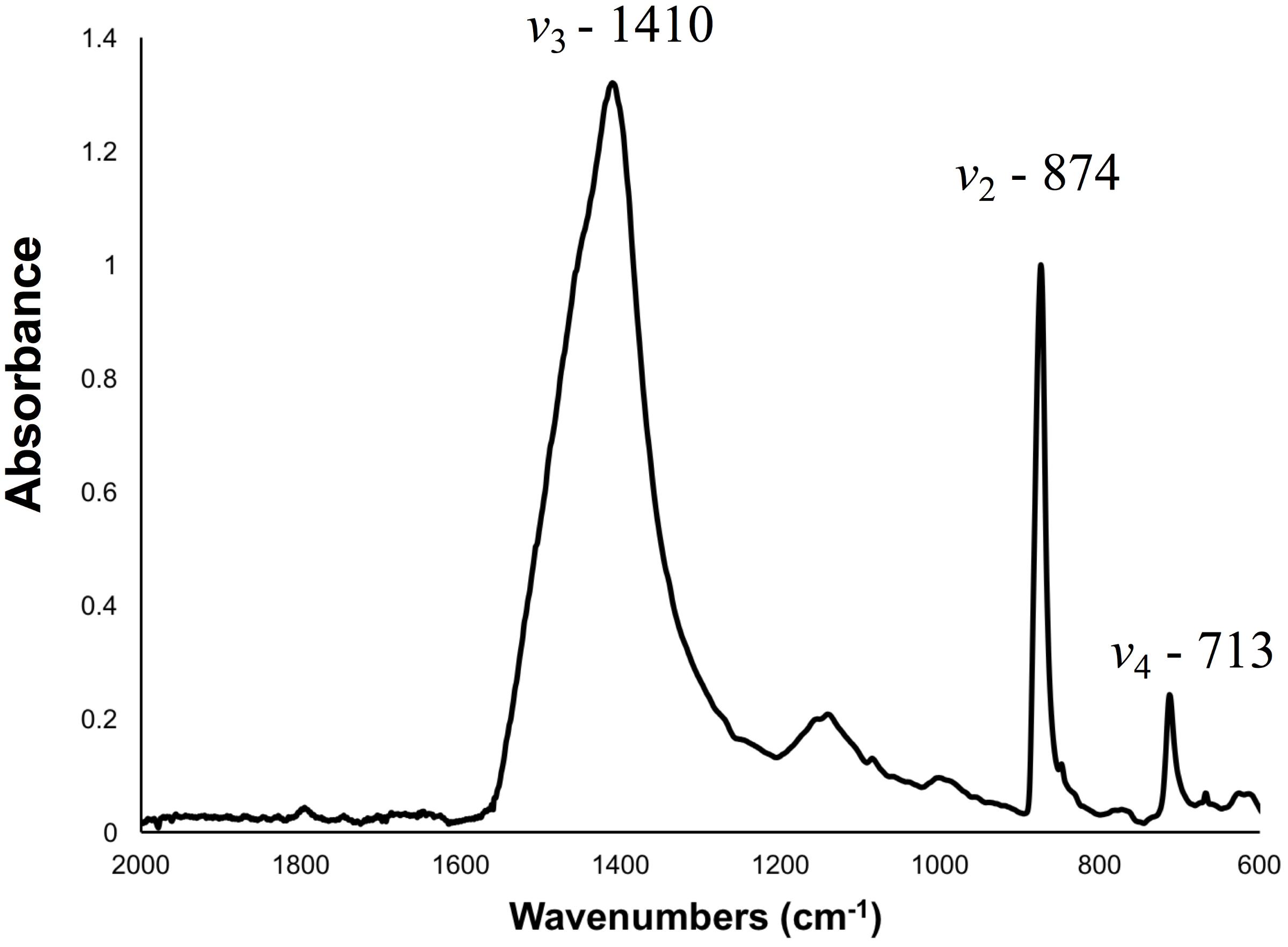
FIGURE 3. Representative FTIR spectra of a powdered barnacle (A. amphitrite) shell with peaks characteristic of calcite. The ν2, ν3, and ν4 peaks, used for crystallinity and atomic order assessments, are labeled.
Elemental composition was assessed on polished shell cross-sections using EDS. Eight elements were identified in all samples: in order of abundance, Ca, O, C, S, Sr, Mg, Na, and Cl. The vast majority of the shell was comprised of Ca, O and C, with all other elements occurring at less than 1 wt %. Exposure pH did not affect calcium content when assessed in the base or parietal plates (Tables 2, 3). While no elements showed a significant effect of pH, magnesium content tended to be lower (on average by 19%) in barnacles exposed pH 7.50 as compared to those at pH 8.01 (Table 3; Tukey HSD: p = 0.052; Supplementary Figure S1).
Discussion
Barnacles are dominant members of marine intertidal communities and their success depends on both the firm attachment provided by their proteinaceous adhesive and the protection imparted by their calcified shell plates. Here we assessed if a reduction in static seawater pH, at levels predicted within the next 200 years (i.e., to pHT 7.78 and 7.50, based on oceanic projections), would alter physiology, adhesion, and shell formation in the cosmopolitan barnacle A. amphitrite. Changes in the abundance of A. amphitrite could affect the composition of biofouling communities. We found that barnacles were generally robust to reduced pH, with no effect of pH on physiological metrics, and, contrary to our prediction, adhesive properties were not affected by reduced pH. Shell mass and base plate area were found to be higher in barnacles exposed to even moderately reduced pH, a trend that appears to be driven by increased size of the calcite crystals that comprise the shell. Although microhardness of the shell plates, a measure of resistance to permanent or plastic deformation, was not affected by pH, the length of cracks propagating from indents in the base plate was, suggesting that the toughness of base plates grown at the lowest pH level was reduced. Hence, a reduction in pH resulted in larger barnacles but with base plates that would crack more readily.
Physiology
Over the course of 13 weeks, we found no effect of seawater pH on cumulative mortality, tissue mass, or egg production in A. amphitrite. Assessments of OA responses in juvenile or adult A. amphitrite are limited, but consistent with our observations, McDonald et al. (2009) found no effect of pH on A. amphitrite egg production after 11 weeks exposure to pHNBS 7.4. Campanati et al. (2016) exposed A. amphitrite larvae to reduced pH (pHNBS 7.6) and tracked survival of juveniles settled from these larvae for 11 days. Mortality was actually reduced at low pH as compared to the pHNBS 8.2 control when assessed at 3 and 9 days post-settlement. At their final time point (11 days post settlement), there was no effect of seawater pH on juvenile survival.
Amphibalanus improvisus (Pansch et al., 2014; Eriander et al., 2016) and Elminius modestus (Findlay et al., 2010a) have also been found to be robust to OA, as least in terms of survival and reproduction. Eriander et al. (2016) found no effect of reduced seawater pH (to pHNBS 7.7) on mortality in A. improvisus over 12 weeks, either when the pH reduction was kept at a stable level or when pH was allowed to fluctuate over the course of the day to mimic diurnal pH cycles. Pansch et al. (2014) similarly found no effect of reduced pH on A. improvisus mortality or reproduction (larval release rate) after 20 weeks in juveniles collected from a field site that shows natural variability in seawater pH (Kiel Fjord, Germany). In contrast though, juveniles collected from a field site with limited variability in seawater pH (Tjärnö Archipelago, Sweden) showed increased mortality at the lowest pH tested (pHNBS 7.2). Exposure of Elminius modestus to reduced pH (pHNBS 7.7) for 30 days did not affect mortality when tested at 14 or 19°C (Findlay et al., 2010a).
Semibalanus balanoides, a boreoarcitic species, appears considerably more sensitive to OA. Increased mortality has been shown in both short (30 days) and longer term (80 or 104 days) exposures to reduced pH (pHNBS 7.7–7.8)(Findlay et al., 2009, 2010a; Harvey and Moore, 2017). Barnacles in these studies were collected near the southern limit of the range for S. balanoides. Similar to what was shown for A. improvisus, environmental conditions of the collection site impacted responses to OA (Findlay et al., 2010b). When Findlay et al. (2010b) exposed S. balanoides collected near the northern limit of their range to reduced pH (to pHNBS 7.7 or 7.3) for 20 days, survival of juveniles did not differ from the pHNBS 8.1 control. Hence, barnacles show a mixed response to seawater pH. Individual responses vary by species as well as within a species based on local conditions of the breeding population.
In general, crustaceans tend to be more tolerant of the effects of OA as compared to other taxa that build a mineralized shell (e.g., mollusks, corals: Kroeker et al., 2013) and such tolerance has been hypothesized to be due to their capacity for ion and acid-base regulation (Melzner et al., 2009; Whiteley, 2011). Populations that are routinely exposed to varying environmental conditions are likely to exhibit a considerable scope for physiological adjustment to a changing environment, making them well-adapted physiologically to changes in seawater pH (Wong et al., 2011; Pansch et al., 2012, 2013). In A. amphitrite larvae for example, expression of energy metabolism related proteins was altered at reduced pH, illustrating the potential for proteomic plasticity in this robust species, and a possible mechanism for mediating the stress of OA (Wong et al., 2011). Variations among species and populations may stem from differences in ion and acid-base regulation ability, as well as differences in the ability to adjust energy metabolism (Whiteley, 2011).
Adhesion
We hypothesized that the barnacle adhesive system is sensitive to seawater pH, and based on this hypothesis, tested the prediction that barnacles grown under different levels of pH would vary in adhesive strength and gross morphology of the adhesive plaque. Previous work with marine mussels (Mytilus trossulus) found a reduction in both strength and extensibility of byssal threads when animals were exposed to reduced pH (tested at a range of pHT values from 8.1 to 7.5: O’Donnell et al., 2013). Such changes in mechanical properties of the byssal threads were calculated to lead to a 35-41% reduction in attachment tenacity, which could substantially affect a mussel’s ability to anchor itself in high energy environments. O’Donnell et al. (2013) suggest that this response is due to sensitivity of DOPA (dihydroxyphenylalanine) to pH. DOPA is a major component of byssal threads that is involved in cross-linking and adhesion. A similar response was found in the mussel Mytilus coruscus (Zhao et al., 2017). Decreased pH (tested at a range of pHNBS values from 8.1 to 7.4) led to a decrease in byssal thread breaking force and toughness, as well as a reduction in the number of threads produced. Incorporating mechanical data with thread counts per mussel, Zhao et al. (2017) predicted a 60–65% reduction in attachment tenacity. Reduced seawater pH also led to significant alteration in expression of byssal thread related genes, thereby providing a mechanism that, in combination with direct effects of pH on DOPA chemistry, could explain the response of byssal threads to reduced pH.
Data collected here did not support the hypothesis that the barnacle adhesive system is sensitive to seawater pH; neither adhesive strength (measured in shear following the ASTM standard for measurement of barnacle adhesive strength: ASTM International, 2005) nor the gross morphology of the adhesive plaque (i.e., if the glue layer was thin and hard or thick and gummy) were affected by seawater pH. A number of factors may contribute to the observed difference in sensitivity to pH between marine mussels and the barnacle (A. amphitrite) tested here. First, the Balanomorph barnacle adhesive system is fundamentally different from that of marine mussels in that the adhesive interface is relatively protected from the external environment. Glue is delivered directly to the substrate at the periphery of the base and parietal plates (Saroyan et al., 1970; Burden et al., 2014). Although the cured glue layer is partially hydrated (Barlow et al., 2009), there is no component of the adhesive system that is constantly exposed to seawater, as is the case for a mussel’s byssal threads. Second, the chemistry of barnacle glue differs from that of marine mussels. Barnacle glue is composed of at least ten major proteins, which play differing but specific roles in the adhesion process (Kamino, 2016; So et al., 2016). DOPA, which is pH sensitive (O’Donnell et al., 2013), has not been identified in barnacle glue (Naldrett, 1993; Kamino et al., 1996; Naldrett and Kaplan, 1997), although evidence of oxidative activity and cross-linking has been found (Dickinson, 2008; Golden et al., 2016; Essock-Burns et al., 2017; So et al., 2017). The sensitivity of isolated barnacle glue proteins to altered pH has yet to be assessed. Last, reduced seawater pH may lead to changes in the suite of glue proteins expressed, either in terms of the specific proteins expressed or the relative abundance of these proteins. Such a mechanism has not been tested in barnacles, but hypothetically could compensate for altered structure and activity of individual glue proteins. Recent advances in sequencing of the barnacle glue proteome (Wang et al., 2015; So et al., 2016) will enable direct assessment of proteomic responses to varied seawater conditions.
In our study, barnacles were settled on silicone substrates, which enabled them to be removed intact. Individuals with broken shells were excluded from analysis, and therefore the response to pH described here reflects only adhesive and cohesive properties of the adhesive plaque. When barnacles were settled on hydrophilic glass beakers, the force required to shear the barnacles from the glass was actually enhanced in barnacles exposed to reduced pH (McDonald et al., 2009). In this case, all barnacles broke upon removal. On hydrophilic substrates, mechanical properties of the shell plates are weaker than the adhesive bond between the glue layer and the substrate, and therefore shear removal measurements reflect integrity of the lower shell plates where the force is applied. McDonald et al. (2009) suggest that thickening of the growing edge of the barnacle was responsible for the observed difference in shear removal force. Clearly surface chemistry, and hence whether failure will occur within the adhesive layer or within the shell plates, will mediate the effect of seawater pH on attachment tenacity. Assessments of barnacle responses to varied pH when grown on substrates that naturally occur in the marine environment may shed light on if OA will affect attachment ability in the field.
Shell Formation
Consistent with what was found previously for A. amphitrite (McDonald et al., 2009), reduced seawater pH resulted in elevated shell formation. We found that even a moderate reduction in seawater pH (to pHT 7.78) resulted in greater shell mass as compared to the pHT 8.01 control. After 13 weeks growth, barnacle base plates were larger at reduced pH. Thickness of shell plates, measured directly on cross-sectioned shells, was not affected by seawater pH, although thickness of the parietal plates did tend to increase with decreasing pH. Enhanced shell formation at reduced seawater pH has been documented in several other crustaceans (i.e., Callinectes sapidus, Penaeus plebejus, Homarus americanus: Ries et al., 2009). Given that bicarbonate ion concentration increases under OA (increased pCO2), enhanced growth in crustaceans may stem from their ability to utilize bicarbonate in the mineralization process (Cameron and Wood, 1985; Whiteley, 2011; Roleda et al., 2012). Enhanced growth though, is not universal in crustaceans (e.g., mixed growth responses have been observed in A. improvisus and S. balanoides: Findlay et al., 2010b; Pansch et al., 2014) and likely depends on an individual’s capacity to mitigate the stress of reduced pH (see Section “Physiology”).
Scanning electron microscopy imaging of cross-sectioned barnacle shells revealed that the shell plates were composed of crystals, with dimensions on the order of 1 μm. FTIR spectroscopy confirmed that these crystallites were composed of calcite with no other polymorphs of calcium carbonate present, and EDS spectroscopy identified both magnesium and strontium within the calcitic shell plates. Consistent with previous assessments of A. amphitrite shells, individual crystallites were irregular in shape, did not take on a uniform orientation, and the boundaries of larger crystals appear rough, suggestive of smaller crystallites on the surface of the larger crystals (Khalifa et al., 2011; Lewis et al., 2014). The organic matrix surrounding calcite crystals is composed of chitin, acidic proteins, and sulfate-rich polymers (Fernandez et al., 2002; Rodriguez-Navarro et al., 2006; Khalifa et al., 2011), and in A. amphitrite comprises approximately 2 wt% of the shell (Khalifa et al., 2011). We did not assess the organic matrix specifically in this study, but observations of a double peak at 1145 cm-1 in the FTIR spectrum (taken on whole, crushed shells) are suggestive of sulfate-rich polymers within the organic matrix (Khalifa et al., 2011).
Although seawater pH did not alter the overall shape or orientation of calcite crystals, the size of individual crystals increased dramatically in barnacles grown at reduced seawater pH. Calcite crystals comprising the base plate were nearly twice as large in barnacles at reduced pH (pHT 7.50 or 7.78) as compared to the pHT 8.01 control. Seawater pH resulted in a graded response in parietal plates, with the largest calcite crystals at the lowest seawater pH, a trend that closely followed parietal plate thickness. Shell formation in barnacles is directed by cells of the mantle epithelium, which participate in deposition of organic matrix and calcium transport (Nousek, 1984; Fernandez et al., 2002; Rodriguez-Navarro et al., 2006; Gohad et al., 2009). Crystal nucleation, structure, and orientation of calcite crystals is proposed to be controlled by the organic matrix (Fernandez et al., 2002; Khalifa et al., 2011).
At present, the mechanisms driving such differences in calcite crystal size are unclear. Increased size of calcite crystals under reduced seawater pH may reflect differences in the process of organic matrix deposition by mantle cells or the rate by which matrix deposition occurs. Similar to what was observed here, an increase in the size of shell microstructures (folia) was observed in eastern oysters (Crassostrea virginica) when exposed to pHNBS 7.5 for 20 weeks (Beniash et al., 2010). In this case it was proposed that energy limitations could impede organic matrix deposition or cell division. Here though, we did not observe an effect of seawater pH on A. amphitrite soft tissue mass, and therefore it does not appear as though animals at reduced pH were functioning under an energy deficit. An alternative hypothesis is that reduced seawater pH led to differences in intracellular pH, which could alter the ability of cells to participate in the mineralization process. In another crustacean (the Tanner crab, Chionoecetes bairdi), a reduction in intracellular pH was observed in animals held at reduced pH, a response that was proposed to have implications on the shell formation process (Meseck et al., 2016). Direct assessments of intracellular pH in barnacles grown at reduced pH as well as further investigation into the role of cells in the shell formation process in barnacles would be helpful in evaluating these mechanisms.
Changes in the shell formation process under reduced pH may have implications in terms of functionality of the shell. The base plates of barnacles grown at the lowest pH tested (pHT 7.50) tended to break more easily than those of barnacles grown at pHT 7.78 or 8.01. Rigorous mechanical testing supported this observation. When tested in the base plate, hardness, a material’s ability to resist plastic or permanent deformation, was not affected by pH, but the cracks that propagated from mechanical tests were longer at low pH, indicating that reduced seawater pH led to a reduction in toughness. This response may partially be driven by the dramatic increase in calcite crystal size at low pH. Larger crystals would imply a lower ratio of organic to inorganic material within the shell. Organic matrix plays an important toughening role in biological materials, serving to trap and deflect cracks (Fratzl et al., 2007; Beniash et al., 2010; Meyers and Chen, 2014). Therefore, as the ratio of organic matrix to inorganic mineral decreases, crack deflection ability is also diminished. This mechanism, however, cannot fully explain the trends observed, given that animals at pHT 7.78 showed larger calcite crystal size but did not exhibit a reduction in base plate toughness.
Several additional factors could contribute to the observed reduction in base plate toughness. Assessment of the shell organic matrix was beyond the scope of this study, but given the ability of the organic matrix to trap and deflect cracks (Fratzl et al., 2007; Meyers and Chen, 2014) alterations in the composition or density of the organic matrix could also lead to changes in toughness. Considering the calcite crystals themselves, two factors could have influenced mechanical properties. First, organic constituents (e.g., amino acids) can be occluded within biogenic calcite crystals and can dramatically impact mechanical properties via alterations in the crystal lattice (Cho et al., 2016; Kim et al., 2016). If the identity or quantity of these organic inclusions was altered at low pH, this could influence shell mechanical properties. Second, inclusion of magnesium into the calcite crystal lattice can have a major impact on mechanical properties of shells, with the addition of even small amounts of magnesium leading to enhanced mechanical properties (Kunitake et al., 2012; Long et al., 2014). Correspondingly, in the base plate of pHT 7.50 barnacles, we observed a trend (p = 0.056) toward decreased magnesium content as compared to animals at pHT 7.78 or 8.01. Such a reduction in magnesium content could contribute to the observed reduction in toughness. At this point it is unclear if the reduced magnesium content at low pH is due to lower uptake and incorporation of magnesium, or increased dissolution of weekly bound magnesium (Findlay et al., 2009).
A reduction in the force needed to break parietal plates was observed previously in A. amphitrite (McDonald et al., 2009) and A. improvisus (Pansch et al., 2013) after exposure to reduced pH. Force needed to break parietal plates was not assessed in this study, but at the micro-scale, we did not observe an effect of seawater pH on parietal plate hardness or crack propagation. Hence differences in force needed to break parietal plates may stem from structural changes in the plates (e.g., local dissolution of mineral: McDonald et al., 2009), rather than differences in their material properties. We did not observe damage or erosion of parietal plates for any of the treatments in this study, although alkalinity was consistently elevated at pHT 7.50, suggesting that some dissolution of shells at this pH may have occurred.
Neither shell crystallinity nor atomic disorder, both measures of the level of structural order within a crystal at the atomic scale, were affected by seawater pH. Changes in formation conditions or the composition of molecules at the time of crystal formation could affect these metrics (Khalifa et al., 2011). Interestingly, although the mean values of these metrics were not significantly affected by seawater pH, variance around the mean was dramatically greater at reduced pH as compared to pHT 8.01 (e.g., for atomic disorder, standard error at reduced pH was 4–5 times greater than at pHT 8.01). This suggests that reduced pH may increase variability among individuals in their ability to control the environment in which mineral forms. A similar response in terms of increased variability in crystal properties among individuals at decreased pH was observed previously in the marine mussel, Mytilus californianus (McCoy et al., 2018).
Conclusion
A reduction in static seawater pH at levels predicted within the next 200 years (i.e., to pHT 7.78 and 7.50, based on oceanic projections: Caldeira and Wickett, 2003; Doney et al., 2009) had little impact on physiological and adhesive metrics in the barnacle A. amphitrite. Shell growth, though, was significantly enhanced at reduced pH, while toughness of the base plate was diminished at pHT 7.50. If these changes impact the survival of A. amphitrite in the field, and how growth in a dynamic, natural environment would affect the magnitude of such changes, are yet to be determined. Alterations in the abundance of this ecologically dominant species, if they were to occur, would undoubtedly affect the composition of biofouling communities. Given the economic impact of marine biofouling (Callow and Callow, 2011; Schultz et al., 2011), additional assessments of A. amphitrite under changing environmental conditions are warranted. Multi-stressor and transgenerational assessments, as well as experiments that test natural sources of mortality in barnacles (e.g., predators or hydrodynamic stresses) would be especially helpful in predicting population level responses in A. amphitrite.
Data Availability Statement
The raw data supporting the conclusions of this manuscript can be found in the Supplementary Material.
Ethics Statement
This study was carried out in accordance with standard procedures for invertebrates.
Author Contributions
JN developed the seawater exposure system, collected and compiled data on seawater chemistry, physiology and adhesive metrics, and oversaw daily operations of the exposure. SP assessed shell thickness, mechanical properties, and crystallinity. KS and DT collected data on mortality, egg presence, adhesive properties, and shell mass and area. CM developed a prototype seawater exposure system and initiated a pilot study. JO’M, JH, and RM prepared shells and collected data on atomic disorder and RM analyzed atomic disorder data. BO and DR provided juvenile barnacles and algae and provided expertise on barnacle growth and assessment throughout the exposure. GD conceived of the experiments, analyzed final datasets, oversaw the experiment, and wrote the manuscript. All authors contributed to editing of the manuscript.
Funding
This material is based upon research supported by the Office of Naval Research under Award Number (N00014-14-1-0491) to GD.
Conflict of Interest Statement
The authors declare that the research was conducted in the absence of any commercial or financial relationships that could be construed as a potential conflict of interest.
Acknowledgments
The authors would like to thank Julian Sison, Shai Bejerano, Christine Makdisi, Aparna Yarram, and Mihir Soni for assistance in barnacle maintenance.
Supplementary Material
The Supplementary Material for this article can be found online at: https://www.frontiersin.org/articles/10.3389/fmars.2018.00369/full#supplementary-material.
FIGURE S1 | Shell assessments of barnacles, Amphibalanus amphitrite, exposed to one of three levels of pHT for 13 weeks (mean ± s.e.m.). Groups marked with different letters are significantly different as shown by Tukey HSD post-hoc analysis. Sample sizes can be found in Table 3 of the main text.
DATA SHEET S1 | Raw data for physiology, adhesion, and shell formation metrics.
Abbreviations
EDS, energy dispersive X-ray spectroscopy; FTIR, Fourier-transform infrared [spectroscopy]; OA, ocean acidification; pHNBS, pH calibrated against National Bureau of Standards buffers; pHT, pH total hydrogen ion concentration scale; SEM, scanning electron microscopy; TA, total alkalinity.
References
ASTM International (2005). Standard Test Method for Measurement of Barnacle Adhesion Strength in Shear. West Conshohocken, PA: ASTM International.
Barlow, D. E., Dickinson, G. H., Orihuela, B., Rittschof, D., and Wahl, K. J. (2009). In situ ATR-FTIR characterization of primary cement interfaces of the barnacle Balanus amphitrite. Biofouling 25, 359–366. doi: 10.1080/08927010902812009
Baumann, H., Wallace, R. B., Tagliaferri, T., and Gobler, C. J. (2015). Large natural pH, CO2 and O2 fluctuations in a temperate tidal salt marsh on diel, seasonal, and interannual time scales. Estuar. Coast. 38, 220–231. doi: 10.1007/s12237-014-9800-y
Beniash, E., Aizenberg, D., Addadi, L., and Weiner, S. (1997). Amorphous calcium carbonate transforms into calcite during sea urchin larval spicule growth. Proc. R. Soc. B 264, 461–465. doi: 10.1098/rspb.1997.0066
Beniash, E., Ivanina, A., Lieb, N. S., Kurochkin, I., and Sokolova, I. M. (2010). Elevated level of carbon dioxide affects metabolism and shell formation in oysters Crassostrea virginica. Mar. Ecol. Prog. Ser. 419, 95–108. doi: 10.1016/j.aquatox.2017.06.009
Berglin, M., and Gatenholm, P. (2003). The barnacle adhesive plaque: morphological and chemical differences as a response to substrate properties. Colloid Surf. B 28, 107–117. doi: 10.1016/S0927-7765(02)00149-2
Burden, D. K., Spillmann, C. M., Everett, R. K., Barlow, D. E., Orihuela, B., Deschamps, J. R., et al. (2014). Growth and development of the barnacle Amphibalanus amphitrite: time and spatially resolved structure and chemistry of the base plate. Biofouling 30, 799–812. doi: 10.1080/08927014.2014.930736
Caldeira, K., and Wickett, M. E. (2003). Anthropogenic carbon and ocean pH. Nature 425, 365–365. doi: 10.1038/425365a
Callow, J. A., and Callow, M. E. (2011). Trends in the development of environmentally friendly fouling-resistant marine coatings. Nat. Commun. 2:244. doi: 10.1038/ncomms1251
Cameron, J. N., and Wood, C. M. (1985). Apparent H+ excretion and CO2 dynamics accompanying carapace mineralization in the blue crab (Callinectes sapidus) following molting. J. Exp. Biol. 114, 181–196.
Campanati, C., Yip, S., Lane, A., and Thiyagarajan, V. (2016). Combined effects of low pH and low oxygen on the early-life stages of the barnacle Balanus amphitrite. ICES J. Mar. Sci. 73, 791–802. doi: 10.1093/icesjms/fsv221
Cho, K. R., Kim, Y. Y., Yang, P., Cai, W., Pan, H., Kulak, A. N., et al. (2016). Direct observation of mineral–organic composite formation reveals occlusion mechanism. Nat. Commun. 7:10187. doi: 10.1038/ncomms10187
De Gregorio, B. T., Stroud, R. M., Burden, D. K., Fears, K. P., Everett, R. K., and Wahl, K. J. (2015). Shell structure and growth in the base plate of the barnacle Amphibalanus amphitrite. ACS Biomat. Sci. Eng. 1, 1085–1095. doi: 10.1080/08927014.2014.930736
Dickinson, G. H. (2008). Barnacle Cement: a Polymerization Model Based on Evolutionary Concepts. Ph.D. Thesis, Duke University, Durham, NC.
Dickson, A. G., Sabine, C. L., and Christian, J. R. (2007). Guide to Best Practices for Ocean CO2 Measurements. PICES Special Publication 3. Sidney: North Pacific Marine Science Organization.
Doney, S. C., Fabry, V. J., Feely, R. A., and Kleypas, J. A. (2009). Ocean acidification: the other CO2 problem. Annu. Rev. Mar. Sci. 1, 169–192. doi: 10.1146/annurev.marine.010908.163834
Eriander, L., Wrange, A. L., and Havenhand, J. (2016). Simulated diurnal pH fluctuations radically increase variance in—but not the mean of—growth in the barnacle Balanus improvisus. ICES J. Mar. Sci. 73, 596–603. doi: 10.1093/icesjms/fsv214
Essock-Burns, T., Gohad, N. V., Orihuela, B., Mount, A. S., Spillmann, C. M., Wahl, K. J., et al. (2017). Barnacle biology before, during and after settlement and metamorphosis: a study of the interface. J. Exp. Biol. 220, 194–207. doi: 10.1242/jeb.145094
Fernandez, M. S., Vergara, I., Oyarzun, A., Arias, J. I., Rodriguez, R., Wiff, J. P., et al. (2002). “Extracellular matrix molecules involved in barnacle shell mineralization,” in Proceedings of the MRS Online Library Archive, Warrendale, PA, 724.
Findlay, H. S., Kendall, M. A., Spicer, J. I., and Widdicombe, S. (2009). Future high CO2 in the intertidal may compromise adult barnacle Semibalanus balanoides survival and embryonic development rate. Mar. Ecol. Prog. Ser. 389, 193–202. doi: 10.3354/meps08141
Findlay, H. S., Kendall, M. A., Spicer, J. I., and Widdicombe, S. (2010a). Post-larval development of two intertidal barnacles at elevated CO2 and temperature. Mar. Biol. 157, 725–735. doi: 10.1007/s00227-009-1356-1
Findlay, H. S., Kendall, M. A., Spicer, J. I., and Widdicombe, S. (2010b). Relative influences of ocean acidification and temperature on intertidal barnacle post-larvae at the northern edge of their geographic distribution. Estuar. Coast. Shelf Sci. 86, 675–682. doi: 10.1016/j.ecss.2009.11.036
Fratzl, P., Gupta, H. S., Fischer, F. D., and Kolednik, O. (2007). Hindered crack propagation in materials with periodically varying Young’s modulus - lessons from biological materials. Adv. Mater. 19, 2657–2661. doi: 10.1002/adma.200602394
Gohad, N. V., Dickinson, G. H., Orihuela, B., Rittschof, D., and Mount, A. S. (2009). Visualization of putative ion-transporting epithelia in Amphibalanus amphitrite using correlative microscopy: potential function in osmoregulation and biomineralization. J. Exp. Mar. Biol. Ecol. 380, 88–98. doi: 10.1016/j.jembe.2009.09.008
Golden, J. P., Burden, D. K., Fears, K. P., Barlow, D. E., So, C. R., Burns, J., et al. (2016). Imaging active surface processes in barnacle adhesive interfaces. Langmuir 32, 541–550. doi: 10.1021/acs.langmuir.5b03286
Harvey, B. P., and Moore, P. J. (2017). Ocean warming and acidification prevent compensatory response in a predator to reduced prey quality. Mar. Ecol. Prog. Ser. 563, 111–122. doi: 10.3354/meps11956
Holm, E. R., Orihuela, B., Kavanagh, C., and Rittschof, D. (2005). Variation among families for characteristics of the adhesive plaque in the barnacle Balanus amphitrite. Biofouling 21, 121–126. doi: 10.1080/08927010512331344188
Kamino, K. (2008). Underwater adhesive of marine organisms as the vital link between biological science and material science. Mar. Biotechnol. 10, 111–121. doi: 10.1007/s10126-007-9076-3
Kamino, K. (2016). “Barnacle underwater attachment,” in Biological Adhesives, eds A. M. Smith and J. A. Callow (Cham: Springer), 153–176.
Kamino, K., Inoue, K., Maruyama, T., Takamatsu, N., Harayama, S., and Shizuri, Y. (2000). Barnacle cement proteins. Importance of disulfide bonds in their insolubility. J. Biol. Chem. 275, 27360–27365.
Kamino, K., Odo, S., and Maruyama, T. (1996). Cement proteins of the acorn barnacle, Megabalanus rosa. Biol. Bull. 190, 403–409. doi: 10.2307/1543033
Khalifa, G. M., Weiner, S., and Addadi, L. (2011). Mineral and matrix components of the operculum and shell of the barnacle Balanus amphitrite: calcite crystal growth in a hydrogel. Cryst. Growth Des. 11, 5122–5130. doi: 10.1021/cg2010216
Kim, Y. Y., Carloni, J. D., Demarchi, B., Sparks, D., Reid, D. G., Kunitake, M. E., et al. (2016). Tuning hardness in calcite by incorporation of amino acids. Nat. Mater. 15, 903–910. doi: 10.1038/nmat4631
Kroeker, K. J., Kordas, R. L., Crim, R., Hendriks, I. E., Ramajo, L., Singh, G. S., et al. (2013). Impacts of ocean acidification on marine organisms: quantifying sensitivities and interaction with warming. Glob. Change Biol. 19, 1884–1896. doi: 10.1111/gcb.12179
Kroeker, K. J., Kordas, R. L., Crim, R. N., and Singh, G. G. (2010). Meta-analysis reveals negative yet variable effects of ocean acidification on marine organisms. Ecol. Lett. 13, 1419–1434. doi: 10.1111/j.1461-0248.2010.01518.x
Kunitake, M. E., Baker, S. P., and Estroff, L. A. (2012). The effect of magnesium substitution on the hardness of synthetic and biogenic calcite. MRS Commun. 2, 113–116. doi: 10.1557/mrc.2012.20
LeFurgey, A., Freudenrich, C., Wallace, N., Ingram, P., and Wilbur, K. (1995). The onset of biomineralization during cyprid to juvenile metamorphosis of the barnacle (Balanus amphitrite amphitrite). FASEB J. 9:A639.
Lewis, A. C., Burden, D. K., Wahl, K. J., and Everett, R. K. (2014). Electron backscatter diffraction (EBSD) study of the structure and crystallography of the barnacle Balanus amphitrite. JOM 66, 143–148. doi: 10.1007/s11837-013-0793-y
Long, X., Ma, Y., and Qi, L. (2014). Biogenic and synthetic high magnesium calcite–a review. J. Struct. Biol. 185, 1–14. doi: 10.1016/j.jsb.2013.11.004
Lunden, J. J., Turner, J. M., Mcnicholl, C. G., Glynn, C. K., and Cordes, E. E. (2014). Design, development, and implementation of recirculating aquaria for maintenance and experimentation of deep-sea corals and associated fauna. Limnol. Oceanogr. Meth. 12, 363–372. doi: 10.4319/lom.2014.12.363
McCoy, S. J., Kamenos, N. A., Chung, P., Wootton, T. J., and Pfister, C. A. (2018). A mineralogical record of ocean change: decadal and centennial patterns in the California mussel. Glob. Change Biol. 24, 2554–2562. doi: 10.1111/gcb.14013
McDonald, M. R., Mcclintock, J. B., Amsler, C. D., Rittschof, D., Angus, R. A., Orihuela, B., et al. (2009). Effects of ocean acidification over the life history of the barnacle Amphibalanus amphitrite. Mar. Ecol. Prog. Ser. 385, 179–187. doi: 10.3354/meps08099
Melzner, F., Gutowska, M. A., Langenbuch, M., Dupont, S., Lucassen, M., Thorndyke, M. C., et al. (2009). Physiological basis for high CO2 tolerance in marine ectothermic animals: pre-adaptation through lifestyle and ontogeny? Biogeosciences 6, 2313–2331. doi: 10.5194/bg-6-2313-2009
Meseck, S. L., Alix, J. H., Swiney, K. M., Long, W. C., Wikfors, G. H., and Foy, R. J. (2016). Ocean acidification affects hemocyte physiology in the Tanner crab (Chionoecetes bairdi). PLoS One 11:e0148477. doi: 10.1371/journal.pone.0148477
Meyers, M. A., and Chen, P. Y. (2014). Biological Materials Science: Biological Materials, Bioinspired Materials, and Biomaterials. Cambridge: Cambridge University Press. doi: 10.1017/CBO9780511862397
Morris, S., and Taylor, A. C. (1983). Diurnal and seasonal variation in physico-chemical conditions within intertidal rock pools. Estuar. Coast. Shelf Sci. 17, 339–355. doi: 10.1016/0272-7714(83)90026-4
Moulin, L., Catarino, A. I., Claessens, T., and Dubois, P. (2011). Effects of seawater acidification on early development of the intertidal sea urchin Paracentrotus lividus (Lamarck 1816). Mar. Pollut. Bull. 62, 48–54. doi: 10.1016/j.marpolbul.2010.09.012
Naldrett, M. J. (1993). The importance of sulfur cross-links and hydrophobic interactions in the polymerization of barnacle cement. J. Mar. Biol. Assoc. UK 73, 689–702. doi: 10.1017/S0025315400033221
Naldrett, M. J., and Kaplan, D. L. (1997). Characterization of barnacle (Balanus eburneus and B. crenatus) adhesive proteins. Mar. Biol. 127, 629–635. doi: 10.1007/s002270050053
Nousek, N. A. (1984). Shell formation and calcium transport in the barnacle Chthamalus fragilis. Tissue Cell 16, 433–442. doi: 10.1016/0040-8166(84)90061-2
O’Donnell, M. J., George, M. N., and Carrington, E. (2013). Mussel byssus attachment weakened by ocean acidification. Nat. Clim. Change 3, 587–590. doi: 10.1038/nclimate1846
Pansch, C., Nasrolahi, A., Appelhans, Y. S., and Wahl, M. (2012). Impacts of ocean warming and acidification on the larval development of the barnacle Amphibalanus improvisus. J. Exp. Mar. Biol. Ecol. 420, 48–55. doi: 10.1016/j.jembe.2012.03.023
Pansch, C., Nasrolahi, A., Appelhans, Y. S., and Wahl, M. (2013). Tolerance of juvenile barnacles (Amphibalanus improvisus) to warming and elevated pCO2. Mar. Biol. 160, 2023–2035. doi: 10.1007/s00227-012-2069-4
Pansch, C., Schaub, I., Havenhand, J., and Wahl, M. (2014). Habitat traits and food availability determine the response of marine invertebrates to ocean acidification. Glob. Change Biol. 20, 765–777. doi: 10.1111/gcb.12478
Pitombo, F. B. (2004). Phylogenetic analysis of the Balanidae (Cirripedia, Balanomorpha). Zool. Scr. 33, 261–276. doi: 10.1111/j.0300-3256.2004.00145.x
Regev, L., Poduska, K. M., Addadi, L., Weiner, S., and Boaretto, E. (2010). Distinguishing between calcites formed by different mechanisms using infrared spectrometry: archaeological applications. J. Archaeol. Sci. 37, 3022–3029. doi: 10.1016/j.jas.2010.06.027
Riebesell, U., Fabry, V. J., Hansson, L., and Gattuso, J. P. (2011). Guide to Best Practices for Ocean Acidification Research and Data Reporting. Luxembourg: Office for Official Publications of the European Communities.
Ries, J. B., Cohen, A. L., and Mccorkle, D. C. (2009). Marine calcifiers exhibit mixed responses to CO2-induced ocean acidification. Geology 37, 1131–1134. doi: 10.1130/G30210A.1
Rittschof, D., Branscomb, E., and Costlow, J. (1984). Settlement and behavior in relation to flow and surface in larval barnacles, Balanus amphitrite Darwin. J. Exp. Mar. Biol. Ecol. 82, 131–146. doi: 10.1016/0022-0981(84)90099-6
Rittschof, D., Clare, A., Gerhart, D., Mary, S. A., and Bonaventura, J. (1992). Barnacle in vitro assays for biologically active substances: toxicity and settlement inhibition assays using mass cultured Balanus amphitrite amphitrite Darwin. Biofouling 6, 115–122. doi: 10.1080/08927019209386217
Rittschof, D., Orihuela, B., Stafslien, S., Daniels, J., Christianson, D., Chisholm, B., et al. (2008). Barnacle reattachment: a tool for studying barnacle adhesion. Biofouling 24, 1–9. doi: 10.1080/08927010701784920
Rodriguez-Navarro, A. B., Cabraldemelo, C., Batista, N., Morimoto, N., Alvarez-Lloret, P., Ortega-Huertas, M., et al. (2006). Microstructure and crystallographic-texture of giant barnacle (Austromegabalanus psittacus) shell. J. Struct. Biol. 156, 355–362. doi: 10.1016/j.jsb.2006.04.009
Roleda, M. Y., Boyd, P. W., and Hurd, C. L. (2012). Before ocean acidification: calcifier chemistry lessons. J. Phycol. 48, 840–843. doi: 10.1111/j.1529-8817.2012.01195.x
Saroyan, J. R., Lindner, E., and Dooley, C. A. (1970). Repair and reattachment in the Balanidae as related to their cementing mechanism. Biol. Bull. 139, 333–350. doi: 10.2307/1540088
Schultz, M. P., Bendick, J. A., Holm, E. R., and Hertel, W. M. (2011). Economic impact of biofouling on a naval surface ship. Biofouling 27, 87–98. doi: 10.1080/08927014.2010.542809
So, C. R., Fears, K. P., Leary, D. H., Scancella, J. M., Wang, Z., Liu, J. L., et al. (2016). Sequence basis of barnacle cement nanostructure is defined by proteins with silk homology. Sci. Rep. 6:36219. doi: 10.1038/srep36219
So, C. R., Scancella, J. M., Fears, K. P., Essock-Burns, T., Haynes, S. E., Leary, D. H., et al. (2017). Oxidase activity of the barnacle adhesive interface involves peroxide-dependent catechol oxidase and lysyl oxidase enzymes. ACS Appl. Mater. Inter. 9, 11493–11505. doi: 10.1021/acsami.7b01185
Truchot, J. P., and Duhamel-Jouve, A. (1980). Oxygen and carbon dioxide in the marine intertidal environment: diurnal and tidal changes in rockpools. Respir Physiol. 39, 241–254. doi: 10.1016/0034-5687(80)90056-0
Waldbusser, G. G., Voigt, E. P., Bergschneider, H., Green, M. A., and Newell, R. I. (2011). Biocalcification in the eastern oyster (Crassostrea virginica) in relation to long-term trends in Chesapeake Bay pH. Estuar. Coast. 34, 221–231. doi: 10.1007/s12237-010-9307-0
Walker, G. (1972). The biochemical composition of the cement of the two barnacle species, Balanus hameri and Balanus crenatus. J. Mar. Biol. Assoc. UK 52, 429–435. doi: 10.1017/S0025315400018786
Wang, Z., Leary, D. H., Liu, J., Settlage, R. E., Fears, K. P., North, S. H., et al. (2015). Molt-dependent transcriptomic analysis of cement proteins in the barnacle Amphibalanus amphitrite. BMC Genomics 16:859. doi: 10.1186/s12864-015-2076-1
Whiteley, N. M. (2011). Physiological and ecological responses of crustaceans to ocean acidification. Mar. Ecol. Prog. Ser. 430, 257–271. doi: 10.3354/meps09185
Wiegemann, M., and Watermann, B. (2003). Peculiarities of barnacle adhesive cured on non-stick surfaces. J. Adhes. Sci. Technol. 17, 1957–1977. doi: 10.1163/156856103770572070
Wong, K. K. W., Lane, A. C., Leung, P. T. Y., and Thiyagarajan, V. (2011). Response of larval barnacle proteome to CO2-driven seawater acidification. Comp. Biochem. Physiol. Part D Genomics Proteomics 6, 310–321. doi: 10.1016/j.cbd.2011.07.001
Wootton, J. T., Pfister, C. A., and Forester, J. D. (2008). Dynamic patterns and ecological impacts of declining ocean pH in a high-resolution multi-year dataset. Proc. Natl. Acad. Sci. U.S.A. 105, 18848–18853. doi: 10.1073/pnas.0810079105
Keywords: biomineralization, climate change, mechanical properties, biofouling, cement, adhesive tenacity, calcite, Balanus
Citation: Nardone JA, Patel S, Siegel KR, Tedesco D, McNicholl CG, O’Malley J, Herrick J, Metzler RA, Orihuela B, Rittschof D and Dickinson GH (2018) Assessing the Impacts of Ocean Acidification on Adhesion and Shell Formation in the Barnacle Amphibalanus amphitrite. Front. Mar. Sci. 5:369. doi: 10.3389/fmars.2018.00369
Received: 01 March 2018; Accepted: 25 September 2018;
Published: 22 October 2018.
Edited by:
Pei-Yuan Qian, Hong Kong University of Science and Technology, Hong KongReviewed by:
Adam Michael Reitzel, University of North Carolina at Charlotte, United StatesCiro Rivera-Casas, Florida International University, United States
Copyright © 2018 Nardone, Patel, Siegel, Tedesco, McNicholl, O’Malley, Herrick, Metzler, Orihuela, Rittschof and Dickinson. This is an open-access article distributed under the terms of the Creative Commons Attribution License (CC BY). The use, distribution or reproduction in other forums is permitted, provided the original author(s) and the copyright owner(s) are credited and that the original publication in this journal is cited, in accordance with accepted academic practice. No use, distribution or reproduction is permitted which does not comply with these terms.
*Correspondence: Gary H. Dickinson, ZGlja2luZ2FAdGNuai5lZHU=