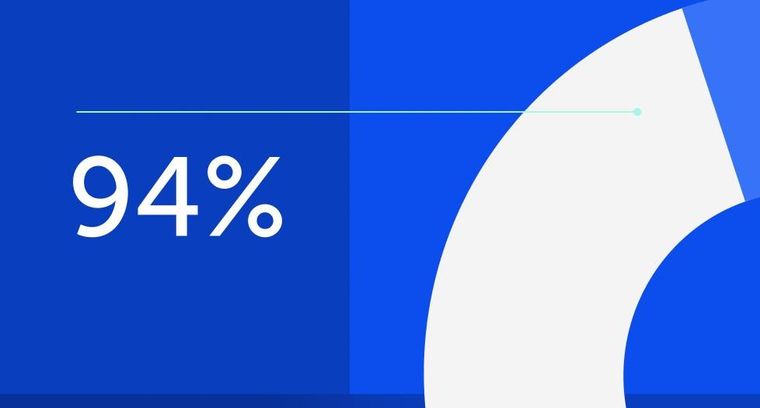
94% of researchers rate our articles as excellent or good
Learn more about the work of our research integrity team to safeguard the quality of each article we publish.
Find out more
ORIGINAL RESEARCH article
Front. Mar. Sci., 04 October 2018
Sec. Global Change and the Future Ocean
Volume 5 - 2018 | https://doi.org/10.3389/fmars.2018.00337
This article is part of the Research TopicSuccesses at the Interface of Ocean, Climate and HumansView all 16 articles
The Paris Agreement target of limiting global surface warming to 1.5–2∘C compared to pre-industrial levels by 2100 will still heavily impact the ocean. While ambitious mitigation and adaptation are both needed, the ocean provides major opportunities for action to reduce climate change globally and its impacts on vital ecosystems and ecosystem services. A comprehensive and systematic assessment of 13 global- and local-scale, ocean-based measures was performed to help steer the development and implementation of technologies and actions toward a sustainable outcome. We show that (1) all measures have tradeoffs and multiple criteria must be used for a comprehensive assessment of their potential, (2) greatest benefit is derived by combining global and local solutions, some of which could be implemented or scaled-up immediately, (3) some measures are too uncertain to be recommended yet, (4) political consistency must be achieved through effective cross-scale governance mechanisms, (5) scientific effort must focus on effectiveness, co-benefits, disbenefits, and costs of poorly tested as well as new and emerging measures.
The ocean provides most of the life-supporting environment on the planet. It hosts a large portion of biodiversity, plays a major role in climate regulation, sustains a vibrant economy and contributes to food security worldwide. Severe impacts on key marine ecosystems and ecosystem services are projected in response to the future increase in global mean temperature and concurrent ocean acidification, deoxygenation, and sea-level rise (Hoegh-Guldberg et al., 2014; Pörtner et al., 2014; Gattuso et al., 2015). These impacts scale to CO2 emissions: they will be considerably worse with a high emissions scenario than with a scenario that limits the temperature increase to 2°C relative to pre-industrial levels (Bopp et al., 2013). Current emission reduction pledges under the 2015 Paris Agreement (UNFCCC, 2015) are, however, insufficient to keep global temperature below +2°C in 2100 relative to pre-industrial level (Rogelj et al., 2016) and to reach targets for the United Nations Sustainable Development Goals. Increased ambition, with additional actions, is therefore required.
Further reductions in atmospheric greenhouse gas emissions are achievable through: (1) a shift from fossil fuels to renewable energy; (2) improved energy efficiency; (3) carbon capture and storage (CCS) at the point of CO2 generation; and (4) the protection and enhancement of natural carbon sinks (Griscom et al., 2017; Rockström et al., 2017). The risk of failing to meet climate targets via emissions reduction has increased interest in solar radiation management (National Research Council, 2015b) and carbon dioxide removal from the atmosphere (National Research Council, 2015a; Williamson, 2016; Hansen et al., 2017). For example, the implementation of bioenergy with carbon capture and storage is a major component of a roadmap to reduce global emissions from ∼40 Gt CO2 year-1 in 2020 to ∼5 Gt CO2 year-1 by 2050 (Rockström et al., 2017). Such an ambitious roadmap, however, poses significant political, economic, and environmental challenges because of the land, water, and nutrient requirements to produce the biomass (potentially in competition with existing ecosystems, land use, and food production), the cost and feasibility of carbon capture and storage, and the fact that such systems have yet to be proven effective at the required scales (Anderson and Peters, 2016; Smith et al., 2016; Boysen et al., 2017). Additionally, and even under a successful mitigation scenario, impacts are expected at the local scale, hence the need for enhanced adaptation measures.
To date, policy responses to climate change and its impacts have largely focussed on land-based actions (Field and Mach, 2017) while relatively little attention has been paid to ocean-based potential (Rau et al., 2012; Billé et al., 2013), despite the recent launch of the Ocean Pathway initiative by the Presidency of the 23rd Conference of the Parties (COP23) of the United Nations Framework Convention on Climate Change (UNFCCC). The ocean already removes about 25% of anthropogenic CO2 emissions (Le Quéré et al., 2018) and has the potential to remove and store much more (Rau, 2014). Thus, ocean-based actions could significantly reduce the magnitude and rate of ocean warming, ocean acidification, and sea-level rise, as well as their impacts on marine ecosystems and ecosystem services. They could also play a significant role in helping to reduce global warming and its impacts on the non-ocean surface of the planet – and on human society. However, there may be associated risks to ocean life and people, and there is a lack of guidance for prioritizing ocean-based interventions since there has been relatively little research, development and deployment in this field. Important issues include determining the effectiveness of a given approach in countering changes in climate drivers and/or impacts, possible spatial and temporal scales of deployment, associated positive and negative climate, environmental, economic, and societal impacts (Russell et al., 2012), and hence the implications for ethics, equity, and governance (Preston, 2013; Burns et al., 2016; Williamson and Bodle, 2016).
To fill this gap, we assess the potential of 13 categories of ocean-based measures or schemes to reduce climate-related drivers globally and/or locally (<∼100 km2), as well as to reduce adverse impacts on selected, important and sensitive marine ecosystems and ecosystem services. The three drivers considered are ocean warming, ocean acidification and sea-level rise, although others such as hypoxia, extreme events, and changes in storminess and precipitation can also be important. We focus on four ecosystems and habitats (warm-water coral reefs, mangroves and salt-marshes, seagrass beds, and Arctic biota) and four ecosystem services (finfish fisheries, fish aquaculture, coastal protection, and bivalve fisheries and aquaculture), which are particularly vulnerable to climate impacts and are critical for livelihoods and food security. The potential of each ocean-based measure is assessed in terms of the following eight environmental, technological, social, and economic criteria: (1) potential effectiveness to increase net carbon uptake and moderate ocean warming, ocean acidification, and sea level rise; (2) technological readiness; (3) lead time until full potential effectiveness; (4) duration of benefits; (5) co-benefits; (6) disbenefits; (7) cost effectiveness; and (8) governability from an international perspective. This expert assessment is based on an extensive literature review and is supported by Supplementary Materials (SM) that provide details on the terminology, assessment methods, results, and supporting literature.
Ecosystems have different sensitivities to ocean warming, ocean acidification and sea-level rise (Figure 1A and section “SM3.3” of the Supplementary Materials). Interactions between drivers can be complex: additive, synergistic, or antagonistic (Crain et al., 2008). There are big gaps in multiple-drivers research (Crain et al., 2008; Riebesell and Gattuso, 2015) but experimental strategies to assess the biological ramifications of multiple drivers of global ocean change have become available (Boyd et al., 2018).
FIGURE 1. Sensitivity of key ecosystems (A) and ecosystem services (B) to key climate-related drivers. The scores of this expert assessment and their justification are provided in section “SM3.3” of the Supplementary Materials.
Of the four ecosystems or habitats considered here, coral reefs and Arctic biota are the most imminently threatened and will be affected to a greater degree sooner than others, with high risk that key functions will be lost globally, as identified in the 5th assessment report (AR5) of the Intergovernmental Panel on Climate Change (IPCC) (Hoegh-Guldberg et al., 2014; Pörtner et al., 2014). Coral reefs are very sensitive to ocean warming and acidification (Hoegh-Guldberg et al., 2007; Gattuso et al., 2014; Hughes et al., 2017a). They have suffered extensive losses in the past three decades due to high sea surface temperature combined with local stressors such as overfishing, destructive fishing, coastal development, and pollution. All projections indicate that the thermal conditions driving major losses will increase in frequency and exceed thresholds for the majority of reefs by 2050 (Gattuso et al., 2014; Pörtner et al., 2014). Over the 21st century under the high emissions Representative Concentration Pathway RCP8.5 scenario (van Vuuren et al., 2011), 99% of the world’s coral reefs are expected to experience annual severe bleaching due to thermal stress (van Hooidonk et al., 2016). The thermal sensitivity of coral reefs is compounded by ocean acidification (Hoegh-Guldberg et al., 2014), which diminishes coral growth and calcification (Albright et al., 2018) and can lead to increased bioerosion and vulnerability to storm damage (Andersson and Gledhill, 2013).
Arctic biota are also highly sensitive to climate change, particularly ice-associated biota that are rapidly declining in Arctic summers (Wassmann et al., 2010; Pörtner et al., 2014; Kohlbach et al., 2017). Within the Arctic, ecosystem responses vary greatly depending on ambient variability, degree of warming, and nutrient advection (Hunt et al., 2016). Warming and freshening may also impact ecosystem production by differentially increasing respiration rates and reducing nutrient supply (Duarte et al., 2012) as well as enhancing the degree of ocean acidification due to freshening (Pörtner et al., 2014). Arctic organisms that seem particularly sensitive to ocean acidification include calcifiers such as bivalves and planktonic pteropods that are key links in ocean food webs (Comeau et al., 2010; Duarte et al., 2012).
Mangroves and saltmarshes are highly sensitive to sea-level rise (Kirwan and Megonigal, 2013; Lovelock et al., 2015), particularly where coastal development and steep topography block landward migration and insufficient sediment is delivered to support accretion. A preliminary global modeling effort suggests that a 50 cm sea-level rise by 2100 would result in a loss of 46 to 59% of global coastal wetlands (up to 78% loss under 110 cm rise), but losses are sensitive to assumptions about human coastal development and may be reduced if additional tidal hydrodynamic feedbacks are included (Spencer et al., 2016). Warming and acidification are not projected to have significant direct effects on mangroves and saltmarshes, but may have positive or negative effects at local scales due to changes in species composition, phenology, productivity, and latitudinal range of distribution (Ward et al., 2016).
Temperate seagrass ecosystems are sensitive to ocean warming. For example, the thermal regime of the Mediterranean Sea already exceeds the upper thermal limit of the endemic Posidonia oceanica in some areas (Marbà and Duarte, 2010; Jordà et al., 2012). Seagrass and fleshy algae may expand in Arctic regions with warming and loss of ice cover (Krause-Jensen and Duarte, 2014). Some may benefit from carbonate chemistry changes associated with ocean acidification as their photosynthesis is CO2-limited (Raven and Beardall, 2014) but sensitive calcifiers growing in the meadows are negatively impacted (Martin et al., 2008).
The ecosystem services considered in this study are all highly sensitive to ocean warming (Weatherdon et al., 2016; Figure 1B and section “SM3.3” of the Supplementary Materials). Global potential fisheries catches and species turnover, for instance, are projected to decrease by about 3 Mt and increase by 10%, respectively, for every 1°C of global surface warming (Cheung et al., 2016). These patterns are similar for finfish and shellfish aquaculture, as ∼90% of current finfish and shellfish mariculture production is from open-water farming where environmental conditions closely match those in the nearby ocean (Callaway et al., 2012). Shellfish fisheries and mariculture, in particular, are threatened by the combined effects of warming (Mackenzie et al., 2014), ocean acidification (Barton et al., 2012; Gazeau et al., 2013) and deoxygenation (Gobler et al., 2014). Despite possible genetic adaptation over generations (Thomsen et al., 2017), impacts on shellfish are expected to be high to very high when CO2 concentrations exceed those projected for 2100 in the low to moderate RCP2.6 and 4.5 CO2 emissions scenarios (Gattuso et al., 2015; Cooley et al., 2016). In addition, finfish mariculture often focuses on high trophic level species that are dependent on wild capture fisheries for feed (Troell et al., 2014) and some operations still largely rely on wild captured fish fry and juveniles (Diana, 2009). Thus, mariculture is likely to be subject to similar climatic stresses as fish stocks in the wild.
The sensitivity of coastal protection, notably wave attenuation and shoreline stabilization, to climate-related drivers differs for each ecosystem considered (Spalding et al., 2014). The cumulative impacts of increasing sea-surface temperature, ocean acidification, and non-climatic stressors such as land-based pollution reduce reefs’ ability to keep pace with sea-level rise (Yates et al., 2017). The consequences of sea-level rise on biologically structured coastal ecosystems raise concerns as these habitats are estimated to currently reduce wave height by 30 to 90% (in order of highest to lowest wave reduction: coral reefs, saltmarshes, mangroves, and seagrasses) (Fonseca and Cahalan, 1992; Duarte et al., 2013; Ferrario et al., 2014; Narayan et al., 2016). Historical global losses in coastal ecosystems [30 to 50% for mangroves since the 1940s, 29% for seagrass since 1879, 25% for saltmarshes since the 1800s (Waycott et al., 2009; Mcleod et al., 2011)] and degradation of coral reefs [30–75% since prehuman times (Pandolfi et al., 2003)] have already reduced their potential to provide ecosystem services. Projections suggest that 90% of coral reefs worldwide could be lost if warming exceeds 1.5°C (Frieler et al., 2013).
Four types of actions to reduce the scale and impacts of climate change are considered (Figure 2): (1) reduction of atmospheric greenhouse gas concentrations, (2) solar radiation management, (3) protection of biota and ecosystems, and (4) manipulation of biological and ecological adaptation. The actions in the first two categories (referred to as global actions hereafter, although some forms of solar radiation management could be local) aim to either reduce the main cause of climate change at the global scale (primarily the increase in atmospheric CO2 concentration) or to counteract warming through increasing albedo in the atmosphere or at the Earth’s surface, thereby increasing the proportion of solar radiation that is reflected back to space. The actions in the other two categories (referred to as local actions hereafter) aim to reduce the risk of climate change impacts locally, either by reducing the locally experienced drivers (site-specific acidification and warming, and relative sea-level rise) and/or reducing the sensitivity of organisms and ecosystems to these drivers (Bates et al., 2017; Cheung et al., 2017). Vegetation and alkalinization (see Box 1 and section “SM1” of the Supplementary Materials for descriptions) are evaluated for both global and local aims as they can be deployed globally to reduce changes in climate-related drivers and impacts, as well as locally to reduce the sensitivity of marine ecosystems and services to specific drivers such as relative sea-level rise and ocean acidification.
FIGURE 2. Potential ocean solutions. Four main groups are considered: addressing the causes of climate change (i.e., reducing anthropogenic greenhouse gas emissions or increasing the long-term removal of greenhouse gases, primarily CO2), solar radiation management, protection of biota and ecosystems (habitats, species, resources, etc.), and manipulation of biological and ecological adaptation.
Ocean-based solutions. Measures that address the causes of global climate change either reduce anthropogenic greenhouse gas emissions or increase their long-term removal from the atmosphere. Five measures are considered in this group, including negative emissions technologies (see Minx et al., 2018) which are critical for achieving the long-term climate goals of the Paris Agreement (UNFCCC, 2015). (1) Ocean-based renewable energy (hereafter renewable energy) comprises the production of energy using offshore wind turbines and harvesting of energy from tides, waves, ocean currents, and thermal stratification (Pelc and Fujita, 2002). (2) The restoration and conservation of coastal vegetation (hereafter vegetation), primarily saltmarshes, mangroves and seagrasses (also referred to as “blue carbon ecosystems”), seeks to enhance their carbon sink capacity and avoid emissions from their existing large carbon stocks if degraded or destroyed (Mcleod et al., 2011; Herr and Landis, 2016). This measure is considered not only in terms of global implementation – i.e., assuming theoretical worldwide conservation and restoration of all such habitats that have been degraded or lost due to human activities – but also local implementation, providing local mitigation and adaptation benefits in addition to other co-benefits. (3) Fertilization involves the artificial increase in the ocean’s primary production and, hence, carbon uptake by phytoplankton in the open ocean, to be achieved primarily by adding soluble iron to surface waters where it is currently lacking, mostly in mid-ocean gyres and the Southern Ocean (Aumont and Bopp, 2006). (4) Alkalinization describes the addition of a variety of alkaline substances that consume CO2 and/or neutralize acidity (Rau, 2011; Renforth and Henderson, 2017), primarily achieved by raising the concentration of carbonate or hydroxide ions in surface waters, and thereby shifting the associated chemical equilibria in seawater to increase the oceanic uptake of atmospheric CO2. The feasibility and effectiveness of adding alkalinity are considered at both global and local scales. In either case the alkalinity would be derived from land-based mineral or synthetic chemical sources or from locally available marine material (e.g., waste shells). The alkalinity would then require transport to and distribution within the marine environment. (5) Land-ocean hybrid methods include the use of the ocean and its sediments to store biomass, CO2 or alkalinity derived from terrestrial sources. Examples are crop residue storage on the seafloor (Strand and Benford, 2009), marine storage of CO2 from land-based bio-energy or from direct air capture of CO2 (Sanz-Pérez et al., 2016) and conversion of such CO2 to alkaline forms for ocean storage (Rau, 2011). Hybrid methods also include techniques involving marine-to-land transfers, such as using marine biomass to fuel biomass energy with carbon capture and storage (BECCS) on land or using such biomass to form biochar as a soil amendment.
Another area of action to counter global and ocean warming (but which does not directly address the greenhouse gas cause) is solar radiation management (SRM, also known as sunlight reflection methods). Several schemes were described, including stratospheric aerosol injection (National Research Council, 2015b). Two ocean-based schemes are considered here. (6) Marine cloud brightening (hereafter cloud brightening) involves the large-scale aerial spraying of seawater or other substances into the lower atmosphere to increase the amount of sunlight clouds reflect back into space (Latham et al., 2012; Kravitz et al., 2013). Sub-global implementation could also be considered (Latham et al., 2013). (7) Increased surface ocean albedo (hereafter albedo enhancement) is here considered to be achieved by long-lived ocean micro-bubbles or foams, produced either by commercial shipping (Crook et al., 2016) or by vessels dedicated to that task.
Four measures relate to the protection of biota and ecosystems. (8) Reducing pollution refers to decreasing release of anthropogenic, harmful substances. Pollution can exacerbate hypoxia and ocean acidification especially in coastal waters (Cai et al., 2011) while increasing the sensitivity of marine organisms and ecosystems to climate-related drivers (Alava et al., 2017). (9) Restoring hydrological regimes (restoring hydrology) relates to the maintenance and restoration of marine hydrological conditions, primarily in coastal waters, including both the tidal and riverine delivery of water and sediments, to alleviate local changes in climate-related drivers (Howard et al., 2017). (10) Eliminating overexploitation includes ensuring the harvest and extraction of living resources are within biologically safe limits for sustainable use by humans and to maintain ecosystem function and, in the case of non-living resources (e.g., sand and minerals), in levels that avoid irreversible ecological impacts. For example, in over-exploited ecosystems, pelagic species that are smaller and faster turnover generally increase in dominance (Cheung et al., 2007). Abundance of these pelagic species tends to track environmental conditions more closely than large demersal fishes (Winemiller, 2005), the latter are often depleted in over-exploited systems (Cheung et al., 2007). Thus, fisheries with increased dominance of pelagic species are generally more sensitive to changes in environmental conditions from climate change (Planque et al., 2010). Although species with higher turnover rates may theoretically have more capacity to adapt evolutionarily to environmental changes (Jones and Cheung, 2018), the scope and rate of such adaptive response for most fishes are unclear (Munday et al., 2013). Also, over-exploited fish stocks with largely reduced abundance may also have reduced genetic diversity and variability, and consequently the population will have a reduced scope for adaptation under climate change. (11) The protection of habitats and ecosystems (protection) refers to the conservation of habitats and ecosystems, primarily through marine protected areas (MPAs). For example, increased abundance of marine species is expected to enhance productivity of the surrounding areas which can help buffer against climate impacts and increase resilience (Roberts et al., 2017).
In the category “manipulation of biological and ecological adaptation” of organisms and ecosystems to the changing ocean conditions, two measures are assessed. (12) Assisted evolution involves large-scale genetic modification, captive breeding and release of organisms with enhanced stress tolerance (van Oppen et al., 2015). (13) Relocation and reef restoration involves not only the restoration of degraded coral and oyster reefs (e.g., van Oppen et al., 2017), but also their enhancement and active relocation, with the potential creation of new habitats and use of more resilient species or strains. Note that restoration and protection of vegetated coastal habitats (seagrasses, mangroves, and saltmarshes) is considered in the vegetation measure.
Other ocean-based measures have been proposed but little research has been conducted on their potential. They include large-scale seaweed aquaculture for supplementing cattle feed to reduce methane emissions and counteract acidification locally (Machado et al., 2016; Duarte, 2017). Abiotic methods of removing or stripping CO2 from seawater have also been proposed or demonstrated in the laboratory (Eisaman et al., 2012; Willauer et al., 2014; Koweek et al., 2016), as well as marine-based interventions that increase uptake and reduce emissions of other greenhouse gases such as CH4 and N2O (e.g., Poffenbarger et al., 2011; Stolaroff et al., 2012). Research and testing of new, unconventional methods of ocean and climate management are in their infancy, and additional methods are likely to emerge.
Whereas some of the solutions assessed here are still at a very-early or experimental stage, others have been implemented and refined over many decades, though not always specifically designed to address climate change impacts. The global implementation of renewable energy, vegetation, eliminating overexploitation, and protection exhibit a sharp acceleration in the past two decades (Figure 3). For example, global cumulative offshore wind potential has grown 3-fold in less than 5 years to reach 15,000 MW in 2016 (Global Wind Energy Council, 2016). MPAs now cover more than 3% of the global ocean (Boonzaier and Pauly, 2016), 7% of the overexploited fish stocks have been rebuilt (Kleisner et al., 2013) and the global area of avoided loss of mangroves has been estimated at 40,000 km2 (Hamilton and Casey, 2016).
FIGURE 3. Contemporary history of the global implementation of some ocean solutions. (A) Recent changes in the global cumulative offshore wind potential (European Wind Energy Association, 2011; Global Wind Energy Council, 2016), global cumulative surface of ocean iron fertilization experiment patches (Yoon et al., 2016), global area of avoided loss of mangroves (Valiela et al., 2001; Hamilton and Casey, 2016), rebuilding of fish stocks (Kleisner et al., 2013) (in % of total fish stocks), and global cumulative surface of MPAs (Boonzaier and Pauly, 2016) (in % of the global ocean surface). (B) Future progress needed to reach full implementation of targets for all measures above, i.e., 300 EJ year-1 for offshore wind, all ocean high nutrient and low chlorophyll areas for iron fertilization, 10 and 30% of the global ocean for MPAs (Convention for Biological Diversity, 2010; O’Leary et al., 2017), all overexploited and collapsed fish stocks in the process of rebuilding (in 2014, 46% of the total fish stock was overexploited or collapsed) (Cheung et al., 2017), and pre-disturbance extent of mangroves (Valiela et al., 2001). (C) Launch date of some major international conventions or protocols providing governance frameworks for the solutions described in panel (A). For example, initially dealing with marine pollution, the London Convention and the London Protocol also prohibit ocean iron fertilization (except for research purposes).
To estimate effectiveness, we first assess the potential of each measure –assumed here to be implemented at its maximum physical capacity– to bridge the gap between the high-emissions trajectory (RCP8.5, our baseline scenario) and a stringent emission-reduction scenario (RCP2.6) expected to keep mean global temperature increase below 2°C by 2100 (van Vuuren et al., 2011) (see section “SM2” of the Supplementary Materials). The differences between RCP8.5 and RCP2.6 in the year 2100 are estimated to be ∼1,400 Pg C for avoided emissions; ∼2°C for reduced sea surface warming; ∼0.25 pH units for avoided sea surface acidification; and a reduction in sea-level rise of between 0.26 and 1.1 m (Jones et al., 2013; DeConto and Pollard, 2016).
The effectiveness of the global measures is assessed in terms of maximum possible effectiveness to reduce ocean warming, ocean acidification, and sea-level rise (Figure 4A), and duration of the effect (Figure 4B). This maximum effectiveness is theoretical and almost certainly not achievable but provides the full potential of each approach. Two of the global solutions, renewable energy and alkalinization, stand out as having the highest theoretical potential for addressing all drivers (Figure 4A). This is obvious for renewable energy because of the enormous energy potential of tides, waves, ocean currents, and thermal stratification, estimated at up to 7,400 EJ year-1 (Rogner et al., 2000; Lewis et al., 2011) and well exceeding future human energy needs. Any replacement of fossil fuels by marine renewables results in permanently avoided greenhouse gas emissions.
FIGURE 4. Assessment of ocean-based measures to address key ocean drivers. Scores 1 to 5: very low, low, moderate (thicker circle), high, and very high. Confidence levels of the potential effectiveness to moderate ocean warming, ocean acidification, and sea-level rise are shown in panel (A) (1∗ to 5∗; very low, low, moderate, high, very high; see section “SM2.1” of the Supplementary Materials). Details on the assessment can be found in section “SM3” of the Supplementary Materials.
A similarly large and permanent intervention could be provided by large-scale alkalinization, by which CO2 is consumed and stored either as dissolved bicarbonate and carbonate ions or as precipitated calcium carbonate, neutralizing ocean acidity. However, the feasibility and benefits of doing this must be weighed against the financial costs and environmental impacts of mining or producing vast quantities of alkaline material, distributed at global scales, and the potential biotic impacts of the trace elements or contaminants that alkalinity might contain (Renforth and Henderson, 2017).
Land-ocean hybrid methods greatly expand the mitigation potential offered by either land-based or ocean-based approaches individually. For example, the use of marine biomass for bioenergy with carbon capture and storage (BECCS) fuel eliminates limitations on terrestrial fuel capacity posed by competition for land, water, and nutrients. In turn, conversion of CO2 from land-based biomass energy to ocean alkalinity and subsequent storage in the ocean greatly expands CO2 storage capacity and beneficial use (via countering ocean acidification) relative to more conventional CCS approaches. However, a comprehensive understanding of the full range of options, and their costs, benefits and tradeoffs requires further research (Rau, 2014).
Albedo enhancement also has a very large potential effectiveness in moderating warming (Figure 4A), as a relatively small enhancement of the albedo of the dark ocean surface by less than 0.05 could compensate the entire GHG-driven perturbation in the Earth’s radiation balance (Crook et al., 2016; Garciadiego Ortega and Evans, 2018). However, the duration of the effect is only as long as the albedo stays high, likely to be days to months for ocean foams (Figure 4B) and, as SRM in general, it does not limit ocean acidification as atmospheric CO2 concentration remains elevated (Tjiputra et al., 2016). Similar considerations apply to marine cloud brightening, although modeling studies indicate more limited effectiveness (Kravitz et al., 2013; Stjern et al., 2017).
Other potential solutions face physical and/or biogeochemical limitations (Figure 4A). A global deployment of iron fertilization for 100 years could sequester a maximum of ∼70 Pg C (ref. Aumont and Bopp, 2006) because other nutrient or light limitations occur when marine algae are iron-replete (Oschlies et al., 2010). Some measures demonstrate limited potential for reducing warming, acidification and sea-level rise at global scales, such as vegetation for instance. Even with very high carbon storage and avoided net emissions, the vegetation measure is constrained by the limited global area of potentially vegetated habitats, although with some scope to artificially expand that area; e.g., via seaweed aquaculture (Duarte et al., 2017; Hawken, 2017).
Local measures have a relatively low effectiveness to reduce warming, acidification, and sea-level rise at the global scale (Figure 4A). However, some have a high to very high effectiveness to moderate local ocean acidification (pollution reduction and alkalinization) and relative sea-level rise (vegetation, protection, restoring hydrology, as well as relocation and reef restoration).
The duration of the effects varies greatly between the different methods (Figure 4B). It is close to permanent for renewables as long as the infrastructure is maintained. The effects of protection are also considered permanent as long as MPAs are enforced, although future climate change will decrease their ability to provide climate mitigation and adaptation benefits (Bruno et al., 2018). The effects of vegetation can be close to permanent as long as these ecosystems are maintained or increased in the face of natural and anthropogenic pressures. In contrast, the effects of fertilization have a finite duration. Once iron fertilization is stopped, a large portion of the additional ocean carbon uptake will outgas back to the atmosphere on decadal to centennial time scales (Aumont and Bopp, 2006). By capturing and storing CO2 for long time periods or permanently, alkalinization and hybrids methods such as conversion of CO2 to ocean alkalinity or marine BECCS generally have long duration of the effect. In contrast, the effect of albedo enhancement and cloud brightening is short-lived (days to weeks). The loss of most benefits following abrupt termination is a characteristic of all SRM schemes (Jones et al., 2013). It is projected to increase both ocean and land temperature velocities to unprecedented speeds (Trisos et al., 2018).
Technical feasibility is evaluated by considering current technological readiness (ranging from schemes at the concept stage to schemes already deployed) and for lead time until full potential effectiveness, i.e., the time needed to reach full implementation (ranging from days to decades; see section “SM2” of the Supplementary Materials). Two local measures have the highest technical feasibility (Figure 4B): protection and restoring hydrology. Vegetation (both global and local) and renewable energy also have a high technical feasibility, closely followed by eliminating overexploitation, reducing pollution and relocation and reef restoration. Five global schemes have the lowest technical feasibility: fertilization, cloud brightening, alkalinization, albedo enhancement, and hybrid methods. The local measure assisted evolution also scores very low on this criterion. These low scores generally reflect lack of testing and deployment at scale, thus they also possess high uncertainty.
The cost effectiveness of the global and local solutions is assessed, in US$ per tonne of CO2 emissions reduced and in US$ per hectare of surface area of implementation, respectively (Figure 4E and section “SM3.5” of the Supplementary Materials). The costs considered here are best estimates from the literature for the direct monetary costs of implementation. The non-monetary costs of implementation are considered through assessing co-benefits, disbenefits, and governability, as discussed below. Since cost effectiveness is a relative metric, it does not reflect the effectiveness of a measure to reduce changes in the drivers. For instance, cloud brightening is cost-effective despite having a moderate maximum effectiveness to moderate ocean warming, ocean acidification, and sea-level rise (Figures 2, 4A). Restoration of vegetation to increase CO2 capture has a very low cost effectiveness but conservation of vegetation to avoid further emissions is very cost-effective. For example, conserving mangroves to avoid further CO2 emissions is considerably cheaper than restoring mangroves to enhance CO2 uptake [4–10 vs. 240 US$/t CO2 (Siikamaki et al., 2012; Bayraktarov et al., 2016)]. Cloud brightening, protection, and renewable energy have the highest cost efficiency while albedo enhancement, vegetation and relocation and reef restoration have the lowest. Note that cost effectiveness generally increases over time and with increasing scale of implementation, due to learning and economies of scale, and that there is uncertainty in many of these estimates (see section “SM3.5” of the Supplementary Materials) as reflected in the low levels of confidence. This generally is a consequence of lack of economic data from testing/deployment of many of these methods at relevant scales.
Governance is the “effort to craft order, thereby to mitigate conflict, and realize mutual gains” (Williamson, 2000) amongst actors from public, private, and civil society sectors. Here, we assess the governability of global and local ocean measures in terms of the potential capability of the international community to implement them, managing associated conflicts and harnessing mutual benefits (see section “SM2.9” of the Supplementary Materials). We focus on the international dimension of decision and action to reflect the global scope of the study, despite the fact that we recognize that global and local measures do not face the same constrains for implementation – e.g., bi- or multi-lateral diplomatic issues for the former (e.g., Smit, 2014; Cinner et al., 2016; Rabitz, 2016) and local institutional and population reluctance challenges for the latter (e.g., Cinner et al., 2016).
On that basis, the governability of a scheme increases with its effectiveness (Ostrom, 2007), the predictability of its effects (Hagedorn, 2008; Ostrom, 2009), its co-benefits, the absence of disbenefits together with the presence of national-level net benefits, the presence of enabling institutions and the absence of constraining institutions, and higher normative consensus amongst relevant actors (Abbott and Snidal, 1998; Barrett, 2005). Global governability is likely to be much higher when there are national-level net benefits (i.e., national benefits outweigh the negative environmental impacts and national costs of implementation), since single nation states may then implement measures without having to rely on international cooperation (Kaul et al., 1999). This is the case for protection, vegetation as well as for relocation and reef restoration (Figure 4E and section “SM3.6” of the Supplementary Materials). Conversely, ocean-based SRM measures (cloud brightening and albedo enhancement), while being more effective in addressing drivers globally, are considered to have low governability because their implementation generally involves international cooperation to solve the free-riding dilemma with regard to global public goods (Pasztor et al., 2017). Thus nations are likely to be reluctant to unilaterally take on extra costs that may reduce their own economic competitiveness (Preston, 2013; Rabitz, 2016; Williamson and Bodle, 2016). Additionally, SRM measures entail potentially significant disbenefits and high uncertainties (Figures 4D, 5; sections “SM3.4 and SM3.4.3” of the Supplementary Materials), which further reduce their present governability. Renewable energy is in an intermediate position: renewables are becoming more economically competitive compared with fossil-fuel based energy, thereby providing national-level incentives to implementation. Taken together, the scores exhibit a fundamental tradeoff in climate policy: global measures are more effective than local ones in addressing the climate problem, but they are in general more difficult to implement due to challenges in global governance.
Reducing the climate-related impacts depends on two attributes, the effectiveness to reduce exposure to warming, acidification, and sea-level rise (Figure 4A; sections “SM3.1 and SM3.2” of the Supplementary Materials) and the sensitivity of ecosystems to changes in these drivers (Figure 1; section “SM3.3”). Differences in these attributes lead to different reduction of impacts both among drivers and ecosystems (Figure 5). For example, renewable energy consistently scores very high in its combined effectiveness to reduce the impacts because it reduces exposure to all three drivers. In contrast, relocation and reef restoration is one of the less effective measures in reducing impacts because, despite the fact that restoration can reduce relative sea-level rise, it does not necessarily reduce exposure to ocean warming and acidification in situ unless the relocation involves species or habitat transfers to localities that are cooler and/or have higher pH. Another example is albedo enhancement, the effectiveness of which is very high to reduce the impacts of warming, high for sea-level rise and very low for acidification. Thus aside from solutions like massive and rapid deployment of marine renewable energy, multiple and in some cases non-traditional solutions targeting different drivers may be needed, the combination of which will be ecosystem-specific. For example, solutions that target warming and acidification are more important to reduce the impacts on coral reefs and Arctic biota, whereas solutions that are most effective to reduce the impacts of sea-level rise will be more relevant for mangroves and saltmarshes.
FIGURE 5. Contribution of ocean-based solutions to reduce the impacts of key ocean drivers on key ecosystems (A–D) and ecosystem services (E–H). The combined potential effectiveness represents the average potential effectiveness to reduce the impacts of ocean warming, ocean acidification, and sea-level rise (see section “SM3” of the Supplementary Materials). Scores 1 to 5: very low, low, moderate (thicker circle), high, and very high.
While the most effective measures to reduce exposure to all three drivers are the global ones (Figure 4A), they do not generally reduce the sensitivity of the ecosystems to climate-related drivers. In contrast, local solutions have low or moderate effectiveness to reduce changes in climate-related drivers. They aim to moderate impacts primarily through reducing non-climatic drivers that affect the health and resilience of coastal ecosystems and marine environments such as pollution, overexploitation, overfishing, and coastal development (Halpern et al., 2015). Thus, local solutions have a high level of co-benefits and generally induce a low level of disbenefits since many have a long history of successfully mitigating non-climate stressors – the value of which is considered in this study as co-benefits (Figure 5). The most effective measures across all ecosystems (high to very high effectivenesses to reduce the impacts of ocean warming, ocean acidification, and sea-level rise; Figure 4C) are renewable energy, alkalinization, hybrid methods, vegetation (local) and albedo enhancement, with renewable energy showing the greatest combined effectiveness. Protection, restoring hydrology, and eliminating overexploitation also score relatively high to reduce impacts on seagrass habitats, mangroves and saltmarshes (Figure 5). Relocation and reef restoration and cloud brightening consistently have the lowest combined potential effectiveness; however, if reef restoration were considered separately from relocation, it would score higher (especially with regard to reducing local relative sea-level rise).
The potential to reduce the impacts of non-climatic drivers is a key attribute of local measures because it increases the resilience of ecosystems to climate change (O’Leary et al., 2017). For example, protection and eliminating overexploitation can support high reproductive outputs and juvenile recruitment following climate-related mass mortalities, allowing for population recovery from extreme events (Micheli et al., 2012; Roberts et al., 2017). Moreover, these measures produce co-benefits, such as spillover benefits of MPAs to adjacent areas supporting shellfish fisheries and aquaculture, and few, if any disbenefits, especially for coral reefs and vegetated marine habitats (Roberts et al., 2017). Some MPAs are more affected by coral bleaching than fished areas because they harbor more thermally sensitive corals (Graham et al., 2008) but there is a strong case that protected coral reefs recover better (Cinner et al., 2013).
Whilst local solutions can decrease the total (climate- and non-climate related) impacts and improve ecosystems’ resilience, they cannot eliminate all of the climate-related component of impacts. For example, water quality and fishing pressure had minimal effect on the unprecedented bleaching of 2016 (Hughes et al., 2017b). Furthermore, and despite local protections, the changes associated with a high CO2 emission scenario will result in further habitat and species losses throughout low-latitude and tropical MPAs, for example through the effects of warming on habitat-forming species such as corals, thereby reducing their beneficial roles (Bruno et al., 2018).
Despite the fact that most solutions implemented at local scales have a limited effectiveness to reduce the impacts of warming, acidification, and sea-level rise globally, they all have some beneficial effects, which could help in countering global climate impact if scaled beyond their current implementation. For example, seaweeds and seagrasses can reduce ocean acidification locally (e.g., Unsworth et al., 2012; Mcleod et al., 2013) and can potentially buffer adjacent coral populations by off-setting decreases in seawater pH (Camp et al., 2016).
Sensitive ecosystem services are also expected to benefit from the implementation of measures that have the highest potential effectiveness in addressing climate drivers globally, such as renewable energy and alkalinization (Figure 4A). Our assessment, however, suggests that these measures may also lead to significant disbenefits (Figures 5B–E, sections “SM3.4 and SM3.4.3” of the Supplementary Materials). For instance, the addition of non-carbonate alkaline minerals may perturb biogeochemical processes though the release of mineral constituents such as cadmium, nickel, chromium, iron, and silicon (Hartmann et al., 2013). This may alter the pattern of primary and secondary production, and increase contaminant accumulation along the food chain (Russell et al., 2012; Alava et al., 2017), possibly impacting fisheries and aquaculture production, and the coastal protection value of coastal habitats. Furthermore, alkalinization is only moderately effective in reducing the impacts of sea-level rise, which is the primary driver affecting mangroves and saltmarshes. A similar conclusion applies to most of the global measures, notably cloud brightening and albedo enhancement, where large-scale deployment may risk high levels of disbenefits. In contrast, although our assessment suggests that large-scale renewable energy may lead to some local collateral damages on ecosystem services when these systems are deployed in coastal ecosystems, these impacts may be largely moderated through careful planning and consultation (Pelc and Fujita, 2002). In contrast, minimal damage is anticipated for deep-water floating systems currently being tested.
Measures that are most effective to reduce climate-related drivers locally (e.g., relative sea-level rise) often also have the dual benefit of minimizing the impacts from non-climatic drivers affecting coastal and marine ecosystems and environments (e.g., pollution, overexploitation, overfishing, and coastal development). As a result, the most effective local-scale interventions to maintain healthy conditions for fin fisheries, fish and bivalve aquaculture, and coastal protection are eliminating overexploitation, restoring hydrology, reducing pollution, vegetation, and protection (Figure 5). Modeling studies indeed suggest that the increase in stock abundance and productivity by effective management of fisheries and conservation of fish stocks (Costello et al., 2016) is likely to compensate losses from climate change (Cheung et al., 2017). It was shown that sustainable mangrove management interventions support surface elevation gains, thus limiting relative sea-level rise (Sasmito et al., 2016). More generally, protection and vegetation enable mangroves, saltmarshes, coral reefs, and seagrass to reduce the impacts of sea-level rise on coastal communities through wave attenuation and shoreline stabilization. Maintaining the health of ecosystems that provide coastal protection also has significant additional co-benefits to local human communities (e.g., carbon sequestration, water filtering, tourism, food security, recreation; Barbier et al., 2011; Weatherdon et al., 2016), in addition to supporting their resilience to climate impacts (Carilli et al., 2009). It is not surprising then that many countries are actively including marine ecosystems in their national climate plans as shown by the Nationally Determined Contributions submitted under the Paris Agreement (Gallo et al., 2017).
A principal components analysis (see section “SM4” of the Supplementary Materials) was used to reduce the eight dimensions of our assessment dataset defined by the scoring criteria to two latent dimensions that explain most of the variance in the assessment data. Three clusters of schemes emerge (Figure 6). The first one includes alkalinization at the global scale, hybrid methods, albedo enhancement, and cloud brightening, which show high potential effectiveness to reduce warming and acidification, and their impacts. However, there has been relatively little research, testing and application on such solutions, and they generally score low for technological readiness, co-benefits, lack of disbenefits, and global governability. In contrast, the second cluster includes almost all local measures (protection, reducing pollution, vegetation at the local scale, eliminating overexploitation, restoring hydrology and relocation and restoration), and is characterized by low effectiveness to reduce warming and its impacts, and moderate effectiveness to reduce ocean acidification and relative sea-level rise and their impacts. These measures are, however, technologically ready, have significant co-benefits, few disbenefits and can also help to reduce the impacts of non-climatic drivers. Renewable energy stands apart as it exhibits both high potential effectiveness and technology readiness, thus ranging in between clusters 1 and 2. The third cluster includes assisted evolution, alkalinization at the local scale and fertilization, which have low to moderate scores across most criteria assessed.
FIGURE 6. Principal components analysis (PCA) of the attributes of ocean-based solutions. (A) Correlations among criteria, some being averaged across ecosystems and ecosystem services. When two arrows point in the same direction, the criteria are correlated: the scores of most solutions are similar for these two criteria (e.g., both warming-related criteria, co-benefits, and readiness). When they point in opposite directions, criteria are anti-correlated (e.g., moderate warming and global governability). When they are perpendicular, criteria are uncorrelated (e.g., acidification-related criteria and readiness). (B) Positions of solutions in the PCA. Solutions on the right have high scores in the criteria that point to the right and low scores in the attributes that point to the left; a similar reasoning can be made for any direction in this space. Solutions are clustered into three groups, through hierarchical clustering based on their position in the PCA space, and colored accordingly. The first two principal components explain 65% of the variance in the attributes of ocean-based solutions. Attributes or solutions that are not well represented in this space are shown in smaller font (representativity or “Repr.” varies between 0 and 1). See section “SM5” of the Supplementary Materials for details on the PCA approach, and sections “SM3 and SM4” for additional information on the assessment.
Measures which are the most technically feasible also have the highest global governability (Figure 4). They comprise protection, eliminating overexploitation, reducing pollution, vegetation, relocation and reef restoration, and renewable energy. Except for the latter, all these measures are local. Their governability is high to very high except for restoring hydrology and assisted evolution (moderate or low). Global measures such as albedo enhancement, fertilization, hybrid methods, cloud brightening, and alkalinization have a lower overall technical feasibility, partly due to lack of testing and experience, together with moderate to low governability. Yet none of these schemes do much to reduce or moderate the impacts of the climate-related drivers considered in this study (ocean warming, ocean acidification and sea-level rise).
Such conclusions highlight the need for multiple-scale and multiple-stakeholder initiatives, hence calling for improved international governance mechanisms to ensure coherency in ocean-based climate action. These governance challenges are, however, constrained by controversies on the potential solutions, which scientific investigations and policy engagement can help overcome. Controversies are mostly in the “addressing the causes of climate change” and “solar radiation management” areas of action (Figure 2). They include: the moral hazard dilemma, i.e., that development and deployment of alternative solutions might decrease effort on emission reductions (Preston, 2013; McLaren, 2016); the risk of premature lock-in of suboptimal solutions and path dependencies (Burns et al., 2016; Reynolds et al., 2016); and concerns regarding controllability and transnational effects (Williamson and Bodle, 2016). Ethical issues are also important, relating to informed consent and potential adverse impacts on countries unable to deploy such measures (Svoboda, 2012; Suarez and van Aalst, 2017; Rahman et al., 2018); and vested interests, as production and deployment of innovative measures could be a highly profitable market (Preston, 2013). Controversies related to the “protection of biota and ecosystems” and “manipulation to enhance biological and ecological adaptation” areas of action mostly arise from conflicts relating to local, national and global-scale interests, and the balance between short-term and long-term benefits and disbenefits (Cooley et al., 2016; Cormier-Salem and Panfili, 2016). Such trade-offs between “winners” and “losers” highlight the influence of social norms and values that may differ greatly between different stakeholders (Hopkins et al., 2016; Lubchenco et al., 2016). Testing the veracity of such perceptions via further research and demonstration of novel measures at relevant scales will clarify governance issues.
The global implementation or testing of renewable energy, fertilization, vegetation, eliminating overexploitation, and protection has accelerated sharply in the past two decades (Figure 3). In particular, several local measures (vegetation, protection, and eliminating overexploitation) may achieve their full potential in a few decades at their current rate of deployment.
Nevertheless, the scale of deployment for most solutions remains far below what would be necessary to effectively address climate change drivers and impacts (Figure 4B). Delivering the full potential of global measures such as renewable energy, alkalinization, and hybrid methods requires orders-of-magnitude increases in their research, testing, and deployment. Such action is considered urgent on the basis of the climatic threats to ocean sustainability (Gattuso et al., 2015), and since there are decadal lag times until full maximum effectiveness of all the global measures considered here (Figure 4B and section “SM3.1” of the Supplementary Materials). In the meantime, there will likely be significant increases in climate-related impacts on ocean ecosystems and services, which will reduce ecosystems’ ability to provide local solutions (Albright et al., 2016; Cheung et al., 2016; Cinner et al., 2016), thereby decreasing leeway for action (Gattuso et al., 2015).
It is clear that the familiar and conventional marine management strategies cannot fully counter climate change and its impacts. Accelerating research and deployment of other potential solutions will, however, challenge the capacity of science, policy, and decision-making in evaluating and deploying solutions. Defining road maps to drastically enhance action faces major constraints relating to the large uncertainties in key non-climatic variables. Thus socioeconomic conditions may flip the balance between fossil fuel markets and renewables, potentially catalyzing a rapid acceleration of the deployment of marine renewables, but not necessarily with adequate consideration of local disbenefits. There is also a need to consider a broader range of measures than those assessed here, many of which are still in their infancy and unfamiliar to marine management (e.g., large-scale seaweed aquaculture, or abiotic methods of removing or stripping CO2 from seawater). This calls for the development of policies and funding to foster and promote research into new or emerging ocean and climate management options.
Current pledges under the Paris Agreement are insufficient to hold the global average temperature increase to well below 2°C above pre-industrial levels, calling for a dramatic increase in global mitigation effort. However, even with a full and timely implementation of the Agreement, major impacts on sensitive marine ecosystems such as coral reefs and Arctic biota are expected, requiring additional, ambitious and rapid actions to address climate-related drivers locally, minimize their impacts, and increase resilience. To support efforts to address the ocean’s potential contribution to these mitigation and adaptation goals, our assessment highlights five evidence-based key messages.
First, each measure has tradeoffs. For example, alkalinization scores high in global mitigation potential, but low in technological readiness or global governability. In contrast, measures implemented locally such as protection and reducing pollution have strong co-benefits and high governability, but have a much lower effectiveness to moderate changes in climate-related drivers. Decisions favoring any measure must therefore consider multiple criteria, including effectiveness, feasibility, co-benefits, disbenefits, governability, and cost effectiveness, rather than only the climate-related effectiveness or cost effectiveness.
Second, ocean-based measures with relatively high global effectiveness (such as albedo enhancement) have significant adverse side effects on key marine ecosystems and services. In contrast, local measures rank higher in terms of global governability, co-benefits and lack of disbenefits, and have a moderate ability to reduce climate-related impacts, only offering local opportunities for mitigation. The emerging picture is that actions in addition to local and more conventional marine management are needed to increase chances of avoiding or countering climate impacts. It is unlikely that a single measure will be able to meet a pathway consistent with the Paris Agreement. The introduction of multiple measures, including land-based ones, would require deployment of each of them at decreased scales relative to single-measure deployments, and would also reduce the risk of side effects (see also Minx et al., 2018).
Third, some measures that offer greater effectiveness in countering climate and its impacts (e.g., alkalinization, cloud brightening, albedo enhancement, and assisted evolution) currently exhibit too many uncertainties to be recommended for large-scale deployment until more research is conducted. However, measures with demonstrated potential effectiveness, co-benefits and with no or few disbenefits (renewable energy as well as other local solutions except assisted evolution) are no-regret measures that can be widely deployed immediately, as other potential solutions are explored. The high merits of renewable energy is consistent with the conventional policy approach that the best way to avoid climate impacts (on the marine environment, as well as elsewhere) is to eliminate the primary driver, excess atmospheric CO2 concentration, by drastically reducing CO2 emissions (Gattuso et al., 2015).
Fourth, climate change intervention at multiple scales requires that multiple and diverse actors are involved, hence calling for coordination across scales. Interestingly, besides being central to decisions on global measures, our assessment suggests that the international community can also play an indirect supporting role to the implementation of local solutions. The international community must therefore accelerate diplomatic and political efforts, especially within institutions such as the UNFCCC and the UN Convention on Biological Diversity, to improve existing arrangements or find new ones, and develop facilitative mechanisms for global to local action.
Fifth, since there are controversies and uncertainties on many of the measures we considered, a better scientific understanding of solution benefits, disbenefits, costs, and suitable governance arrangements is needed to inform policy and decision making. For example, 41% of the scores have low to very low levels of confidence (see section “SM3.4” of the Supplementary Materials). A major area of research thus relies in better determining potential effectiveness, cost-effectiveness, and desirability under various greenhouse gas emission scenarios. Furthermore, given the social challenges involved in all potential solutions, social science research is needed for understanding factors that hinder or promote effective and fair governance of ocean-based solutions (Magnan et al., 2016). In turn, this will allow a balanced consideration of new, unconventional ideas (e.g., regional cloud brightening to reduce pressures on coral reefs, advanced hybrid technologies, or innovative governance solutions for reconciling social conflicts associated to measures). This is a prerequisite for providing decision- and policy-makers with robust information, for example through the various products of the sixth assessment cycle of the IPCC. As new knowledge and insights become available, it is key that scientists effectively engage with the general public and decision makers, especially discussing the potential, feasibility, tradeoffs and social preferences of specific measures, and the consequences of failing to deploy solutions on time. This will notably help to increase mutual understanding and serve to reduce confusion and misinformation regarding the realized and future impacts of climate change on the ocean (Gelcich et al., 2014).
Both the marine policy and science communities need to recognize the uncertainties and limitations of currently available climate and ocean management options; support the immediate development of the most promising ones, e.g., renewable energy and local actions that can be scaled up; and acknowledge that new or emerging measures that are not part of current marine management practice might, through further research and testing, prove cost-effective as well as environmentally and socially acceptable.
J-PG, AKM, LB, WWLC, CMD, JH, EM, FM, AO, PW, RB, VIC, RDG, JJM, H-OP, and GHR designed and carried out the research. All co-authors conducted the analyses and wrote the paper.
This is a product of “The Oceans Solutions Initiative”, an expert group supported by the Prince Albert II of Monaco Foundation, the Ocean Acidification International Coordination Centre of the International Atomic Energy Agency, the Veolia Foundation, and the French Facility for Global Environment. AKM thanks the French Government for its support under the “Investissements d’avenir” programme, managed by the French National Research Agency (ANR-10-LABX-14-01). RB was supported by the RESCCUE project funded by the French Development Agency and the French Global Environment Facility (AFD CZZ 2205 01 W and FFEM CZZ 1667 01 H). WWLC acknowledges funding support from the Nippon Foundation-UBC Nereus Program. CMD participation was partly supported by King Abdullah University of Science and Technology (KAUST) through baseline funding. JH was partly supported by the European Union’s Horizon 2020 research and innovation programme under grant agreement number 642018 (GREEN-WIN project) and by the grant SEASCAPE from the Deutsche Forschungsgemeinschaft (DFG) as part of the Special Priority Program (SPP)-1889 “Regional Sea Level Change and Society” (SeaLevel). EM was supported by the Nature Conservancy and the International Climate Initiative (IKI) funded by the German Federal Ministry for the Environment, Nature Conservation, Building and Nuclear Safety. FM was partly supported by the US NSF (DEB-1212124 and OCE-1736830) and JJM by the Netherlands Earth System Science Center. The contents in this manuscript are solely the opinions of the authors and do not constitute a statement of policy, decision, or position on behalf of the Pacific Community.
The authors declare that the research was conducted in the absence of any commercial or financial relationships that could be construed as a potential conflict of interest.
We thank M. Khamla (CNRS) and S. Ghani (King Abdullah University of Science and Technology) for help with artwork.
The Supplementary Material for this article can be found online at: https://www.frontiersin.org/articles/10.3389/fmars.2018.00337/full#supplementary-material
Abbott, K. W., and Snidal, D. (1998). Why states act through formal international organizations. J. Conflict Resolut. 42, 3–32. doi: 10.1177/0022002798042001001
Alava, J. J., Cheung, W. W. L., Ross, P. S., and Sumaila, U. R. (2017). Climate change-contaminant interactions in marine food webs: toward a conceptual framework. Global Change Biol. 23, 3984–4001. doi: 10.1111/gcb.13667
Albright, R., Anthony, K. R., Baird, M., Beeden, R., Byrne, M., Collier, C., et al. (2016). Ocean acidification: linking science to management solutions using the great barrier reef as a case study. J. Environ. Manage. 182, 641–650. doi: 10.1016/j.jenvman.2016.07.038
Albright, R., Takeshita, Y., Koweek, D. A., Ninokawa, A., Wolfe, K., Rivlin, T., et al. (2018). Carbon dioxide addition to coral reef waters suppresses net community calcification. Nature 555, 516–519. doi: 10.1038/nature25968
Anderson K., and Peters G. (2016). The trouble with negative emissions. Science 354, 182–183. doi: 10.1126/science.aah4567
Andersson, A. J., and Gledhill, D. (2013). Ocean acidification and coral reefs: effects on breakdown, dissolution, and net ecosystem calcification. Annu. Rev. Mar. Sci. 5, 321–348. doi: 10.1146/annurev-marine-121211-172241
Aumont, O., and Bopp, L. (2006). Globalizing results from ocean in situ iron fertilization studies. Global Biogeochem. Cycles 20:GB2017. doi: 10.1029/2005GB002591
Barbier, E. B., Hacker, S. D., Kennedy, C., Koch, E. W., Stier, A. C., and Silliman, B. R. (2011). The value of estuarine and coastal ecosystem services. Ecol. Monogr. 81, 169–193. doi: 10.1890/10-1510.1
Barrett, S. (2005). The theory of international environmental agreements. Handb. Environ. Econ. 3, 1457–1516. doi: 10.1016/S1574-0099(05)03028-7
Barton, A., Hales, B., Waldbusser, G. G., Langdon, C., and Feely, R. A. (2012). The Pacific oyster, Crassostrea gigas, shows negative correlation to naturally elevated carbon dioxide levels: Implications for near-term ocean acidification effects. Limnol. Oceanogr. 57, 698–710. doi: 10.4319/lo.2012.57.3.0698
Bates, A. E., Stuart-Smith, R. D., Barrett, N. S., and Edgar, G. J. (2017). Biological interactions both facilitate and resist climate-related functional change in temperate reef communities. Proc. R. Soc. Lon. Ser. B Biol. Sci. 284:20170484. doi: 10.1098/rspb.2017.0484
Bayraktarov, E., Saunders, M. I., Abdullah, S., Mills, M., Beher, J., Possingham, H. P., et al. (2016). The cost and feasibility of marine coastal restoration. Ecol. Appl. 26, 1055–1074. doi: 10.1890/15-1077
Billé, R., Kelly, R., Biastoch, A., Harrould-Kolieb, E., Herr, D., Joos, F., et al. (2013). Taking action against ocean acidification: a review of management and policy options. Environ. Manage. 52, 761–779. doi: 10.1007/s00267-013-0132-7
Boonzaier, L., and Pauly, D. (2016). Marine protection targets: an updated assessment of global progress. Oryx 50, 27–35. doi: 10.1017/S0030605315000848
Bopp, L., Resplandy, L., Orr, J. C., Doney, S. C., Dunne, J. P., Gehlen, M., et al. (2013). Multiple stressors of ocean ecosystems in the 21st century: projections with CMIP5 models. Biogeosciences 10, 6225–6245. doi: 10.5194/bg-10-6225-2013
Boyd, P. W., Collins, S., Dupont, S., Fabricius, K., Gattuso, J. P., Havenhand, J., et al. (2018). Experimental strategies to assess the biological ramifications of multiple drivers of global ocean change - a review. Global Change Biol. 24, 2239–2261. doi: 10.1111/gcb.14102
Boysen, L. R., Lucht, W., Gerten, D., Heck, V., Lenton, T. M., and Schellnhuber H. J. (2017). The limits to global-warming mitigation by terrestrial carbon removal. Earth’s Future 5, 463–474. doi: 10.1002/2016EF000469
Bruno, J. F., Bates, A. E., Cacciapaglia, C., Pike, E. P., Amstrup, S. C., van Hooidonk, R., et al. (2018). Climate change threatens the world’s marine protected areas. Nat. Clim. Change 8, 499–503. doi: 10.1038/s41558-018-0149-2
Burns, E. T., Flegal, J. A., Keith, D. W., Mahajan, A., Tingley, D., and Wagner, G. (2016). What do people think when they think about solar geoengineering? A review of empirical social science literature, and prospects for future research. Earth’s Future 4, 536–542. doi: 10.1002/2016EF000461
Cai, W.-J., Hu, X., Huang, W.-J., Murrell, M. C., Lehrter, J. C., Lohrenz, S. E., et al. (2011). Acidification of subsurface coastal waters enhanced by eutrophication. Nat. Geosci. 4, 766–770. doi: 10.1021/es300626f
Callaway, R., Shinn, A. P., Grenfell, S. E., Bron, J. E., Burnell, G., Cook, E. J., et al. (2012). Review of climate change impacts on marine aquaculture in the UK and Ireland. Aquat. Conserv. Mar. Freshw. Ecosyst. 22, 389–421. doi: 10.1098/rspb.2015.1453
Camp, E. F., Suggett, D. J., Gendron, G., Jompa, J., Manfrino, C., and Smith, D. J. (2016). Mangrove and seagrass beds provide different biogeochemical services for corals threatened by climate change. Front. Mar. Sci. 3:52. doi: 10.3389/fmars.2016.00052
Carilli, J. E., Norris, R. D., Black, B. A., Walsh, S. M., and McField, M. (2009). Local stressors reduce coral resilience to bleaching. PLoS One 4:e6324. doi: 10.1371/journal.pone.0006324
Cheung, W. W. L., Jones, M. C., Lam, V. W. Y., Miller, D. D., Ota, Y., Teh, L., et al. (2017). Transform high seas management to build climate resilience in marine seafood supply. Fish Fisheries. 18, 254–263. doi: 10.1111/faf.12177
Cheung, W. W. L., Reygondeau, G., and Frölicher, T. L. (2016). Large benefits to marine fisheries of meeting the 1.5°C global warming target. Science 354, 1591–1594. doi: 10.1126/science.aag2331
Cheung, W. W. L., Watson, R., Morato, T., Pitcher, T. J., and Pauly, D. (2007). Intrinsic vulnerability in the global fish catch. Mar. Ecol. Progr. Ser. 333, 1–12. doi: 10.3354/meps333001
Cinner, J. E., Huchery, C., Darling, E. S., Humphries, A. T., Graham, N. A., Hicks, C. C., et al. (2013). Evaluating social and ecological vulnerability of coral reef fisheries to climate change. PLoS One 8:e74321. doi: 10.1371/journal.pone.0074321
Cinner, J. E., Huchery, C., MacNeil, M. A., Graham, N. A., McClanahan, T. R., Maina, J., et al. (2016). Bright spots among the world’s coral reefs. Nature 535, 416–419. doi: 10.1038/nature18607
Comeau, S., Jeffree, R., Teyssié, J.-L., and Gattuso, J.-P. (2010). Response of the Arctic pteropod Limacina helicina to projected future environmental conditions. PLoS One 5:e11362. doi: 10.1371/journal.pone.0011362
Convention for Biological Diversity (2010). COP 10 Decision X/2: The Strategic Plan for Biodiversity 2011-2020 – And the Aichi Biodiversity Targets. Nagoya: United Nations Environment Programme, 7.
Cooley, S. R., Ono, C. R., Melcer, S., and Robertson, J. (2016). Community-level actions that can address ocean acidification. Front. Mar. Sci. 2:128. doi: 10.3389/fmars.2015.00128
Cormier-Salem, M. C., and Panfili, J. (2016). Mangrove reforestation: greening or grabbing coastal zones and deltas? Case studies in senegal. Afr. J. Aquat. Sci. 41, 89–98. doi: 10.2989/16085914.2016.1146122
Costello, C., Ovando, D., Clavelle, T., Strauss, C. K., Hilborn, R., Melnychuk, M. C., et al. (2016). Global fishery prospects under contrasting management regimes. Proc. Natl. Acad. Sci. U.S.A. 113, 5125–5129. doi: 10.1073/pnas.1520420113
Crain, C. M., Kroeker, K., and Halpern, B. S. (2008). Interactive and cumulative effects of multiple human stressors in marine systems. Ecol. Lett. 11, 1304–1315. doi: 10.1111/j.1461-0248.2008.01253.x
Crook, J. A., Jackson, L. S., and Forster, P. M. (2016). Can increasing albedo of existing ship wakes reduce climate change? J. Geophys. Res. Atmos. 121, 1549–1558. doi: 10.1002/2015JD024201
DeConto, R. M., and Pollard, D. (2016). Contribution of Antarctica to past and future sea-level rise. Nature 531, 591–597. doi: 10.1038/nature17145
Diana, J. S. (2009). Aquaculture production and biodiversity conservation. BioScience 59, 27–38. doi: 10.1525/bio.2009.59.1.7
Duarte, C. M. (2017). Reviews and syntheses: hidden forests, the role of vegetated coastal habitats in the ocean carbon budget. Biogeosciences 14, 301–310. doi: 10.5194/bg-14-301-2017
Duarte, C. M., Agustí, S., Wassmann, P., Arrieta, J. M., Alcaraz, M., Coello, A., et al. (2012). Tipping elements in the Arctic marine ecosystem. Ambio 41, 44–55. doi: 10.1007/s13280-011-0224-7
Duarte, C. M., Losada, I. J., Hendriks, I. E., Mazarrasa, I., and Marbà, N. (2013). The role of coastal plant communities for climate change mitigation and adaptation. Nat. Clim. Change 3, 961–968. doi: 10.1371/journal.pone.0044727
Duarte, C. M., Wu, J., Xiao, X., Bruhn, A., and Krause-Jensen, D. (2017). Can seaweed farming play a role in climate change mitigation and adaptation. Front. Mar. Sci. 62:1541–1551. doi: 10.3389/fmars.2017.00100
Eisaman, M. D., Parajuly, K., Tuganov, A., Eldershaw, C., Chang, N., and Littau, K. A. (2012). CO2 extraction from seawater using bipolar membrane electrodialysis. Energy Environ. Sci. 5:7346. doi: 10.1039/c2ee03393c
Ferrario, F., Beck, M. W., Storlazzi, C. D., Micheli, F., Shepard, C. C., and Airoldi, L. (2014). The effectiveness of coral reefs for coastal hazard risk reduction and adaptation. Nat. Commun. 5:3794. doi: 10.1038/ncomms4794
Field, C. B., and Mach, K. J. (2017). Rightsizing carbon dioxide removal. Science 356, 706–707. doi: 10.1126/science.aam9726
Fonseca, M. S., and Cahalan, J. A. (1992). A preliminary evaluation of wave attenuation by four species of seagrass. Estuar. Coast. Shelf Sci. 35, 565–576. doi: 10.1016/S0272-7714(05)80039-3
Frieler, K., Meinshausen, M., Golly, A., Mengel, M., Lebek, K., Donner, S. D., et al. (2013). Limiting global warming to 2°C is unlikely to save most coral reefs. Nat. Clim. Change 3, 165–170. doi: 10.1038/nclimate1674
Gallo, N. D., Victor, D. G., and Levin, L. A. (2017). Ocean commitments under the Paris Agreement. Nat. Clim. Change 7, 833–838. doi: 10.1038/nclimate3422
Garciadiego Ortega, E., and Evans, J. R. G. (2018). On the energy required to maintain an ocean mirror using the reflectance of foam. J. Eng. Marit. Environ. (in press). doi: 10.1177/1475090217750442
Gattuso, J.-P., Brewer, P., Hoegh-Guldberg, O., Kleypas, J. A., Pörtner, H.-O., and Schmidt, D. (2014). “Ocean acidification,” in Climate Change 2014: Impacts, Adaptation, and Vulnerability. Part A: Global and Sectoral Aspects. Contribution of Working Group II to the Fifth Assessment Report of the Intergovernmental Panel on Climate Change, eds C. B. Field, V. R. Barros, D. J. Dokken, K. J. Mach, M. D. Mastrandrea, T. F. Bilir et al. (New York, NY: Cambridge University Press), 129–131.
Gattuso, J.-P., Magnan, A., Billé, R., Cheung, W. W. L., Howes, E. L., Joos, F., et al. (2015). Contrasting futures for ocean and society from different anthropogenic CO2 emissions scenarios. Science 349:aac4722. doi: 10.1126/science.aac4722
Gazeau, F., Parker, L. M., Comeau, S., Gattuso, J.-P., O’Connor, W., Martin, S., et al. (2013). Impacts of ocean acidification on marine shelled molluscs. Mar. Biol. 160, 2207–2245. doi: 10.1007/s00227-013-2219-3
Gelcich, S., Buckley, P., Pinnegar, J. K., Chilvers, J., Lorenzoni, I., Terry, G., et al. (2014). Public awareness, concerns, and priorities about anthropogenic impacts on marine environments. Proc. Natl. Acad. Sci. U.S.A. 111, 15042–15047. doi: 10.1073/pnas.1417344111
Global Wind Energy Council (2016). Global Wind Report 2016. Brussels: Global Wind Energy Council, 73.
Gobler, C. J., DePasquale, E. L., Griffith, A. W., and Baumann, H. (2014). Hypoxia and acidification have additive and synergistic negative effects on the growth, survival, and metamorphosis of early life stage bivalves. PLoS One 9:e83648. doi: 10.1371/journal.pone.0083648
Graham, N. A., McClanahan, T. R., MacNeil, M. A., Wilson, S. K., Polunin, N. V., Jennings, S., et al. (2008). Climate warming, marine protected areas and the ocean-scale integrity of coral reef ecosystems. PLoS One 3:e3039. doi: 10.1371/journal.pone.0003039
Griscom, B. W., Adams, J., Ellis, P. W., Houghton, R. A., Lomax, G., Miteva, D. A., et al. (2017). Natural climate solutions. Proc. Natl. Acad. Sci. U.S.A. 114, 11645–11650. doi: 10.1073/pnas.1710465114
Hagedorn, K. (2008). Particular requirements for institutional analysis in nature-related sectors. Eur. Rev. Agric. Econ. 35, 357–384. doi: 10.1093/erae/jbn019
Halpern, B. S., Frazier, M., Potapenko, J., Casey, K. S., Koenig, K., Longo, C., et al. (2015). Spatial and temporal changes in cumulative human impacts on the world’s ocean. Nat. Commun. 6:7615. doi: 10.1038/ncomms8615
Hamilton, S. E., and Casey, D. (2016). Creation of a high spatio-temporal resolution global database of continuous mangrove forest cover for the 21st century (CGMFC-21). Global Ecol. Biogeogr. 25, 729–738. doi: 10.1111/geb.12449
Hansen, J., Sato, M., Kharecha, P., von Schuckmann, K., Beerling, D. J., Cao, J., et al. (2017). Young people’s burden: requirement of negative CO2 emissions. Earth Syst. Dynam. 8, 577–616. doi: 10.5194/esd-8-577-2017
Hartmann, J., West, J., Renforth, P., Köhler, P., De La Rocha, C. L., Wolf-Gladrow, D. A., et al. (2013). Enhanced chemical weathering as a geoengineering strategy to reduce atmospheric carbon dioxide, a nutrient source and to mitigate ocean acidification. Rev. Geophys. 51, 113–149. doi: 10.1002/rog.20004
Hawken, P. (2017). Drawdown: the Most Comprehensive Plan Ever Proposed to Reverse Global Warming. New York, NY: Penguin Books, 256.
Herr, D., and Landis, E. (2016). Coastal Blue Carbon Ecosystems. Opportunities for Nationally Determined Contributions. Policy Brief. Gland: International Union for the Conservation of Nature, 28.
Hoegh-Guldberg, O., Cai, R., Brewer, P., Fabry, V., Hilmi, K., Jung, S., et al. (2014). “The ocean,” in Climate Change 2014: Impacts, Adaptation, and Vulnerability. Part B: Regional Aspects. Contribution of Working Group II to the Fifth Assessment Report of the Intergovernmental Panel on Climate Change, eds C. B. Field, V. R. Barros, D. J. Dokken, K. J. Mach, M. D. Mastrandrea, T. F. Bilir et al. (New York, NY: Cambridge University Press), 1655–1731.
Hoegh-Guldberg, O., Mumby, P. J., Hooten, A. J., Steneck, R. S., Greenfield, P., Gomez, E., et al. (2007). Coral reefs under rapid climate change and ocean acidification. Science 318, 1737–1742. doi: 10.1126/science.1152509
Hopkins, C. R., Bailey, D. M., and Potts, T. (2016). Perceptions of practitioners: managing marine protected areas for climate change resilience. Ocean Coast. Manage. 128, 18–28. doi: 10.1016/j.ocecoaman.2016.04.014
Howard, R. J., Day, R. H., Krauss, K. W., From, A. S., Allain, L., and Cormier, N. (2017). Hydrologic restoration in a dynamic subtropical mangrove-to-marsh ecotone: hydrologic restoration in a mangrove-marsh ecotone. Restor. Ecol. 25, 471–482. doi: 10.1111/rec.12452
Hughes, T. P., Barnes, M. L., Bellwood, D. R., Cinner, J. E., Cumming, G. S., Jackson, J. B. C., et al. (2017a). Coral reefs in the Anthropocene. Nature 546, 82–90. doi: 10.1038/nature22901
Hughes, T. P., Kerry, J. T., Álvarez-Noriega, M., Álvarez-Romero, J. G., Anderson, K. D., Baird, A. H., et al. (2017b). Global warming and recurrent mass bleaching of corals. Nature 543, 373–377. doi: 10.1038/nature21707
Hunt, G. L., Drinkwater, K. F., Arrigo, K., Berge, J., Daly, K. L., Danielson, S., et al. (2016). Advection in polar and sub-polar environments: Impacts on high latitude marine ecosystems. Progr. Oceanogr. 149, 40–81. doi: 10.1016/j.pocean.2016.10.004
Jones, C., Robertson, E., Arora, V., Friedlingstein, P., Shevliakova, E., Bopp, L., et al. (2013). Twenty-first-century compatible CO2 emissions and airborne fraction simulated by CMIP5 earth system models under four representative concentration pathways. J. Clim. 26, 4398–4413. doi: 10.1175/JCLI-D-12-00554.1
Jones, M. C., and Cheung, W. W. L. (2018). Using fuzzy logic to determine the vulnerability of marine species to climate change. Global Change Biol. 24, e719–e731. doi: 10.1111/gcb.13869
Jordà, G., Marbà, N., and Duarte, C. M. (2012). Mediterranean seagrass vulnerable to regional climate warming. Nat. Clim. Change 2, 821–824. doi: 10.1038/nclimate1533
Kaul, I., Grunberg, I., and Stern, M. A. (1999). Global Public Goods: International Cooperation in the 21st Century. New York, NY: Oxford University Press, xxxviii, 546. doi: 10.1093/0195130529.001.0001
Kirwan, M. L., and Megonigal, J. P. (2013). Tidal wetland stability in the face of human impacts and sea-level rise. Nature 504, 53–60. doi: 10.1038/nature12856
Kleisner, K., Zeller, D., Froese, R., and Pauly, D. (2013). Using global catch data for inferences on the world’s marine fisheries. Fish Fish. 14, 293–311. doi: 10.1111/j.1467-2979.2012.00469.x
Kohlbach, D., Schaafsma, F. L., Graeve, M., Lebreton, B., Lange, B. A., David, C., et al. (2017). Strong linkage of polar cod (Boreogadus saida) to sea ice algae-produced carbon: evidence from stomach content, fatty acid and stable isotope analyses. Progr. Oceanogr. 152, 62–74. doi: 10.1016/j.pocean.2017.02.003
Koweek, D. A., Mucciarone, D. A., and Dunbar, R. B. (2016). Bubble stripping as a tool to reduce high dissolved CO2 in coastal marine ecosystems. Environ. Sci. Technol. 50, 3790–3797. doi: 10.1021/acs.est.5b04733
Krause-Jensen, D., and Duarte, C. M. (2014). Expansion of vegetated coastal ecosystems in the future Arctic. Front. Mar. Sci. 1:77. doi: 10.3389/fmars.2014.00077
Kravitz, B., Forster, P. M., Jones, A., Robock, A., Alterskjær, K., Boucher, O., et al. (2013). Sea spray geoengineering experiments in the geoengineering model intercomparison project (GeoMIP): experimental design and preliminary results. J. Geophys. Res. Atmos. 118:186. doi: 10.1002/jgrd.50856
Latham, J., Bower, K., Choularton, T., Coe, H., Connolly, P., Cooper, G., et al. (2012). Marine cloud brightening. Philos. Trans. R. Soc. Lon. Ser. A Mathemat. Phys. Eng. Sci. 370, 4217–4262. doi: 10.1098/rsta.2012.0086
Latham, J., Kleypas, J., Hauser, R., Parkes, B., and Gadian, A. (2013). Can marine cloud brightening reduce coral bleaching? Atmos. Sci. Lett. 14, 214–219. doi: 10.1002/asl2.442
Le Quéré, C., Andrew, R. M., Friedlingstein, P., Sitch, S., Pongratz, J., Manning, A. C., et al. (2018). Global carbon budget 2017. Earth Syst. Sci. Data 10, 405–448. doi: 10.5194/essd-10-405-2018
Lewis, A., Estefen, S., Huckerby, J., Musial, W., Pontes, T., Torres-Martinez, J., et al. (2011). “Ocean energy,” in Renewable Energy Sources and Climate Change Mitigation. Special Report of the Intergovernmental Panel on Climate Change, eds O. Edenhofer, R. Pichs-Madruga, Y. Sokona, K. Seyboth, P. Matschoss, S. Kadner et al. (Cambridge: Cambridge University Press), 497–534.
Lovelock, C. E., Cahoon, D. R., Friess, D. A., Guntenspergen, G. R., Krauss, K. W., Reef, R., et al. (2015). The vulnerability of Indo-Pacific mangrove forests to sea-level rise. Nature 526, 559–563. doi: 10.1038/nature15538
Lubchenco, J., Cerny-Chipman, E. B., Reimer, J. N., and Levin, S. A. (2016). The right incentives enable ocean sustainability successes and provide hope for the future. Proc. Nat. Acad. Sci. U.S.A. 113, 14507–14514. doi: 10.1073/pnas.1604982113
Machado, L., Magnusson, M., Paul, N. A., Kinley, R., de Nys, R., and Tomkins, N. (2016). Dose-response effects of Asparagopsis taxiformis and Oedogonium sp. on in vitro fermentation and methane production. J. Appl. Phycol. 28, 1443–1452. doi: 10.1007/s10811-015-0639-9
Mackenzie, C. L., Ormondroyd, G. A., Curling, S. F., Ball, R. J., Whiteley, N. M., and Malham, S. K. (2014). Ocean warming, more than acidification, reduces shell strength in a commercial shellfish species during food limitation. PLoS One 9:e86764. doi: 10.1371/journal.pone.0086764
Magnan, A. K., Colombier, M., Billé, R., Hoegh-Guldberg, O., Joos, F., Pörtner, H.-O., et al. (2016). Implications of the paris agreement for the ocean. Nat. Clim. Change 6, 732–735. doi: 10.1038/nclimate3038
Marbà, N., and Duarte, C. M. (2010). Mediterranean warming triggers seagrass (Posidonia oceanica) shoot mortality. Global Change Biol. 16, 2366–2375. doi: 10.1111/j.1365-2486.2009.02130.x
Martin, S., Rodolfo-Metalpa, R., Ransome, E., Rowley, S., Gattuso, J.-P., and Hall-Spencer, J. (2008). Effects of naturally acidified seawater on seagrass calcareous epibionts. Biol. Lett. 4, 689–692. doi: 10.1098/rsbl.2008.0412
McLaren, D. (2016). Mitigation deterrence and the “moral hazard” of solar radiation management. Earth’s Future 4, 596–602. doi: 10.1002/2016EF000445
Mcleod, E., Anthony, K. R. N., Andersson, A., Beeden, R., Golbuu, Y., Kleypas, J., et al. (2013). Preparing to manage coral reefs for ocean acidification: lessons from coral bleaching. Front. Ecol Environ. 11:20–27. doi: 10.1890/110240
Mcleod, E., Chmura, G. L., Bouillon, S., Salm, R., Björk, M., Duarte, C. M., et al. (2011). A blueprint for blue carbon: toward an improved understanding of the role of vegetated coastal habitats in sequestering CO2. Front. Ecol. Environ. 9:552–560. doi: 10.1890/110004
Micheli, F., Saenz-Arroyo, A., Greenley, A., Vazquez, L., Espinoza Montes, J. A., Rossetto, M., et al. (2012). Evidence that marine reserves enhance resilience to climatic impacts. PLoS One 7:e40832. doi: 10.1371/journal.pone.0040832
Minx, J. C., Lamb, W. F., Callaghan, M. W., Fuss, S., Hilaire, J., Creutzig, F., et al. (2018). Negative emissions—Part 1: research landscape and synthesis. Environ. Res. Lett. 13:063001. doi: 10.1088/1748-9326/aabf9b
Munday, P. L., Pratchett, M. S., Dixson, D. L., Donelson, J. M., Endo, G. G. K., Reynolds, A. D., et al. (2013). Elevated CO2 affects the behavior of an ecologically and economically important coral reef fish. Mar. Biol. 160, 2137–2144. doi: 10.1007/s00227-012-2111-6
Narayan, S., Beck, M. W., Wilson, P., Thomas, C., Guerrero, A., Shepard, C., et al. (2016). Coastal Wetlands and Flood Damage Reduction: Using Risk Industry-Based Models to assess natural defenses in the Northeastern USA. London: Lloyd’s Tercentenary Research Foundation, 23.
National Research Council (2015a). Climate Intervention: Carbon Dioxide Removal and Reliable Sequestration. Washington, D.C.: National Academies Press, 140.
National Research Council (2015b). Climate Intervention: Reflecting Sunlight to Cool Earth. Washington, DC: National Academy of Sciences, 220.
O’Leary, J. K., Micheli, F., Airoldi, L., Boch, C., De Leo, G., Elahi, R., et al. (2017). The resilience of marine ecosystems to climatic disturbances. BioScience 67, 208–220. doi: 10.1093/biosci/biw161
Oschlies, A., Koeve, W., Rickels, W., and Rehdanz, K. (2010). Side effects and accounting aspects of hypothetical large-scale Southern Ocean iron fertilization. Biogeosciences 7, 4017–4035. doi: 10.5194/bg-7-4017-2010
Ostrom, E. (2007). A diagnostic approach for going beyond panaceas. Proc. Natl. Acad. Sci. U.S.A. 104, 15181–15187. doi: 10.1073/pnas.0702288104
Ostrom, E. (2009). A general framework for analyzing sustainability of social-ecological systems. Science 325, 419–422. doi: 10.1126/science.1172133
Pandolfi, J. M., Bradbury, R. H., Sala, E., Hughes, T. P., Bjorndal, K. A., Cooke, R. G., et al. (2003). Global trajectories of the long-term decline of coral reef ecosystems. Science 301, 955–958. doi: 10.1126/science.1085706
Pasztor, J., Scharf, C., and Schmidt, K.-U. (2017). How to govern geoengineering? Science 357:231. doi: 10.1126/science.aan6794
Pelc, R., and Fujita, R. M. (2002). Renewable energy from the ocean. Mar. Policy 26, 471–479. doi: 10.1016/S0308-597X(02)00045-3
Planque, B., Fromentin, J.-M., Cury, P., Drinkwater, K. F., Jennings, S., Perry, R. I., et al. (2010). How does fishing alter marine populations and ecosystems sensitivity to climate. J. Mar. Syst. 79, 403–417. doi: 10.1016/j.jmarsys.2008.12.018
Poffenbarger, H. J., Needelman, B. A., and Megonigal, J. P. (2011). Salinity influence on methane emissions from tidal marshes. Wetlands 31, 831–842. doi: 10.1007/s13157-011-0197-0
Pörtner H.-O., Karl D., Boyd P., Cheung W., Lluch-Cota S. E., Nojiri Y., et al. (2014). “Ocean systems,” in Climate Change 2014: Impacts, Adaptation, and Vulnerability. Part A: Global and Sectoral Aspects. Contribution of Working Group II to the Fifth Assessment Report of the Intergovernmental Panel on Climate Change, eds C. B. Field, V. R. Barros, D. J. Dokken, K. J. Mach, M. D. Mastrandrea, T. F. Bilir et al. (New York, NY: Cambridge University Press), 411–484.
Preston, C. J. (2013). Ethics and geoengineering: reviewing the moral issues raised by solar radiation management and carbon dioxide removal. Wiley Interdiscipl. Rev. Clim. Change 4, 23–37. doi: 10.1002/wcc.198
Rabitz, F. (2016). Going rogue? Scenarios for unilateral geoengineering. Futures 84, 98–107. doi: 10.1016/j.futures.2016.11.001
Rahman, A. A., Artaxo, P., Asrat, A., and Parker, A. (2018). Developing countries must lead on solar geoengineering research. Nature 556, 22–24. doi: 10.1038/d41586-018-03917-8
Rau, G. H. (2011). CO2 mitigation via capture and chemical conversion in seawater. Environ. Sci. Technol. 45, 1088–1092. doi: 10.1021/es102671x
Rau, G. H. (2014). “Enhancing the ocean’s role in CO2 mitigation in global environmental change,” in Handbook of Global Environmental Pollution, Vol. 1, ed. B. Freedman (Dordrecht: Springer), 817–824.
Rau, G. H., McLeod, E. L., and Hoegh-Guldberg, O. (2012). The need for new ocean conservation strategies in a high-carbon dioxide world. Nat. Clim. Change 2, 720–724. doi: 10.1038/nclimate1555
Raven, J. A., and Beardall, J. (2014). CO2 concentrating mechanisms and environmental change. Aquat. Bot. 118, 24–37. doi: 10.1016/j.aquabot.2014.05.008
Renforth, P., and Henderson, G. (2017). Assessing ocean alkalinity for carbon sequestration. Rev. Geophys. 55, 636–674. doi: 10.1002/2016RG000533
Reynolds, J. L., Parker, A., and Irvine, P. (2016). Five solar geoengineering tropes that have outstayed their welcome: five solar geoengineering tropes. Earth’s Future 4, 562–568. doi: 10.1002/2016EF000416
Riebesell, U., and Gattuso, J.-P. (2015). Lessons learned from ocean acidification research. Nat. Clim. Change 5, 12–14. doi: 10.1038/nclimate2456
Roberts, C. M., O’Leary, B. C., McCauley, D. J., Cury, P. M., Duarte, C. M., Lubchenco, J., et al. (2017). Marine reserves can mitigate and promote adaptation to climate change. Proc. Natl. Acad. Sci. U.S.A. 114, 6167–6175. doi: 10.1073/pnas.1701262114
Rockström, J., Gaffney, O., Rogelj, J., Meinshausen, M., Nakicenovic, N., and Schellnhuber, H. J. (2017). A roadmap for rapid decarbonization. Science 355, 1269–1271. doi: 10.1126/science.aah3443
Rogelj, J., den Elzen, M., Höhne, N., Fransen, T., Fekete, H., Winkler, H., et al. (2016). Paris agreement climate proposals need a boost to keep warming well below 2°C. Nature 534, 631–639. doi: 10.1038/nature18307
Rogner, H. H., Barthel, F., Cabrera, M., Faaij, A., Giroux, M., Hall, D., et al. (2000). “Energy resources,” in World Energy Assessment: Energy and the Challenge of Sustainability, ed. UNDP (New York, NY: World Energy Council), 135–171.
Russell, L. M., Rasch, P. J., Mace, G. M., Jackson, R. B., Shepherd, J., Liss, P., et al. (2012). Ecosystem impacts of geoengineering: a review for developing a science plan. Ambio 41, 350–369. doi: 10.1007/s13280-012-0258-5
Sanz-Pérez, E. S., Murdock, C. R., Didas, S. A., and Jones, C. W. (2016). Direct capture of CO2 from ambient air. Chem. Rev. 116, 11840–11876. doi: 10.1021/acs.chemrev.6b00173
Sasmito, S. D., Murdiyarso, D., Friess, D. A., and Kurnianto, S. (2016). Can mangroves keep pace with contemporary sea level rise? A global data review. Wetlands Ecol. Manage. 24, 263–278. doi: 10.1007/s11273-015-9466-7
Siikamaki, J., Sanchirico, J. N., and Jardine, S. L. (2012). Global economic potential for reducing carbon dioxide emissions from mangrove loss. Proc. Natl. Acad. Sci. U.S.A. 109, 14369–14374. doi: 10.1073/pnas.1200519109
Smit, E. C. (2014). Geoengineering: issues of accountability in international law. Nevada Law J. 15, 1060–1089.
Smith, P., Davis, S. J., Creutzig, F., Fuss, S., Minx, J., Gabrielle, B., et al. (2016). Biophysical and economic limits to negative CO2 emissions. Nat. Clim. Change 6, 42–50. doi: 10.1038/nclimate2870
Spalding, M. D., Ruffo, S., Lacambra, C., Meliane, I., Hale, L. Z., Shepard, C. C., et al. (2014). The role of ecosystems in coastal protection: adapting to climate change and coastal hazards. Ocean Coast. Manag. 90, 50–57. doi: 10.1016/j.ocecoaman.2013.09.007
Spencer, T., Schuerch, M., Nicholls, R. J., Hinkel, J., Lincke, D., Vafeidis, A. T., et al. (2016). Global coastal wetland change under sea-level rise and related stresses: the DIVA Wetland Change Model. Global Planet. Change 139, 15–30. doi: 10.1016/j.gloplacha.2015.12.018
Stjern, C. W., Muri, H., Ahlm, L., Boucher, O., Cole, J. N. S., Ji, D., et al. (2017). Response to marine cloud brightening in a multi-model ensemble. Atmos. Chem. Phys. 18, 621–634. doi: 10.5194/acp-18-621-2018
Stolaroff, J. K., Bhattacharyya, S., Smith, C. A., Bourcier, W. L., Cameron-Smith, P. J., and Aines, R. D. (2012). Review of methane mitigation technologies with application to rapid release of methane from the Arctic. Environ. Sci. Technol. 46, 6455–6469. doi: 10.1021/es204686w
Strand, S. E., and Benford, G. (2009). Ocean sequestration of crop residue carbon: recycling fossil fuel carbon back to deep sediments. Environ. Sci. Technol. 43, 1000–1007. doi: 10.1021/es8015556
Suarez, P., and van Aalst, M. K. (2017). Geoengineering: a humanitarian concern. Earth’s Future 5, 183–195. doi: 10.1002/2016EF000464
Svoboda, T. (2012). The ethics of geoengineering: moral considerability and the convergence hypothesis. J. Appl. Philos. 29, 243–256. doi: 10.1111/j.1468-5930.2012.00568.x
Thomsen, J., Stapp, L. S., Haynert, K., Schade, H., Danelli, M., Lannig, G., et al. (2017). Naturally acidified habitat selects for ocean acidification–tolerant mussels. Sci. Adv. 3:e1602411. doi: 10.1126/sciadv.1602411
Tjiputra, J. F., Grini, A., and Lee, H. (2016). Impact of idealized future stratospheric aerosol injection on the large-scale ocean and land carbon cycles. J. Geophys. Res. Biogeosci. 121, 2–27. doi: 10.1002/2015JG003045
Trisos, C. H., Amatulli, G., Gurevitch, J., Robock, A., Xia, L., and Zambri, B. (2018). Potentially dangerous consequences for biodiversity of solar geoengineering implementation and termination. Nat. Ecol. Evol. 2, 475–482. doi: 10.1038/s41559-017-0431-0
Troell, M., Naylor, R. L., Metian, M., Beveridge, M., Tyedmers, P. H., Folke, C., et al. (2014). Does aquaculture add resilience to the global food system. Proc. Natl. Acad. Sci. U.S.A. 111, 13257–13263. doi: 10.1073/pnas.1404067111
Unsworth, R. K. F., Collier, C. J., Henderson, G. M., and McKenzie, L. J. (2012). Tropical seagrass meadows modify seawater carbon chemistry: implications for coral reefs impacted by ocean acidification. Environ. Res. Lett. 7:024026. doi: 10.1088/1748-9326/7/2/024026
Valiela, I., Bowen, J. L., and York, J. K. (2001). Mangrove forests: one of the world’s threatened major tropical environments. BioScience 51, 807–815. doi: 10.1641/0006-3568(2001)051[0807:MFOOTW]2.0.CO;2
van Hooidonk, R., Maynard, J., Tamelander, J., Gove, J., Ahmadia, G., Raymundo, L., et al. (2016). Local-scale projections of coral reef futures and implications of the Paris agreement. Sci. Rep. 6:39666. doi: 10.1038/srep39666
van Oppen, M. J. H., Gates, R. D., Blackall, L. L., Cantin, N., Chakravarti, L. J., Chan, W. Y., et al. (2017). Shifting paradigms in restoration of the world’s coral reefs. Global Change Biol. 23, 3437–3448. doi: 10.1111/gcb.13647
van Oppen, M. J. H., Oliver, J. K., Putnam, H. M., and Gates, R. D. (2015). Building coral reef resilience through assisted evolution. Proc. Natl. Acad. Sci. U.S.A. 112, 2307–2313. doi: 10.1073/pnas.1422301112
van Vuuren, D. P., Edmonds, J., Kainuma, M., Riahi, K., Thomson, A., Hibbard, K., et al. (2011). The representative concentration pathways: an overview. Clim. Change 109, 5–31. doi: 10.1007/s10584-011-0148-z
Ward, R. D., Friess, D. A., Day, R. H., and MacKenzie, R. A. (2016). Impacts of climate change on mangrove ecosystems: a region by region overview. Ecosyst. Health Sustainability 2:e01211. doi: 10.1002/ehs2.1211
Wassmann, P., Duarte, C. M., Agustí, S., and Sejr, M. K. (2010). Footprints of climate change in the Arctic marine ecosystem. Global Change Biol. 17, 1235–1249. doi: 10.1111/j.1365-2486.2010.02311.x
Waycott, M., Duarte, C. M., Carruthers, T. J. B., Orth, R. J., Dennison, W. C., Olyarnik, S., et al. (2009). Accelerating loss of seagrasses across the globe threatens coastal ecosystems. Proc. Natl. Acad. Sci. U.S.A. 106:12377. doi: 10.1073/pnas.0905620106
Weatherdon, L. V., Magnan, A. K., Rogers, A. D., Sumaila, U., and Cheung, W. W. (2016). Observed and projected impacts of climate change on marine fisheries, aquaculture, coastal tourism, and human health: an update. Front. Mar. Sci. 3:48. doi: 10.3389/fmars.2016.00048
Willauer, H. D., DiMascio, F., Hardy, D. R., and Williams, F. W. (2014). Feasibility of CO2 extraction from seawater and simultaneous hydrogen gas generation using a novel and robust electrolytic cation exchange module based on continuous electrodeionization technology. Ind. Eng. Chem. Res. 53, 12192–12200. doi: 10.1021/ie502128x
Williamson, O. E. (2000). The new institutional economics: taking stock, looking ahead. J. Econ. Lit. 38, 595–613. doi: 10.1257/jel.38.3.595
Williamson, P., and Bodle, R. (2016). Update on Climate Geoengineering in Relation to the Convention on Biological Diversity: Potential Impacts and Regulatory Framework, CBD Technical Series No. 84. Montreal: Secretariat of the Convention on Biological Diversity, 158.
Winemiller, K. O. (2005). Life history strategies, population regulation, and implications for fisheries management. Can. J. Fish. Aquat. Sci. 62, 872–885. doi: 10.1139/f05-040
Yates, K. K., Zawada, D. G., Smiley, N. A., and Tiling-Range, G. (2017). Divergence of seafloor elevation and sea level rise in coral reef ecosystems. Biogeosciences 14, 1739–1772. doi: 10.5194/bg-14-1739-2017
Yoon, J. -E., Yoo, K. -C., Macdonald, A. M., Yoon, H. I., Park, K. -T., Yang, E. -J., et al. (2016). Ocean iron fertilization experiments: past–present–future with introduction to Korean Iron Fertilization Experiment in the Southern Ocean (KIFES) Project. Biogeosciences Discuss. doi: 10.5194/bg-2016-472
Keywords: climate change, ocean acidification, ocean solutions, global, local, governance
Citation: Gattuso J-P, Magnan AK, Bopp L, Cheung WWL, Duarte CM, Hinkel J, Mcleod E, Micheli F, Oschlies A, Williamson P, Billé R, Chalastani VI, Gates RD, Irisson J-O, Middelburg JJ, Pörtner H-O and Rau GH (2018) Ocean Solutions to Address Climate Change and Its Effects on Marine Ecosystems. Front. Mar. Sci. 5:337. doi: 10.3389/fmars.2018.00337
Received: 27 June 2018; Accepted: 03 September 2018;
Published: 04 October 2018.
Edited by:
Peng Xiu, South China Sea Institute of Oceanology (CAS), ChinaReviewed by:
Anthony J. Richardson, The University of Queensland, AustraliaCopyright © 2018 Gattuso, Magnan, Bopp, Cheung, Duarte, Hinkel, Mcleod, Micheli, Oschlies, Williamson, Billé, Chalastani, Gates, Irisson, Middelburg, Pörtner and Rau. This is an open-access article distributed under the terms of the Creative Commons Attribution License (CC BY). The use, distribution or reproduction in other forums is permitted, provided the original author(s) and the copyright owner(s) are credited and that the original publication in this journal is cited, in accordance with accepted academic practice. No use, distribution or reproduction is permitted which does not comply with these terms.
*Correspondence: Jean-Pierre Gattuso, Z2F0dHVzb0BvYnMtdmxmci5mcg==
Disclaimer: All claims expressed in this article are solely those of the authors and do not necessarily represent those of their affiliated organizations, or those of the publisher, the editors and the reviewers. Any product that may be evaluated in this article or claim that may be made by its manufacturer is not guaranteed or endorsed by the publisher.
Research integrity at Frontiers
Learn more about the work of our research integrity team to safeguard the quality of each article we publish.