- 1Sorbonne Université, UMR 7208 BOREA (CNRS), Paris, France
- 2UMR6197 Laboratoire de Microbiologie des Environnements Extrêmes and Laboratoire Environnement Profond, Ifremer (UBO, CNRS) CS 10070, Plouzané, France
- 3UMR 8187 Laboratoire d'Océanologie et Géosciences (Univ. Lille, CNRS, Univ. Côte d'Opale), Wimereux, France
- 4Sorbonne Université, UFR927 and UF918, Paris, France
- 5Muséum National d'Histoire Naturelle – UMR7245 (MNHN CNRS) Mécanismes de Communication et Adaptation des Micro-organismes (MCAM), Paris, France
- 6Institut Universitaire de France, Paris, France
Mussels within the subfamily Bathymodiolinae, in particular the larger Bathymodiolus species (sensu lato) thriving at cold seeps and hydrothermal vents, are among the most iconic fauna to colonize deep-sea reducing habitats globally. Fuelled by energy derived from chemosynthetic symbioses, their contribution to ecosystem productivity is conspicuous, with many bathymodioline species forming dense, extensive aggregates. Chemosymbiotic mussels play crucial roles as ecosystem engineers, both through the formation of spatially heterogeneous biogenic reefs and in redistributing reduced-fluid emissions. The notable absence of Bathymodiolinae outside of reducing ecosystems affirms their dependency on these ephemeral habitats, placing spatiotemporal constraints on dispersal to, and colonization of nascent, chemosynthetically active substrata. Thus, although symbioses may explain why these mussels are so productive in deep-sea reducing habitats, species' survival over successive generations depends largely upon the adaptive characteristics of their lifecycle as a whole. Despite accumulating data on the biology and ecology of adults however, details remain fragmented regarding earlier developmental junctures during their development. This paper therefore brings together results from research undertaken over recent years on this topic, providing a synthesis of various lifecycle aspects of bathymodiolins from the earliest stages of development, gametogenesis, through to sexual maturity, including the intrinsic, emerging role of symbionts. The review provides a comprehensive overview of our current understanding and identifies areas where further study into these keystone organisms is warranted. The benefits of applying an integrated, lifecycle approach when evaluating the potential impacts of global change and anthropogenic activities upon deep-sea fauna and their habitats are then discussed.
Introduction
Deep-sea habitats subject to reducing environmental conditions are colonized by a diverse array of specialist and generalist metazoan fauna, supported by chemosynthetic microbial primary production. Composed of bacteria and archeae, these primary producers are capable of metabolizing chemically reduced compounds, and so thrive where suitable electron donors (i.e., reduced compounds) and electron acceptors (oxygen) occur simultaneously at anaerobic-aerobic redox interfaces (Cavanaugh et al., 2005; Stewart et al., 2005). Some of the most productive metazoan taxa are those that engage in chemosynthetic bacterial symbioses, where metabolic capabilities of the symbionts deliver energy resources that would otherwise be inaccessible directly to the host.
One of the most iconic and widespread of these taxa are the “deep sea mussels” classified within the subfamily Bathymodiolinae (bathymodioline mussels) found in reducing environments across the globe. A number of independent multi-gene phylogenetic studies have identified several poly- and pararphyletic genera within the Bathymodiolinae (itself paraphyletic, as it currently stands), including Bathymodiolus, Idas, and Adipicola (Lorion et al., 2013; Thubaut et al., 2013b; Liu et al., 2018). Notable examples include the “Bathymodiolus” childressi clade, whose members appear to be more closely related to Gigantidas spp., and several species from the genera Idas, Adipicola, and Terua for which exist a long history of synonyms and, in some cases, the putative erection of new genera (e.g., Nypamodiolus, Thubaut et al., 2013b). Accordingly, quotation marks herein highlight instances where a species is currently classified within a genus known to be inaccurate, while question marks identify morphospecies for which no molecular data exists (e.g., Table 1). The World Register for Marine Species (WoRMS at www.marinespecies.org) lists 80 names of actual and extinct species (not all of them valid) within eight genera, and many more are awaiting formal description. Despite being phylogenetically diverse and differing by orders of magnitude in size (maximum shell lengths range from a few millimeters to almost 40 cm), bathymodiolins display several common characteristics. Most notably, bacterial symbionts have been identified in all but one species investigated, and they provide some-to-all of the hosts nutrition (Duperron, 2010). At many hydrothermal-vent, cold-seep and organic-fall habitats, these symbiotic associations can sustain dense aggregations of mytilids. Beyond the confines of these habitats, the Bathymodiolinae are conspicuously absent. By extension, since these habitats are frequently ephemeral and fragmented, separated by wide expanses of unsuitable oligotrophic habitat, the cessation of reducing conditions can cause bathymodioline populations to collapse entirely. Thus, while symbioses may explain why bathymodiolins are so productive in deep-sea reducing habitats, species survival over successive generations by the repeated colonization of nascent, chemosynthetically active habitat, depends more upon the adaptive characteristics of their lifecycle as a whole.
This paper summarizes and integrates results from research undertaken over recent years, examining aspects of bathymodioline lifecycles along a developmental trajectory from gametogenesis to sexual maturity (Figure 1). This includes adult reproductive biology, fertilization and early development, planktonic larval duration and associated dispersal, larval settlement, and patterns in post-larval growth toward maturity. Although knowledge gaps persist, overall trends in the lifecycles of deep-sea mytilids are presented based on data currently available. Areas where further research is needed are identified, with some discussion concerning current and imminent anthropogenic impacts upon these organisms and their potential consequences for the future of these species.
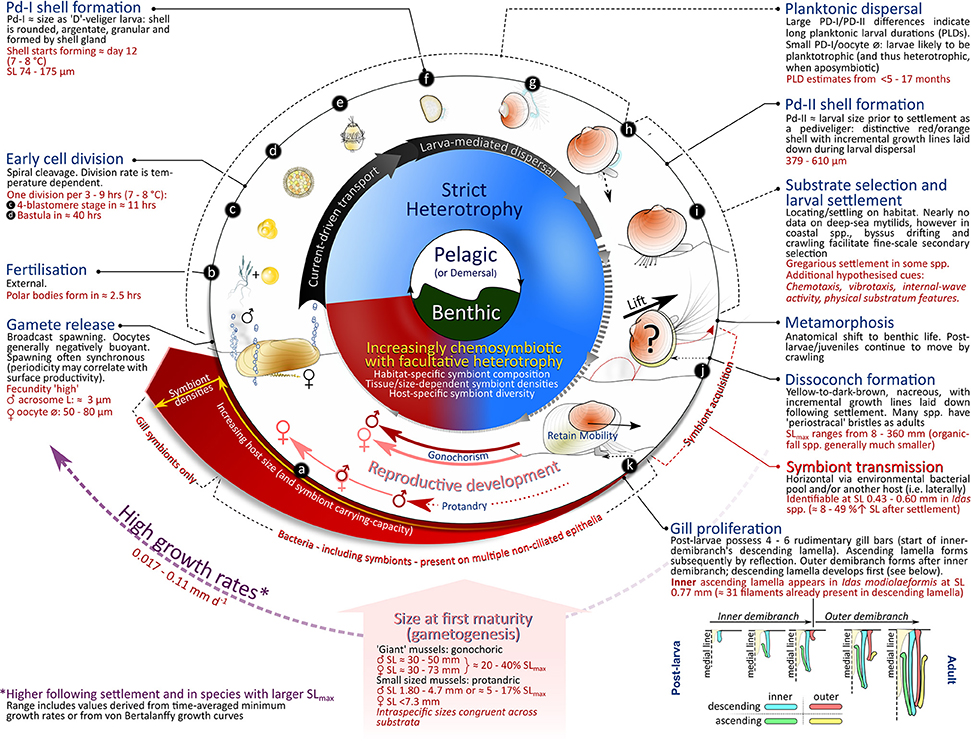
Figure 1. Diagram displaying the full life cycle of bathymodioline mussels, indicating (from center to periphery) habitat, nutritional regime, principal mechanisms of larval transport, illustrations of different developmental stages, critical junctures during the life cycle for which empirical data is available, and status of symbiosis. For further explanation, see main text.
Bathymodiolinae Produce Small Oocytes and Display Complex Sex Determination and Seasonality in Their Reproductive Cycle
Gametogenesis in deep-sea bathymodiolins generally follows the patterns described in documented shallow-water mytilids (Lubey, 1959; Gabbott and Peek, 1991; Eckelbarger and Young, 1999). Gonads probably originate by preformation from germinal stem cells which, during ontogenetic development, appear to proliferate and migrate to form twinned germinal stem-cell clumps in the outer mantle epithelium, around the dorsal region between the mantle and gill. In adults, gonads can extend along the mantle epithelium in larger specimens (S.R. Laming, histological observations in Idas modiolaeformis, Laming, 2014). Gametogenesis takes place in follicular acini arranged in a matrix of connective tissue replete with vesicular and adipogranular cells (Eckelbarger and Young, 1999). Thus the mantle tissue has two physiological roles: the accumulation of pleomorphic somatic reserves and the development of the gonad, which in non-chemosymbiotic shallow-water mytilids, occurs at the expense of these reserves (Gabbott and Peek, 1991). Male sertoli cells and female follicle cells may deliver nutrients to the developing gametes, as in Mytilus edulis. Within a tissue section, it is possible to observe all stages of gametogenesis at once. Tyler et al. (2007) suggested a 5-stage grading system for the maturity status of gonads in deep-sea bathymodiolins. In male gonads, the acinus wall is lined with germinal stem cells that differentiate centripetally toward the central lumen (and along the acinus) by a series of divisions to form spermatogonia, spermatocytes, spermatids and ultimately, tailed spermatozoa. Mature spermatozoan head lengths for example measure between 3 and 4.4 μm in I. modiolaeformis (Gaudron et al., 2012). In females, germinal stem cells line the acini walls to which oogonia, pre-vitellogenic and vitellogenic (pedunculated) oocytes are also attached (Tyler et al., 2007; Gaudron et al., 2012). Fully grown oocytes in Bathymodiolinae are among the smallest for deep-sea bivalves (Le Pennec and Beninger, 2000). Maximum diameter of fully-grown oocytes measured by classical histology display highly conservative ranges from 50–80 μm (Table 1, Figure 1). Gametes released spontaneously by aquarium-held I. modiolaeformis and “Idas” simpsoni corroborate these estimates with a mean oocyte diameter of 59 ± 2.3 μm (S.R. Laming, pers. observation, from pooled oocytes of both species, Figure 2 and Laming, 2014).
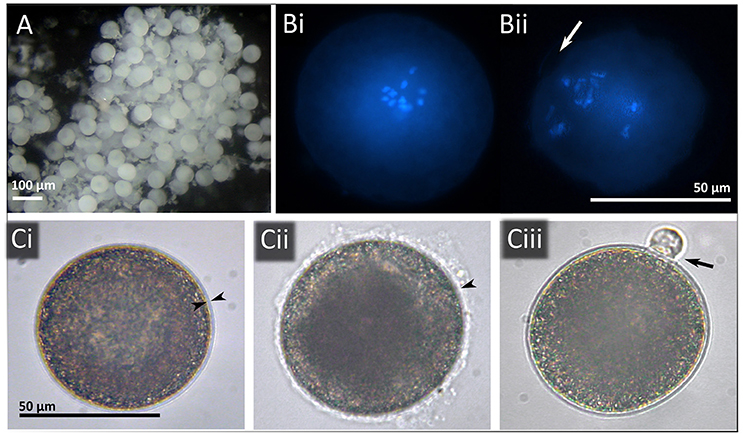
Figure 2. (A) Oocytes realized by an “Idas” simpsoni female. (B) Hoechst Staining identifying (i) released, but unfertilised, oocyte in Metaphase I; (ii) 4-cell division stage. Arrow identifies detachment of membrane/possible polar body. (C) Phase contrast microscope images of fertilized oocytes, (i) arrowheads delimit fertilization membrane; (ii) with jelly coat and; (iii) following formation of polar body.
Limited data are available on fecundity, because of a lack of observed spawning events and the difficulty in replicating suitable nutritional and physiological conditions in aquaria. However, based on the tendency for bathymodiolins to produce small oocytes, high fecundities have been inferred (Tyler and Young, 1999), though they are not yet quantified in published literature. In I. washingtonius, fecundity is described as being high (Tyler et al., 2009), however, no actual value per individual is provided. In I. modiolaeformis and “I.” simpsoni, estimates of between 380–410 oocytes per individual have been recorded (SR. Laming, data reported in Laming, 2014). A single specimen of the asymbiotic species I. argenteus (shell length, SL, 5.26 mm) recovered from a wood-fall experiment, had around 3,000 oocytes at various stages of development, suggesting high reproductive output for a relatively small organism (Dean, 1993). Small oocyte diameters certainly explain how species with limited body sizes can be fecund and spawn repeatedly. This combination of high overall output but low energy investment per single oocyte is not a general feature of all deep-sea bivalves. In other families including the Vesicomyidae and Solemyidae, oocytes are several times larger. Vesicomyidae, for example, produce oocytes with diameters generally around 150–200 μm, up to 382 μm in the large Calyptogena magnifica and down to 97.4 μm in the remarkably small (adult SL 2–6 mm) Isorropodon bigoti (Le Pennec and Beninger, 2000; Szafranski et al., 2014). Oocytes in Solemyidae reach up to 660 μm in Acharax alinae (Beninger and Le Pennec, 1997).
Sex determination in deep-sea bathymodiolins is complex and at times, poorly resolved. Many larger bathymodiolins appear to be gonochoric, including two sets of phylogenetically distant sister species: B. azoricus and B. puteoserpentis from hydrothermal vent sites along the Mid-Atlantic Ridge (MAR) and “Bathymodiolus” mauritanicus and “B.” childressi from cold seeps in the West Nigerian margin and Gulf of Mexico respectively (Eckelbarger and Young, 1999; Tyler et al., 2007; Arellano and Young, 2009; Duperron et al., 2013), However, Le Pennec and Beninger (1997) identified immature oocytes in the periphery of male acini in B. elongatus suggesting protandric hermaphrodism, and Comtet et al. (1999) suggested that B. azoricus might also exhibit hermaphroditism. Specimens of B. thermophilus found on hydrothermal vents along the East Pacific Rise (EPR, at 11–13°N) were initially reported as protandric as part of the species description (Kenk and Wilson, 1985). The current view however, is that this species is gonochoric, based on specimens collected from the Galapagos Rift and 13°N (Le Pennec and Beninger, 2000). More recently, rare examples of protogynous hermaphroditism have been described for the predominantly gonochoric species B. septemdierum (Rossi and Tunnicliffe, 2017). In the smaller-sized chemosymbiotic bathymodiolins, sex switching is pervasive, supported by compelling evidence for protandric hermaphroditism identified in all species examined to date (Table 1, Figure 1 stage a): seep and wood-associated I. modiolaeformis; bone-colonizing I. washingtonius; wood-colonizing, asymbiotic (larviphagous) I. argenteus and; bone-colonizing Adipicola pacifica (Tyler et al., 2009; Kinoshita et al., 2010; Ockelmann and Dinesen, 2011; Gaudron et al., 2012). Limited data indicate that despite being only distantly related to the genus Idas, “I.” simpsoni found colonizing seeps, sunken wood and bone remains may undergo similar changes. Functional males are typically much smaller than females in these mussels. Size at first maturity in males—the size at which the production of functional male gametes is first recorded - ranges between SLs of <1.7 mm and <1.8 mm in I. washingtonius and in “I.” simpsoni respectively, and <2.3 mm in I. modiolaeformis, whereas functional females have SLs over 4.5 and 7 mm, respectively (Gaudron et al., 2012; Génio et al., 2014; Laming et al., 2015a; Table 1). Due to the ephemeral nature of the organic-fall habitats from which these small protandric mytilids are often collected, larger specimens are relatively rare in sampled populations. Consequently, sex ratios are often heavily biased toward males. Tyler et al. (2009) identified only 10% females in their study of I. washingtonius. The story may be even more complex, with the identification of functional males of I. modiolaeformis displaying female acini either empty, or containing lysed oocytes (Gaudron et al., 2012). These observations support the hypothesis of a size-dependent sex change or iterative sex switching during growth. A specimen is either male or female at given point in time, where putatively functional hermaphrodites are very rarely reported (e.g., 16 out of 117 specimens in Tyler et al., 2009). When they are, it is usually with oocytes notably smaller than those of functional females, suggesting they could be undergoing a sexual transition. Sex determination could be influenced by epigenetic factors, as suggested for other wood-colonizing bivalves (e.g., Gaudron et al., 2016), while larger females likely benefit from higher fecundity.
In shallow-water mytilids, reproductive activity is constrained by environmental seasonality. Following relatively low productivity during winter, changes in the surrounding environment that might forecast an increase in food availability are thought to be used as cues for the onset of gametogenesis. Deep-sea species were once considered to be isolated from the driving processes that dictate reproductive seasonality in surface waters, due to a perceived absence of environmental cues. Species were therefore presumed to reproduce continuously. However, frequent instances of evidence to the contrary suggest that this hypothesis is misguided. There is evidence to support seasonal reproduction in the MAR vent-species Bathymodiolus azoricus, based both on its skewed population structure and on analyses of gamete production in samples collected periodically from Menez Gwen (800 m) using acoustically released cages (Comtet and Desbruyères, 1998; Comtet et al., 1999; Dixon et al., 2006). These data point toward a reproductive cycle comprising a single, annual spawning event in January with reproductive development recommencing in July. It correlated with surface chlorophyll-a measures, a proxy for planktonic primary production. Astoundingly, this pattern persisted in aquarium-held mussels. A temporal sampling regime coupled with histological analyses also revealed gametogenesis in “B.” childressi to be seasonal in specimens collected at the Brine Pool, an active methane seep 650 m deep on the Louisiana slope. Gamete development was synchronous across females and males, with spawning continuing deep into February following its onset in October. By March, evidence of oocyte growth and spermatozoan proliferation suggested gametogenesis had recommenced (Tyler et al., 2007; Arellano and Young, 2009). In the bone-colonizing species I. washingtonius, gametogenesis also appears to be discontinuous. However gametogenesis in this species appears not to be synchronized across all individuals, such that only a putative spawning period from March to April could be identified (Tyler et al., 2009). Spawning patterns remain unresolved for most other species on account of a lack of intra-annual temporal sampling. There is evidence, however, to support discontinuous spermatogenesis in both B. puteoserpentis and B. elongatus from Snake Pit vent site on the Mid-Atlantic Ridge and from the North Fiji Basin respectively, and a readiness to spawn in November in both sexes of I. modiolaeformis collected from methane seeps in the eastern Mediterranean (Le Pennec and Beninger, 1997; Gaudron et al., 2012). There are also species in which semi-continuous spawning may predominate. To date these include B. thermophilus from the EPR (based on gametogenesis, Tyler and Young, 1999) and several smaller-sized Idas spp., inferred from observed settlement patterns (Dean, 1993; Ritt et al., 2012; Laming et al., 2014). When identified, seasonality in the reproductive cycle of deep-sea species was initially thought to be in response to pulses of phytodetritus (Tyler, 1988; Eckelbarger and Watling, 1995). Later, hypotheses suggested that gametogenesis and gamete release might be initiated by the detection of deep-sea environmental cues that can forecast increases in surface primary production (Tyler et al., 2007). It is not yet clear whether cyclical environmental factors play a critical role as reproductive cues but examples might include seasonal variations in temperature, or tidal rhythms. Although reproductive trends may be derived from both spawning patterns and histological observations of gamete production, these biological processes are distinct. Le Pennec and Beninger (2000) draw attention to this distinction, suggesting that gametogenesis is more likely to be discontinuous in species where seasonal variations in the amount of inter-acinal tissue are also observed. This is because the presence of mature gametes in reproductive tissues does not necessarily indicate that spawning is imminent, only that it is viable.
Fertilization and Early Development
Fertilization experiments are particularly hard to perform on deep-sea species, often—though not always—necessitating pressure-maintaining cells for development to occur (Pradillon et al., 2001; but see Arellano and Young, 2009). In mytilids generally, the most effective methods for induction are shock treatments (mechanical, thermal or saline, Mestre et al., 2009), but these don't always prove effective in deep-sea species. In “Bathymodiolus” childressi, spawning was induced instead by injecting serotonin (0.4–0.5 ml at 2 mmol l−1) into the anterior adductor muscle (Arellano and Young, 2009). By contrast, the injection of serotonin into the anterior adductor muscle proved ineffective in “Idas” simpsoni and I. modiolaeformis, for which shock treatments were successful (S.R. Laming, unpublished data). Hoechst staining revealed a subsample of the “I.” simpsoni oocytes to be in Metaphase I of meiosis, and thus mature (Figure 2).
In the shallow-water species M. edulis and Modiolus modiolus, the ciliated swimming embryo is attained within the first 4–6 h depending on temperature (Bayne, 1965; Mestre et al., 2009), placing the second cleavage and thus 4-cell stage within the first 2 h after fertilization. Early rates of embryonic development in “B.” childressi are lower, where the second cleavage typically takes place between 7 and 15 h after fertilization (though at 7–8°C, Arellano and Young, 2009, Figure 1 stages c-e). The 64-cell (blastula) stage is reached after about 40 h, vs. 10 h in M. trossulus from the same study, followed by a brief trochophore stage (about 7 days at 7–8°C), and the first “D”-shell veligers at 8 days. Recent fertilization assays in Idas spp. revealed the 4-cells stage to be reached after 11 h at 14°C (Laming, 2014), but embryonic development beyond the 4-cell stage did not occur, as the embryos died following these observations.
Facts and Speculations About Larval Life, Consequences for Dispersal
Planktotrophy was long thought to be the prevalent larval mode of marine invertebrates, particularly in the tropics (Thorson, 1950; Pechenik, 1999), but also in deep-sea environments (Young, 1994; Pechenik, 1999; Tyler and Young, 2003). This larval mode requires minimal energy investment, as the oocytes hatch quickly into heterotrophic, planktonic larvae with a minimal yolk reserve. Large numbers of oocytes may be produced per unit of energy expended, and the capacity to feed permits an extended period of larval transport, facilitating dispersal. The diameter of oocytes of Bathymodiolinae are considered too small to contain the energetic yolk reserve necessary for lecithotrophy, thus larval development is likely to be planktotrophic regardless of adult size (Tyler and Young, 2003). Coastal shallow-water mytilids are broadcast spawners, releasing unfertilised gametes into the water column directly. In M. edulis, developing embryos hatch at approximately 70–110 μm, upon the formation of the embryonic prodissoconch I shell (PI) (Lutz et al., 1980; ~95 μm, Bayne, 1965, 1976). Larvae are planktotrophic and settle at prodissoconch II (PII) sizes of 260–320 μm after up to 4 weeks in the water column. Bathymodiolins display similar PI sizes (74–137 μm), but PII shell dimensions are much larger (379–580 μm, Table 1, Figure 1 stages f-h). This fact was first recognized in the pacific hydrothermal vent bathymodiolin Bathymodiolus thermophilus (Lutz et al., 1980). Since PI and PII dimensions are indicative of hatching and settling sizes respectively, these measures and the relative differences between them were used as a proxy for larval mode and growth during pelagic larval life. The sizeable differences in dimensions between in PI and PII in many bathymodioline species provide evidence for extended larval dispersal phases relative to those of shallow-water species. Basic estimates for pelagic larval duration (PLDs) of 9–16.5 months have been derived for “B.” childressi (Arellano and Young, 2009; Arellano et al., 2014). Species found on organic falls display comparable PI and PII sizes (Table 1), despite markedly smaller recorded adult shell lengths. A propensity for long-lived planktotrophic larvae may thus be a universal ancestral trait based on these data, as evidenced by fossils of the Eocene Vulcanidas goederti and Oligocene Bathymodiolus satsopensis for which PII measured 480 and 430 μm, respectively (Kiel and Amano, 2013). This sets the Bathymodiolinae apart from other chemosymbiotic bivalves, in which lecithotrophic development seems to predominate based on larger oocyte diameters, much larger PI SLs and consequently, lower PI:PII ratios [e.g., Solemyidae, Nucinellidae, most Thyasiridae and Lucinidae, and Vesicomyidae, (Gustafson and Reid, 1988; Taylor and Glover, 2000; Von Cosel and Salas, 2001; Krylova and Sahling, 2010)]. Thus planktotrophy and lecithotrophy have both proven to be successful modes of larval development in deep-sea chemosymbiotic bivalves.
Studies assessing intraspecific genetic variability across geographically discrete populations have provided indirect evidence of far-reaching dispersal, based on a lack of spatial structuring in some species (Craddock et al., 1995; Won et al., 2003; Faure et al., 2009, 2015; Vrijenhoek, 2010), even in species that occupy different types of reducing habitat within a given geographic area (e.g., “B.”japonicus and “B.” platifrons, Kyuno et al., 2009; I. modiolaeformis and “I.” simpsoni, Laming et al., 2015b). Historic far-reaching larval dispersal may have been instrumental in the formation of a number of bathymodiolin species complexes (I. macdonaldi-modiolaeformis, Duperron et al., 2013; Lorion et al., 2013; e.g., “B.” childressi-clade, Thubaut et al., 2013b; B. septemdierum-brevior-elongatus-marisindicus complex, Breusing et al., 2015). However, very few studies have actually investigated larval dispersal per se (e.g., McVeigh et al., 2017). This has proven difficult because the vertical distribution of larvae, which determines how they will disperse, is mostly unknown. Larvae of “B.” childressi, for example, were found to occur either several hundred meters above the seafloor at much shallower depths in the water column, or within deep-sea bottom currents where bathymodiolin adults live (Arellano and Young, 2011). These data were used to inform larval dispersal models. By employing validated ocean-current models for the Gulf of Mexico, authors estimated median dispersal distances of around 500 km for larvae originating from the Louisiana Slope, assuming an estimated PLD of 390 days (~13 months) (Young et al., 2012). Simulated larvae were generally retained within the Gulf of Mexico. However, a small proportion was able to reach suitable sites outside of this region, including the Laurentian Fan and Blake Ridge. This demonstrates the potential means by which longer larval life could translate into longer dispersal distances, but it also reaffirms the importance of transport conditions. Generally speaking, the link between PLD and larval dispersal decouples with increasing PLDs (Shanks, 2009).
Larval Settlement and Recruitment
In shallow-water mytilids, the pediveliger larva can swim using its velum or crawl using its foot, subsequent to initial settlement. It must select and colonize a suitable habitat and metamorphose toward a benthic mode of life. Bathymodioline pediveligers face similar challenges, presumably aided by the array of sensory and nervous anatomy at their disposal (Laming et al., 2015a). These larvae are the transitional stage between the pelagos and benthos and are thought to respond strongly to local settlement cues, descending rapidly (Figure 1, stages h-i). In shallow-water mytilids, interactions with the physical environment appear to be the most influential, including current flow and substratum characteristics (Pernet et al., 2003). Algal waterborne compounds or elevated oxygen levels can induce positive larval responses (Dobretsov and Wahl, 2001; Alfaro, 2005). Neurochemical signaling is probably also involved since the use of neuroactive compounds can induce or block pediveliger settlement behavior (Alfaro et al., 2011; Yang et al., 2011). Some mytilids, particularly biofouling species, have been shown to respond positively to sound (or vibrations) at a frequency typical of a large ocean-going vessel (Wilkens et al., 2012).
For Bathymodiolinae, crawling has been recorded in juvenile and adult specimens in Idas argenteus, I. modiolaeformis, and “I.” simpsoni, (Rodrigues et al., 2015; Supplementary Material 1). However, the factors that trigger larval settlement are not yet known. Considering the vast array of signature chemicals available to bathymodioline larvae approaching reducing habitats, chemical cueing may be important. Bathymodioline mussels are regularly found occurring on experimental colonization devices, in particular those that consist of organic material (bones, plants). Smaller-sized species have been sampled on a regular basis from various device types, locations and depths, often at rather high densities. Plantigrades of I. modiolaeformis were recorded on wooden substrates following only 2 weeks deployment in the eastern Mediterranean (Gaudron et al., 2010). Longer 18-month cow-bone deployments yielded high densities of “I.” simpsoni (up to 6.27 individuals cm−2) on in the Setúbal Canyon (Génio et al., 2014). Many bathymodioline species form dense beds as adults, yet it is not yet clear whether this is a direct result of gregarious larval settlement behavior or post-settlement population dynamics. Multiple size cohorts have been identified in large Bathymodiolus spp. from deeper sites and in “B.” childressi at realtively shallow sites, evidenced by multi-modal shell-length distributions in each case (Nix et al., 1995; Comtet and Desbruyères, 1998; Tyler et al., 2007; Arellano and Young, 2009). This suggests a degree of periodicity in recruitment with massive recruitment episodes, possibly linked with the aforementioned periodicity in reproduction. Such larval input incurs high pre-settlement rates of mortality, as illustrated by the high densities of dead plantigrade shells observed around aggregates of “I.” simpsoni in the Marmara Sea (Ritt et al., 2012).
Post Larval Growth to Maturity, and the Onset of Symbiosis
In adult Bathymodiolinae, energy derived from established symbioses with bacterial partners probably provides many fitness advantages, not least, in augmenting reproductive and somatic growth. Such advantages rely on establishing a functioning symbiosis. A few studies have examined post-larval and juvenile development in bathymodiolins, with particular focus on the differentiation of reproductive tissues, gill-filament proliferation and the first appearance of symbionts in host tissues (timing and proliferation). As with many of the smaller-sized bathymodioline species in which reproductive development has been examined, I. modiolaeformis displays protandric hermaphroditism (section Bathymodiolinae Produce Small Oocytes and Display Complex Sex Determination and Seasonality in Their Reproductive Cycle and Table 1). By applying a basic time-averaged growth-rate estimate to the net increase in SL attained prior to reaching first maturity, age at first maturity is estimated to be <4 months following settlement in specimens from wood fragments (Gaudron et al., 2010; Laming et al., 2014). Size at first maturity as a male may occur at even smaller SLs, in bone-associated “I.” simpsoni from the Setúbal submarine canyon (Génio et al., 2014); applying a time-averaged growth-rate estimate of 0.025–0.029 mm d−1, this suggests that maturity in “I.” simpsoni is reached at an age of <2 months. In a separate 6-month long wood-colonization experiment carried out in the Lacaze-Duthiers Canyon (France), all “I.” simpsoni were shown to be already mature, suggesting that on wood-falls maturation is also fast (Laming, 2014). Size and age at first maturity as a female in this species remains poorly defined, since the smallest “I.” simpsoni female found in a separate 23-month wood deployment in the Lacaze-Duthiers Canyon was already quite large (SL: 18.1 mm). Collectively these rates of maturation are well within the longevity of larger wood and bone accumulations, making these mussels well adapted to their temporally finite habitats (Smith and Baco, 2003; Bernardino et al., 2010; Cunha et al., 2013). Although only rough estimates, they suggest that small chemosymbiotic bathymodiolins reach sexual maturity within an impressively short passage of time, not unlike the xylophagid wood-boring bivalves that initially colonize deep-sea wood habitats (Gaudron et al., 2016).
Among the most remarkable features of the Bathymodiolinae clade is the occurrence of bacterial symbionts associated with the paired filibranch gills. In all species but I. argenteus, bacteria occur on or within gill epithelial cells located in the non-ciliated abfrontal and lateral regions of the gill filaments (reviewed in Duperron, 2010). The process of bacterial transmission has been described recently. First of all, in contrast to vesicomyid clams for which trans-ovarial maternal transmission of symbionts has been demonstrated (Endow and Ohta, 1990; Cary, 1994; Szafranski et al., 2014), bacteria have never been found in the male or female gonads or gametes of the Bathymodiolinae investigated to date (Le Pennec and Beninger, 1997; Eckelbarger and Young, 1999; Gaudron et al., 2012; Laming et al., 2014). The mussels have thus been thought to acquire their symbionts horizontally. Only a limited number of studies examining six bathymodioline species in total have documented the presence of bacterial symbionts during the very early life stages. The first of these identified putative methane-oxidizing symbionts in “Bathymodiolus” childressi juveniles at SL ≥4mm from the Brine Pool site in the Gulf of Mexico, and both methane- and sulfur -oxidizing symbionts in post-larvae of B. azoricus and B. heckaerae collected from Lucky Strike vent and Blake Ridge methane-hydrate seep respectively (Streams et al., 1997; Salerno et al., 2005). Symbiont-specific FISH analyses have since confirmed the presence of both sulfur- and methane-oxidizing symbionts in B. azoricus and B. puteoserpentis juveniles (SLs 4–21 mm, Wentrup et al., 2013). However, as no aposymbiotic specimens have ever been found, the exact timing of symbiont acquisition remains unresolved (Figure 1 stage j).
Symbiont proliferation in the context of gill development during host ontogeny has only been investigated quite recently. In both I. modiolaeformis and “I.” simpsoni, observed patterns of gill development echo those identified in the shallow-water mytilid M. edulis (Cannuel et al., 2009). The descending lamellae of the inner demibranchs develop first, and grow in length and filament number before the initial development of the ascending lamellae of the inner demibranchs (Laming et al., 2014, 2015a, Figure 1 stage k). In these species and in B. azoricus and B. puteoserpentis, undifferentiated epithelial cells found in the posterior “budding” zone of each gill lamella gives rise to new gill filaments as lamellae increase in size (Wentrup et al., 2013). In I. modiolaeformis, based on fluorescence microscopy and classical histology, bacteria were only present in host specimens of SL ≥0.59 mm, for which dissoconch shell growth was already evident (representing a 50% increase in SL compared to the PII). In a parallel study on “I.” simpsoni, symbionts were detected after only an 8% increase in SL compared with PII, at 0.43 mm (Laming et al., 2015a). These observations suggest that symbiotic bacteria are absent in dispersing larvae of these species, and are then acquired shortly after settlement. Of course, the pre-acquisition of a low seeding population of bacteria, below method-specific detection limits, cannot be ruled out. The manner in which these symbioses manifest themselves appears to be similar for all six host species (i.e., B. azoricus, “B. heckerae, B. puteoserpentis, B.” childressi, I. modiolaeformis, and “I.” simpsoni). Bacterial symbionts first associate with some or all of those epithelial tissues in the pallial cavity that are exposed to inhalant flow, including retractor sheaths and visceral, mantle, foot, and non-ciliated gill epithelia (Streams et al., 1997; Salerno et al., 2005; Wentrup et al., 2013; Laming et al., 2014, 2015a). Then, at some point, following an increase in body size, the association becomes increasingly specific until ultimately, symbionts are only detectable in the non-ciliated gill epithelia (Figure 1). According to recent FISH-based evidence for B. azoricus and B. puteoserpentis, after newly formed aposymbiotic gill filaments emerge from the posterior “budding” zone of the gill lamellae, they acquire their symbionts from neighboring, longer-established filaments, a process that appears to continue throughout the lifetime of the host (Wentrup et al., 2015).
The question of where bacteria originate from prior to transmission still remains unresolved. In a study examining the symbiont composition of I. modiolaeformis and “I.” simpsoni using partial 16S rRNA-encoding gene sequences as a means of symbiont identification, it was shown that specimens of I. modiolaeformis from a given sampling site harbor similar symbionts, but that different symbionts can occur in specimens from different sampling sites (Laming et al., 2015b). This supports the hypothesis that in this species at least, acquisition occurs via locally available bacterial lineages. Bacterial 16S rRNA-encoding sequences almost identical to bathymodioline symbionts have been identified from several environmental samples (see Crépeau et al., 2011; Petersen et al., 2012; Szafranski et al., 2015a). However, numerous free-living bacteria known to be incapable of establishing symbioses display sequences highly similar to those of symbionts, so further evidence is needed to demonstrate whether free-living forms of these symbionts actually occur. Recent genomic studies provide evidence of genome reduction in bathymodiolin-associated sulfur-oxidizing symbionts, which casts further doubt as to whether such symbionts could live outside their hosts (Ikuta et al., 2016). Whether horizontal transmission of symbionts occurs through free-living forms of bacteria capable of living outside their hosts, or through lateral acquisition from symbionts liberated by proximal hosts that are capable of brief transitions through the environment thus remains an open question.
Horizontal acquisition coupled with a life-long competency for acquiring symbionts probably explains why chemosymbiotic Bathymodiolinae can sometimes harbor a diverse range of symbiont lineages composed of either multiple bacterial groups with distinct metabolic capabilities (such as sulfur- and methane-oxidizers, methylotrophs, Colwellia, and Bacteroidetes), or closely-related yet-distinct lineages of sulfur-oxidizers, such as is observed in B. heckerae and I. modiolaeformis (Duperron et al., 2007, 2008, 2009). With this level of flexibility, a single host species can harbor different symbiont types as a function of habitat type and location (Laming et al., 2015b).
Nutritional Shifts Along the Life Cycle
Adult bathymodiolins of most species rely on their bacterial partners for much of their nutrition, with some species retaining rudimentary digestive systems only. That said, growing evidence points to a retained capacity to filter feed (Page et al., 1991). Studies in aquaria have demonstrated the assimilation of carbon from algae, radio-labeled water-borne bacteria, and naturally-occurring plankton (Pile and Young, 1999). The retention of a fully-formed digestive system appears to be the norm in smaller-sized bathymodiolins from organic falls (Gustafson et al., 1998; Thubaut et al., 2013a; Laming et al., 2014, 2015a). In I. modiolaeformis and “I.” simpsoni, live examinations under a dissecting microscope reveal the mediated convection of debris in the vicinity of the gills in a way similar to that of shallow-water mussels (Supplementary Material 2). Given the earliest, post-settlement developmental stages of these species possess components of a functioning digestive system and are either aposymbiotic or display low abundance of symbiotic bacteria, the diets of juvenile bathymodiolins are likely to undergo a transition from heterotrophy-to-mixotrophy-to-chemosymbiosis with increasing size. This is supported by a model-based study examining B. azoricus from the relatively shallow hydrothermal vent site Menez Gwen (800 m), in which a shift toward greater reliance on symbionts during the growth of the host was hypothesized (Martins et al., 2008). Similar transitions are thought to take place in other chemosymbiotic molluscs (e.g., Gigantopelta spp., Chen et al., 2017). Despite these data and hypotheses, the current challenge is to accurately measure the relative importance of different nutritional resources in nature. Stable isotopes of carbon and nitrogen have often been used to trace nutritional uptake in bathymodioline mussels. Although this approach is effective when symbiotic nutrition dominates and isotopic signatures are unambiguous, as can be the case in vent and seep species (Fisher, 1995), it is far more problematic where large inputs of terrigenous organic matter occur with less clear-cut signatures.
Adapting to Change: The Cumulative Impacts of Inherent and Anthropogenic Environmental Stressors
The Bathymodiolinae thrive at cold seeps, hydrothermal vents and in association with organic falls, sometimes reaching extremely high densities. These high biomasses underline their importance for ecosystem productivity and functioning, however spatial isolation and the ephemeral nature of their habitat places bathymodiolins at greater risk from anthropogenic disturbance. Although most reducing habitats colonized by Bathymodiolinae appear remote from the surface, the deep sea is now known to be subject to similar anthropogenic disturbances to those documented in shallow waters (Ramirez-Llodra et al., 2010). Human activities that pose an immediate or future threat to deep-sea reducing environments include climate-change induced environmental shifts (e.g., global rises in ocean temperatures, deoxygenation, and acidification), resource exploitation (fossil-fuel extraction, deep-sea mining and fishing/whaling) and impacts from the accumulation of marine pollutants and debris in the environment and food webs. The severity of these impacts will be dependent upon both the reducing habitat in question and the developmental stage being impacted. Beyond the visible impacts that human activities can have on adult bathymodiolins and other fauna living within established benthic communities, the consequences for the remainder of their lifecycle are far more difficult to evaluate. Although the physico-chemical stimuli that trigger larval settlement in Bathymodiolinae remain speculative, early developmental phases may be acutely sensitive to additional anthropogenic sources of physiological stress, including the release of toxic compounds, increasing turbidity, or potentially misleading physical and chemical cues (Hauton et al., 2017).
The role climate change plays in rising sea levels has been discussed at length in the public domain as the implications for society are self-evident. However, perhaps of greater concern and more relevant to the deep sea, is the potential for avenues for oceanic thermohaline circulation to undergo large scale alterations as oceans warm, glacial run-off increases and annual sea-ice formation continues to diminish. These changes may influence net larval transport both in terms of distance and direction, with consequences for bathymodiolin population connectivity. Any negative impacts on dispersal will be exacerbated by shortened larval duration, as a result of higher temperature-dependent developmental rates. For larvae that migrate to surface waters during development, rising surface temperatures may also influence surface primary production, affecting larval food supply. Declines in dissolved oxygen are intrinsic to global warming. As a consequence, oxygen minima (OM) are expanding globally and if trends continue unabated, shifts in impacted species distributions are expected (Stramma et al., 2010). It is, however, unclear to what extent OM will impact on deep-sea communities in other reducing environments. Although oceans are expected to become more acidic in the future, as atmospheric CO2 saturates the ocean's buffering capacity, in naturally acidic reducing environments such as those at hydrothermal vents, natural varibility in the pH and chemical composition of seawater is several orders of magnitude greater than those predicted from ocean acidification (e.g., Tunnicliffe et al., 2009). However, molluscan larvae are known to be sensitive to ocean acidification (Kroeker et al., 2013), so bathymodiolins may see increased mortality and decalcification, and decreased rates of growth and development during this period of their lifecycle.
In both oil and mineral extraction, habitat destruction and the resuspension of both sediment and potentially toxic chemical compounds during operations that require drilling or cutting (e.g., copper and other metals or lubricating organics, see Hauton et al., 2017) may prove highly deleterious for local and regional benthic communities. Resuspended sediment loading may smother many sessile organisms including Bathymodolinae, in the immediate vicinity of mining activity. If waste water produced as a byproduct of de-watering the mineral slurry is to be dumped back into the sea, this could compound the impact of sediment loading (Miller et al., 2018).
The incidence of dead whales reaching the seafloor is thought to have markedly diminished over the last century as a consequence of whaling and fishing pressure (Smith and Baco, 2003). This translates to a considerable loss of habitat for fauna that colonize their decomposing, sulphidic skeletal remains.
As many bathymodioline species have very limited geographic ranges based on current records, this makes them highly susceptible to extinction events, for example when a vent site's activity is disrupted or redirected by mining operations. However, some solace may be taken from indications that reducing habitats could be less fragmented than previously thought; recent modeling approaches allude to the predicted existence of unreported vent sites, acting as stepping stones between known study sites on the Mid-Atlantic Ridge (Breusing et al., 2016). The Bathymodiolinae may be at an advantage, as lifecycle characteristics such as early symbiont acquisition, rapid maturation rates, high fecundity and long-lived, potentially far-reaching planktotrophic larvae probably help coping with environmental disturbance. In addition to retaining some mobility as adults, flexible chemosymbioses in species harboring multiple symbiont types also offer a means to cope with environmental variations, such as in water chemistry (Laming et al., 2015b; Szafranski et al., 2015b). Conversely however, those bathymodiolin mussels already living at the physiological limits of their existence in environments with multiple, inherent sources of stress could be highly susceptible to further changes in their environment.
Open Questions and Hypotheses, and How to Explore Them
Despite the headway that has been made regarding the lifecycle ecology of bathymodioline mussels and their associated bacteria, several themes remain poorly addressed. Documenting lifecycle ecology requires inter-annual time-series sampling at sites where adults are well established (e.g., by employing acoustic-release cages, Dixon et al., 2006). Deep-sea observatories such as Neptune and MomarSat exist already but their sampling potential remains limited, being dependent on imaging, and the monitoring of physico-chemical characteristics only (Barnes et al., 2007; Sarrazin et al., 2014). Where exactly bathymodiolins develop as larvae in the water column, the duration of dispersal and to what degree larvae are retained near their source, all remain a mystery despite some indication sometimes exist (Arellano et al., 2014). Sampling and identifying larvae near their benthic habitats has proven difficult and nigh-on impossible when sampling farther from larval source and sink sites. Both sediment traps and larval pumps have been used with varying degrees of success, however the identification of larvae to the species level often requires lengthy molecular-based approaches (Comtet et al., 2000; Pradillon et al., 2007; Beaulieu et al., 2009). Many of the assumptions made about larval dispersal and settlement behavior are derived from connectivity studies and data from distantly related analogous shallow-water species. To better understand these processes further empirical work is necessary, with particular emphasis on ex-situ experimental designs using reared larvae.
In tandem with advancing our understanding of bathymodioline larval biology, there is also a need to better describe distribution and connectivity patterns mediated by larval dispersal in the deep-sea. This is an emerging field of research, with the biogeography of deep-sea reducing habitats evolving as new communities are described. The level of connectivity across organic falls remains difficult to assess due their less predictable distribution and the absence of regional environmental anomalies as a means to locate them easily (e.g., such as temperature and water chemistry anomalies for vents). Clearly, as more sites are discovered and explored and the resolution of population genetics increases, the resolution and thus reliability of the global biogeography for deep-sea species will improve. The use of reliable modeling approaches based on detailed knowledge of ocean currents already allows us to create reliable predictions for dispersal—and thus connectivity—in marine organisms, and should be further advanced alongside any new discoveries in larval biology, species distributions and genetics.
More work can still be done to improve our understanding of the onset of symbiosis from the point of view of the holobiont lifecycle, the underlying biochemical, and physiological mechanisms of cellular communication and the evolutionary, origins of the symbiosis. Lateral acquisition from other established specimens and from a pool of free-living bacteria are not mutually exclusive, and symbiont release as viable free-living bacteria has not been explored. This will ultimately require ex-situ experimental work coupled with (meta)genomics methods (see for example Ikuta et al., 2016).
Current international initiatives that have largely arisen in the wake of the Census of Marine Life (CoML, particularly the Biogeography of Deep-Water Chemosynthetic Ecosystems field project, ChEss) are focused on the expansion of our understanding of basic larval biology, including the supply of larvae to newly available substrate for colonization; ecosystem function and environmental characterisation; the biodiversity and distribution of deep-sea species in these habitats; and the impact of deep-sea resource exploitation. A better understanding of the whole life cycle of organisms, including Bathymodiolinae, will be critical in implementing management strategies for reducing-habitat networks with realistic protection policies, culminating in research-informed Marine Protected Areas (Van Dover et al., 2011). Deep-sea marine science is now at a critical juncture in its history. Deep-sea environments are subject to multiple levels of environmental impact, including deep-sea fisheries and imminent mineral-extraction exploration using huge underwater drilling apparatus. This comes at a time when we still have so much to learn and gain from describing these habitats more definitively (Van Dover, 2014). Because they are widespread at chemosynthesis-based ecosystems and important to their functioning, Bathymodiolinae are one of the most important target groups to study. Like their coastal relatives, they are resistant to various toxic compounds and behave as bio-accumulators. Consequently, the Bathymodiolinae may prove to be useful bio-indicators of both pollution and elevated levels of stress from anthropogenic disturbance at sites where they occur.
Author Contributions
SL, SG, and SD prepared the table. SL prepared the figures and supplementary material. All authors contributed substantially to the text, and approved it for publication.
Funding
This study received funding from CHEMECO ESF EURODEEP (EURODEEP/0001/2007), the Institut Universitaire de France (ACSYMB project), and SL was supported by a grant from the MARES Joint Doctorate (Erasmus Mundus FPA 2011–0016).
Conflict of Interest Statement
The authors declare that the research was conducted in the absence of any commercial or financial relationships that could be construed as a potential conflict of interest.
Acknowledgments
We thank Marina R. Cunha (University of Aveiro), whose welcome input informed aspects of the review. We thank Sorbonne Université, the Muséum National d'Histoire Naturelle and Ifremer for additional funding. We also thank the editor and reviewers for their comments, which helped improve the manuscript.
Supplementary Material
The Supplementary Material for this article can be found online at: https://www.frontiersin.org/articles/10.3389/fmars.2018.00282/full#supplementary-material
Supplementary Material 1. Movie displaying the crawling behavior of Idas argenteus. The pericardium can be seen beating through the posteriodorsal region of the shell. The foot extends and attaches to substratum via the ‘heel’ and the tip (in which there is a sensory pit) searches within its immediate proximity. ‘Crawling’ is achieved through the subsequent contraction of the foot.
Supplementary Material 2. As in shallow water mytilids, particle transport is coordinated by ciliary action in Idas simpsoni, where lateral cilia, latero-frontal cirri, and frontal cilia in the gill filaments can be identified. Debris in the seawater (including released oocytes) was first captured and transported ventrally between interfilamental lateral cilia, and then anteriorly along the ventral feeding groove toward the labial palps and mouth (beyond field of view). The first particles to arrive at the mouth are ingested directly. A mucuous string forms with increased input and subsequent particles become entrained in mucous-bound balls that rotate within the ciliated labial palps to be sorted.
References
Alfaro, A. C. (2005). Effect of water flow and oxygen concentration on early settlement of the New Zealand green-lipped mussel, Perna canaliculus. Aquaculture 246, 285–294. doi: 10.1016/j.aquaculture.2005.02.049
Alfaro, A. C., Young, T., and Ganesan, A. M. (2011). Regulatory effects of mussel (Aulacomya maoriana Iredale 1915) larval settlement by neuroactive compounds, amino acids and bacterial biofilms. Aquaculture 322–323, 158–68. doi: 10.1016/j.aquaculture.2011.08.038
Arellano, S. M., Van Gaest, A. L., Johnson, S. B., Vrijenhoek, R. C., and Young, C. M. (2014). Larvae from deep-sea methane seeps disperse in surface waters. Proc. R. Soc. Lond. B. Biol. Sci. 281:20133276. doi: 10.1098/rspb.2013.3276
Arellano, S. M., and Young, C. M. (2009). Spawning, development, and the duration of larval life in a deep-sea cold-seep mussel. Biol. Bull. 216, 149–162. doi: 10.1086/BBLv216n2p149
Arellano, S. M., and Young, C. M. (2011). Temperature and salinity tolerances of embryos and larvae of the deep-sea mytilid mussel “Bathymodiolus” childressi. Mar. Biol. 158, 2481–2493. doi: 10.1007/s00227-011-1749-9
Barnes, C. R., Best, M. M. R., Bornhold, B. D., Juniper, S. K., Pirenne, B., and Phibbs, P. (2007). “The “NEPTUNE Project - a cabled ocean observatory in the NE Pacific: overview, challenges and scientific objectives for the installation and operation of Stage I in Canadian waters,” in 2007 Symposium on Underwater Technology and Workshop on Scientific Use of Submarine Cables and Related Technologies. 2007, 308–313.
Bayne, B. L. (1965). Growth and the delay of metamorphosis of the larvae of Mytilus edulis (L.). Ophelia 2, 1–47. doi: 10.1080/00785326.1965.10409596
Bayne, B. L. (1976). Marine Mussels, their Ecology and Physiology. London: Cambridge University Press.
Beaulieu, S. E., Mullineaux, L. S., Adams, D. K., and Mills, S. W. (2009). Comparison of a sediment trap and plankton pump for time-series sampling of larvae near deep-sea hydrothermal vents. Limnol. Oceanogr. Methods 7, 235–248. doi: 10.4319/lom.2009.7.235
Beninger, P. G., and Le Pennec, M. (1997). Reproductive characteristics of a primitive bivalve from a deep-sea reducing environment: giant gametes and their significance in Acharax alinae (Cryptodonta: Solemyidae). Mar. Ecol. Prog. Ser. 157, 195–206. doi: 10.3354/meps157195
Berg, C. J. Jr. (1985). Reproductive strategies of mollusks from abyssal hydrothermal vent communities. Bull. Biol. Soc. Washington 6, 185–197.
Bernardino, A. F., Smith, C. R., Baco, A. R., Altamira, I., and Sumida, P. Y. G. (2010). Macrofaunal succession in sediments around kelp and wood falls in the deep NE Pacific and community overlap with other reducing habitats. Deep Sea Res. I 57, 708–723. doi: 10.1016/j.dsr.2010.03.004
Breusing, C., Biastoch, A., Drews, A., Metaxas, A., Jollivet, D., Vrijenhoak, R. C., et al. (2016). Biophysical and population genetic models predict the presence of “phantom” stepping stones connecting Mid-Atlantic Ridge vent ecosystems. Curr. Biol. 26, 2257–2267. doi: 10.1016/j.cub.2016.06.062
Breusing, C., Johnson, S. B., Tunnicliffe, V., and Vrijenhoek, R. C. (2015). Population structure and connectivity in Indo-Pacific deep-sea mussels of the Bathymodiolus septemdierum complex. Conserv. Genet. 16, 1415–1430. doi: 10.1007/s10592-015-0750-0
Cannuel, R., Beninger, P. G., McCombie, H., and Bordy, P. (2009). Gill development and its functional and evolutionary implications in the blue mussel Mytilus edulis (Bivalvia: Mytilidae). Biol. Bull. 217, 173–188. doi: 10.1086/BBLv217n2p173
Cary, S. C. (1994). Vertical transmission of a chemoautotrophic symbiont in the protobranch bivalve, Solemya reidi. Mol. Mar. Biol. Biotechnol. 3, 121–130.
Cavanaugh, C. M., McKiness, Z. P., Newton, I. L. G., and Stewart, F. J. (2005). “Marine chemosynthetic symbioses,” in The Prokaryotes: An Evolving Electronic Resource for the Microbiological Community, ed M. Dworkin et al. (New York, NY: Springer-Verlag) 475–507.
Chen, C., Uematsu, K., Linse, K., and Sigwart, J. (2017). By more ways than one: rapid convergence at hydrothermal vents shown by 3D anatomical reconstruction of Gigantopelta (Mollusca: Neomphalina). BMS Evol. Biol. 17:62. doi: 10.1186/s12862-017-0917-z
Colaço, A., Martins, I., Laranjo, M., Pires, L., Leal, C., Prieto, C., et al. (2006). Annual spawning of the hydrothermal vent mussel, Bathymodiolus azoricus, under controlled aquarium conditions, at atmospheric pressure. J. Exp. Mar. Biol. Ecol. 333, 166–171. doi: 10.1016/j.jembe.2005.12.005
Comtet, T., and Desbruyères, D. (1998). Population structure and recruitment in mytilid bivalves from the Lucky Strike and Menez Gwen hydrothermal vent fields (37°17'N and 37°50'N on the Mid-Atlantic Ridge). Mar. Ecol. Prog. Ser. 163, 165–177.
Comtet, T., Jollivet, D., Khripounoff, A., Segonzac, M., and Dixon, D. (2000). Molecular and morphological identification of settlement-stage vent mussel larvae, Bathymodiolus azoricus (Bivalvia Mytilidae), preserved in situ at active vent fields on the Mid-Atlantic Ridge. Limnol. Oceanogr. 45, 1655–1661. doi: 10.4319/lo.2000.45.7.1655
Comtet, T., Le Pennec, M., and Desbruyères, D. (1999). Evidence of a sexual pause in Bathymodiolus azoricus (Bivalvia: Mytilidae) from hydrothermal vents of the Mid-Atlantic Ridge. J. Mar. Biol. Assoc. U. K. 79, 1149–1150. doi: 10.1017/S0025315499001514
Craddock, C., Hoeh, W. R., Gustafson, R. G., Lutz, R. A., Hashimoto, R., and Vrijenhoek, R. C. (1995). Evolutionary relationships among deep-sea mytilids (Bivalvia : Mytilidae) from hydrothermal vents and cold water methane/sulfide seeps. Mar. Biol. 121, 477–485. doi: 10.1007/BF00349456
Crépeau, V., Cambon-Bonavita, M. A., Lesongeur, F., Randrialivelo, H., Sarradin, P. M., Sarrazin, J., et al. (2011). Diversity and function in microbial mats from the Lucky Strike hydrothermal vent field. FEMS Microbiol. Ecol. 76, 524–540. doi: 10.1111/j.1574-6941.2011.01070.x
Cunha, M. R., Matos, F. L., Génio, L., Moura, C. J., Ravara, A., and Rodrigues, C. F. (2013). Are organic falls bridging reduced environments in the deep sea? - Results from colonization experiments in the Gulf of Cádiz. PLoS ONE 8:e76688. doi: 10.1371/journal.pone.0076688
Dean, H. (1993). A population study of the bivalve Idas argenteus Jeffreys, 1876,(Bivalvia: Mytilidae) recovered from a submerged wood block in the deep North Atlantic Ocean. Malacologia 35, 21–41.
Dixon, D. R., Lowe, D. M., Miller, P. I., and Villemein, J. R. (2006). Evidence of seasonal reproduction in the Atlantic vent mussel Bathymodiolus azoricus, and an apparent link with the timing of photosynthetic primary production. J. Mar. Biol. Assoc. U. K. 86, 1363–1371. doi: 10.1017/S0025315406014391
Dobretsov, S., and Wahl, M. (2001). Recruitment preferences of blue mussel spat Mytilus edulis for different substrata and microhabitats in the White Sea (Russia). Hydrobiologia 445, 27–35. doi: 10.1023/A:1017502126707
Duperron, S. (2010). “The diversity of deep-sea mussels and their bacterial symbioses” in The Vent and Seep Biota, ed S. Biota Kiel (Basingstoke, UK: Springer) 137–167.
Duperron, S., Gaudron, S. M., Rodrigues, C. F., Cunha, M. R., Decker, C., and Olu, K. (2013). An overview of chemosynthetic symbioses in bivalves from the North Atlantic and Mediterranean Sea. Biogeosciences 10, 3241–3267. doi: 10.5194/bg-10-3241-2013
Duperron, S., Halary, S., Lorion, J., Sibuet, M., and Gaill, F. (2008). Unexpected co-occurence of 6 bacterial symbionts in the gill of the cold seep mussel Idas sp. (Bivalvia: Mytilidae). Environ. Microbiol. 10, 433–45. doi: 10.1111/j.1462-2920.2007.01465.x
Duperron, S., Lorion, J., Samadi, S., Gros, O., and Gaill, F. (2009). Symbioses between deep-sea mussels (Mytilidae: Bathymodiolinae) and chemosynthetic bacteria: diversity, function and evolution. C R Biol. 332, 298–310. doi: 10.1016/j.crvi.2008.08.003
Duperron, S., Sibuet, M., MacGregor, B. J., Kuypers, M. M. M., Fisher, C. R., and Dubilier, N. (2007). Diversity, relative abundance, and metabolic potential of bacterial endosymbionts in three Bathymodiolus mussels (Bivalvia: Mytilidae) from cold seeps in the Gulf of Mexico. Environ Microbiol. 9, 1423–1438. doi: 10.1111/j.1462-2920.2007.01259.x
Eckelbarger, K. J., and Watling, L. (1995). Role of phylogenetic constraints in determining reproductive patterns in deep-sea invertebrates. Invertebr. Biol. 114, 256–269. doi: 10.2307/3226880
Eckelbarger, K. J., and Young, C. M. (1999). Ultrastructure of gametogenesis in a chemosynthetic mytilid bivalve (Bathymodiolus childressi) from a bathyal, methane seep environment (northern Gulf of Mexico). Mar. Biol. 135, 635–646. doi: 10.1007/s002270050664
Endow, K., and Ohta, S. (1990). Occurence of bacteria in the primary oocytes of vesicomyid clam Calyptogena soyoae. Mar. Ecol. Prog. Ser. 64, 309–317. doi: 10.3354/meps064309
Faure, B., Jollivet, D., Tanguy, A., Bonhomme, F., and Bierne, N. (2009). Speciation in the deep sea: multi-locus analysis of divergence and gene flow between two hybridizing species of hydrothermal vent mussels. PLoS ONE 4:e6485. doi: 10.1371/journal.pone.0006485
Faure, B., Schaeffer, S. W., and Fisher, C. R. (2015). Species distribution and population connectivity of deep-sea mussels at hydrocarbon seeps in the Gulf of Mexico. Plos ONE 10:e0118460. doi: 10.1371/journal.pone.0118460
Fisher, C. R. (1995). Toward an Appreciation of Hydrothermal-vent Animals: Their Environment, Physiological Ecology, and Tissue Stable Isotope Values. Hoboken, NJ: American Geophysical Union.
Gabbott, P. A., and Peek, K. (1991). Cellular biochemistry of the mantle tissue of the mussel Mytilus edulis L. Aquaculture 94, 165–176. doi: 10.1016/0044-8486(91)90116-O
Gaudron, S. M., Demoyencourt, E., and Duperron, S. (2012). Reproductive traits of the cold seep mussel Idas modiolaeformis: from gametogenesis to larval dispersal. Biol. Bull. 222, 6–16. doi: 10.1086/BBLv222n1p6
Gaudron, S. M., Haga, T., Wang, H., Lamong, S. R., and Duperron, S. (2016). Plasticity in reproduction and nutrition in wood-boring bivalves Xylophaga atlantica from the Mid-Atlantic Ridge. Mar. Biol. 163:213. doi: 10.1007/s00227-016-2988-6
Gaudron, S. M., Pradillon, F., Pailleret, M., Duperron, S., Le Bris, N., and Gaill, F. (2010). Colonization of organic substrates deployed in deep-sea reducing habitats by symbiotic species and associated fauna. Mar. Environ. Res. 70, 1–12. doi: 10.1016/j.marenvres.2010.02.002
Génio, L., Rodrigues, C. F., Guedes, I. F., Almeida, H., Duperron, S., and Hilario, A. (2014). Mammal carcasses attract a swarm of mussels in the deep Atlantic: insights into colonization and biogeography of a chemosymbiotic species. Mar. Ecol. 36, 71–81. doi: 10.1111/maec.12217
Gustafson, R., Turner, R., Lutz, R., and Vrijen hoek, R. C. (1998). A new genus and five new species of mussels (Bivavlia, Mytilidae) from deep-sea sulfide/hydrocarbon seeps in the Gulf of Mexico. Malacologia 40, 63–112.
Gustafson, R. G., and Reid, R. G. B. (1988). Association of bacteria with larvae of the gutless protobranch bivalve Solemya reidi (Cryptodonta: Solemyidae). Mar. Biol. 97, 389–401. doi: 10.1007/BF00397769
Hauton, C., Brown, A., Thatje, S., Mestre, N. C., Bebianno, M. J., Martins, I., et al. (2017). Identifying toxic impacts of metals potentially released during deep-sea mining—A synthesis of the challenges to quantifying risk. Front. Mar. Sci. 2017:4. doi: 10.3389/fmars.2017.00368
Hilario, A., Cunha, M. R., Génio, L., Marçal, A. R., Ravara, A., Rodrigues, C. F., et al. (2015). First clues on the ecology of whale falls in the deep Atlantic Ocean: results from an experiment using cow carcasses. Mar. Ecol. 36, 82–90. doi: 10.1111/maec.12246
Ikuta, T., Takaki, Y., Nagai, Y., Shimamura, S., Tsuda, M., Kawagucci, S., et al. (2016). Heterogeneous composition of key metabolic gene clusters in a vent mussel symbiont population. ISME J. 10, 990–1001. doi: 10.1038/ismej.2015.176
Kenk, V. C., and Wilson, B. R. (1985). A new mussel (Bivlavia: Mytilidae) from hydrothermal vents in the Galapagos Rift zone. Malacologia 26, 253–271.
Kiel, S., and Amano, K. (2013). The earliest bathymodiolin mussels: an evaluation of Eocene and Oligocene taxa from deep-sea methane seep deposits in western Washington State, USA. J. Paleontol. 87, 589–602. doi: 10.1666/12-135
Kinoshita, G., Kawato, M., Shinoazaki, A., Yamamoto, T., Okoshi, K., Kubokawa, K., et al. (2010). Protandric hermaphroditism in the whale-fall mussel Adipicola Pacifica. Cah. Biol. Mar. 51, 423–427.
Kroeker, K. J., Kordas, R. L., Crim, R., Hendricks, I. E., Ramajo, L., Singh, G. S., et al. (2013). Impacts of ocean acidification on marine organisms: quantifying sensitivities and interaction with warming. Glob. Change Biol. 19, 1884–1896. doi: 10.1111/gcb.12179
Krylova, E. M., and Sahling, H. (2010). Vesicomyidae (Bivalvia): current taxonomy and distribution. PLoS ONE 5:e9957. doi: 10.1371/journal.pone.0009957
Kyuno, A., Shintaku, M., Fujita, Y., Matsumoto, H., Utsumi, M., Watanabe, H., et al. (2009). Dispersal and differentiation of deep-sea mussels of the genus Bathymodiolus (Mytilidae, Bathymodiolinae). J. Mar. Biol 2009:625672. doi: 10.1155/2009/625672
Laming, S. R. (2014). Ph.D. thesis, Université Pierre et Marie Curie. Available online at https://tel.archives-ouvertes.fr/tel-01135209
Laming, S. R., Duperron, S., Cunha, M. R., and Gaudron, S. M. (2014). Settled, symbiotic, then sexually mature: adaptive developmental anatomy in the deep-sea, chemosymbiotic mussel Idas modiolaeformis. Mar. Biol. 161, 1319–1333. doi: 10.1007/s00227-014-2421-y
Laming, S. R., Duperron, S., Gaudron, S. M., Hilário, A., and Cunha, M. R. (2015a). Adapted to change: the rapid development of symbiosis in newly settled, fast-maturing chemosymbiotic mussels in the deep sea. Mar. Environ. Res. 112, 100–112. doi: 10.1016/j.marenvres.2015.07.014
Laming, S. R., Szafranski, K. M., Rodrigues, C. F., Gaudron, S. M., Cunha, M. R., Hilario, A., et al. (2015b). Fickle or faithful: the roles of host and environmental context in determining symbiont composition in two bathymodioline mussels. PLoS ONE 10:e0144307. doi: 10.1371/journal.pone.0144307
Le Pennec, M., and Beninger, P. G. (1997). Ultrastructural characteristics of spermatogenesis in three species of deep-sea hydrothermal vent mytilids. Can. J. Zool. 75, 308–316. doi: 10.1139/z97-039
Le Pennec, M., and Beninger, P. G. (2000). Reproductive characteristics and strategies of reducing-system bivalves. Comp. Bioch. Physiol. Part A 126, 1–16. doi: 10.1016/S0742-8413(00)00100-6
Liu, J., Zhang, S., and Nagalingum, N. S. (2018). Phylogeny and evolutionary radiation of the marine mussels (Bivalvia: Mytilidae) based on mitochondrial and nuclear genes. Mol. Phyl. Evol. 126, 233–240. doi: 10.1016/j.ympev.2018.04.019
Lorion, J., Kiel, S., Faure, B., Kawato, M., Ho, S. Y., Mashall, B., et al. (2013). Adaptive radiation of chemosymbiotic deep-sea mussels. Proc. R. Soc. B. 280:20131243 doi: 10.1098/rspb.2013.1243
Lubey, P. (1959). Recherches sur le cycle sexuel et l'émission des gamètes chez les mytilidés et les pectinidés (Mollusques bivalves). Rev. Trav. Inst. Pêch. Marit. 23, 387–548.
Lutz, R., Jablonski, D., Rhoads, D., and Turner, R. D. (1980). Larval dispersal of a deep-sea hydrothermal vent bivalve from the Galapagos Rift. Mar. Biol. 57, 127–133. doi: 10.1007/BF00387378
Martins, I., Colaço, A., Dando, P. R., Martins, I., Desbruyères, D., Sarradin, P. M., et al. (2008). Size-dependant variations on the nutritional pathway of Bathymodiolus azoricus demonstrated by a C-flux model. Ecol. Model 217, 59–71. doi: 10.1016/j.ecolmodel.2008.05.008
McVeigh, D. M., Eggleston, D. B., Todd, A. C., Young, C. M., and He, R. (2017). The influence of larval migration and dispersal depth on potential larval trajectories of a deep-sea bivalve. Deep Sea Res. I 127, 57–64. doi: 10.1016/j.dsr.2017.08.002
Mestre, N. C., Thatje, S., and Tyler, P. A. (2009). The ocean is not deep enough: pressure tolerances during early ontogeny of the blue mussel Mytilus edulis. Proc. R. Soc. Lond. B. Biol. Sci. 276, 717–726. doi: 10.1098/rspb.2008.1376
Miller, K. A., Thompson, K. F., Johnston, P., and Santillo, D. (2018). An overview of seabed mining including the current state of development, environmental impacts and knowledge gaps. Front. Mar. Sci. 4:418. doi: 10.3389/fmars.2017.00418
Nix, E. R., Fisher, C. R., Vodenichar, J. S., and Scott, K. M. (1995). Physiological ecology of a mussel with methanotrophic endosymbionts at three hydrocarbon seep sites in the Gulf of Mexico. Mar. Biol. 122, 605–617. doi: 10.1007/BF00350682
Ockelmann, K. W., and Dinesen, G. E. (2011). Life on wood - the carnivorous deep-sea mussel Idas argenteus (Bathymodiolinae, Mytilidae, Bivalvia). Mar. Biol. Res. 7, 71–84. doi: 10.1080/17451001003714504
Page, H., Fiala-Médioni, A., Fisher, C., and Childress, J. J. (1991). Experimental evidence for filter-feeding by the hydrothermal vent mussel, Bathymodiolus thermophilus. Deep Sea Res. I. 38, 1455–1461. doi: 10.1016/0198-0149(91)90084-S
Pechenik, J. A. (1999). On the advantages and disadvantages of larval stages in benthic marine invertebrate life cycles. Mar. Ecol. Prog. Ser. 177, 269–297. doi: 10.3354/meps177269
Pelorce, J., and Poutiers, J. M. (2009). Une nouvelle espèce de Bathymodiolinae (Mollusca, Bivalvia, Mytilidae) associée à des os de baleine coulés en Méditerranée. Zoosystema 31, 975–985. doi: 10.5252/z2009n4a11
Pernet, F., Tremblay, R., and Bourget, E. (2003). Settlement success, spatial pattern and behavior of mussel larvae Mytilus spp. in experimental “downwelling” systems of varying velocity and turbulence. Mar. Ecol. Prog. Ser. 260, 125–40. doi: 10.3354/meps260125
Petersen, J. M., Wentrup, C., Verna, C., Knittel, K., and Dubilier, N. (2012). Origins and evolutionary flexibility of chemosynthetic symbionts from deep-sea animals. Biol. Bull. 223, 123–137. doi: 10.1086/BBLv223n1p123
Pile, A. J., and Young, C. M. (1999). Plankton availability and retention efficiencies of cold-seep symbiotic mussels. Limnol. Ocean. 44, 1833–1839. doi: 10.4319/lo.1999.44.7.1833
Pradillon, F., Schmidt, A., Peplies, J., and Dubilier, N. (2007). Species identification of marine invertebrate early stages by whole-larvae in situ hybridisation of 18S ribosomal RNA. Mar. Ecol. Prog. Ser. 333, 103–116. doi: 10.3354/meps333103
Pradillon, F., Shillito, B., Young, C. M., and Gaill, F. (2001). Deep sea ecology - developmental arrest in vent worm embryos. Nature 413:6857. doi: 10.1038/35099674
Ramirez-Llodra, E., Brandt, A., Danovaro, R., De Mol, B., Escobar, E., German, C. R., et al. (2010). Deep, diverse and definitely different: unique attributes of the world's largest ecosystem. Biogeosciences 7, 2851–2899. doi: 10.5194/bg-7-2851-2010
Ritt, B., Duperron, S., Lorion, J., Lazar, C. S., and Sarrazin, J. (2012). Integrative study of a new cold-seep mussel (Mollusca: Bivalvia) associated with chemosynthetic symbionts in the Marmara Sea. Deep Sea Res. Part I 67, 121–132. doi: 10.1016/j.dsr.2012.05.009
Rodrigues, C. F., Laming, S. R., Gaudron, S. M., Oliver, P. G., Le Bris, N., and Duperron, S. (2015). A sad tale: has the small mussel Idas argenteus lost its symbionts? Biol. J. Linn. Soc. 114, 398–405. doi: 10.1111/bij.12431
Rossi, G. S., and Tunnicliffe, V. (2017). Trade-offs in a high CO2 habitat on a subsea volcano: condition and reproductive features of a bathymodioline mussel. Mar. Ecol. Prog. Ser. 574, 49–64. doi: 10.3354/meps12196
Salerno, J. L., Macko, S. A., Hallam, S. J., Bright, M., Win, Y. J., McKiness, Z., et al. (2005). Characterization of symbiont populations in life-history stages of mussels from chemosynthetic environments. Biol. Bull. 208, 145–155. doi: 10.2307/3593123
Sarrazin, J., Cuvelier, D., Peton, L., Legendre, P., and Sarradin, P. M. (2014). High-resolution dynamics of a deep-sea hydrothermal mussel assemblage monitored by the EMSO-Açores MoMAR observatory. Deep Sea Res. Part I 90, 62–75. doi: 10.1016/j.dsr.2014.04.004
Shanks, A. L. (2009). Pelagic larval duration and dispersal distance revisited. Biol. Bull. 216, 373–385. doi: 10.1086/BBLv216n3p373
Signorelli, J. H., and Crespo, E. (2017). First record of the genus Adipicola (Mollusca: Bivalvia: Mytilidae) and description of a new species from the Argentine SW Atlantic Ocean. Zootaxa 4318, 325–338. doi: 10.11646/zootaxa.4318.2.6
Smith, C. R., and Baco, A. R. (2003). Ecology of whale falls at the deep-sea floor. Ocean Mar. Biol. Ann. Rev. 41, 311–354.
Stewart, F. J., Newton, L. G., and Cavanaugh, C. M. (2005). Chemosynthetic endosymbioses: adaptations to oxic-anoxic interfaces. Trends Microbiol. 13, 439–448. doi: 10.1016/j.tim.2005.07.007
Stramma, L., Schmidtko, S., Levin, L. A., and Johnson, G. C. (2010). Ocean oxygen minima expansions and their biological impacts. Deep Sea Res. I 57, 587–595. doi: 10.1016/j.dsr.2010.01.005
Streams, M. E., Fisher, C. R., and Fiala-Medioni, A. (1997). Methanotrophic symbiont location and fate of carbon incorporated from methane in a hydrocarbon seep mussel. Mar. Biol. 129, 465–476. doi: 10.1007/s002270050187
Szafranski, K. M., Deschamps, P., Cunha, M. R., Gaudron, S. M., and Duperron, S. (2015a). Colonization of plant substrates at hydrothermal vents and cold seeps in the northeast Atlantic and Mediterranean and occurrence of symbiont-related bacteria. Front. Microbiol. 6:162. doi: 10.3389/fmicb.2015.00162
Szafranski, K. M., Gaudron, S. M., and Duperron, S. (2014). Direct evidence for maternal inheritance of bacterial symbionts in small deep-sea clams (Bivalvia: Vesicomyidae). Naturwissenschaften 101, 373–383. doi: 10.1007/s00114-014-1165-3
Szafranski, K. M., Piquet, B., Shillito, B., Lallier, F. H., and Duperron, S. (2015b). Relative abundances of methane- and sulfur-oxidizing symbionts in gills of the deep-sea hydrothermal vent mussel Bathymodiolus azoricus under pressure. Deep Sea Res. Part I 101, 7–13. doi: 10.1016/j.dsr.2015.03.003
Taylor, J. D., and Glover, E. (2000). “Functional anatomy, chemosymbiosis and evolution of the Lucinidae,” in The Evolutionary Biology of the Bivalvia eds E. M. Harper, J. D. Taylor, and J. A. Crame (London: Geological Society), 207–25.
Thorson, G. (1950). Reproductive and larval ecology of marine bottom invertebrates. Biol. Rev. 25, 1–45. doi: 10.1111/j.1469-185X.1950.tb00585.x
Thubaut, J., Corbari, L., Gros, O., Duperron, S., Couloux, A., and Samadi, S. (2013a). Integrative biology of Idas iwaotakii (Habe, 1958), a “model species” associated with sunken organic substrates. PLoS ONE 8:e69680. doi: 10.1371/journal.pone.0069680
Thubaut, J., Puillandre, N., Faure, B., Cruaud, C., and Samadi, S. (2013b). The contrasted evolutionary fates of deep-sea chemosynthetic mussels (Bivalvia, Bathymodiolinae). Ecol. Evol. 3, 4748–4766. doi: 10.1002/ece3.749
Tunnicliffe, V., Davies, K. T. A., Butterfield, D. A., Embley, R. W., Rose, J. M., and Chadwick, W. W. (2009). Survival of mussels in extremely acidic waters on a submarine volcano. Nat. Geosci. 2, 344–348. doi: 10.1038/ngeo500
Tyler, P. A., Marsh, L., Baco-Taylor, A., and Smith, C. R. (2009). Protandric hermaphroditism in the whale-fall bivalve mollusc Idas washingtonia. Deep Sea Res. II 56, 1689–1699. doi: 10.1016/j.dsr2.2009.05.014
Tyler, P. A., and Young, C. M. (1999). Reproduction and dispersal at vents and cold seeps. J. Mar. Biol. U. K. 79, 193–208. doi: 10.1017/S0025315499000235
Tyler, P. A., and Young, C. M. (2003). Dispersal at hydrothermal vents: a summary of recent progress. Hydrobiologia 503, 9–19. doi: 10.1023/B:HYDR.0000008492.53394.6b
Tyler, P. A., Young, C. M., Dolan, E., Arellano, S. M., Brooke, S. D., and Baker, M. (2007). Gametogenic periodicity in the chemosynthetic cold-seep mussel “Bathymodiolus” childressi. Mar. Biol. 150, 829–840. doi: 10.1007/s00227-006-0362-9
Van Dover, C. L. (2014). Impacts of anthropogenic disturbances at deep-sea hydrothermal vent ecosystems: a review. Mar. Environ. Res. 102, 59–72. doi: 10.1016/j.marenvres.2014.03.008
Van Dover, C. L., Smith, C. R., Ardron, J., Arnaud Haond, S., Beaudouin, Y. C., Bezaury Creel, J. E., et al. (2011). Environmental Management of Deep-Sea Chemosynthetic Ecosystems: Justification of and Considerations for a Spatially Based Approach. Kingston. Jamaica: International Seabed Authority. Available online at: https://www.isa.org.jm/sites/default/files/files/documents/tstudy9.pdf
Von Cosel, R., and Salas, C. (2001). Vesicomyidae (Mollusca : Bivalvia) of the genera Vesicomya, Waisiuconcha, Isorropodon and Callogonia in the eastern Atlantic and the Mediterranean. Sarsia 86, 333–366. doi: 10.1080/00364827.2001.10425523
Vrijenhoek, R. C. (2010). Genetic diversity and connectivity of deep-sea hydrothermal vent metapopulations. Mol. Ecol. 19, 4391–4411. doi: 10.1111/j.1365-294X.2010.04789.x
Warén, A., and Carrozza, F. (1990). Idas ghisottii sp. n., a new mytilid bivalve associated with sunken wood in the Mediterranean. Boll. Malacol. 26, 19–24. Available online at: https://www.biodiversitylibrary.org/page/49956830#page/29/mode/1up
Wentrup, C., Wendeberg, A., Huang, J. Y., Borowski, C., and Dubilier, N. (2013). Shift from widespread symbiont infection of host tissues to specific colonization of gills in juvenile deep-sea mussels. ISME J. 7, 1244–1247. doi: 10.1038/ismej.2013.5
Wentrup, C., Wendeberg, A., Schimak, M., Borowski, C., and Dubilier, N. (2015). Forever competent: deep-sea bivalves are colonized by their chemosynthetic symbionts throughout their lifetime. Environ. Microbiol. 16, 3699–3713. doi: 10.1111/1462-2920.12597
Wilkens, S. L., Stanley, J. A., and Jeffs, A. G. (2012). Induction of settlement in mussel (Perna canaliculus) larvae by vessel noise. Biofouling 28, 65–72. doi: 10.1080/08927014.2011.651717
Won, Y., Young, C. R., Lutz, R. A., and Vrijenhoek, R. C. (2003). Dispersal barriers and isolation among deep-sea mussel populations (Mytilidae: Bathymodiolus) from Eastern Pacific hydrothermal vents. Mar. Ecol. 12, 169–184. doi: 10.1046/j.1365-294X.2003.01726.x
Yang, J. L., Li, Y. F., Bao, W. Y., Satuito, C. G., and Kitamura, H. (2011). Larval metamorphosis of the mussel Mytilus galloprovincialis Lamarck, 1819 in response to neurotransmitter blockers and tetraethylammonium. Biofouling 27, 193–199. doi: 10.1080/08927014.2011.553717
Young, C. M. (1994). “A tale of two dogmas: the early history of deep-sea reproductive biology,” in Reproduction, Larval Biology, and Recruitment of the Deep-Sea Benthos, eds C. M. Young, and K. J. Eckelbarger (New York, NY: Columbia University Press), 1–25.
Keywords: ontogeny, bathymodiolinae, nutrition, development, larvae, symbiosis, reducing habitats
Citation: Laming SR, Gaudron SM and Duperron S (2018) Lifecycle Ecology of Deep-Sea Chemosymbiotic Mussels: A Review. Front. Mar. Sci. 5:282. doi: 10.3389/fmars.2018.00282
Received: 13 April 2018; Accepted: 23 July 2018;
Published: 21 August 2018.
Edited by:
Alex David Rogers, University of Oxford, United KingdomReviewed by:
Chong Chen, Japan Agency for Marine-Earth Science and Technology, JapanAna Hilário, University of Aveiro, Portugal
Rhian G. Waller, University of Maine, United States
Copyright © 2018 Laming, Gaudron and Duperron. This is an open-access article distributed under the terms of the Creative Commons Attribution License (CC BY). The use, distribution or reproduction in other forums is permitted, provided the original author(s) and the copyright owner(s) are credited and that the original publication in this journal is cited, in accordance with accepted academic practice. No use, distribution or reproduction is permitted which does not comply with these terms.
*Correspondence: Sébastien Duperron, c2ViYXN0aWVuLmR1cGVycm9uQG1uaG4uZnI=