- 1Laboratoire des Sciences de l'Environnement Marin, Institut Universitaire Européen de la Mer, Université de Bretagne Occidentale, UMR 6539 CNRS/UBO/IRD/Ifremer, Plouzané, France
- 2Laboratoire des Sciences de l'Environnement Marin, Ifremer, UMR 6539 CNRS/UBO/IRD/Ifremer, Plouzané, France
- 3Laboratoire d'Océanologie et de Géosciences, Université du Littoral Côte d'Opale, UMR 8187 CNRS, Wimereux, France
- 4Institut des Molécules et Matériaux du Mans, UMR 6283 CNRS, Université du Maine, Le Mans, France
Marine plastic pollution is a major environmental issue. Given their ubiquitous nature and small dimensions, ingestion of microplastic (MP) and nanoplastic (NP) particles and their subsequent impact on marine life are a growing concern worldwide. Transfers along the trophic chain, including possible translocation, for which the hazards are less understood, are also a major preoccupation. Effects of MP ingestion have been studied on animals through laboratory exposure, showing impacts on feeding activity, reserve depletion and inflammatory responses, with consequences for fitness, notably reproduction. However, most experimental studies have used doses of manufactured virgin microspheres that may not be environmentally realistic. As for most ecotoxicological issues, the environmental relevance of laboratory exposure experiments has recently been debated. Here we review constraints and priorities for conducting experimental exposures of marine wildlife to microplastics based on the literature, feedback from peer reviewers and knowledge gained from our experience. Priorities are suggested taking into account the complexity of microplastics in terms of (i) aggregation status, surface properties and interactions with organic and inorganic materials, (ii) diversity of encountered particles types and concentrations, (iii) particle bioavailability and distribution in experimental tanks to achieve reproducibility and repeatability in estimating effects, and (iv) strict experimental procedures to verify the existence of genuine translocation. Relevant integrative approaches encompass a wide spectrum of methods from -omics to ecophysiological approaches, including modeling, are discussed to provide novel insights on the impacts of MP/NP on marine ecosystems from a long-term perspective. Knowledge obtained in this way would inform stakeholders in such a way as to help them mitigate impacts of the micro- and nano-plastic legacy.
Introduction
Production of plastics is constantly increasing to sustain our broadening uses in daily life (Thompson et al., 2009). As a direct consequence, plastic waste in the environment is a growing problem (e.g., Barnes et al., 2009); plastic waste entering the oceans was calculated for 2010 at 4-12 million tons per year and is predicted to increase by an order of magnitude by 2025 in the absence of waste management improvements (Jambeck et al., 2015). Once in the environment, plastic debris fragments into smaller particles such as microplastics (MP; <5 mm, NOAA, 2008), and presumably nanoplastics (NP; defined as particles <100 nm or <1,000 nm according to Galloway et al. (2017) and Gigault et al. (2018), respectively) whose presence in the Atlantic gyre has been recently suggested (Ter Halle et al., 2017). MP can also be produced as such, mainly in the form of microbeads used in cosmetics, synthetic fibers discharged with washing waters, and industrial abrasives. Microplastics have been reported in the environment worldwide, from surface waters to deep-sea sediments (Eriksen et al., 2013; Vianello et al., 2013; Wright et al., 2013b; Cózar et al., 2014; Lusher et al., 2015), even in areas far from human activities such as in polar waters (Lusher et al., 2015; Cózar et al., 2017; Munari et al., 2017; Obbard, 2018). All environmental matrices appear contaminated: surface waters (Moore et al., 2001; Eriksen et al., 2013), the water column (Lattin et al., 2004; Ng and Obbard, 2006), sediments (Van Cauwenberghe et al., 2013; Vianello et al., 2013) and biota (Murray and Cowie, 2011; Fossi et al., 2012; Lusher et al., 2013; Devriese et al., 2015; Figure 1).
Given their ubiquitous nature and small dimensions (Cózar et al., 2014), their ingestion by and subsequent impact on marine life-including transfer of biological or chemical contaminants-is a growing concern (e.g., Wright et al., 2013a). This is especially true when considering transfer along the trophic chain and possible translocation, for which the hazards are less well understood (GESAMP, 2016; Figure 1).
Overall, experimental studies focusing on the effects of MP on marine organisms have increased sharply over the past few years (Figure 2). Substantial effects have been reported on feeding activity, reserve depletion, impairment of oxidative balance and the immune system, and inflammatory responses, with impacts on animal fitness, notably reproduction (e.g., Wright et al., 2013a; Paul-Pont et al., 2016; Rochman et al., 2016; Sussarellu et al., 2016; Galloway et al., 2017). Effects may scale up to the community level, as suggested by recent publications demonstrating modifications in nutrient cycles and benthic assemblage structures upon exposure to MP (Green, 2016; Green et al., 2016), as well as increasing disease risk for coral communities (Lamb et al., 2018).
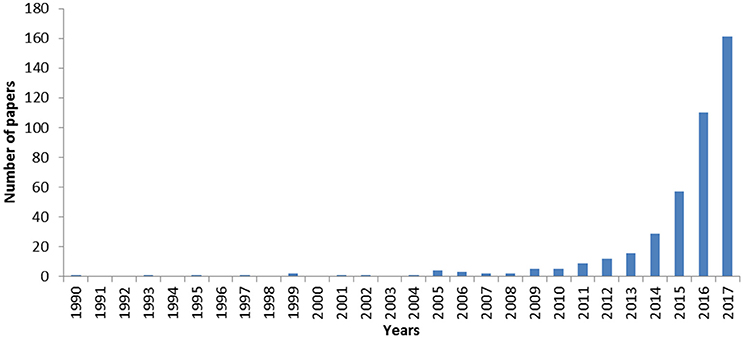
Figure 2. Number of published scientific papers related to the assessment of the microplastic effects on aquatic organisms through lab experiments over time. Source: Web of Science; Period: 1975-2017; Keywords: (microplastic* OR “micro-plastic*” OR nanoplastic* OR “nano-plastic*” OR “plastic particle*) AND (impact* OR exposure OR experimental OR lab* OR tank) AND (ocean* OR sea OR seas OR marin* OR seawater* OR water OR aquatic).
However, most previous experimental studies used unrealistic scenarios with mostly high doses of manufactured virgin microspheres that may not be representative of the variety of microplastics found in the environment. The difficulty of performing laboratory experiments to assess MP toxicity lies in the fact that MP consist of a complex, dynamic mixture of polymers and additives, to which organic material and contaminants can successively bind, along with microorganisms, influencing their density, surface charge, bioavailability and toxicity (Galloway et al., 2017). As a consequence, the environmental relevance of laboratory exposure experiments has recently been debated and challenged (Lenz et al., 2016; Rochman, 2016). In order to meet decision-makers' expectations, it is critical/essential to consider MP shape, interaction with organic matter, and the biological and chemical loads of microplastics, as well as using exposure doses as close as possible to environmentally realistic concentrations (Huvet et al., 2016; Karami, 2017). Based on the literature, feedback from peer reviewers and knowledge gained from our experience, we make a review of the constraints and priorities for experimental exposures of marine organisms to MP and NP. Having defined the main specific features of MP and NP that need to be taken into account in laboratory studies, we identify the major limitations that could be avoided in such studies, and discuss some ways to improve how MP and NP are handled in laboratory experiments. Finally, we cover the use of mesocosm experiments, which advantageously combine a similar level of control to that offered by laboratory experiments with some of the complexity of natural ecosystems. Such a combination may benefit from integrative analytical approaches ranging from -omics to ecophysiological approaches, including modeling, in order to objectively assess the complex and dynamic impacts of MP and NP on marine ecosystems.
Which Particles Should be Used in Laboratory Experiments?
Polymer Nature
Among the “Big Six” [polypropylene (PP), high- and low-density polyethylene (HDPE and LDPE), polyvinyl chloride (PVC), polyurethane (PUR), polyethylene terephthalate (PET), and polystyrene (PS)], which account for 80% of plastic production in Europe (PlasticsEurope, 2016) and are the most frequently reported plastics in marine environments (Browne et al., 2010; Karapanagioti et al., 2011; Vianello et al., 2013), polyethylene (PE) and PP are presently the polymers predominantly recovered in all environmental compartments (Isobe et al., 2014; Enders et al., 2015; Frère et al., 2017), in accordance with the scale of their global manufacture and use worldwide (Antunes et al., 2013; GESAMP, 2016). Assuming that the chemical nature of these polymers can modulate their impact, it appears relevant to test the big six first, both separately and in complex mixtures. However, experiments may also consider geographical differences especially in estuarine and coastal ecosystems, e.g., polyester is generally dominant in fibers collected at sea but it can be locally nylon due to local activities. Biodegradable polymers would deserve attention in the near future. Exposure experiments in the micro- and nano-ranges mainly use PS and, to a lesser extent, PE microbeads (reviewed by Phuong et al., 2016) due to the lack of commercial micro- and nano-beads made of other polymers. Previous studies testing the influence of polymer type are difficult to interpret as the detailed chemical compositions (including all additives among which some may be hazardous) are never given because manufacturers unwilling to provide them (e.g., Green et al., 2016). It therefore remains difficult to compare the effects of polymers based on their monomer chemistry when part of the other unidentified constituents may be as toxic if not more so than the monomers themselves (Hermabessiere et al., 2017). To address this issue and helping deciphering the respective influence of the physical (mechanical) and chemical toxicities of MP, the production of laboratory model polymers without additives or with controlled introduction of the most common additives would clarify the toxicity of MP according to their polymer nature. Other important aspects (such as morphology, weathering of MP) would be more hardly accounted for using laboratory produced model particles because natural weathering and organic coating in environmental conditions are complex processes that are difficult to mimic in laboratory. With the current level of knowledge, laboratory particles can be classified by size, by polymer nature, can be artificially weathered or even colonized by microorganisms.
Besides these aspects, polymers exhibit different densities that affect their buoyancy, behavior and bioavailability to marine organisms. As reported by Wright et al. (2013b), pelagic filter/suspension feeders and plankton feeders are more likely to encounter positively buoyant, low-density plastics such as PE (density 0.91–0.94) while high density plastics such as PET (density 1.38) are expected to sink, thus becoming available for supra-benthic and benthic suspension/deposit feeders as well as detritivores. Therefore, focusing on the most produced polymers according to their bioavailability for a given species in a given area is a key point to consider when assessing microplastic effects on model organisms. For instance, wild bivalves living close to a harbor may not be exposed to the same MP (paints, PE fragments) as those cultivated in high-density farming areas (PP rope fibers, PS foam fragments). Therefore, to improve experiment relevance, in-situ measurements of the types and forms of MP in a given area should be envisaged even though it may be very costly and time-consuming. Nevertheless, such data should be used with caution, as up to day traditional sampling methods (e.g., manta trawl) do not take into account vertical distribution and small size particles.
Shape
Several types of MP can be distinguished according to their morphology: spheres (beads, pellets, and granules) that are produced as such (primary MP); and fibers (filaments and lines), films, fragments, and foams (Free et al., 2014; Karami, 2017), which are produced from the fragmentation of larger plastic debris (secondary MP). Accumulation of MP of different shapes, such as planar, granular, fragments or fibers, has been shown in different organisms (Lusher et al., 2013; De Witte et al., 2014; Devriese et al., 2015; Li et al., 2015). Few studies have examined the relative contributions of different MP sources in aquatic environments. A first detailed estimation of the different sources of MP in Norway showed that the sources of primary MP (between 3.7 and 15.5%) are of minor importance compared with secondary MP sources (between 84.5 and 96.3%) (Sundt et al., 2014). If these estimates are correct and valid at a wider environmental scale, then studies using primary MP (such as microbeads) are disproportionally frequent compared with those using secondary MP. This imbalance has occurred because microbeads are the most easily commercially-available product to use in tests, mostly with embedded fluorescent labeling or dyes for easier detection (Yokota et al., 2017). Even though uniformly sized and shaped analytical grade commercial microbeads are useful tools for establishing the basic patterns of ingestion and organism responses to MP exposure, their exclusive use in laboratory experiments may lead to a biased representation relative to the full range of microplastics found in water bodies (Free et al., 2014; Mazurais et al., 2015; Figure 3). Indeed, Graham and Thompson (2009) revealed that some benthic organisms like sea cucumber could preferentially ingest plastic fragments over other shapes. Recently, Gray and Weinstein (2017) revealed that the ingestion, residence time and toxicity of particles in shrimp depended on particle shape and size. For instance, they showed a higher retention time of spheres than fragments in the gut, whereas in the gills they observed a hierarchy of retention patterns for fragments > spheres > fibers. Moreover, fragments and fibers increased the mortality rate of both shrimp and daphnia relative to beads (Gray and Weinstein, 2017; Ziajahromi et al., 2017) while no apparent post-ingestion effects were observed on brine shrimp (Artemia sp.) exposed to 10 × 40 μm nylon fibers (Cole, 2016). Given the lack of data regarding impacts of fibrous particles on organisms compared with their spherical counterparts, it appears necessary to assess their persistence in the environment to better understand their potential impacts.
The difficulty of conducting experiments with secondary MP lies in the fact that they are by definition more difficult to collect or produce, especially regarding the need to obtain sufficient amounts of relevant sizes. They are also more difficult to monitor over the course of laboratory exposures. These forms are indeed irregular and, with the exception of nylon tubes (Cole, 2016), most often non-standard in shape and size, making it difficult to design reproducible ecotoxicology studies. Furthermore, their composition, original source, and traceability are often impossible to determine consistently (Kedzierski, 2017).
Despite these limitations, using microfibers and fragments and not only beads are of interest. A recent method using a cryotome and Nile Red staining was developed to produce labeled nylon microfibers (40-100 μm length and 10–28 μm width) (Cole, 2016). To obtain realistic fragments, the best practical method is to mill plastic objects from everyday life to obtain MP of various sizes and shapes and/or use MP directly sampled at sea (Graham and Thompson, 2009; Ogonowski et al., 2016). Several approaches can be tested for comparison purposes and depending on the monomer nature: (i) physical degradation (milling) of commercial pellets in order to obtain fragments and (ii) milling of micro- and nano-thick films before and after photo-degradation, as plastics become more brittle after UV exposure. Samples are obtained in the form of a powder containing a wide range of particle size and shape, which would be expected to better mimic real particles in the environment. Such a powder would require dissolution in an appropriate solvent, which will also require testing for intrinsic toxicity before use (as done with Tween in Ostroumov, 2003; Paul-Pont et al., 2016). Because it is difficult to fine tune such milling, sieving may be necessary to remove an unwanted fraction, especially the smallest sizes such as NP, or to produce specific size classes according to the ingestion capacity of the studied animals. The first major pre-requisite to perform laboratory experiments using laboratory-generated fragments and fibers is to accurately determine size distribution, shape and behavior (buoyancy and aggregation) in seawater. Some of these parameters (e.g., size and/or shape) can be monitored using microscopy coupled with image analysis software or an electronic particle counter mostly employed for phytoplankton counts (Huvet et al., 2015). More complete but unaffordable techniques are Atomic Force Microscopy (or laser granulometry) and 3D-optical profilometry (morphology and surface properties), scanning and transmission electron microscopy (shape and size), and diffusion light scattering or x-rays for NP and small MP (aggregation, charge). A major drawback of these techniques is that they can be quite time consuming. It is noteworthy that milling large plastic objects to obtain enough micro-fragments of appropriate and precise size range is still very challenging and requires significant methodological improvements.
Size
In the natural environment, marine organisms encounter plastic pieces with a wide size range from nanometers to meters (e.g., Mattsson et al., 2015; Galloway et al., 2017; Ter Halle et al., 2017). Particle size controls the probability of consumption and thus potential adverse effects (Wright et al., 2013b). In the literature, different approaches have been tested to study MP ingestion, trophic transfer and impacts: (i) exposure to a specific size (Farrell and Nelson, 2013; Setälä et al., 2014; Watts et al., 2016); (ii) exposure to several sizes separately (Lee et al., 2013; Cole and Galloway, 2015; Jeong et al., 2016, 2017); and (iii) exposure to a mixture of different sizes (Von Moos et al., 2012; Avio et al., 2015; Green, 2016; reviewed by Phuong et al., 2016). All approaches offer different advantages. Using a broad range of sizes helps to determine the size range of particles that organisms can ingest or interact with. The experiment performed by Erni-Cassola et al. (2017) with a mixture of MP from a few micrometers to 5 mm, sampled from surface sea waters, is particularly relevant in this respect. However, ensuring homogeneous distribution of particles across experimental conditions may be difficult to achieve and reproduce. Most studies conventionally chose an MP size distribution in the same range as that of the test organisms' preys. For example, particles from 7 to 30 μm appeared to be preferentially ingested by several zooplankton groups (decapods larvae, copepods and chaetognaths) although this varied according to species (Cole et al., 2013). Adult Pacific oysters Crassostrea gigas preferentially ingested 6-μm spheres over 2 μm ones with a 5-fold difference (Sussarellu et al., 2016). The size range of ingested particles also depends on biological stage. Indeed, the diameter of the mouth of a juvenile oyster was estimated at around 80 μm, and even smaller during the larval stages (Cole and Galloway, 2015), thus limiting the size of particles ingested.
Although MP ingestion is certainly the most studied entry process, it is not the only mechanism of entry, especially when considering nanoplastics (NP). Besides the usual digestive ingestion, respiration processes of organisms such as fish can also favor MP-NP entry. Effects were also reported to be size-dependent, especially for nanometric sizes, due to the physico-chemical properties of NP (Lee et al., 2013; Jeong et al., 2016, 2017; Mattsson et al., 2017). MP ingestion is often reported to cause problems in the digestive tract (satiation, clogging, inflammations, ulcers, etc.) with impacts expected on energy balance (Sussarellu et al., 2016), whilst potential translocation of NP could induce different adverse effects within organs and on various physiological functions (Kashiwada, 2006; Wright et al., 2013b; Mattsson et al., 2015, 2017). Mixing particles of different sizes clearly produces a more realistic scenario (Von Moos et al., 2012; Mazurais et al., 2015) and should be favored in laboratory exposures as long as the particle size distribution is well characterized prior to the experiment. Measuring the preferentially ingested size by analysis of gut and fecal content using histology, digestible fluorescent coating (Karakolis et al., 2018), microscopy and/or cytometry tools would also help to identify the most bioavailable and, putatively, the most toxic fraction according to species, physiological state and biological stage. It would also allow the assessment of potential digestive fragmentation processes that could reduce MP to NP, as recently demonstrated for Antarctic krill Euphausia superba (Dawson et al., 2018).
Concentrations
The need of elucidating eco-toxicity of MP is the main justification for testing concentrations of MP far above those found in marine waters (Pittura et al., 2018). Applying environmentally relevant microplastic doses is still a challenge for decision support (Huvet et al., 2016; Lenz et al., 2016; Rochman, 2016) especially when considering the lack of consistent field quantifications of MP as small as those used in most experimental studies (Filella, 2015). Environmental data on MP contamination in surface water and sediment are numerous (Table 1) and are compiled in the freely available Litter Database “Litterbase”(http://litterbase.awi.de/litter)1 Among reported values, some of the highest have been found in the southern North Sea (1,700,000 items m−3; ~ 8.5 mg L−1) for debris >80 μm (Dubaish and Liebezeit, 2013); in South Korea (15,560 items m−3; ~ 0.0778 mg L−1) for debris >333 μm (Song et al., 2014), and in a Swedish bay (2,400 items m−3; ~0.012 mg L−1) for debris >80 μm (Norén, 2007; Table 1). The MP (2 and 6 μm) mass concentration used for exposure in Sussarellu et al. (2016) and Paul-Pont et al. (2016), for instance, was in the range of the highest estimated field concentration obtained from manta trawl sampling: >333 μm. However, the corresponding number of particles per volume was on average 1,000 times higher than the highest estimate of 1.7 MP mL−1 for particles >80 μm obtained by Dubaish and Liebezeit (2013). However, small MP were recently demonstrated to be increasingly abundant following a power-law increase (by a factor of ~2.2) with a decreasing particle size in sea surface samples (Erni-Cassola et al., 2017). Similarly, recent publications demonstrated a high percentage (80%) of small MP (25–50 μm, for which few data are available) in surface water or sediment, compared with large sized particles (Enders et al., 2015; Bergmann et al., 2017). Applying the power-law increase factor calculated by Erni-Cassola et al. (2017) to the >80 μm plastic debris collected by Dubaish and Liebezeit (2013) gives an estimated average of 1,000 MP mL−1 for particles whose size is centered around 4 μm, and therefore in the range of those used in Sussarellu et al. (2016) and Paul-Pont et al. (2016), but still several orders of magnitude higher than the estimate of 1.4 MP mL−1 based on (Norén, 2007) data. If such a high concentration for small MP was confirmed in local aquatic hotspots, the environmental relevance of “high” concentration previously used in most laboratory experiments should be carefully revised. This is especially true for benthic species, as MP concentration at the sediment–water interface is estimated to be high, reaching up to 16.9 mg L−1 (Besseling et al., 2014a,b). Plastic contamination is indeed estimated from surface layer sampling, although small MP seem to have a lower residence time than larger debris in this compartment (Enders et al., 2015). This partly explains the lower contamination level of surface water compared to sediment, especially at the water–sediment interface (Vianello et al., 2013; Martin et al., 2017). For still smaller particles, the detection and quantification of the nano-fraction in natural environments remains a challenge. Promising studies are on their way, with a recent first demonstration of nano-sized putative plastic particles (comprised between 1 and 999 nm) in natural seawater samples collected in the North Atlantic Subtropical Gyre (Ter Halle et al., 2017), and the very recent high contamination levels discovered in Artic (Peeken et al., 2018). Combination of analytical methods such as asymmetric field flow fractionation (A4F), dynamic light scattering (DLS) and pyrolysis gas chromatography coupled with mass spectrometry (Pyr-GC/MS) may allow the determination of nano-sized particle concentrations in natural environments in the near future; the next challenge will be quantification to determine the level of contamination by small microplastics faced by marine organisms. Meanwhile, until data on NP and the smallest MP become available, the use of dose–response experiments like those performed by Jeong et al. (2016, 2017) is of interest. Indeed, although effects of MP may not be considered as “dose-dependent” in natural conditions, this approach could still provide relevant insights on toxicity thresholds for a given contaminant and organism.
Weathering Implications
Modification of Surface Properties
Once they have entered the environment, all plastic fragments or pellets undergo aging, a term used to encompass all changes in polymer properties over a given period of time. These changes can independently and/or simultaneously affect polymer composition, and modify the physical integrity of the particle and its surface properties (White, 2006). When such changes occur after a long time in the natural environment, the term used is “natural” aging or weathering. Weathering of polymers in the marine environment leads to polymer degradation through the addition of a number of complex processes: organic matter coating of their surface, photo-oxidation, hydrolysis, mechanical abrasion, additive release and pollutant adsorption, micro-organism colonization, and possibly biodegradation. Surface coverage by a complex mixture of organic and inorganic molecules (defined as an “ecocorona”; Galloway et al., 2017) is the first modification of surface properties that occurs when MP and NP are introduced into natural seawater, as this brings them immediately into contact with a more complex medium containing natural colloids, inorganic (e.g., poly-ions and minerals) and organic matter (e.g., mixtures of polysaccharides, proteins, lipids, and nucleic acids, Keller et al., 2010). The nature of the ecocorona will have a greater influence on smaller than larger particles due to their higher surface to volume ratio (Lin et al., 2014; Mattsson et al., 2015) and may change plastic surface characteristics (Canesi and Corsi, 2016), their identity, and the way they interact with organisms. For instance, an ecocorona around polystyrene NP created by proteins released by Daphnia magna caused heightened uptake, retention, and toxicity of NP (Nasser and Lynch, 2016). Ecocorona formation and colonization by microorganisms occur rapidly, at the scale of hours to days (see section Biofouling and Hetero-Aggregation), while other weathering mechanisms such as additive leakage and photo-oxidation are longer term processes (taking months or years; Figure 4). Moreover, complex interactions can occur between processes, e.g., the presence of a biofilm on the polymer surface can hinder photo-oxidation, but degradation and fragmentation of polymers increases their active surface available for colonization (Rummel et al., 2017). Hence, over their stay in the environment, pieces of plastic debris will exhibit various physical and chemical properties as well as biological modifications (Figure 4). Their geometries, surface properties and chemical composition will be permanently altered (Andrady, 1990; Rajakumar et al., 2009) and these changes could strongly modify their behavior, bioavailability, and ultimately their toxicity.
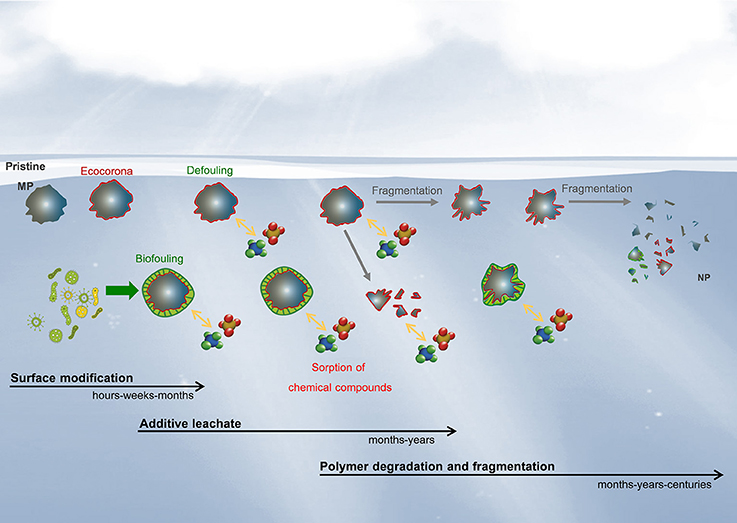
Figure 4. Hypothetical evolution of the physicochemical and biological modifications of microplastics and nanoplastics released in aquatic environments.
The results of very long weathering times on polymer fragments in the marine environment have recently been described by Ter Halle et al. (2017), based on samples from the North Atlantic Gyre. These authors showed that even the most robust polymers such as PE were severely damaged during weathering, with a decrease in molar mass and degradation of polymer chains, especially for micro-sized fragments (<1 mm). Regarding macroplastics, a significant decrease of the native functional groups of PET bottles was reported from approximately 15 years spent in marine environment (Ioakeimidis et al., 2016). Most exposure studies are conducted using pristine polymers. However, comparing pristine and weathered PE pellets over a few days to a few months in the environment (Rochman et al., 2013b, 2014; Nobre et al., 2015; Bråte et al., 2018) has revealed different effects on exposed organisms. Indeed, in one case, fish were more impaired when exposed to weathered pellets than new ones, possibly as a consequence of organic compound adsorption on the polymers during their “life” in marine waters (Rochman et al., 2013b). In contrast, Nobre et al. (2015) reported lower toxicity of stranded pellets than pristine ones, suggesting that polymers release part of their additives during weathering. These discrepancies are not surprising as it is assumed that the effects of environmental weathering of plastic particles will differ depending on both polymer and additive compositions, as well as on the environmental conditions during weathering. For instance, PE, PP, and PS pellets exposed to UV radiation showed different degradation states according to the environment (air > ultrapure water > synthetic seawater) as well as discrepancies in terms of surface functional groups and textures according to polymer type, as observed by FTIR, Raman, and SEM (Cai et al., 2018). Further experiments should be conducted to determine the importance of plastic weathering in impact studies.
To increase the environmental relevance of laboratory exposures, MP weathered in natural or artificial environments (from several days to weeks) can be prepared from pristine plastics to allow ecocorona formation and modification of chemical, physical and biological surface properties of the particles as detailed in the following sections.
Particle Charges in Seawater
MP and NP charge and aggregation have been little reviewed compared with their other aspects. In the absence of sedimentation, sub-micron particles move freely due to Brownian motion in water and finally aggregate (coagulate or flocculate) over time. This behavior depends highly on the particle surface charges and on the nature of the ions in the medium. In the absence of surface charges, hydrophobic particles are not thermodynamically stable and aggregate very easily, forming agglomerates. However, when particles interact with molecules present in seawater they can gain surface charges (Keller et al., 2010). Interestingly, this interaction differs according to plastic characteristics such as composition and size, as noted by Fotopoulou and Karapanagioti (2015) who studied the surface alteration of beached polyethylene (PE) and polypropylene (PP) plastic pellets compared with virgin pellets. The beached PE pellets mostly had a highly eroded surface and a negative charge, while virgin plastics and beached PP pellets had a neutral charge. In recent years, particle charge has been considered important in the field of plastics due to increasing production and use of small plastic particles (micro- and nano-sized) in various consumer products (Leslie, 2012). Materials that are insoluble in water when they are neutralized, can become soluble if they are charged since electrostatic repulsions fight against attractive van der Waals forces, as explained by classic DLVO theory (named after its authors Derjaguin, Landau, Verwey, and Overbeek; Derjaguin, 1941; Verwey et al., 1999). This is well illustrated for NP, considering hydrophobic dispersions stabilized with surface charges in water. Most commercially-available nano-beads are made of polystyrene (PS) and commonly have surface functionalization, with anionic (COOH) or cationic (NH2) groups displaying negative and positive charges, respectively, to give them stability (Casado et al., 2013). Nevertheless, once resuspended in seawater, the charge can change following complex molecular mechanisms due to interaction of the surface groups with high number of ions (Hofmeister, 1888; Cole and Galloway, 2015). In the rich environment of marine salts, specific ions might have specific chemical interactions with surface groups and thus neutralize particle charges (Hofmeister, 1888). In addition, Afshinnia et al. (2018) reported that natural colloids from the medium (e.g., humic substances) could influence the charge of the particles, suppressing the positive charge and enhancing the negative one, depending on both the point zero charge (pzc) of the particles and the pH of the solution. An ecocorona can favor the adhesion of the particles to each other or instead reduce their flocculation in accordance with new surface affinities (Yu et al., 2017). MP and NP charge is an important factor since surface properties play a notable role in determining its effects on organisms. Several studies have revealed a higher impact of positively charged nano-polystyrene particles on different marine organisms: Crustacea (Bergami et al., 2016, 2017; Nasser and Lynch, 2016) Bivalvia (Balbi et al., 2017) Equinodea (Della Torre et al., 2014), and Chlorophyceae (Bergami et al., 2017). This may be due to the interaction between the positive charge and biological membranes, which generally contain at least a small fraction of negatively charged lipids among larger numbers of neutral or zwitterionic ones (Rossi et al., 2014). Aggregation and charge of NP employed in experiments should be monitored to avoid confounding effects and misinterpretation.
Chemical Aspects
MP can be associated with many chemical agents such as hydrophobic organic chemicals (HOC) and additives (Oehlmann et al., 2009; Teuten et al., 2009). This is of concern as these contaminants can be noxious for wildlife and cause effects such as endocrine-disruption (Talsness et al., 2009; Teuten et al., 2009; Manikkam et al., 2013). In natural environments, sorption of HOC to plastic waste occurs as a result of their hydrophobic characteristics and the magnitude of this sorption is chemical and polymer dependent (Rochman et al., 2013a; Bakir et al., 2014). Evaluating risks associated with MP as vectors of HOC has been widely done in laboratory exposure experiments and an increase in HOC bioaccumulation in organism tissues has often been reported after exposure to contaminated MP (Teuten et al., 2007; Besseling et al., 2013; Rochman et al., 2013b; Avio et al., 2015), although not systematically (Paul-Pont et al., 2016). In vitro studies demonstrated that MP transfer through the digestive tract can enhance leaching of HOC due to changes in pH and temperature (Bakir et al., 2014). However, to our knowledge, most experimental studies carried out so far did not take into account (i) the potential bias due to their unrealistically high MP concentrations, and (ii) the role of other suspended particles (detritus, colloids, bacteria, phytoplankton, organic matter, food, etc.) capable of transferring HOC in higher amounts because of their higher abundance in marine ecosystems compared with MP (Bakir et al., 2016; Herzke et al., 2016; Koelmans et al., 2016; Paul-Pont et al., 2016; Besseling et al., 2017). Koelmans et al. (2016) extensively reviewed this question using field, laboratory and modeling data and concluded that, given the currently known low concentration of large MP (>333 μm) in the oceans, exposure to HOC via plastic is likely to be negligible compared with other natural pathways. However, a possible shift from mechanical to chemical toxicity through the release of additives and HOC according to time of exposure (from 7 up to 28 days), was recently hypothesized (Pittura et al., 2018). Caution must be taken however as (i) marine plastic litter is expected to increase over coming decades and the concentrations above which an effect can be seen on HOC bioaccumulation could be exceeded locally; and (ii) little is known regarding the NP and small MP fraction in the oceans (<10 μm; see section Concentrations), which could mean that we are currently underestimating MP concentrations in the oceans. The relative importance of such NP and small MP fractions in the transfer of HOC might still be underestimated, especially considering that for the same plastic mass, the surface available for HOC adsorption is inversely related to the size of plastic debris pieces (Velzeboer et al., 2014). Finally, the presence of an ecocorona is also expected to influence the plastic–HOC interaction by modifying HOC sorption processes (Koelmans et al., 2009) and oxidation, leading to the potential production of metabolites that may be more toxic than the original compound. There is a need to further testing to what extent organic matter/ecocorona limits or, on the contrary, favors HOC adsorption and detrimental impacts (Galloway et al., 2017).
Far fewer studies have focused on plastic additives or plastic leachates than on HOC, although plastic additives are widely used throughout manufacturing to improve plastic properties (flame retardants, plasticizers, stabilizers, antioxidants, etc.) at very high concentrations ranging from 7% (non-fibrous plastic; Geyer et al., 2017) to 60% (PVC; Net et al., 2015) of the plastic polymer mass. Transfer and toxicity of plastic additives to marine organisms upon plastic ingestion has been demonstrated both in laboratory experiments and field studies (Browne et al., 2013; Rochman et al., 2013b). However, Koelmans et al. (2014) demonstrated via modeling approaches that only a limited transfer of nonylphenol and bisphenol A occurred from MP to both lugworm and North Sea cod, compared with aqueous environmental concentrations of these additives. Indeed, leaching of plastic additives from plastic debris to surrounding seawater may occur rapidly and concentrations of the major additives (phthalates, bisphenol A, polybrominated diphenyl ethers, and nonylphenols) ranging from pg L−1 to μg L−1 have been recorded in natural environments (Hermabessiere et al., 2017). Many studies have reported significant toxicity of plastic leachates (obtained from various plastic debris) on aquatic organisms such as fish, bivalves and crustaceans (reviewed in Hermabessiere et al., 2017). More recently, Martínez-Gómez et al. (2017) focused on the commercially-available particles often used in laboratory experiments, reporting significant toxicity of virgin and aged PS and HPDE MP as well as their leachates on the fertilization success and embryonic development of sea urchin. Interestingly, plastic leachates were found to have higher embryo-toxicity than the virgin and aged materials themselves. Most commercial brands offer particles that are supposed to be free of additives or residual monomers; however, most of the time no technical specifications are available from the supplier. For instance, a chemical analysis performed on virgin micro-PS (Polysciences) revealed putative endocrine disruptors such as bibenzyl and 1(2H)naphthalenone,3,4,dihydro4phenyl (Sussarellu et al., 2016). Overall, such studies suggest that commercial microplastics frequently used as model materials in laboratory experiments may leach unknown chemicals such as additives or residual toxic monomers. With the aim of understanding mechanical effects of MP, washing commercial MP in seawater is relevant to allow the leaching of unwanted adsorbed toxic compounds before performing exposure experiments. Alternatively, studies focusing on comparing additive-free MP vs. MP loaded with known amounts of specific additives are of interest to ascertain both physical and chemical toxicities resulting from MP ingestion.
Biofouling and Hetero-Aggregation
Besides contaminants, microorganisms, and rafting organisms can rapidly develop at the surface of plastic debris on the ecocorona layer (Galloway et al., 2017) within the first few hours (Datta et al., 2016). Yokota et al. (2017) reported that, regardless of their size, positively charged plastics promote microorganism adhesion. Recent studies using high-throughput sequencing have demonstrated that MP-associated microorganism assemblages (including bacteria, microalgae, protozoans and fungi; no information for viruses is yet available) are distinct from those present in the surrounding media or other particulate organic and inorganic matter (McCormick et al., 2014; Amaral-Zettler et al., 2015; De Tender et al., 2015). These specific plastic-associated communities were recently termed the “plastisphere” by Zettler et al. (2013).
Despite an increased research interest in the characterization of the communities forming biofilms on MP, very little is known regarding the reciprocal effects of these biofilms on MP fate (reviewed in Rummel et al., 2017). Although most experimental studies investigating MP impacts on marine biota have used manufactured virgin MP without taking into account the absence/presence of a biofilm on the particles and/or the formation of hetero-aggregates, a few exceptions included these aspects (Green, 2016; Martínez-Gómez et al., 2017). A biofilm can lead to the cohesion of plastic particles with microorganisms which can be defined as hetero-aggregation. This shortcoming needs to be carefully addressed, as hetero-aggregate formation has been shown to influence MP vertical distribution in the water column (Campos et al., 2013; Long et al., 2015), which will inevitably modify the availability of MP for pelagic vs. benthic organisms (Long et al., 2017; Yokota et al., 2017). Modifications of size, shape and surface properties on biofilm/hetero-aggregate formation may also affect the ingestion of MP by zooplankton and filter feeders. For instance, 3-week-aged microbeads were preferred over pristine ones by females of Acartia longiremis as well as by juvenile copepodites and adult Calanus finmarchicus (Vroom et al., 2017). The preference for aged MP was suggested to be linked to the formation of a biofilm containing similar microbes to those that copepods feed on in the water column, secreting chemical exudates that enhance chemo-detection and particle attractiveness as food items. Also, ingestion of nano-PS (100 nm) by mussels was increased when they were incorporated into hetero-aggregates rather than remaining as free particles, which were probably too small to be efficiently retained by the gills (Ward and Kach, 2009). Conversely, large hetero-aggregates may not be efficiently ingested, possibly explaining the accumulation of MP on the deep-sea floor in the absence of major grazing processes (Van Cauwenberghe et al., 2013). In addition, biofilm formation can (i) alter the diffusion of HOC from or into the particles as previously shown for passive samplers, usually made of PE, and glass beads (Wicke et al., 2008; Harman et al., 2009), and (ii) facilitate the metabolism of HOC leading to the degradation of bio-degradable contaminants including PAH, heavy metals, and pharmaceutical compounds and/or the production of metabolites of greater toxicity (Sowada et al., 2017; Tiwari et al., 2017). Therefore, this aspect should be taken into account in laboratory studies focusing on the role of MP in the transfer of contaminants into marine organisms/ecosystems.
Finally, a few recent studies investigated the role of MP microbial assemblages as vectors for pathogens in vitro (Foulon et al., 2016) and in vivo after passage through the gut of lugworms (Kesy et al., 2016) and mussels (Kesy et al., 2017). Preliminary results suggest a minor role of the particle itself and a greater importance of the presence of primary colonizers influencing chemotactic attraction to the particle surface. Furthermore, a laboratory trial attempted to clarify the role of microplastics as possible vectors of pathogenic vibrios in oysters (Cassone et al., 2014), but methodological limitations prevented us from drawing any conclusion as it was impossible to work out the origin of the detected infection in the oysters, i.e., to distinguish between the Vibrio aestuarianus attached to the plastic beads and the free V. aestuarianus bacteria still present in the seawater medium at low concentrations.
Finally, using mostly secondary microplastics collected at sea that have undergone physicochemical and biological modifications related to weathering processes in the environment may appear at first sight as a nice environmentally relevant approach. However in such experimental design, it may be difficult or even impossible to disentangle the potential observed toxicity as some may be due to partially unknown molecules or microorganisms at the particle surface (Figure 5). If experiments are performed using particles not collected from the environment (i.e., home-made or commercially-available MP), washing and weathering pristine microplastics in natural seawater before performing exposure experiments will allow (i) the modification of MP surface properties including modification of surface charge, formation of a natural ecocorona and biofilm colonization; and (ii) the leaching of unwanted adsorbed toxic compounds in order to get closer to realistic environmental conditions (Figures 5, 6).
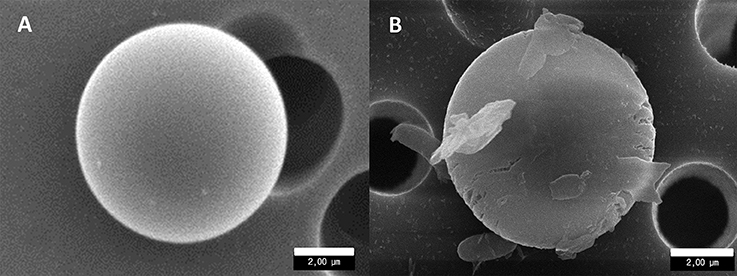
Figure 6. Scanning electron microscopy observations of 5-μm PMMA beads in pristine condition (A) and showing signs of erosion, presence of an organic corona and attached bacteria (B).
Achieving Reproducible and Environmentally Relevant Exposure of Marine Organisms
Phytoplankton
Only a few studies have explored the interactions of MP and NP with phytoplankton and related biological impacts (Bhattacharya et al., 2010; Davarpanah and Guilhermino, 2015; Lagarde et al., 2016; Sjollema et al., 2016; Long et al., 2017; Zhang, C. et al., 2017). Most of these studies were limited to experimental approaches and monospecific cultures. Although the tested concentrations of MP were high, ranging from 4 mg to 2 g L−1, overall effects on growth and physiological parameters were low or absent. Significant impact on growth rate was reported for the marine flagellate Dunaliella tertiolecta exposed to 250 mg L−1 of 50 μm micro-PS (Sjollema et al., 2016). Exposure to micro-PVC (average 1 μm) at a concentration of 50 mg L−1 led to a significant reduction of Skeletonema costatum growth and photosynthetic activity (Zhang, C. et al., 2017). These pioneering studies produced some conflicting results and do not allow us to confidently exclude that environmental concentrations of MP/NP could negatively affect freshwater and marine phytoplankton in aquatic environments. While the ecological relevance of laboratory experiments is arguable because they are far from reflecting the complexity of the marine environment, they may still contribute to exploring the effect of MP/NP pollution on phytoplankton. However, biases, artifacts, and experimental flaws need to be borne in mind.
Prior to considering the effects of MP/NP on phytoplankton per se, it is of primary importance to first assess how MP/NP and phytoplankton/microalgae interact with each other. Indeed, MP/NP and microalgae interactions depend on particle physico-chemical characteristics (including size, shape, and charge) and on the species and physiological status of the microalgae (Lagarde et al., 2016; Long et al., 2017). For instance, hetero-aggregation of 2-μm PS with microalgal cells appeared more frequently with diatoms than with Prymnesiophycea or dinoflagellates (Long et al., 2017). This study also revealed that formation of hetero-aggregates is favored during the stationary phase of microalgal culture, probably in relation to an increase in cell stickiness, exopolysaccharide (EPS) production, and/or bacterial aggregates that changed with the age of the culture (Long et al., 2017). However, as in most of the studies cited above, absence of EPS measurement is a major flaw when considering the influence of the ecocorona on MP and NP behavior (see section Modification of Surface Properties).
The other forgotten player in phytoplankton–MP/NP interactions is the bacterial community associated with microalgal culture (such cultures are rarely, if ever, axenic), which varies in concentration and species assemblage according to microalga species, origin, culture condition and age. It is of high interest (as for all kinds of experiments using phytoplankton culture) to quantify bacterial concentration and describe their general characteristics using microscopy and cytometry tools. If some significant influence of the bacterial community is suspected, additional characterization by molecular tools should be considered.
Also, as for any experimental exposure of organisms to MP/NP, it is paramount to quantify the bioavailability and distribution of MP in the experimental systems. MP distribution in different media (e.g., suspended, floating, adsorbed to experimental containers, trapped in organic aggregates, or adsorbed on microalgae or bacteria) must be assessed for each species and experiment to obtain accurate values of the actual MP concentration to which the microalgae are exposed. Long et al. (2017) clearly showed that micro-PS may attach to glassware, form homo-aggregates and hetero-aggregates with phytoplankton cells, residual organic matter, and/or bacterial exudates. Unfortunately, these control measurements, which are tedious and time consuming, need to be performed for every MP/NP tested as their distribution and behavior is expected to change according to polymer nature, size, shape, charge, biofilm and additive content.
Zooplankton, Fish, and Shellfish
There is abundant recent literature examining the presence of MP in different organisms including zooplankton, fish, and shellfish species in different natural marine environments (e.g., Desforges et al., 2014; De Witte et al., 2014; Van Cauwenberghe and Janssen, 2014; Devriese et al., 2015; Rummel et al., 2016; Wesch et al., 2016; Güven et al., 2017; reviewed in Phuong et al., 2016). These surveys clearly revealed a high variability of MP ingestion regardless of the trophic level of the fish or shellfish species concerned; they also tended to indicate a higher frequency of contamination in pelagic feeders and suspension/filter feeders than in other groups. Ingestion rates measured on natural zooplankton communities revealed that 83% of Brown shrimps assessed (Nephrops sp.) in the north Clyde Sea (Murray and Cowie, 2011), 63% of shrimps (Crangon crangon) in the UK and 3% of the copepod Neocalanus cristatus and 6% of the euphausids Euphasia pacifica in the northeast Pacific consumed plastic debris, most of which were fragments or fibers (Desforges et al., 2014; Devriese et al., 2015). Studies on fish reported between 2 and 40% of individuals to be contaminated, with a mean number of particles from 1 to 7.2 per individual (Boerger et al., 2010; Foekema et al., 2013; Lusher et al., 2013). For mollusks, especially mussels, this MP load varied from 0.2 to 0.5 plastic particles per gram of tissue (De Witte et al., 2014; Van Cauwenberghe and Janssen, 2014) while for zooplankton contamination generally increased with the size of the organisms and ranged from mean value of 0.026 ± 0.05 for copepods (Desforges et al., 2014) to 1.23 ± 0.99 particles ind−1 for shrimps (Devriese et al., 2015). One practical consequence of these observations is the fact that bioassays for studying MP impacts on both zooplankton and fish could be implemented according to taxonomic group, species developmental stage and trophic level. Mode of feeding is an additional factor to take into account when considering exposure experiments with fish and jellyfish (e.g., ctenophores and cnidarians). The buoyant or non-buoyant nature of the targeted MP will also strongly dictate the species chosen to assess the effects of MP and the mode of exposure (bathing vs. trophic pathway).
Bathing is certainly the most prevalent exposition mode used in MP bioassays, probably because it is technically the easiest to implement even though the equal probability of encounter must be verified for each exposed organism by using a dedicated homogenization system (e.g., water current in a kreisel-like incubator or rotation of incubated bottles) and particle counting (using flow cytometry for instance) throughout the entire tanks to ensure homogeneous distribution. Bathing makes it possible to study a broad range of external and/or internal MP effects on zooplankton, fish, and shellfish (Wright et al., 2013b) by both contact (particularly via particle adherence to the carapace/skin/ectoderm, feeding and swimming appendages/tentacles, and gills) and ingestion and its potential effects (e.g., clogging or accumulation in the digestive tract; Bergami et al., 2016). In such assays, it is logical to use either buoyant MP, which are tested free in presence of live prey (microalgae, Artemia, copepods) or feed pellets. In zooplankton studies, ingestion rates are often derived from gut content (i.e., a snapshot of the number of particles eaten at a given time) or from analysis of microplastic-laden fecal pellets. Karakolis et al. (2018) recently highlighted a number of biases linked to gut content and fecal pellet analyses of MP, encouraging the use of digestible coatings (protein/fluorophore) for accurate estimation of MP intake. Biased estimates of MP intake can originate from MP that pass through the digestive tract without being emitted as fecal pellets, or that undergo digestive fragmentation as NP (Dawson et al., 2018); the same holds for MP that adhere to organisms or fecal pellets and that could be erroneously taken into account though not ingested. Finally, operator-dependent errors in counts and measures can strongly limit experimental efficacy. MP coating is an innovative method that needs to be investigated in future experiments, regarding possible combination of coating/fluorophore, types of plastics and experimental conditions.
While most zooplankton are too small to develop true vision (i.e., the capability to form an image) and rely on chemosensing/mechanosensing to detect prey, predatory organisms like fish could confuse suspended MP with potential food/prey, although particle color should be considered since it may modulate the capacity of predator fish to discriminate them from food or preys (Carlos De Sá et al., 2015).
To specifically study the effects of MP ingestion by fish, trophic transfer experiments should be implemented either with artificial feeds or live food. Live fish preys containing MP could be obtained with several mesozooplanktonic organisms (>200 μm) depending on targeted fish species or stages. Setälä et al. (2014) described a simple procedure incubating MP with live preys in bottles placed on a plankton wheel (1 rpm). Importantly, the incubation time that allows the maximal incorporation of MP into the live preys needs to be determined for each species-prey-plastic combination tested by scoring the number of ingested MP by microscopic observation. MP-contaminated live preys could then be given to fish larvae or adults according to their usual feeding protocol. MP could be incorporated into aquafeed pellets, by manual insertion under the microscope as described by Grigorakis et al. (2017) in goldfish, in order to ensure that fish would ingest a precise amount of MP. Such a strategy is very useful for learning about gut retention specificities according to plastic types and shapes. However, it may not be applicable to long-term experiments as the preparation procedure of such aquafeed is time-consuming and may not be realistically manageable. An easier strategy is to incorporate MP at known ratio within aquafeed pellets by aqueous mixing with feed pellets or ingredients (Mazurais et al., 2015; Pedà et al., 2016); then, after gentle air-drying, the feeds can be easily sieved to obtain a precise size range depending on the fish species studied.
For filter/suspension feeders, the way in which MP-NP may interact with phytoplankton is expected to have consequences for MP-NP trophic transfer. MP are likely to form hetero-aggregates when incubated in natural seawater and could possibly modify ingestion by filter/suspension feeders in both natural environment and experimental conditions (Long et al., 2015; Vroom et al., 2017; section Biofouling and Hetero-Aggregation). Therefore, integrating trophic pathway is of great interest to assess particle ingestion and toxicity in laboratory exposures handling filter/suspension feeders. Green (2016) reduced the buoyancy of neutral MP (high density polyethylene and polylactic acid) by mixing them with cultures of Isochrysis galbana 3 days prior to exposure in order to make them bioavailable to the flat oyster Ostrea edulis. Although such studies remain scarce, they highlight the role of phytoplankton as a potential vector for MP-NP trophic transfer in marine food webs via a more realistic expected scenario.
The post-ingestion process generally ends with microplastic-laden fecal pellets exhibiting reduced sinking rates compared to those derived from a natural food diet, particularly when loaded with PS particles (e.g., Cole et al., 2016). Regarding zooplankton grazers, particularly copepods, coprophagy of MP, i.e., ingestion of entire fecal pellets loaded with MP can lead to a second MP recycling that could induce additional impacts on organisms. Both coprorexhy (fecal pellet fragmentation into smaller pieces) and coprochaly (disruption of the fecal pellet peritrophic membrane) will cause a partial dispersal of the fecal pellet content and particularly in the release of MP into the incubation medium. While working with acknowledged coprophagous/coprochalous copepod species (e.g., Calanus helgolandicus, Oithona similis, Acartia tonsa, and Temora longicornis), incubation should be shortened to avoid fecal pellet production and secondary exposure to MP. Otherwise, primary or secondary (i.e., via fecal pellets) ingestion of MP and their impacts will remain difficult to distinguish. In any case, for exposure experiments using either large (fish, shellfish or jellyfish) or small (zooplankton or shellfish larval stages) water volumes, collection of non-ingested food and feces on a 1-μm filter is absolutely essential for all experimental MP exposures in order to prevent any dissemination of microbeads in waste water effluents (Mazurais et al., 2015; Paul-Pont et al., 2016; Sussarellu et al., 2016). Peer reviewers must request an explanation of the treatment procedures used for experimental water effluents. For NP, it is still technically difficult to prevent particle escape with experimental outflow, making it impossible to carry out experiments with running seawater and therefore producing a large water volume to treat. Until the necessary technology to prevent accidental release becomes available, NP exposures should be restricted to small water volumes that can be treated (burned) by specialized companies.
Evidence and Uncertainties on the Translocation of Microplastics in Marine Organisms
Although it has often been reported that spherical MP particles are rapidly egested (e.g., Mazurais et al., 2015; Cole et al., 2016), the possibility that small microspheres or microparticles of irregular shape could be transferred into tissues of marine organisms other than the digestive tract and then through the food web to humans (Wright et al., 2013b; Setälä et al., 2014; Grigorakis et al., 2017) raises some concerns. When organisms are exposed to micro- or nanoparticles, some of these could pass through the epithelia and enter the circulatory system and sometimes the tissues. This phenomenon is called “translocation.” Two entry routes are possible for aquatic organisms: (i) by passive diffusion through the epithelia in direct contact with the external environment (skin, gills or mantle) during water filtration or respiration processes; and/or (ii) by transfer through the digestive epithelium after ingestion. Once epithelial barriers have been passed, particles may be distributed to other tissues via the circulatory system, and potentially pass through cell membranes, including the nuclear membrane. Translocation efficiency primarily depends on particle size, but also on shape, nature, charge, concentration, and the organism concerned (Gratton et al., 2008; Lunov et al., 2011; Bannunah et al., 2014). In recent years, several studies have focused on or discussed the translocation of plastic microparticles within marine organisms (Supplementary file 1), and this remains a topical issue. Observation of translocation is very challenging, and the route of MP entry is not yet identified. There are key points that need to be taken into consideration in studies aiming to demonstrate translocation in marine organisms. Below, we show by means of examples the strengths and weaknesses of the different techniques used to date in both experimental and field studies to accurately assess translocation.
Strengths and Weaknesses of Protocols Designed to Study Translocation
The main techniques used up to now to study translocation have been microscopy to visualize MP within tissues and flow cytometry for circulating fluids. Upon exposure of bivalves to microplastics, it is likely that MP can enter the pallial cavity and adhere to the mantle or gills and possibly embedded in mucus; This possibility is one of the conclusion of Kolandhasamy et al. (2018) working on experimental exposure of mussels to 0.05-5 mm MP: “adherence rather than ingestion led to the accumulation of microplastics in those organs (foot, mantle) which are not involved in ingestion.” Considering that bivalve hemolymph is sampled by suction, with a depression effect in the adductor muscle through the pallial cavity and the body, the possibility of hemolymph contamination during sampling could not be eliminated. Furthermore, translocation could not be demonstrated by histological analysis on transversal sections of eight oysters after 2, 5 or 8 weeks of exposure, demonstrating that “micro-PS particles were only detected in the stomach and intestine” (Sussarellu et al., 2016). Based on these observations, it seems important to improve protocols aiming to demonstrate translocation of plastic particles in marine organisms.
One of the first and the most frequently cited publications addressing translocation in marine invertebrates is Browne et al. (2008). These authors used the same hemolymph sampling method as described above, being particularly careful to avoid contamination as “shell water was drained from each mussel prior to hemolymph extraction.” Nevertheless, the possibility of contamination during sampling cannot be totally eliminated by flow cytometry analysis alone and histological evidence of the presence of microplastics in the non-digestive tissues would help to exclude artefactual contamination. This is why histology and fluorescent microscopy are among the most frequently used techniques in studies on translocation (Supplementary file 1), although strict experimental and analytical protocols must again be used. Darmody et al. (2015) used epifluorescence microscopy to follow the fate of fluorescent styrene-maleic acid (SMA) microbeads (1–2 μm in size) encapsulated in alginate upon ingestion by oysters (Ostrea edulis). However, the microscope features (emission and excitation filters) were not compatible with one of the fluorescent probes used, and no histological sections of control oysters were reported, even though autofluorescence of bivalve tissue is a well-known phenomenon (Heaney et al., 2011). When exposed to 0.05 μm beads, the copepod Paraoithona nana exhibited “fluorescence dispersed throughout the body”, which differed from specimens exposed to 0.5 and 6 μm beads, where fluorescence was “mostly limited to the digestive organs.” Jeong et al. (2017) stated that this fluorescence pattern “could be explained by translocation of polystyrene microbeads across the cellular membranes through the digestive organs of P. nana.” although this could not be clearly verified. Reporting the presence of MP inside specific organs, such as the liver as in Collard et al. (2017), should also be avoided when the same study also makes a statement such as the following “it was unfortunately not possible to precisely localize MPs in the liver because of the conservation and the cryosections preparation which altered the tissue structure.”
Conclusive dedicated techniques would be an asset to reveal translocation phenomena. For instance, an in vitro approach applying Ussing chambers should be considered to accurately show transepithelial transport of MP/NP and potential associated effects on passive or active flux across the enterocyte membranes (Hamilton, 2011; Herrmann and Turner, 2016). This would provide proof of translocation and information on the underlying mechanisms. Histology also appears to be one of the most suitable techniques, provided that there are appropriate controls. The collection of samples must be done very carefully, following strict rules to prevent contamination. Among these, the flesh should be rinsed before dissection, as in Browne et al. (2008), to limit the risk of contamination by MP located outside the tissues. A control comprising tissues of unexposed individuals should also be systematically included for epifluorescence microscopy to take into account tissue and/or non-plastic particle autofluorescence. Finally, cryohistology should be used rather than classic histology as solvents and paraffin embedment may impair MP integrity within tissues and their Raman signal when using a micro-Raman on histological slides.
How Does Particle Size Influence Translocation?
Various sizes of microplastic particles (from 0.5 to 280 μm, Supplementary file 1) have been tested in translocation experiments on multiple marine models. The routes of entry differ according to size and thus between MP and NP. The probability of translocation is considered much higher for NP than for MP. Nanoparticles can enter any tissue by endocytosis, phagocytosis (for aggregates) or passive membrane movement (Gustafson et al., 2015). Silver nanoparticles (5–20 mm) detected using transmission electron microscopy (TEM) and electron-dispersive x-ray analysis (EDS), translocated into brain, heart, yolk, and blood of Zebrafish embryos exposed for 72 h to 5–100 μg mL−1, mainly by endocytosis (Asharani et al., 2008). For plastics, translocation has been shown in fish for nanoparticles of polystyrene (39.4, 53, 180 nm) (Kashiwada, 2006; Mattsson et al., 2017; Supplementary file 1). Regarding MP, Lusher et al. indicate that “Microplastics larger than this (0.5 mm) do not readily pass through the gut wall without pre-existing damage, and the likelihood of translocation into tissues is too low to warrant regular investigation” (Lusher et al., 2017).
It is likely that MP < 10 μm are compatible with passage through an epithelium since, for example, bivalve hemocytes (about 5 μm) are known to cross the digestive epithelia (Haberkorn et al., 2010; Rolton et al., 2016) using membrane surface recognition elements. Plastic particles would not be recognized by biological systems and their passage would therefore probably be passive or use non-selective transporters and depend on the presence and nature of their eco- or biocorona (Galloway et al., 2017). Such coating mechanisms involving proteins and biomolecules was previously suggested to occur at the surface of nanomaterials in biological fluids, thus influencing their interaction with cells and tissues (Monopoli et al., 2012). The most detailed studies performed are those on mammals, for which the uptake of diverse types of inert microparticles mainly occurs in the digestive track through normal enterocytes and specific M-cells of Peyer's patches (Pappo and Ermak, 1989; Hussain et al., 2001). To the best of our knowledge, however, such structures have not yet been found in fish, shellfish or zooplankton.
Future challenges will be to characterize entry mechanisms of MP/NP at the cellular and molecular levels, the largest size of particles able to translocate, and possible effects of form, shape, corona and biofouling. More specifically, three set of actions are required: (i) designing protocols specifically dedicated to translocation studies and preventing contamination; (ii) studying the detailed mechanisms of MP/NP entry through the different epithelia using suitable or innovative approaches and methods (Ussing chambers; electron microscopy; MP radiotracing using isotope-coating technology; Oberhänsli et al., 2017); and (iii) quantifying this phenomenon. Afterwards, to estimate the specific physiological consequences of translocation, it is crucial to assess whether the translocation is “limited” to the circulatory system, whether and how MP/NP interact with biological membranes, and if they can penetrate into cells within which interactions are possible (mitochondria, DNA) inducing stress responses such as ROS production and/or apoptosis.
Is there an Ideal Way to Experimentally Study the Effects of MP on Marine Organisms?
Ecotoxicology is defined as “the study of harmful effects of chemicals upon ecosystems, which includes effects on individuals and consequent effects at the levels of population and above” (Walker et al., 2012). One of its first aims is to inform the public (scientists, policy makers, and citizens) on the potential hazards associated with a given contaminant, and how best to protect our environment. As regulatory decisions regarding a given contaminant rely partly on data from the ecotoxicology literature, it is our responsibility to provide high quality data. Recently, a set of recommendations were proposed to design, conduct, analyze and report ecotoxicological studies in the most detailed and transparent manner possible in order to improve their relevance, reproducibility and value (Hanson et al., 2017; Tincani et al., 2017). These baseline expectations can be fully applied to microplastic studies and concern (i) the characterization of the contaminant and actual exposure in experimental conditions/units, (ii) the experimental design and conditions (replication, randomization), (iii) the characteristics and relevance of test organisms, (iv) the adequate interpretation of the endpoints, (v) the quality of data reporting, (vi) the robustness of statistical analyses, and (vii) the availability of the raw data. Given the variety of microplastic types and mixtures in marine environments, special considerations must be made to properly assess the complexity of MP/NP in natural ecosystems (as detailed in sections Which Particles Should Be Used in Laboratory Experiments? and Weathering Implications), and to choose the most relevant MP and exposure route according to the species habitat and mode of nutrition (as detailed in section Achieving Reproducible and Environmentally Relevant Exposure of Marine Organisms). Another step forward would be the integration of the complex interactions occurring between species in a given ecosystem and how this may influence MP exposure, availability and toxicity, including ingestion, fate in organisms and effects. As field evidence of contaminant-specific adverse effects is almost always impossible to obtain in marine science due to the large open-scale characteristics of marine ecosystems, high variation of physicochemical properties of seawater and co-occurrence of contaminants in impacted areas; mesocosm studies may offer a solution. Indeed, as mesocosms combine the control possible in laboratory experiments with some of the complexity of natural ecosystems, they are a relevant research direction for this field (Sagarin et al., 2016). Long-term exposures in small mesocosms with several sizes of MP (PLA: 0.6–363 μm; HDPE: 0.48–316 μm) showed the destabilization of ecosystem equilibrium, modification of respiration/filtration rates of bivalves, species richness, and offspring recruitment (Green, 2016; Green et al., 2016). However, while mesocosms approach the complexity of environmental scenarios, the understanding of observed effects occurring at different ecological integration levels (molecule, cell, organ, organism, community) calls for multidisciplinary approaches combining ecophysiology, cellular aspects, -omics, and modeling.
“Integrative Biology” concerns levels of integration of life into its environment and integrates the functional and comparative analysis of genomes. It largely originates from the rapid development of new technologies, from genomics and genome sequencing to functional analysis for gene, protein and metabolite networks (e.g., transcriptomics, proteomics, and metabolomics). Based on molecular biology or genomics methods, physiology has gained in precision and has also considerably increased its ability to comprehensively capture functional assemblages for both individual and communities levels and the finest anatomical and cellular elements including when these are disrupted by natural and anthropogenic factors. Since MP and NP may have a wide range of effects on marine organisms depending on their type, shape, size, and the nature of additives, pollutants, and microorganisms they carry, these high-throughput techniques seem particularly well suited to studying the physiological processes and metabolic pathways impaired by complex MP/NP contaminations in mesocosms and laboratory experiments. They can usefully be associated with life-trait endpoints to understand the overall response of organisms, as mentioned for other areas of ecotoxicology (Garcia-Reyero and Perkins, 2011; Jager et al., 2013). Genomic-based endpoints may be more powerful for detecting effects and the presence of stressors such as endocrine disruptors, which are often present below the detection or quantification limits of chemical analysis methods commonly used (GC-MS/MS). Compared to chemical quantification approaches, bioassays are advantageous as they can respond to undetectable trace contaminants and integrate the biological effects of all compounds present, taking into account factors such as bioavailability, synergism, or antagonism. Bio-assays coupled with bio-marker analyses are sometimes more powerful than chemical quantification methods for assessing estrogenicity (Kiyama and Wada-Kiyama, 2015). Finally, bio-energetic modeling such as the Dynamic Energy Budget (DEB) theory (Kooijman, 2010) offers a way to describe how an organism acquires and uses energy for physiological functions, maintenance, growth, maturation and migration, in addition to how physiological performance is influenced by environmental variables (Nisbet et al., 2012). The strength of modeling is to help the design of experimental procedures, testing initial hypotheses or, on the contrary, providing explanatory hypotheses to the observed data (e.g., Sussarellu et al., 2016).
Conclusion
Many challenges remain to be overcome to adequately address concerns about MP/NP toxicity to marine organisms and ecosystems so as to provide stakeholders with the necessary data to limit the impact of the microplastic legacy. Laboratory exposure experiments must be carefully designed to cover the wide range of MP/NP contaminations in marine environments, and multidisciplinary approaches involving physics, chemistry and biology appear more than ever essential in this field of research (Figure 7). Because it is not possible to reasonably address all aspects of MP/NP forms, concentrations, mixtures and chemical and biological characteristics at once in each experiment, the scientific question and objectives behind each set of experiments must be clearly defined in order to adequately prioritize the features of MP/NP that potentially account for confounding effects on the endpoints of exposure evaluations. Even if realistic experiments considering ecosystem scenarios are called for decision support, fundamental studies unraveling origin(s) of MP/NP toxicity remain unavoidable as a large body of basic information is missing. A single study cannot be expected to provide all the answers and effectively capture the synergies and antagonisms of contaminants in marine ecosystems. It will be desirable to compile evidences from multiple sources; the more rigorous studies are, the more relevant a meta-analysis will be to quantitatively assess risks of MP/NP in environments.
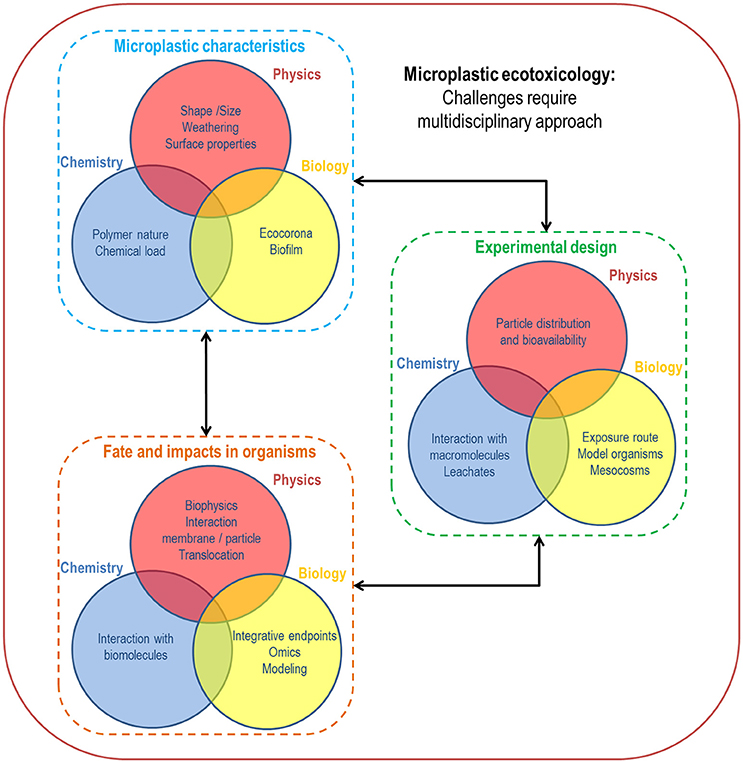
Figure 7. Main challenges in microplastic ecotoxicology requiring multidisciplinary approaches including physics, chemistry and biology.
Author Contributions
All authors listed have made a substantial, direct and intellectual contribution to the work, and approved it for publication.
Conflict of Interest Statement
The authors declare that the research was conducted in the absence of any commercial or financial relationships that could be construed as a potential conflict of interest.
Acknowledgments
This work was supported by the ANR CESA (ANR-15-CE34-0006-02, NANOPLASTICS project) and by the Fonds Unique Interministériel (French government funding) and the local communities (CR Bretagne, CR PACA, CD29, CATPM, and Brest Metropole) as part of the MICROPLASTIC2 project. We acknowledge M. Alumno-Bruscia and S. Pouvreau for helpful discussions and H. McCombie for her help in editing the English. KT was funded by a French doctoral research grant from Ifremer (50%) and Region Bretagne (50%). CG-F was supported by the SAD INMEMO 9245 regional project related to the Sustainable attractiveness strategy of Brittany (France).
Supplementary Material
The Supplementary Material for this article can be found online at: https://www.frontiersin.org/articles/10.3389/fmars.2018.00252/full#supplementary-material
Footnotes
1. ^Litterbase. Distribution of litter types in different realms. Http://litterbase.awi.de/litter. Accessed January 7,2018
References
Afshinnia, K., Marrone, B., and Baalousha, M. (2018). Potential impact of natural organic ligands on the colloidal stability of silver nanoparticles. Sci. Total Environ. 625, 1518–1526. doi: 10.1016/j.scitotenv.2017.12.299
Akhbarizadeh, R., Moore, F., and Keshavarzi, B. (2018). Investigating a probable relationship between microplastics and potentially toxic elements in fish muscles from northeast of Persian Gulf. Environ. Pollut. 232, 154–163. doi: 10.1016/j.envpol.2017.09.028
Amaral-Zettler, L. A., Zettler, E. R., Slikas, B., Boyd, G. D., Melvin, D. W., Morrall, C. E., et al. (2015). The biogeography of the Plastisphere: implications for policy. Front. Ecol. Environ. 13, 541–546. doi: 10.1890/150017
Andrady, A. L. (1990). Weathering of polyethylene (LDPE) and enhanced photodegradable polyethylene in the marine environment. J. Appl. Polym. Sci. 39, 363–370. doi: 10.1002/app.1990.070390213
Antunes, J. C., Frias, J. G. L., Micaelo, A. C., and Sobral, P. (2013). Resin pellets from beaches of the Portuguese coast and adsorbed persistent organic pollutants. Estuar. Coast. Shelf. S. 130, 62–69. doi: 10.1016/j.ecss.2013.06.016
Asharani, P. V., Lian Wu, Y., Gong, Z., and Valiyaveettil, S. (2008). Toxicity of silver nanoparticles in zebrafish models. Nanotoxicology 19:255102. doi: 10.1088/0957-4484/19/25/255102
Au, S. Y., Bruce, T. F., Bridges, W. C., and Klaine, S. J. (2015). Responses of Hyalella azteca to acute and chronic microplastic exposures. Environ. Toxicol. Chem. 34, 2564–2572. doi: 10.1002/etc.3093
Avio, C. G., Gorbi, S., Milan, M., Benedetti, M., Fattorini, D., D'Errico, G., et al. (2015). Pollutants bioavailability and toxicological risk from microplastics to marine mussels. Environ. Pollut. 198, 211–222. doi: 10.1016/j.envpol.2014.12.021
Bakir, A., O'Connor, I. A., Rowland, S. J., Hendriks, A. J., and Thompson, R. C. (2016). Relative importance of microplastics as a pathway for the transfer of hydrophobic organic chemicals to marine life. Environ. Pollut. 219, 56–65. doi: 10.1016/j.envpol.2016.09.046
Bakir, A., Rowland, S. J., and Thompson, R. C. (2014). Enhanced desorption of persistent organic pollutants from microplastics under simulated physiological conditions. Environ. Pollut. 185, 16–23. doi: 10.1016/j.envpol.2013.10.007
Balbi, T., Camisassi, G., Montagna, M., Fabbri, R., Franzellitti, S., Carbone, C., et al. (2017). Impact of cationic polystyrene nanoparticles (PS-NH2) on early embryo development of Mytilus galloprovincialis: effects on shell formation. Chemosphere 186, 1–9. doi: 10.1016/j.chemosphere.2017.07.120
Baldwin, A. K., Corsi, S. R., and Mason, S. A. (2016). Plastic debris in 29 Great Lakes tributaries: relations to watershed attributes and hydrology. Envir. Sci. Technol. 50, 10377–10385. doi: 10.1021/acs.est.6b02917
Bannunah, A. M., Vllasaliu, D., Lord, J., and Stolnik, S. (2014). Mechanisms of nanoparticle internalization and transport across an intestinal epithelial cell model: effect of size and surface charge. Mol. Pharm. 11, 4363–4373. doi: 10.1021/mp500439c
Barnes, D. K., Galgani, F., Thompson, R. C., and Barlaz, M. (2009). Accumulation and fragmentation of plastic debris in global environments. Philos. T. Roy. Soc. B. 364, 1985–1998. doi: 10.1098/rstb.2008.0205
Bergami, E., Bocci, E., Vannuccini, M. L., Monopoli, M., Salvati, A., Dawson, K. A., et al. (2016). Nano-sized polystyrene affects feeding, behavior and physiology of brine shrimp Artemia franciscana larvae. Ecotox. Environ. Sage. 123, 18–25. doi: 10.1016/j.ecoenv.2015.09.021
Bergami, E., Pugnalini, S., Vannuccini, M. L., Manfra, L., Faleri, C., Savorelli, F., et al. (2017). Long-term toxicity of surface-charged polystyrene nanoplastics to marine planktonic species Dunaliella tertiolecta and Artemia franciscana. Aquat. Toxicol. 189, 159–169. doi: 10.1016/j.aquatox.2017.06.008
Bergmann, M., Wirzberger, V., Krumpen, T., Lorenz, C., Primpke, S., Tekman, M. B., et al. (2017). High quantities of microplastic in Arctic deep-sea sediments from the HAUSGARTEN Observatory. Envir. Sci. Technol. 51, 11000–11010. doi: 10.1021/acs.est.7b03331
Besseling, E., Foekema, E. M., van den Heuvel-Greve, M. J., and Koelmans, A. A. (2017). The Effect of microplastic on the uptake of chemicals by the lugworm Arenicola marina (L.) under environmentally relevant exposure conditions. Envir. Sci. Technol. 51, 8795–8804. doi: 10.1021/acs.est.7b02286
Besseling, E., Wang, B., Lürling, M., and Koelmans, A. A. (2014a). Nanoplastic affects growth of S. Obliquus and reproduction of D. Magna. Envir. Sci. Technol. 48, 12336–12343. doi: 10.1021/es503001d
Besseling, E., Wang, B., Lürling, M., and Koelmans, A. A (2014b) Correction to: Nanoplastic affects growth of S. obliquus and reproduction of D. magna. Environ. Sci. Technol. 48, 12336–12343.
Besseling, E., Wegner, A., Foekema, E. M., Van Den Heuvel-Greve, M. J., and Koelmans, A. A. (2013). Effects of microplastic on fitness and PCB bioaccumulation by the lugworm Arenicola marina (L.). Envir. Sci. Technol. 47, 593–600. doi: 10.1021/es302763x
Bhattacharya, P., Lin, S., Turner, J. P., and Ke, P. C. (2010). Physical adsorption of charged plastic nanoparticles affects algal photosynthesis. J. Phys. Chem. C. 114, 16556–16561. doi: 10.1021/jp1054759
Boerger, C. M., Lattin, G. L., Moore, S. L., and Moore, C. J. (2010). Plastic ingestion by planktivorous fishes in the North Pacific Central Gyre. Mar. Pollut. Bull. 60, 2275–2278. doi: 10.1016/j.marpolbul.2010.08.007
Bråte, I. L., Blazquez, M., Brooks, S. J., and Thomas, K. V. (2018). Weathering impacts the uptake of polyethylene microplastics from toothpaste in Mediterranean mussels (M. galloprovincialis). Sci. Total. Environ. 626, 1310–1318. doi: 10.1016/j.scitotenv.2018.01.141
Brennecke, D., Ferreira, E. C., Costa, T. M., Appel, D., da Gama, B. A., and Lenz, M. (2015). Ingested microplastics (> 100 μm) are translocated to organs of the tropical fiddler crab Uca rapax. Mar. Pollut. Bull. 96, 491–495. doi: 10.1016/j.marpolbul.2015.05.001
Browne, M. A., Dissanayake, A., Galloway, T. S., Lowe, D. M., and Thompson, R. C. (2008). Ingested microscopic plastic translocates to the circulatory system of the mussel, Mytilus edulis (L.). Envir. Sci. Technol. 42, 5026–5031. doi: 10.1021/es800249a
Browne, M. A., Galloway, T. S., and Thompson, R. C. (2010). Spatial patterns of plastic debris along estuarine shorelines. Envir. Sci. Technol. 44, 3404–3409. doi: 10.1021/es903784e
Browne, M. A., Niven, S. J., Galloway, T. S., Rowland, S. J., and Thompson, R. C. (2013). Microplastic moves pollutants and additives to worms, reducing functions linked to health and biodiversity. Curr. Biol. 23, 2388–2392. doi: 10.1016/j.cub.2013.10.012
Bruck, S., and Ford, A. T. (2018). Chronic ingestion of polystyrene microparticles in low doses has no effect on food consumption and growth to the intertidal amphipod Echinogammarus marinus? Environ. Pollut. 233, 1125–1130. doi: 10.1016/j.envpol.2017.10.015
Cai, L., Wang, J., Peng, J., Wu, Z., and Tan, X. (2018). Observation of the degradation of three types of plastic pellets exposed to UV irradiation in three different environments. Sci. Total. Environ. 628, 740–747. doi: 10.1016/j.scitotenv.2018.02.079
Carlos de Sá, L., Luís, L. G., and Guilhermino, L. (2015). Effects of microplastics on juveniles of the common goby (Pomatoschistus microps): confusion with prey, reduction of the predatory performance and efficiency, and possible influence of developmental conditions. Environ. Pollut. 196, 359–362. doi: 10.1016/j.envpol.2014.10.026
Campos, B., Rivetti, C., Rosenkranz, P., Navas, J. M., and Barata, C. (2013). Effects of nanoparticles of TiO2 on food depletion and life-history responses of Daphnia magna. Aquat. Toxicol. 130, 174–183. doi: 10.1016/j.aquatox.2013.01.005
Canesi, L., and Corsi, I. (2016). Effects of nanomaterials on marine invertebrates. Sci. Total. Environ. 565, 933–940. doi: 10.1016/j.scitotenv.2016.01.085
Casado, M. P., Macken, A., and Byrne, H. J. (2013). Ecotoxicological assessment of silica and polystyrene nanoparticles assessed by a multitrophic test battery. Environ. Int. 51, 97–105. doi: 10.1016/j.envint.2012.11.001
Cassone, A.-L., Lambert, C., Miner, P., Hegaret, H., Fabioux, C., Le Goïc, N., et al. (2014). “First experimental assay to demonstrate microplastics as possible vectors of pathogenic Vibrio on oysters Crassostrea gigas,” in Poster at the Physiomar Meeting (La Serena).
Castillo, A. B., Al-Maslamani, I., and Obbard, J. P. (2016). Prevalence of microplastics in the marine waters of Qatar. Mar. Pollut. Bull. 111, 260–267. doi: 10.1016/j.marpolbul.2016.06.108
Claessens, M., De Meester, S., Van Landuyt, L., De Clerck, K., and Janssen, C. R. (2011). Occurrence and distribution of microplastics in marine sediments along the Belgian coast. Mar. Pollut. Bull. 62, 2199–2204. doi: 10.1016/j.marpolbul.2011.06.030
Cole, M. (2016). A novel method for preparing microplastic fibers. Sci. Rep. 6:34519. doi: 10.1038/srep34519
Cole, M., and Galloway, T. S. (2015). Ingestion of nanoplastics and microplastics by Pacific oyster larvae. Envir. Sci. Technol. 49, 14625–146320. doi: 10.1021/acs.est.5b04099
Cole, M., Lindeque, P., Fileman, E., Halsband, C., Goodhead, R., Moger, J., et al. (2013). Microplastic ingestion by zooplankton. Envir. Sci. Technol. 47, 6646–6655. doi: 10.1021/es400663f
Cole, M., Lindeque, P. K., Fileman, E., Clark, J., Lewis, C., Halsband, C., et al. (2016). Microplastics alter the properties and sinking rates of zooplankton faecal pellets. Envir. Sci. Technol. 50, 3239–3246. doi: 10.1021/acs.est.5b05905
Collard, F., Gilbert, B., Compère, P., Eppe, G., Das, K., Jauniaux, T., et al. (2017). Microplastics in livers of European anchovies (Engraulis encrasicolus, L.). Environ. Pollut. 229, 1000–1005. doi: 10.1016/j.envpol.2017.07.089
Collignon, A., Hecq, J. H., Galgani, F., Voisin, P., Collard, F., and Goffart, A. (2012). Neustonic microplastic and zooplankton in the North Western Mediterranean Sea. Mar. Pollut. Bull. 64, 861–864. doi: 10.1016/j.marpolbul.2012.01.011
Costa, M. F., Do Sul, J. A. I., Silva-Cavalcanti, J. S., Araújo, M. C. B., Spengler, Â., and Tourinho, P. S. (2010). On the importance of size of plastic fragments and pellets on the strandline: a snapshot of a Brazilian beach. Environ. Monit. Assess. 168, 299–304. doi: 10.1007/s10661-009-1113-4
Cózar, A., Echevarría, F., González-Gordillo, J. I., Irigoien, X., Úbeda, B., Hernández-León, S., et al. (2014). Plastic debris in the open ocean. Proc. Natl. Acad. Sci. U.S.A. 111, 10239–10244. doi: 10.1073/pnas.1314705111
Cózar, A., Martí, E., Duarte, C. M., García-de-Lomas, J., van Sebille, E., Ballatore, T. J., et al. (2017). The Arctic Ocean as a dead end for floating plastics in the North Atlantic branch of the Thermohaline Circulation. Sci. Adv. 3:e1600582. doi: 10.1126/sciadv.1600582
Darmody, G., Maloy, A. P., Lynch, S. A., Prado-Alvarez, M., Cotterill, J., Wontner-Smith, T., et al. (2015). Tissue targeting of the European flat oyster, Ostrea edulis, using microencapsulated microbeads as a biological proxy. Aquacult. Int. 23, 647–659. doi: 10.1007/s10499-014-9842-y
Datta, M. S., Sliwerska, E., Gore, J., Polz, M. F., and Cordero, O. X. (2016). Microbial interactions lead to rapid micro-scale successions on model marine particles. Nat. Commun. 7:11965. doi: 10.1038/ncomms11965
Davarpanah, E., and Guilhermino, L. (2015). Single and combined effects of microplastics and copper on the population growth of the marine microalgae Tetraselmis chuii. Estuar. Coast. Shelf. S. 167, 269–275. doi: 10.1016/j.ecss.2015.07.023
Dawson, A. L., Kawaguchi, S., King, C. K., Townsend, K. A., King, R., Huston, W. M., et al. (2018). Turning microplastics into nanoplastics through digestive fragmentation by Antarctic krill. Nat. Commun. 9:1001. doi: 10.1038/s41467-018-03465-9
Della Torre, C., Bergami, E., Salvati, A., Faleri, C., Cirino, P., Dawson, K. A., et al. (2014). Accumulation and embryotoxicity of polystyrene nanoparticles at early stage of development of sea urchin embryos Paracentrotus lividus. Envir. Sci. Technol. 48, 12302–12311. doi: 10.1021/es502569w
Derjaguin, B. V. (1941). Theory of the stability of strongly charged lyophobic sols and the adhesion of strongly charged particles in solutions of electrolytes. Acta. Physiochim. URS. 14, 633–662.
Desforges, J. P., Galbraith, M., Dangerfield, N., and Ross, P. S. (2014). Widespread distribution of microplastics in subsurface seawater in the NE Pacific Ocean. Mar. Pollut. Bull. 79, 94–99. doi: 10.1016/j.marpolbul.2013.12.035
De Tender, C. A., Devriese, L. I., Haegeman, A., Maes, S., Ruttink, T., and Dawyndt, P. (2015). Bacterial community profiling of plastic litter in the Belgian part of the North Sea. Envir. Sci. Technol. 49, 9629–9638. doi: 10.1021/acs.est.5b01093
Devriese, L. I., van der Meulen, M. D., Maes, T., Bekaert, K., Paul-Pont, I., Frère, L., et al. (2015). Microplastic contamination in brown shrimp (Crangon crangon, Linnaeus 1758) from coastal waters of the Southern North Sea and Channel area. Mar. Pollut. Bull. 98, 179–187. doi: 10.1016/j.marpolbul.2015.06.051
De Witte, B., Devriese, L., Bekaert, K., Hoffman, S., Vandermeersch, G., Cooreman, K., et al. (2014). Quality assessment of the blue mussel (Mytilus edulis): comparison between commercial and wild types. Mar. Pollut. Bull. 85, 146–155. doi: 10.1016/j.marpolbul.2014.06.006
Di, M., and Wang, J. (2018). Microplastics in surface waters and sediments of the Three Gorges Reservoir, China. Sci. Total. Environ. 616, 1620–1627. doi: 10.1016/j.scitotenv.2017.10.150
Doyle, M. J., Watson, W., Bowlin, N. M., and Sheavly, S. B. (2011). Plastic particles in coastal pelagic ecosystems of the Northeast Pacific ocean. Mar. Environ. Res. 71, 41–52. doi: 10.1016/j.marenvres.2010.10.001
Dubaish, F., and Liebezeit, G. (2013). Suspended microplastics and black carbon particles in the Jade system, southern North Sea. Water. Air. Soil. Poll. 224, 1352. doi: 10.1007/s11270-012-1352-9
Enders, K., Lenz, R., Stedmon, C. A., and Nielsen, T. G. (2015). Abundance, size and polymer composition of marine microplastics ≥ 10μm in the Atlantic Ocean and their modelled vertical distribution. Mar. Pollut. Bull. 100, 70–81. doi: 10.1016/j.marpolbul.2015.09.027
Eriksen, M., Maximenko, N., Thiel, M., Cummins, A., Lattin, G., Wilson, S., et al. (2013). Plastic pollution in the South Pacific subtropical gyre. Mar. Pollut. Bull. 68, 71–76. doi: 10.1016/j.marpolbul.2012.12.021
Erni-Cassola, G., Gibson, M. I., Thompson, R. C., and Christie-Oleza, J. A. (2017). Lost, but found with Nile Red: a novel method for detecting and quantifying small microplastics (1 mm to 20 μm) in environmental samples. Envir. Sci. Technol. 51, 13641–13648. doi: 10.1021/acs.est.7b04512
Esiukova, E. (2017). Plastic pollution on the Baltic beaches of Kaliningrad region, Russia. Mar. Pollut. Bull. 114, 1072–1080. doi: 10.1016/j.marpolbul.2016.10.001
Farrell, P., and Nelson, K. (2013). Trophic level transfer of microplastic: Mytilus edulis (L.) To Carcinus maenas (L.). Environ. Pollut. 177, 1–3. doi: 10.1016/j.envpol.2013.01.046
Filella, M. (2015). Questions of size and numbers in environmental research on microplastics: methodological and conceptual aspects. Environ. Chem. 12, 527–538. doi: 10.1071/EN15012
Foekema, E. M., De Gruijter, C., Mergia, M. T., van Franeker, J. A., Murk, A. J., and Koelmans, A. A. (2013). Plastic in North Sea fish. Envir. Sci. Technol. 47, 8818–8824. doi: 10.1021/es400931b
Fossi, M. C., Panti, C., Guerranti, C., Coppola, D., Giannetti, M., Marsili, L., et al. (2012). Are baleen whales exposed to the threat of microplastics? A case study of the Mediterranean fin whale (Balaenoptera physalus). Mar. Pollut. Bull. 64, 2374–2379. doi: 10.1016/j.marpolbul.2012.08.013
Fotopoulou, K. N., and Karapanagioti, H. K. (2015). Surface properties of beached plastics. Environ. Sci. Pollut. R. 22, 11022–11032. doi: 10.1007/s11356-015-4332-y
Foulon, V., Le Roux, F., Lambert, C., Huvet, A., Soudant, P., and Paul-Pont, I. (2016). Colonization of polystyrene microparticles by Vibrio crassostreae : light and electron microscopic investigation. Envir. Sci. Technol. 50, 10988–10996. doi: 10.1021/acs.est.6b02720
Free, C. M., Jensen, O. P., Mason, S. A., Eriksen, M., Williamson, N. J., and Boldgiv, B. (2014). High-levels of microplastic pollution in a large, remote, mountain lake. Mar. Pollut. Bull. 85, 156–163. doi: 10.1016/j.marpolbul.2014.06.001
Frère, L., Paul-Pont, I., Rinnert, E., Petton, S., Jaffré, J., Bihannic, I., et al. (2017). Influence of environmental and anthropogenic factors on the composition, concentration and spatial distribution of microplastics: a case study of the Bay of Brest (Brittany, France). Environ. Pollut. 225, 211–222. doi: 10.1016/j.envpol.2017.03.023
Galloway, T. S., Cole, M., Lewis, C., Atkinson, A., and Allen, J. I. (2017). Interactions of microplastic debris throughout the marine ecosystem. Nat. Ecol. Evol. 1:116. doi: 10.1038/s41559-017-0116
Garcia-Reyero, N., and Perkins, E. J. (2011). Systems biology: leading the revolution in ecotoxicology. Environ. Toxicol. Chem. 30, 265–273. doi: 10.1002/etc.401
GESAMP (2016). “Sources, fate and effects of microplastics in the marine environment: part two of a global assessment,” in IMO/FAO/UNESCO-IOC/UNIDO/WMO/IAEA/UN/UNEP/UNDP Joint Group of Experts on the Scientific Aspects of Marine Environmental Protection, eds P. J. Kershaw and C. M. Rochman (London: Micropress Printers Ltd), 220.
Geyer, R., Jambeck, J. R., and Law, K. L. (2017). Production, use, and fate of all plastics ever made. Sci. Adv. 3:e1700782. doi: 10.1126/sciadv.1700782
Gigault, J., Ter Halle, A., Baudrimont, M., Pascal, P.-Y., Gauffre, F., Phi, T.-L., et al. (2018). Current opinion: what is a nanoplastic? Environ. Polllut. 230, 1030–1034. doi: 10.1016/j.envpol.2018.01.024
Graham, E. R., and Thompson, J. T. (2009). Deposit-and suspension-feeding sea cucumbers (Echinodermata) ingest plastic fragments. J. Exp. Mar. Biol. Ecol. 368, 22–29. doi: 10.1016/j.jembe.2008.09.007
Gratton, S. E., Ropp, P. A., Pohlhaus, P. D., Luft, J. C., Madden, V. J., Napier, M. E., et al. (2008). The effect of particle design on cellular internalization pathways. Proc. Natl. Acad. Sci. U.S.A. 105, 11613–11618. doi: 10.1073/pnas.0801763105
Gray, A. D., and Weinstein, J. E. (2017). Size- and shape-dependent effects of microplastic particles on adult daggerblade grass shrimp (Palaemonetes pugio). Environ. Toxicol. Chem. 36, 3074–3080. doi: 10.1002/etc.3881
Green, D. S. (2016). Effects of microplastics on European flat oysters, Ostrea edulis and their associated benthic communities. Environ. Pollut. 216, 95–103. doi: 10.1016/j.envpol.2016.05.043
Green, D. S., Boots, B., O'Connor, N. E., and Thompson, R. (2016). Microplastics affect the ecological functioning of an important biogenic habitat. Envir. Sci. Technol. 51, 68–77. doi: 10.1021/acs.est.6b04496
Grigorakis, S., Mason, S. A., and Drouillard, K. G. (2017). Determination of the gut retention of plastic microbeads and microfibers in goldfish (Carassius auratus). Chemosphere 169, 233–238. doi: 10.1016/j.chemosphere.2016.11.055
Gustafson, H. H., Holt-Casper, D., Grainger, D. W., and Ghandehari, H. (2015). Nanoparticle uptake: the phagocyte problem. Nano Today, 10, 487–510. doi: 10.1016/j.nantod.2015.06.006
Güven, O., Gökdag, K., Jovanović, B., and Kideyş, A. E. (2017). Microplastic litter composition of the Turkish territorial waters of the Mediterranean Sea, and its occurrence in the gastrointestinal tract of fish. Environ. Pollut. 223, 286–294. doi: 10.1016/j.envpol.2017.01.025
Haberkorn, H., Lambert, C., Le Goïc, N., Moal, J., Suquet, M., Guéguen, M., et al. (2010). Effects of Alexandrium minutum exposure on nutrition-related processes and reproductive output in oysters Crassostrea gigas. Harmful Algae 9, 427–439. doi: 10.1016/j.hal.2010.01.003
Hamilton, K. L. (2011). Ussing's “Little Chamber”: 60 Years+ old and counting. Front. Physiol. 2:6. doi: 10.3389/fphys.2011.00006
Hanson, M. L., Wolff, B. A., Green, J. W., Kivi, M., Panter, G. H., Warne, M. S. J., et al. (2017). How we can make ecotoxicology more valuable to environmental protection. Sci. Total. Environ. 578, 228–235. doi: 10.1016/j.scitotenv.2016.07.160
Harman, C., Thomas, K. V., Tollefsen, K. E., Meier, S., Bøyum, O., and Grung, M. (2009). Monitoring the freely dissolved concentrations of polycyclic aromatic hydrocarbons (PAH) and alkylphenols (AP) around a Norwegian oil platform by holistic passive sampling. Mar. Pollut. Bull. 58, 1671–1679. doi: 10.1016/j.marpolbul.2009.06.022
Heaney, S. A., Maloy, S. P., and Slater, J. W. (2011). Evaluation of fixatives and autofluorescence reduction treatments for marine bivalve larvae. J. Mar. Biol. Assoc. U.K. 91, 1567–1576. doi: 10.1017/S0025315411000208
Hermabessiere, L., Dehaut, A., Paul-Pont, I., Lacroix, C., Jezequel, R., Soudant, P., et al. (2017). Occurrence and effects of plastic additives on marine environments and organisms: a review. Chemosphere 182, 781–793. doi: 10.1016/j.chemosphere.2017.05.096
Herrmann, J. R., and Turner, J. R. (2016). Beyond Ussings chambers: contemporary thoughts on integration of transepithelial transport. Am. J. Physiol-Cell. Ph. 310, C423–C431. doi: 10.1152/ajpcell.00348.2015
Herzke, D., Anker-Nilssen, T., Nøst, T. H., Götsch, A., Christensen-Dalsgaard, S., Langset, M., et al. (2016). Negligible impact of ingested microplastics on tissue concentrations of persistent organic pollutants in northern fulmars off coastal Norway. Envir. Sci. Technol. 50, 1924–1933. doi: 10.1021/acs.est.5b04663
Hofmeister, F. (1888). Zur Lehre von der Wirkung der Salze. II. Arch. Exp. Pathol. Pharm. 24, 247–260.
Hussain, N., Jaitley, V., and Florence, A. T. (2001). Recent advances in the understanding of uptake of microparticulates across the gastrointestinal lymphatics. Adv. Drug. Deliver. Rev. 50, 107–142. doi: 10.1016/S0169-409X(01)00152-1
Huvet, A., Béguel, J. P., Cavaleiro, N. P., Thomas, Y., Quillien, V., Boudry, P., et al. (2015). Disruption of amylase genes by RNA interference affects reproduction in the Pacific oyster Crassostrea gigas. J. Exp. Biol. 218, 1740–1747. doi: 10.1242/jeb.116699
Huvet, A., Paul-Pont, I., Fabioux, C., Lambert, C., Suquet, M., Thomas, Y., et al. (2016). Reply to Lenz et al.: quantifying the smallest microplastics is the challenge for a comprehensive view of their environmental impacts. Proc. Natl. Acad. Sci. U.S.A. 113, E4123– E4124. doi: 10.1073/pnas.1607221113
Ioakeimidis, C., Fotopoulou, K. N., Karapanagioti, H. K., Geraga, M., Zeri, C., Papathanassiou, E., et al. (2016). The degradation potential of PET bottles in the marine environment: an ATR-FTIR based approach. Sci. Rep. 6, 1–8. doi: 10.1038/srep23501
Isobe, A., Kubo, K., Tamura, Y., Nakashima, E., and Fujii, N. (2014). Selective transport of microplastics and mesoplastics by drifting in coastal waters. Mar. Pollut. Bull. 89, 324–330. doi: 10.1016/j.marpolbul.2014.09.041
Jager, T., Barsi, A., and Ducrot, V. (2013). Hormesis on life-history traits: is there such thing as a free lunch? Ecotoxicology 22, 263–270. doi: 10.1007/s10646-012-1022-0
Jambeck, J. R., Geyer, R., Wilcox, C., Siegler, T. R., Perryman, M., Andrady, A., et al. (2015). Plastic waste inputs from land into the ocean. Science 347, 768–771. doi: 10.1126/science.1260352
Jeong, C.-B., Kang, H.-M., Lee, M.-C., Kim, D.-H., Han, J., Hwang, D.-S., et al. (2017). Adverse effects of microplastics and oxidative stress-induced MAPK/Nrf2 pathway-mediated defense mechanisms in the marine copepod Paracyclopina nana. Sci. Rep. 7:41323. doi: 10.1038/srep41323
Jeong, C. B., Won, E. J., Kang, H. M., Lee, M. C., Hwang, D. S., Hwang, U. K., et al. (2016). Microplastic size-dependent toxicity, oxidative stress induction, and p-JNK and p-p38 activation in the monogonont rotifer (Brachionus koreanus). Envir. Sci. Technol. 50, 8849–8857. doi: 10.1021/acs.est.6b01441
Kaposi, K. L., Mos, B., Kelaher, B. P., and Dworjanyn, S. A. (2014). Ingestion of microplastic has limited impact on a marine larva. Envir. Sci. Technol. 48, 1638–1645. doi: 10.1021/es404295e
Karakolis, E. G., Nguyen, B., Bem You, J., Graham, P. J., Rochman, C. M., and Sinton, D. (2018). Digestible fluorescent coatings for cumulative quantification of microplastic ingestion. Environ. Sci. Technol. Lett. 5, 62–67. doi: 10.1021/acs.estlett.7b00545
Karami, A. (2017). Gaps in aquatic toxicological studies of microplastics. Chemosphere 184, 841–848. doi: 10.1016/j.chemosphere.2017.06.048
Karami, A., Golieskardi, A., Choo, C. K., Larat, V., Karbalaei, S., and Salamatinia, B. (2018). Microplastic and mesoplastic contamination in canned sardines and sprats. Sci. Total. Environ. 612, 1380–1386. doi: 10.1016/j.scitotenv.2017.09.005
Karapanagioti, H. K., Endo, S., Ogata, Y., and Takada, H. (2011). Diffuse pollution by persistent organic pollutants as measured in plastic pellets sampled from various beaches in Greece. Mar. Pollut. Bull. 62, 312–317. doi: 10.1016/j.marpolbul.2010.10.009
Kashiwada, S. (2006). Distribution of nanoparticles in the see-through medaka (Oryzias latipes). Environ. Health. Persp. 114, 1697–1702. doi: 10.1289/ehp.9209
Katzenberger, T. D. (2015). Assessing the Biological Effects of Exposure to Microplastics in the Three-Spined Stickleback (Gasterosteus aculeatus) (Linnaeus 1758). Ph.D. these, University of York.
Kedzierski, M. (2017). Pollutions du Milieu Littoral par les Microplastiques: Méthodes d'Évaluation. Ph.D. thesis, University of Southern Brittany (France).
Keller, A. A., Wang, H., Zhou, D., Lenihan, H. S., Cherr, G., Cardinale, B. J., et al. (2010). Stability and aggregation of metal oxide nanoparticles in natural aqueous matrices. Envir. Sci. Technol. 44, 1962–1967. doi: 10.1021/es902987d
Kesy, K., Hentzsch, A., Klaeger, F., Oberbeckmann, S., Mothes, S., and Labrenz, M. (2017). Fate and stability of polyamide-associated bacterial assemblages after their passage through the digestive tract of the blue mussel Mytilus edulis. Mar. Pollut. Bull. 125, 132–138. doi: 10.1016/j.marpolbul.2017.08.016
Kesy, K., Oberbeckmann, S., Müller, F., and Labrenz, M. (2016). Polystyrene influences bacterial assemblages in Arenicola marina-populated aquatic environments in vitro. Environ. Pollut. 219, 219–227. doi: 10.1016/j.envpol.2016.10.032
Kiyama, R., and Wada-Kiyama, Y. (2015). Estrogenic endocrine disruptors: molecular mechanisms of action. Environ. Int. 83, 11–40. doi: 10.1016/j.envint.2015.05.012
Koelmans, A. A., Bakir, A., Burton, G. A., and Janssen, C. R. (2016). Microplastic as a vector for chemicals in the aquatic Environment: critical review and model-supported reinterpretation of empirical studies. Envir. Sci. Technol. 50, 3315–3326. doi: 10.1021/acs.est.5b06069
Koelmans, A. A., Besseling, E., and Foekema, E. M. (2014). Leaching of plastic additives to marine organisms. Environ. Pollut. 187, 49–54. doi: 10.1016/j.envpol.2013.12.013
Koelmans, A. A., Nowack, B., and Wiesner, M. R. (2009). Comparison of manufactured and black carbon nanoparticle concentrations in aquatic sediments. Environ. Pollut. 157, 1110–1116. doi: 10.1016/j.envpol.2008.09.006
Kolandhasamy, P., Su, L., Li, J., Qu, X., Jabeen, K., and Shi, H. (2018). Adherence of microplastics to soft tissue of mussels: a novel way to uptake microplastics beyond ingestion. Sci. Total. Environ. 610, 635–640. doi: 10.1016/j.scitotenv.2017.08.053
Kooijman, S. A. L. M. (2010). Dynamic Energy Budget Theory for Metabolic Organisation. (Melbourne: Cambridge University Press), 508.
Lagarde, F., Olivier, O., Zanella, M., Daniel, P., Hiard, S., and Caruso, A. (2016). Microplastic interactions with freshwater microalgae: hetero-aggregation and changes in plastic density appear strongly dependent on polymer type. Environ. Pollut. 215, 331–339. doi: 10.1016/j.envpol.2016.05.006
Lamb, J. B., Willis, B. L., Fiorenza, E. A., Couch, C. S., Howard, R., Rader, D. N., et al. (2018). Plastic waste associated with disease on coral reefs. Science 359, 460–462. doi: 10.1126/science.aar3320
Lattin, G. L., Moore, C. J., Zellers, A. F., Moore, S. L., and Weisberg, S. B. (2004). A comparison of neustonic plastic and zooplankton at different depths near the southern California shore. Mar. Pollut. Bull. 49, 291–294. doi: 10.1016/j.marpolbul.2004.01.020
Lee, K. w., Shim, W. J., Kwon, O. Y., and Kang, J. (2013). Size-dependent effects of micro polystyrene particles in the marine copepod Tigriopus japonicus. Envir. Sci. Technol. 47, 11278–11283. doi: 10.1021/es401932b
Lenz, R., Enders, K., and Nielsen, T. G. (2016). Microplastic exposure studies should be environmentally realistic. Proc. Natl. Acad. Sci. U.S.A. 113, E4121–E4122. doi: 10.1073/pnas.1606615113
Leslie, H. A. (2012). Microplastic in Noordzee Zwevend stof en Cosmetica. Amsterdam: Eindrapportage W-12/01, Institute for Environmental Studies.
Li, J., Yang, D., Li, L., Jabeen, K., and Shi, H. (2015). Microplastics in commercial bivalves from China. Environ. Pollut. 207, 190–195. doi: 10.1016/j.envpol.2015.09.018
Lin, P. C., Lin, S., Wang, P. C., and Sridhar, R. (2014). Techniques for physicochemical characterization of nanomaterials. Biotechnol. Adv. 32, 711–726. doi: 10.1016/j.biotechadv.2013.11.006
Long, M., Moriceau, B., Gallinari, M., Lambert, C., Huvet, A., Raffray, J., et al. (2015). Interactions between microplastics and phytoplankton aggregates: impact on their respective fates. Mar. Chem. 175, 39–46. doi: 10.1016/j.marchem.2015.04.003
Long, M., Paul-Pont, I., Hégaret, H., Moriceau, B., Lambert, C., Huvet, A., et al. (2017). Interactions between polystyrene microplastics and marine phytoplankton lead to species-specific hetero-aggregation. Environ. Pollut. 228, 454–463. doi: 10.1016/j.envpol.2017.05.047
Lu, Y., Zhang, Y., Deng, Y., Jiang, W., Zhao, Y., Geng, J., et al. (2016). Uptake and accumulation of polystyrene microplastics in zebrafish (Danio rerio) and toxic effects in liver. Envir. Sci. Technol. 50, 4054–4060. doi: 10.1021/acs.est.6b00183
Lunov, O., Syrovets, T., Loos, C., Beil, J., Delacher, M., Tron, K., et al. (2011). Differential uptake of functionalized polystyrene nanoparticles by human macrophages and a monocytic cell line. ACS Nano 5, 1657–1669. doi: 10.1021/nn2000756
Lusher, A. L., McHugh, M., and Thompson, R. C. (2013). Occurrence of microplastics in the gastrointestinal tract of pelagic and demersal fish from the English Channel. Mar. Pollut. Bull. 67, 94–99. doi: 10.1016/j.marpolbul.2012.11.028
Lusher, A. L., Tirelli, V., O'Connor, I., and Officer, R. (2015). Microplastics in Arctic polar waters: the first reported values of particles in surface and sub-surface samples. Sci. Rep. 5:14947. doi: 10.1038/srep14947
Lusher, A. L., Welden, N. A., Sobral, P., and Cole, M. (2017). Sampling, isolating and identifying microplastics ingested by fish and invertebrates. Anal. Methods. 9, 1346–1360. doi: 10.1039/C6AY02415G
Manikkam, M., Tracey, R., Guerrero-Bosagna, C., and Skinner, M. K. (2013). Plastics derived endocrine disruptors (BPA, DEHP and DBP) induce epigenetic transgenerational inheritance of obesity, reproductive disease and sperm epimutations. PLoS ONE 8:e55387. doi: 10.1371/journal.pone.0055387
Martínez-Gómez, C., León, V. M., Calles, S., Gomáriz-Olcina, M., and Vethaak, A. D. (2017). The adverse effects of virgin microplastics on the fertilization and larval development of sea urchins. Mar. Environ. Res. 130, 69-76. doi: 10.1016/j.marenvres.2017.06.016
Martin, J., Lusher, A., Thompson, R. C., and Morley, A. (2017). The deposition and accumulation of microplastics in marine sediments and bottom water from the Irish continental shelf. Sci. Rep. 7:10772. doi: 10.1038/s41598-017-11079-2
Mattsson, K., Hansson, L. A., and Cedervall, T. (2015). Nano-plastics in the aquatic environment. Environ. Sci. Process. Impact. 17, 1712–1721. doi: 10.1039/C5EM00227C
Mattsson, K., Johnson, E. V., Malmendal, A., Linse, S., Hansson, L. A., and Cedervall, T. (2017). Brain damage and behavioural disorders in fish induced by plastic nanoparticles delivered through the food chain. Sci. Rep. 7:11452. doi: 10.1038/s41598-017-10813-0
Mazurais, D., Ernande, B., Quazuguel, P., Severe, A., Huelvan, C., Madec, L., et al. (2015). Evaluation of the impact of polyethylene microbeads ingestion in European sea bass (Dicentrarchus labrax) larvae. Mar. Environ. Res. 112, 78–85. doi: 10.1016/j.marenvres.2015.09.009
McCormick, A., Hoellein, T. J., Mason, S. A., Schluep, J., and Kelly, J. J. (2014). Microplastic is an abundant and distinct microbial habitat in an urban river. Envir. Sci. Technol. 48, 11863–11871. doi: 10.1021/es503610r
Monopoli, M. P., Åberg, C., Salvati, A., and Dawson, K. A. (2012). Biomolecular coronas provide the biological identity of nanosized materials. Nat. Nanotech. 7, 779–786. doi: 10.1038/nnano.2012.207
Moore, C. J., Moore, S. L., Leecaster, M. K., and Weisberg, S. B. (2001). A comparison of plastic and plankton in the North Pacific central gyre. Mar. Pollut. Bull. 42, 1297–1300. doi: 10.1016/S0025-326X(01)00114-X
Moore, C. J., Moore, S. L., Weisberg, S. B., Lattin, G. L., and Zellers, A. F. (2002). A comparison of neustonic plastic and zooplankton abundance in southern California's coastal waters. Mar. Pollut. Bull. 44, 1035–1038. doi: 10.1016/S0025-326X(02)00150-9
Munari, C., Infantini, V., Scoponi, M., Rastelli, E., and Corinaldesi, C. (2017). Microplastics in the sediments of Terra Nova Bay (Ross Sea, Antarctica). Mar. Pollut. Bull. 122, 161–165. doi: 10.1016/j.marpolbul.2017.06.039
Murray, F., and Cowie, P. R. (2011). Plastic contamination in the decapod crustacean Nephrops norvegicus (Linnaeus, 1758). Mar. Pollut. Bull. 62, 1207–1217. doi: 10.1016/j.marpolbul.2011.03.032
Nasser, F., and Lynch, I. (2016). Secreted protein eco-corona mediates uptake and impacts of polystyrene nanoparticles on Daphnia magna. J. Proteomics 137, 45–51. doi: 10.1016/j.jprot.2015.09.005
National Oceanic and Atmospheric Administration (NOAA). (2008). Proceedings of the International Research Workshop on the Occurrence, effects, and fate of Microplastic Marine debris eds C Arthur, J. Baker, and H. Bamford. Technical Memorandum NOS-OR&R-30 (Tacoma, WA University of Washington Tacoma).
Net, S., Sempéré, R., Delmont, A., Paluselli, A., and Ouddane, B. (2015). Occurrence, fate, behavior and ecotoxicological state of phthalates in different environmental matrices. Envir. Sci. Technol. 49, 4019–4035. doi: 10.1021/es505233b
Ng, K. L., and Obbard, J. P. (2006). Prevalence of microplastics in Singapore's coastal marine environment. Mar. Pollut. Bull. 52, 761–767. doi: 10.1016/j.marpolbul.2005.11.017
Nisbet, R. M., Jusup, M., Klanjscek, T., and Pecquerie, L. (2012). Integrating dynamic energy budget (DEB) theory with traditional bioenergetic models. J. Exp. Biol. 215, 892–902. doi: 10.1242/jeb.059675
Nobre, C. R., Santana, M. F. M., Maluf, A., Cortez, F. S., Cesar, A., Pereira, C. D. S., et al. (2015). Assessment of microplastic toxicity to embryonic development of the sea urchin Lytechinus variegatus (Echinodermata: Echinoidea). Mar Pollut. Bull. 92, 99–104. doi: 10.1016/j.marpolbul.2014.12.050
Norén, F. (2007). Small Plastic Particles in Coastal Swedish Waters. KIMO Sweden. Availbale online at: http://www.kimointernational.org/ (Accessed January 8, 2018).
Obbard, R. W. (2018). Microplastics in polar regions: the role of long range transport. Curr. Opin. Env. Sci. Health. 1, 24–29. doi: 10.1016/j.coesh.2017.10.004
Oberhänsli, F., Swarzenski, P., Tolosa, I., Danis, B., and Metian, M. (2017). “Experimental nuclear applications in marine plastics research,” in Poster Session, International Conference on Microplastic Pollution in the Mediterranean sea (Capri).
Oehlmann, J., Schulte-Oehlmann, U., Kloas, W., Jagnytsch, O., Lutz, I., Kusk, K. O., et al. (2009). A critical analysis of the biological impacts of plasticizers on wildlife. Philos. Trans. Roy. Soc. B. 364, 2047–2062. doi: 10.1098/rstb.2008.0242
Ogonowski, M., Schür, C., Jarsén, Å., and Gorokhova, E. (2016). The effects of natural and anthropogenic microparticles on individual fitness in Daphnia magna. PLoS ONE 11:e0155063. doi: 10.1371/journal.pone.0155063
Ostroumov, S. A. (2003). Studying effects of some surfactants and detergents on filter-feeding bivalves. Hydrobiologia 500, 341–344. doi: 10.1023/A:1024604904065
Pappo, J., and Ermak, T. H. (1989). Uptake and translocation of fluorescent latex particles by rabbit Peyer's patch follicle epithelium: a quantitative model for M cell uptake. Clin. Exp. Immunol. 76:144
Paul-Pont, I., Lacroix, C., Fernández, C. G., Hégaret, H., Lambert, C., Le Goïc, N., et al. (2016). Exposure of marine mussels Mytilus spp. to polystyrene microplastics: toxicity and influence on fluoranthene bioaccumulation. Environ. Pollut. 216, 724–737. doi: 10.1016/j.envpol.2016.06.039
Pedà, C., Caccamo, L., Fossi, M. C., Gai, F., Andaloro, F., Genovese, L., et al. (2016). Intestinal alterations in European sea bass Dicentrarchus labrax (Linnaeus, 1758) exposed to microplastics: preliminary results. Environ. Pollut. 212, 251–256. doi: 10.1016/j.envpol.2016.01.083
Peeken, I., Primpke, S., Beyer, B., Gütermann, J., Katlein, C., Krumpen, T., et al. (2018). Arctic sea ice is an important temporal sink and means of transport for microplastic. Nat. Commun. 95, 2041–1723. doi: 10.1038/s41467-018-03825-5
Phuong, N. N., Zalouk-Vergnoux, A., Poirier, L., Kamari, A., Châtel, A., Mouneyrac, C., et al. (2016). Is there any consistency between the microplastics found in the field and those used in laboratory experiments? Environ. Pollut. 211, 111–123. doi: 10.1016/j.envpol.2015.12.035
Pittura, L., Avio, C. G., Giuliani, M. E., d'Errico, G., Keiter, S. H., Cormier, B., et al. (2018). Microplastics as vehicles of environmental PAHs to marine organisms: combined chemical and physical hazards to the mediterranean mussels, Mytilus galloprovincialis. Front. Mar. Sci. 5:103. doi: 10.3389/fmars.2018.00103
PlasticsEurope (2016). Plastics - The Facts 2016: An Analysis of European Latest Plastics Production, Demand and Waste Data. Frankfurt.
Rajakumar, K., Sarasvathy, V., Chelvan, A. T., Chitra, R., and Vijayakumar, C. T. (2009). Natural weathering studies of polypropylene. J. Polym. Environ. 17, 191. doi: 10.1007/s10924-009-0138-7
Reddy, M. S., Basha, S., Adimurthy, S., and Ramachandraiah, G. (2006). Description of the small plastics fragments in marine sediments along the Alang-Sosiya ship-breaking yard, India. Estuar. Coast. Shelf. S. 68, 656–660. doi: 10.1016/j.ecss.2006.03.018
Reisser, J., Slat, B., Noble, K., Du Plessis, K., Epp, M., Proietti, M., et al. (2015). The vertical distribution of buoyant plastics at sea: an observational study in the North Atlantic Gyre. Biogeosciences 12:1249 doi: 10.5194/bg-12-1249-2015
Ribeiro, F., Garcia, A. R., Pereira, B. P., Fonseca, M., Mestre, N. C., Fonseca, T. G., et al. (2017). Microplastics effects in Scrobicularia plana. Mar. Pollut. Bull. 122, 379–391. doi: 10.1016/j.marpolbul.2017.06.078
Rochman, C. M. (2016). Ecologically relevant data are policy-relevant data. Science 352, 1172–1172. doi: 10.1126/science.aaf8697
Rochman, C. M., Browne, M. A., Underwood, J., van Franeker, J. A., Thomposon, R. C., and Amaral-Zettler, L. A. (2016). The ecological impacts of marine debris: unraveling the demonstrated evidence from what is perceived. Ecology 87:14-2070.1. doi: 10.1890/14-2070.1
Rochman, C. M., Hoh, E., Hentschel, B. T., and Kaye, S. (2013a). Long-term field measurement of sorption of organic contaminants to five types of plastic pellets: implications for plastic marine debris. Envir. Sci. Technol. 47, 1646–1654. doi: 10.1021/es303700s
Rochman, C. M., Hoh, E., Kurobe, T., and Teh, S. J. (2013b). Ingested plastic transfers hazardous chemicals to fish and induces hepatic stress. Sci. Rep. 3:3263. doi: 10.1038/srep03263
Rochman, C. M., Kurobe, T., Flores, I., and Teh, S. J. (2014). Early warning signs of endocrine disruption in adult fish from the ingestion of polyethylene with and without sorbed chemical pollutants from the marine environment. Sci. Total. Environ. 493, 656–661. doi: 10.1016/j.scitotenv.2014.06.051
Rolton, A., Vignier, J., Volety, A. K., Pierce, R. H., Henry, M., Shumway, S. E., et al. (2016). Effects of field and laboratory exposure to the toxic dinoflagellate Karenia brevis on the reproduction of the eastern oyster, Crassostrea virginica, and subsequent development of offspring. Harmful Algae 57(Pt A), 13–26. doi: 10.1016/j.hal.2016.04.011
Rossi, G., Barnoud, J., and Conticelli, L. (2014). Polystyrene nanoparticles perturb lipid membranes. J. Phys. Chem. Lett. 5, 241–246. doi: 10.1021/jz402234c
Rummel, C. D., Jahnke, A., Gorokhova, E., Kühnel, D., and Schmitt-Jansen, M. (2017). The Impacts of biofilm formation on the fate and potential effects of microplastic in the aquatic environment. Envir. Sci. Technol. Lett. 4, 258–267. doi: 10.1021/acs.estlett.7b00164
Rummel, C. D., Löder, M. G., Fricke, N. F., Lang, T., Griebeler, E. M., Janke, M., et al. (2016). Plastic ingestion by pelagic and demersal fish from the North Sea and Baltic Sea. Mar. Pollut. Bull. 102, 134–141. doi: 10.1016/j.marpolbul.2015.11.043
Sagarin, R. D., Adams, J., Blanchette, C. A., Brusca, R. C., Chorover, J., Cole, J. E., et al. (2016). Between control and complexity: opportunities and challenges for marine mesocosms. Front. Ecol. Environ. 14, 389–396. doi: 10.1002/fee.1313
Setälä, O., Fleming-Lehtinen, V., and Lehtiniemi, M. (2014). Ingestion and transfer of microplastics in the planktonic food web. Environ. Pollut. 185, 77–83. doi: 10.1016/j.envpol.2013.10.013
Sjollema, S. B., Redondo-Hasselerharm, P., Leslie, H. A., Kraak, M. H., and Vethaak, A. D. (2016). Do plastic particles affect microalgal photosynthesis and growth?. Aquat. Toxicol. 170, 259–261. doi: 10.1016/j.aquatox.2015.12.002
Song, Y. K., Hong, S. H., Jang, M., Han, G. M., and Shim, W. J. (2014). Occurrence and distribution of microplastics in the sea surface microlayer in Jinhae Bay, South Korea. Arch. Environ. Con. Tox. 69, 279–287. doi: 10.1007/s00244-015-0209-9
Sowada, J., Lemoine, L., Schön, K., Hutzler, C., Luch, A., and Tralau, T. (2017). Toxification of polycyclic aromatic hydrocarbons by commensal bacteria from human skin. Arch. Toxicol. 91, 2331–2341. doi: 10.1007/s00204-017-1964-3
Suaria, G., Avio, C. G., Mineo, A., Lattin, G. L., Magaldi, M. G., Belmonte, G., et al. (2016). The Mediterranean Plastic Soup: synthetic polymers in Mediterranean surface waters. Sci. Rep. 6:37551. doi: 10.1038/srep37551
Sundt, P., Schulze, P.-E., and Syversen, F. (2014). Sources of Microplastic-Pollution to the Marine Environment. Report no M-321/2015. Asker: Mepex Consult.
Sussarellu, R., Suquet, M., Thomas, Y., Lambert, C., Fabioux, C., Pernet, M. E. J., et al. (2016). Oyster reproduction is affected by exposure to polystyrene microplastics. Proc. Natl. Acad. Sci. U.S.A. 113, 2430–2435. doi: 10.1073/pnas.1519019113
Talsness, C. E., Andrade, A. J., Kuriyama, S. N., Taylor, J. A., and Vom Saal, F. S. (2009). Components of plastic: experimental studies in animals and relevance for human health. Philos. Trans. R. Soc. B. 364, 2079–2096. doi: 10.1098/rstb.2008.0281
Ter Halle, A., Jeanneau, L., Martignac, M., Jardé, E., Pedrono, B., Brach, L., et al. (2017). Nanoplastic in the north atlantic subtropical gyre. Envir. Sci. Technol. 51, 13689–13697. doi: 10.1021/acs.est.7b03667
Teuten, E. L., Rowland, S. J., Galloway, T. S., and Thompson, R. C. (2007). Potential for plastics to transport hydrophobic contaminants. Envir. Sci. Technol. 41, 7759–7764. doi: 10.1021/es071737s
Teuten, E. L., Saquing, J. M., Knappe, D. R. U., Barlaz, M. A., Jonsson, S., Björn, A., et al. (2009). Transport and release of chemicals from plastics to the environment and to wildlife. Philos. Trans. R. Soc. B. 364, 2027–2045. doi: 10.1098/rstb.2008.0284
Thompson, R. C., Moore, C. J., vom Saal, F. S., and Swan, S. H. (2009). Plastics, the environment and human health: current consensus and future trends. Philos. Trans. Roy. Soc. B. 364, 2153–2166. doi: 10.1098/rstb.2009.0053
Tincani, F. H., Galvan, G. L., Marques, A. E., Santos, G. S., Pereira, L. S., and da Silva, T. A. (2017). Pseudoreplication and the usage of biomarkers in ecotoxicological bioassays. Environ. Toxicol. Chem. 36, 2868–2874. doi: 10.1002/etc.3823
Tiwari, J., Naoghare, P., Sivanesan, S., and Bafana, A. (2017). Biodegradation and detoxification of chloronitroaromatic pollutant by Cupriavidus. Bioresour. Technol. 223, 184–191. doi: 10.1016/j.biortech.2016.10.043
Van Cauwenberghe, L., and Janssen, C. R. (2014). Microplastics in bivalves cultured for human consumption. Environ. Pollut. 193, 65–70. doi: 10.1016/j.envpol.2014.06.010
Van Cauwenberghe, L., Vanreusel, A., Mees, J., and Janssen, C. R. (2013). Microplastic pollution in deep-sea sediments. Environ. Pollut. 182, 495–499. doi: 10.1016/j.envpol.2013.08.013
Velzeboer, I., Kwadijk, C. J. A. F., and Koelmans, A. A. (2014). Strong sorption of PCBs to nanoplastics, microplastics, carbon nanotubes, and fullerenes. Envir. Sci. Technol. 48, 4869–4876. doi: 10.1021/es405721v
Verwey, E. J. W., and Overbeek, J. T. G. (1999). Theory of the Stability of Lyophobic Colloids. Mineola, NY: Dover Publications, Inc.
Vianello, A., Boldrin, A., Guerriero, P., Moschino, V., Rella, R., Sturaro, A., et al. (2013). Microplastic particles in sediments of Lagoon of Venice, Italy: first observations on occurrence, spatial patterns and identification. Estuar. Coast. Shelf. S. 130, 54–61. doi: 10.1016/j.ecss.2013.03.022
Von Moos, N., Burkhardt-Holm, P., and Köhler, A. (2012). Uptake and effects of microplastics on cells and tissue of the blue mussel Mytilus edulis L. after an experimental exposure. Envir. Sci. Technol. 46, 11327–11335. doi: 10.1021/es302332w
Vroom, R. J. E., Koelmans, A. A., Besseling, E., and Halsband, C. (2017). Aging of microplastics promotes their ingestion by marine zooplankton. Environ Pollut. 231, 987–996. doi: 10.1016/j.envpol.2017.08.088
Walker, C. H., Sibly, R. M., Hopkin, S. P., and Peakall, D. B. (2012). Principles of Ecotoxicology. Fourth edn. Boca Raton, FL: CRC Press, 386.
Ward, J. E., and Kach, D. J. (2009). Marine aggregates facilitate ingestion of nanoparticles by suspension-feeding bivalves. Mar. Environ. Res. 68, 137–142. doi: 10.1016/j.marenvres.2009.05.002
Watts, A. J., Lewis, C., Goodhead, R. M., Beckett, S. J., Moger, J., Tyler, C. R., et al. (2014). Uptake and retention of microplastics by the shore crab Carcinus maenas. Envir. Sci. Technol. 48, 8823–8830. doi: 10.1021/es501090e
Watts, A. J., Urbina, M. A., Goodhead, R., Moger, J., Lewis, C., and Galloway, T. S. (2016). Effect of microplastic on the gills of the shore crab Carcinus maenas. Envir. Sci. Technol. 50, 5364–5369. doi: 10.1021/acs.est.6b01187
Wesch, C., Bredimus, K., Paulus, M., and Klein, R. (2016). Towards the suitable monitoring of ingestion of microplastics by marine biota: a review. Environ. Pollut. 218, 1200–1208. doi: 10.1016/j.envpol.2016.08.076
White, J. R. (2006). Polymer ageing: physics, chemistry or engineering? Time to reflect. CR. Chim. 9, 1396–1408. doi: 10.1016/j.crci.2006.07.008
Wicke, D., Böckelmann, U., and Reemtsma, T. (2008). Environmental influences on the partitioning and diffusion of hydrophobic organic contaminants in microbial biofilms. Envir. Sci. Technol. 42, 1990–1996. doi: 10.1021/es702267s
Wright, S. L., Rowe, D., Thompson, R. C., and Galloway, T. S. (2013a). Microplastic ingestion decreases energy reserves in marine worms. Curr. Biol. 23, R1031–R1033. doi: 10.1016/j.cub.2013.10.068
Wright, S. L., Thompson, R. C., and Galloway, T. S. (2013b). The physical impacts of microplastics on marine organisms: a review. Environ. Pollut. 178, 483–492. doi: 10.1016/j.envpol.2013.02.031
Yokota, K., Waterfield, H., Hastings, C., Davidson, E., Kwietniewski, E., and Wells, B. (2017). Finding the missing piece of the aquatic plastic pollution puzzle: interaction between primary producers and microplastics. L&O Lett. 2, 91–104. doi: 10.1002/lol2.10040
Yu, S., Liu, J., Yin, Y., and Shen, M. (2017). Interactions between engineered nanoparticles and dissolved organic matter: a review on mechanisms and environmental effects. J. Environ. Sci. 63, 198–217. doi: 10.1016/j.jes.2017.06.021
Yu, X., Peng, J., Wang, J., Wang, K., and Bao, S. (2016). Occurrence of microplastics in the beach sand of the Chinese inner sea: the Bohai Sea. Environ. Pollut. 214, 722–730. doi: 10.1016/j.envpol.2016.04.080
Zettler, E. R., Mincer, T. J., and Amaral-Zettler, L. A. (2013). Life in the “Plastisphere”: microbial communities on plastic marine debris. Envir. Sci. Technol. 47, 7137–7146. doi: 10.1021/es401288x
Zhang, C., Chen, X., Wang, J., and Tan, L. (2017). Toxic effects of microplastic on marine microalgae Skeletonema costatum: interactions between microplastic and algae. Environ. Pollut. 220, 1282–1288. doi: 10.1016/j.envpol.2016.11.005
Zhang, W., Zhang, S., Wang, J., Wang, Y., Mu, J., Wang, P., et al. (2017). Microplastic pollution in the surface waters of the Bohai Sea, China. Environ. Pollut. 231, 541–548. doi: 10.1016/j.envpol.2017.08.058
Zhao, S., Zhu, L., Wang, T., and Li, D. (2014). Suspended microplastics in the surface water of the Yangtze Estuary System, China: first observations on occurrence, distribution. Mar. Pollut. Bull. 86, 562–568. doi: 10.1016/j.marpolbul.2014.06.032
Keywords: microplastics, nanoplastics, experimental exposure, impacts, marine organisms, physiology
Citation: Paul-Pont I, Tallec K, Gonzalez-Fernandez C, Lambert C, Vincent D, Mazurais D, Zambonino-Infante J-L, Brotons G, Lagarde F, Fabioux C, Soudant P and Huvet A (2018) Constraints and Priorities for Conducting Experimental Exposures of Marine Organisms to Microplastics. Front. Mar. Sci. 5:252. doi: 10.3389/fmars.2018.00252
Received: 04 April 2018; Accepted: 29 June 2018;
Published: 18 July 2018.
Edited by:
Ricardo Beiras, University of Vigo, SpainReviewed by:
Francesco Regoli, Università Politecnica delle Marche, ItalyXavier Cousin, Institut Français de Recherche pour l'Exploitation de la Mer (IFREMER), France
Copyright © 2018 Paul-Pont, Tallec, Gonzalez-Fernandez, Lambert, Vincent, Mazurais, Zambonino-Infante, Brotons, Lagarde, Fabioux, Soudant and Huvet. This is an open-access article distributed under the terms of the Creative Commons Attribution License (CC BY). The use, distribution or reproduction in other forums is permitted, provided the original author(s) and the copyright owner(s) are credited and that the original publication in this journal is cited, in accordance with accepted academic practice. No use, distribution or reproduction is permitted which does not comply with these terms.
*Correspondence: Ika Paul-Pont, aWthLnBhdWxwb250QHVuaXYtYnJlc3QuZnI=