- 1Leibniz Institute for Baltic Sea Research Warnemünde, Rostock, Germany
- 2Center of Limnology, Estonian University of Life Sciences, Tartu, Estonia
- 3Tvarminne Zoological Station, University of Helsinki, Hanko, Finland
Predicted increases in sea surface temperatures are expected to shift the balance between autotrophic production and the heterotrophic degradation of organic matter toward a more heterotrophic system. For early phytoplankton spring blooms at low water temperature the impact of rising temperatures has been mainly investigated in mesocosm experiments, while field observations are scarce. During a Baltic Sea research cruise we examined early spring bloom conditions, characterized by low temperatures (0–3°C), and performed on-board warming experiments to compare the responses of phyto- and bacterioplankton production to an increase in temperature. In the northern Baltic Sea, the low phytoplankton biomass indicated pre-bloom conditions. In the southern Baltic Sea, a diatom-dominated phytoplankton bloom with increased primary production (PP) occurred. Associated with this bloom were increases in bacterial production (BP) and bacterial abundance as well as shifts in bacterial community composition toward an increased proportion of Gammaproteobacteria and Bacteroidetes. However, the low BP/PP ratios (average: 1.2 ± 0.14%) indicated weak coupling between the bacterial and phytoplankton communities. Short-term warming (6 h, Δ+6°C) significantly enhanced PP (mean Q10 1.4) and especially BP (mean Q10 2.3). Hence, the higher water temperature increased both carbon flow into the bacterial community and bacterial processing of organic matter, thereby confirming previous experimental studies. By contrast, BP/PP ratios remained relatively low after warming (average: 1.7 ± 0.5%), unlike in previous mesocosm experiments performed at comparable temperatures and with similar plankton communities. Overall, these results imply that bacterial activities are suppressed during early phytoplankton blooms at low temperatures in the Baltic Sea and are not substantially altered by short-term warming events.
Introduction
Under various greenhouse gas emission scenarios, ocean surface temperatures are predicted to increase 2–5°C by the end of this century (IPCC, 2013). Rising temperatures directly and indirectly impact pelagic organisms and aquatic food webs, leading to changes in the structure and functioning of marine ecosystems (Boyd and Doney, 2002; Sarmiento et al., 2004). Phytoplankton account for ~50% of global net primary production (PP) and are the main energy source for aquatic ecosystems (Field et al., 1998). The major consumers of phytoplankton-derived organic matter are heterotrophic bacteria present in the upper water layers of aquatic ecosystems. The coupling of phytoplankton dissolved organic carbon (DOC) production with DOC consumption by heterotrophic prokaryotes (mostly bacteria) plays a central role in the biogeochemistry of pelagic food webs (Azam, 1998; Ducklow, 2000). The phytoplankton-bacteria relationship and the coupling between the two components is generally analyzed by comparing primary production to bacterial production (BP) rates (Hoppe et al., 2002; Morán et al., 2002, 2013) or, more comprehensively, to bacterial carbon demand (BCD), thereby also including bacterial respiration (del Giorgio et al., 1997; Rivkin and Legendre, 2001). The degree of coupling between autotrophic producers and heterotrophic decomposers in planktonic systems has a strong impact on the fate of organic matter and its partitioning into different pathways, such as microbial utilization, transfer to higher trophic levels, or accumulation and export (Wohlers et al., 2009). The DOC production by phytoplankton also shapes the succession of bacterial taxa and their specific functions (Sarmento and Gasol, 2012; Teeling et al., 2012), and the amount and the composition of the released DOM strongly depend on phytoplankton species and the physiological status of this cell (Nagata, 2000; Thornton, 2014).
In principle, all biological processes are modulated by temperature but the observed effects on metabolism in marine plankton are generally stronger for heterotrophic than for autotrophic organisms (Pomeroy and Deibel, 1986; Morán et al., 2006). The metabolic theory of ecology (Brown et al., 2004) predicts that respiration increases at higher rate than photosynthesis with increasing temperature, due to the lower activation energy of autotrophs (Harris et al., 2006). Moreover, phytoplankton are most often limited by light or nutrient levels (Tilzer et al., 1986), which diminishes the temperature sensitivity of growth (Edwards et al., 2016). The effects of temperature are reflected in reduced bacterial growth during phytoplankton spring blooms at low temperatures (e.g., polar regions), which may temporarily uncouple heterotrophic DOC consumption from autotrophic organic matter production (Pomeroy and Deibel, 1986; Kirchman et al., 2009). Conversely, an increase in water temperature has the potential to intensify the degree of phytoplankton-bacterioplankton coupling by stimulating bacterial growth and substrate consumption more than for the phytoplankton. Therefore, an increase in temperature potentially shifts the balance of autotrophic production and heterotrophic consumption toward the latter (Hoppe et al., 2002; López-Urrutia et al., 2006; Morán et al., 2006; O'Connor et al., 2009; Degerman et al., 2013).
Over the last decade, the impact of temperature changes on phyto-bacterioplankton coupling and the consequences for the marine carbon cycle have mainly been investigated in mesocosm studies that included experimental warming (e.g., Morán et al., 2006; O'Connor et al., 2009; Lindh et al., 2012). For example, the effect of sea surface warming on food web dynamics and pelagic carbon flow patterns has been investigated in several indoor-mesocosm experiments using natural spring plankton communities from the Baltic Sea (Sommer et al., 2012; Wohlers-Zöllner et al., 2012). Among other results, a temperature increase was repeatedly shown to strongly stimulate bacterial abundance, bacterial production (BP), and bacterial respiration, resulting in an increased processing of phytoplankton-derived organic matter by heterotrophic bacteria and a higher carbon flow into the microbial food web (Hoppe et al., 2008; Wohlers et al., 2009; von Scheibner et al., 2014). The results of other experiments, performed both in the Baltic Sea (Müren et al., 2005; Eriksson Wiklund et al., 2009; Degerman et al., 2013; Vaquer-Sunyer et al., 2015) and in other marine areas (e.g., Keller et al., 1999; O'Connor et al., 2009), also revealed that an increase in temperature increases planktonic respiration and intensifies the coupling of primary producers and heterotrophic consumers. Overall, warming-induced increases in heterotrophic activities resulted in a higher net consumption of DOC and subsequently in a reduced net consumption of dissolved inorganic carbon (DIC), thus constituting a positive feedback response to global warming (Wohlers et al., 2009).
However, current knowledge of the underlying mechanisms by which surface water warming influences food web dynamics and phyto-bacterioplankton coupling is still limited, and it is not clear whether the results from mesocosm studies can be extrapolated to in situ conditions. This is due to a paucity of field studies investigating the coupling of phyto- and bacterioplankton production under the in situ conditions of the early spring bloom, when water temperatures are low and the phytoplankton development depends on a first and generally weak stratification of the water column.
The Baltic Sea is a brackish, semi-enclosed shelf sea, with pronounced phytoplankton blooms in spring and autumn. In the southern Baltic, the phytoplankton spring bloom normally occurs between late February and early April whereas in northern regions it often begins later and extends until May, depending on the intensity of the surface irradiance, water stratification, and ice cover (Spilling and Markager, 2008; Wasmund et al., 2008; Klais et al., 2013). The spring blooms are typically dominated by diatoms (e.g., Chaetoceros spp. and Skeletonema costatum) although in some parts of the Baltic Sea (e.g., the central Baltic Sea) cold-water dinoflagellates may be dominant, especially after warmer winters (Wasmund et al., 2008, 2011; Klais et al., 2011, 2013). A strong increase in annual mean surface temperature is predicted for the Baltic Sea, with pronounced winter warming by up to 6°C expected by the end of this century (HELCOM, 2013).
The aim of this study was to investigate the phyto- bacterioplankton coupling during early spring bloom conditions at low water temperatures in the Baltic Sea, and to examine their response to short-term warming. We hypothesized that at these low temperatures the activity and production of planktonic bacteria would be suppressed compared to phytoplankton. For this purpose, we collected water samples from different stations in the northern and southern Baltic Sea, assessed phyto- and bacterioplankton composition and measured primary and bacterial production. Additionally, we performed shipboard incubations, where PP and BP levels in response to an increase in temperature were measured. Some of the results confirmed those of previous mesocosm warming studies, but striking differences with respect to the strength of phyto-bacterioplankton coupling became also obvious.
Materials and Methods
Sampling
Water samples were taken at eight stations (stations 1–8), extending from the Gulf of Finland to the southern Baltic Sea, during a research cruise of the R/V Alkor between March 4 and 11, 2009 (Figure 1). Surface water samples were collected directly after sunrise using a rosette comprising 24 10-L bottles equipped with a conductivity/temperature/depth (CTD) sensor (SeaBird 911) and sensors for fluorescence. The concentrations of inorganic nutrients were determined as described by Grasshoff et al. (1999). To collect microbial biomass for DNA extraction, water samples of 1–1.5 L were filtered onto 0.2-μm polycarbonate filters (without pre-filtration) and stored frozen at −80°C. DNA was extracted as described in Weinbauer et al. (2002). For phytoplankton, 250-mL samples were fixed with Lugol's iodine and a subsample was later counted using an inverted microscope (Utermöhl, 1958). Phytoplankton species identification was performed in agreement with the HELCOM COMBINE protocol and the Checklist of Baltic Sea Phytoplankton Species (Baltic Sea Environment Proceedings No. 95, Helsinki Commission). Phytoplankton cell volumes were calculated after an approximation to geometric standards and converted to phytoplankton biomass (μg C L−1) according to HELCOM recommendations (Olenina et al., 2006).
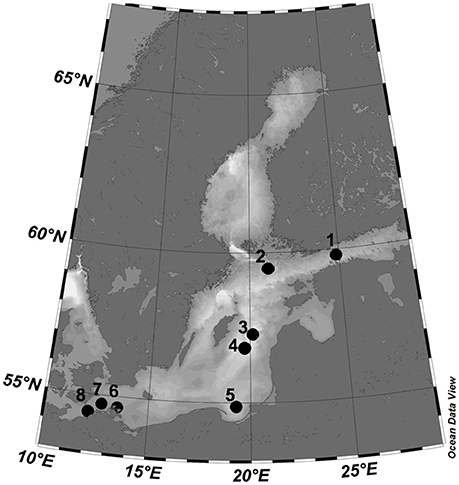
Figure 1. Map of the Baltic Sea, showing the study area and the position of the eight sampling stations. According to the GPS coordinates, the station map was plotted using Ocean Data View (Schlitzer, 2011).
Heterotrophic and autotrophic picoplankton were analyzed by flow cytometry using a FACScalibur (Becton & Dickinson) with a constant flow rate (35 μL min−1) and yellow-green latex beads (0.5 μm, Polysciences), which served as an internal standard. Duplicates of unfiltered 4-mL samples were fixed with 400 μL of 1% paraformaldehyde and 0.05% glutaraldehyde (final concentration), shock frozen in liquid nitrogen, and stored at −20°C. Smaller autotrophic cells, including Synechococcus as well as pico- und nanoeukaryotic phytoplankton (< 5 μm), were distinguished by size and fluorescence (chlorophyll-a and phycoerythrin). Heterotrophic cells were analyzed after staining with 2.5 μM (final concentration) SYBR Green (Molecular Probes). Bacteria were detected by their characteristic position in a plot of side scatter (SSC) vs. green fluorescence (FL1) and were further divided into high nucleic acid (HNA) and low nucleic acid (LNA) bacterial cells as described by Gasol and del Giorgio (2000).
Primary Production and Bacterial Secondary Production
The first CTD in the morning (7:30 a.m. for all stations except stations 1, 5, and 8, 10:30 a.m.) was used to collect surface water (1–2.5 m) for the experimental incubations and the PP and BP measurements at in situ and experimentally increased (+6°C) temperatures. PP was measured using the [14C]-bicarbonate incorporation method of Gragas (1975), with 200 μL of [14C]-bicarbonate (10 μCi/mL) per 250-mL sample and three different light intensities (100, 75, and 50% of in situ light irradiation) to simulate the first few meters of the surface water layer. Triplicate samples of each light intensity and of one sample subjected to dark conditions (covered with aluminum foil) were incubated in a closed transparent incubator for 6 h on the deck of the ship at two different temperatures (Δ0°C and Δ+6°C) (Figure S1). The temperature was carefully adjusted to Δ+6°C using a thermostatic water bath according to the measured ambient water temperature (Table 1). The reactions were terminated by immediately filtering the samples through cellulose-nitrate filters (0.2 μm) and then exposing them to HCl fumes for 10 min before they were fixed with Lumagel scintillation cocktail (Packard). The PP values were used to calculate the surface water production rates (μg C m−3). Due to technical problems in gathering data of daily rates of photosynthetically active radiation (PAR), daily production rates were roughly estimated by doubling the values for the half-day incubations (6 h) (Wasmund et al., 2001).
BP was measured based on [3H]-leucine (306 mCi mmol−1) incorporation as described by Simon and Azam (1989). Triplicate 10-mL aliquots of the unfiltered samples and of one blank were incubated in dark with [3H]-leucine (100 nM final concentration) at two different temperatures (Δ0°C and Δ+6°C) for 2 h before the reactions were stopped by the incorporation of formaldehyde (1% final concentration). The blank consisted of a sample in which formaldehyde was added before the addition of [3H]-leucine. The same thermostatic water bath, as for the PP, was used to adjust the temperature to Δ+6°C. All samples were filtered onto 0.2-μm polycarbonate filters (Millipore) and rinsed with 10 mL of cold 5% trichloroacetic acid. The filters were dissolved in 4 mL of scintillation cocktail (Lumagel Plus) and the incorporated label subsequently counted in a scintillation counter (Packard). BP was calculated, assuming a leucine to carbon conversion factor of 1.5 kgC mol−1 leucine (Kirchman, 2001). Bacterial carbon demand (BCD) was estimated based on BP and an estimate of bacterial respiration, using the models proposed by Rivkin and Legendre (2001) as well as by del Giorgio and Cole (1998), Table 2.
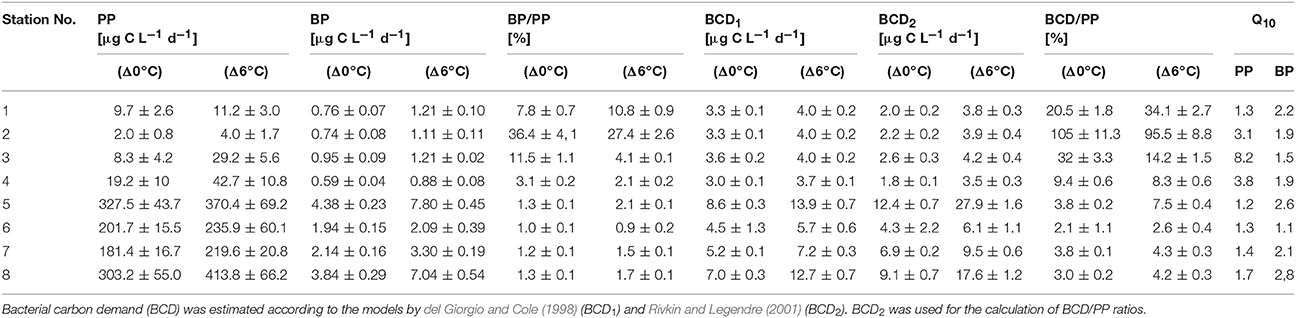
Table 2. Daily primary production (PP), bacterial production (BP), and the resulting BP/PP ratios for Δ0°C and Δ+6°C and derived Q10 values for PP and BP.
Activation energy for metabolic rates (PP and BP) was derived from the Arrhenius equation by
where k1 and k2 are the metabolic rates at in situ and elevated temperature, respectively, T1 and T2 are the corresponding in situ and elevated incubation temperatures in Kelvin (K) and R is the gas constant (8.314472 mol−1 K−1). The Q10 (the relative change in a metabolic rate expected for a 10 K temperature increase) was calculated by using the equation of Raven and Geider (1988):
where Ea is the activation energy, R is the gas constant and T is the mean temperature in Kelvin.
Analysis of Bacterial Community Composition
DNA was amplified using the bacterial 16S rRNA gene primers Bakt_341F (CCTACGGGNGGCWGCAG) and Bakt_805R (GACTACHVGGGTATCTAATCC) (Herlemann et al., 2011) and sequenced using pyrosequencing technology. For data analysis, the resulting sequences were assembled using QIIME 1.9.1 (Caporaso et al., 2010) and the “joins paired-end Illumina reads” function with default settings to merge forward and reverse sequences with an overlap of at least 30 bp. Sequences without overlap were discharged. After converting fastq to fasta using the “convert_fastaqual_fastq” function, the resulting sequences were evaluated using the SILVA NGS pipeline (Klindworth et al., 2013) with default settings. This automated pipeline aligns the reads to a curated database using the SINA aligner (Pruesse et al., 2012), in which problematic reads such as PCR artifacts (including potential chimeras) and non-ribosomal reads are filtered out. The reads were quality filtered with the following settings: reads < 50 aligned nucleotides and reads with >2% ambiguities, >2% homopolymers, or low alignment quality. After alignment, the sequences were dereplicated by clustering according to their 98% sequence identity with each other (pairwise distance and single linkage clustering) using CD-HIT (Li and Godzik, 2006). The longest read in each cluster was BLAST searched against SILVA SSU Ref 128 for the classification of sequences. The resulting classification of the reference sequence of a cluster was mapped to all members of the respective cluster as well as to their replicates. Similar classifications (approximately resembling genus level) were merged to operational taxonomic units (OTUs). Closest related sequences in the SILVA SSU Ref 128 database to the sequence with most abundant reads are shown for the dominant OTUs in Table S1. Sequences having an average BLAST alignment coverage and alignment identity of < 93% were considered as unclassified and assigned to the group “no relative.” OTU counts were rarefied to 1000 reads per sample using the single_rarefraction.py script implemented in Qiime version 2.0 (Caporaso et al., 2010). The 16S rRNA gene sequences were part of a previous study (Herlemann et al., 2016). The raw sequencing data were deposited at the Short Sequence Archive under accession number PRJEB14590.
Statistical Analyses
PP and BP data sets were statistically analyzed using the paired t-test to determine the significance of the temperature effects at the in situ (Δ0°C) and elevated (Δ+6°C) temperatures. The significance level was set at p < 0.05 (IBM SPSS Statistics 20). To compare the dominant taxa between stations, the relative abundances of the >75% most abundant OTUs (>40 reads) were visualized in a heatmap. Explicet (Robertson et al., 2013) was used to estimate richness and Shannon diversity for the eight stations. Bacterial OTUs that significantly differed in their relative abundances between stations were identified using a linear discriminant analysis effect size (LEfSe) analysis (Segata et al., 2011) with the default settings, except the “One against all” strategy, for multi-class analysis.
Results
In situ Conditions of the Baltic Sea
During the cruise in early March, the in situ water temperatures were between 0.1° and 3.2°C (Figure 1, Table 1). Within the first part of the transect (hereafter called “northern stations”), extending from the Gulf of Finland (station 1) to those in the central Baltic Sea (stations 3–4), there was no indication of an ongoing phytoplankton spring bloom. Phytoplankton biomass (5.1–39.2 μg C L−1) (Figure 2A, Table 1) and production (Figure 3A) levels in the surface waters were very low, with no sign of nutrient depletion (Table 1). The phytoplankton community was dominated by the dinoflagellate Scrippsiella sp., which at station 1, for example, contributed ~95% to total phytoplankton biomass (Figure 2A, Table S2). Several large ciliates (mainly Lohmaniella sp.) were also identified. By contrast, at stations in the southern Baltic Sea (5–8), developing phytoplankton blooms were evidenced by biomass and PP levels up to 10 times higher than at the northern stations (PP: 180–330 vs. 2–20 μg C L−1 day−1, respectively; Figures 2A, 3A) and by the depletion of nutrient concentrations, particularly nitrate (Table 1). The phytoplankton communities at the southern stations were dominated by the diatom Skeletonema costatum (59–93% of phytoplankton biomass), with minor contributions by the dinoflagellate Gymnodinium ssp. and other diatoms (Chaetoceros spp., Thalassiosira rotula) (Figure 2A). Overall, 26 different phytoplankton species within nine phyla (e.g., Dinophyta or Heterokontophyta) were detected over the whole transect (Table S2).
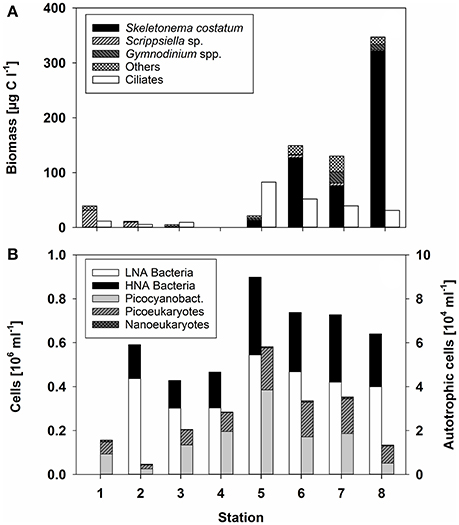
Figure 2. (A) Biomass of the dominant phytoplankton taxa or taxonomic groups, as well as that of ciliates (μg C L−1) according to the different stations. No data were obtained for station 4. (B) Bacterial abundance (106 mL−1) at the different stations. Heterotrophic bacteria were distinguished according to their low and high nucleic acid (LNA and HNA) content. Autotrophic cells, determined by flow cytometry, are divided into Synechococcus, picoeukaryotes, and nanoeukaryotes (gray bars).
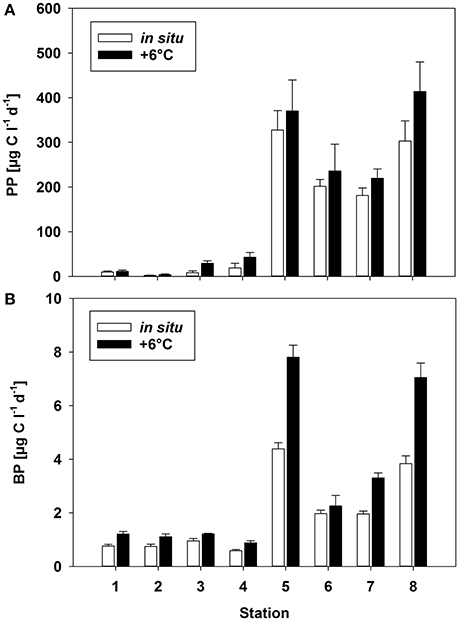
Figure 3. (A) Primary production (PP) and (B) bacterial production (BP) rates [μg C L−1 days−1] at two different temperatures (Δ0°C and Δ+6°C) at the different stations. Mean (±SD) of three replicate measurements.
Heterotrophic bacterial abundance was also higher at the southern (0.64–0.90 × 106 cells mL−1) than at the northern (0.43–0.59 × 106 cells mL−1) stations but the increase was relatively modest (Figure 2B). HNA bacteria accounted for 0.13–0.16 × 106 cells mL−1 at the northern and 0.24–0.35 × 106 cells mL−1 at the southern stations (average 30 ± 4% and 39 ± 3% of total prokaryotes, respectively) (Figure 2B). The difference in BP was much stronger, as the rates measured at the southern stations were 8-fold higher (Figure 3B). Picocyanobacteria (mainly Synechococcus), picoeukaryotes, and nanoeukaryotes, enumerated by flow cytometry, did not show a similarly clear pattern. Their abundance was highest at station 5 and lowest at station 2 (Figure 2B).
Temperature Effect on Bacteria-Phytoplankton Coupling
The experimental temperature rise of △+6°C resulted in a significant increase in PP at all stations (paired t-test, p = 0.008, n = 8) (Figure 3A). This warming-dependent increase in PP was recorded for incubations at 100 and 75% light intensity, while at 50% light intensity PP remained almost constant (Figure S3). The temperature increase of △+6°C led to a stronger relative increase in PP at stations with a low phytoplankton biomass and a low rate of PP (stations 1–4; up to 250% at station 3) than at stations with high PP rates (stations 5–8). The increase at the latter stations during the incubation period was only 20% on average (Figure 3A, Table 2), with the strongest increase (36%) at station 8. The calculated Q10 values were in the range of 1.2–1.7 for the southern, high-PP stations and 1.3–8.2 for the northern, low-PP stations (Figure 2A; Table 2). However, for the northern stations these values should be interpreted with caution as the PP values were very low and the measured increases were in some cases close to the detection limit.
With a warming of Δ+6°C, BP also increased significantly (paired t-test, p = 0.008, n = 8), although with a high variability (mean increase 59 ± 31%) (Figure 3B). At both the northern and the southern stations BP increased by 2- to 4-fold (Figure 3B, Table 2). The resulting Q10 for bacterial production ranged between 1.1 and 2.8 (mean 2.2 ± 0.7) at high-PP stations (5–8) and were comparable with BP at low-PP stations which ranged between 1.5 and 2.2 (mean 1.9 ± 0.3 (Table 2). The calculated ratios of BP/PP and BCD/PP were much lower at the southern stations (5–8), where the phytoplankton blooms had developed, than at the northern non-bloom stations (1–4) (Table 2). This was independent of the chosen model for calculating BCD (Table 2). The experimental temperature increase did not result in significantly different ratios of BP/PP and BCD/PP at any of the northern stations (paired t-test, p = 0.798, n = 8) (Table 2). However, for the southern stations only, warming resulted in clearly increased BP/PP and BCD/PP ratios.
Bacterial Community Composition
The northern stations (1–4) of the Baltic Sea were dominated by Actinobacteria (13.4–26.7% of the total sequences), Alphaproteobacteria (18.7–24.5%), and Bacteroidetes (10.5–13.9%) (Figure 4). At the southern stations (5–8), where the diatom-dominated phytoplankton spring bloom occurred, the same phyla/classes predominated but the proportion of Bacteroidetes was much larger (15.5–40%). The contribution of Cyanobacteria (mainly Synechococcus) was maximal at station 5 (17.9%) and decreased with increasing phytoplankton biomass in the southern Baltic Sea (stations 7 and 8). This pattern was consistent with the cell counts of picocyanobacteria determined by flow cytometry (Figure 2B). Compared to the northern stations (1–4), the proportions of Bacteroidetes and Gammaproteobacteria nearly doubled at the southern stations (5–8), whereas those of Betaproteobacteria, Planctomycetes, and Verrucomicrobia decreased (Figure 4). In addition, the bacterial α-diversity decreased with increasing phytoplankton biomass (number of bacterial genera, Shannon index; Figure S2).
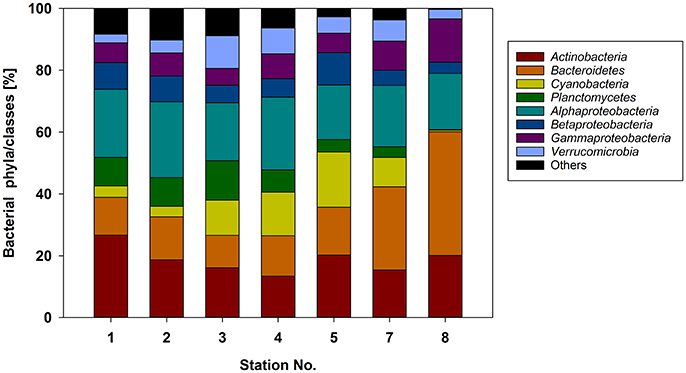
Figure 4. Relative abundance of the major bacterial phyla/classes at the different stations sampled based on 16S rRNA gene analysis.
Among the 75% most abundant bacterial OTUs, LEfSe analysis identified 14 that differed significantly between stations with a high (stations 5–8) and low (stations 1–4) phytoplankton biomass (Figure 5). In the phytoplankton-dominated samples of the southern stations, several OTUs belonging to Flavobacteria (unclassified Cryomorphaceae, Ulvibacter, unclassified Flavobacteriaceae) were particularly abundant, with OTU NS3a reaching a relative abundance of 16.8% at station 8 (Figure 5). LEfSe analysis also identified the enrichment of Candidatus Aquiluna (Actinobacteria) as well as representatives from the unclassified PeM15 group (Actinobacteria) and the BAL58 group (Betaproteobacteria) at the southern stations. The dominant OTUs from the northern stations were more diverse and included representatives from the Alphaproteobacteria, Betaproteobacteria, Acidobacteria, and Chloroflexi but also from the flavobacterial NS9 group. Additionally, several OTUs occurred in higher abundance at all eight of the investigated stations (Figure 5).
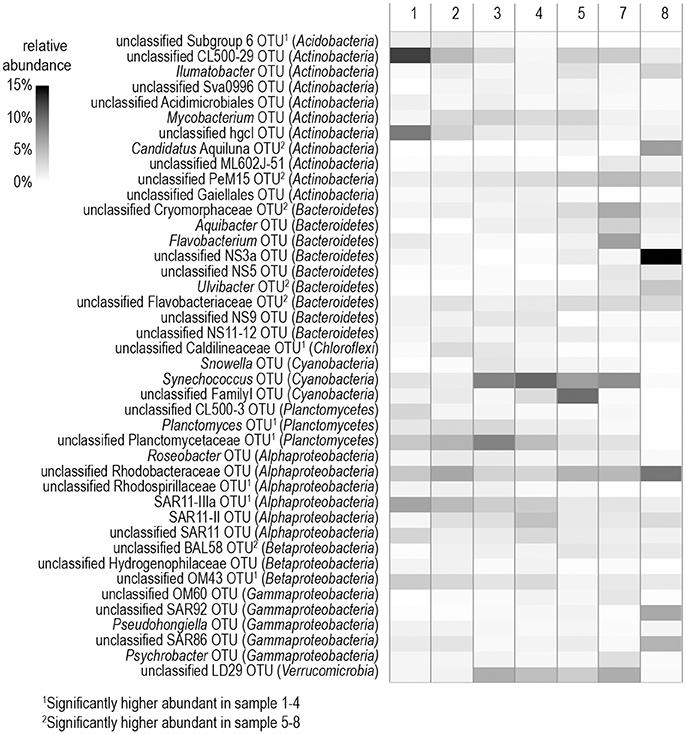
Figure 5. Heatmap of the 75% most abundant operational taxonomic units (OTUs) detected in samples from the different stations. The affiliation with the respective phylum is shown in parentheses.
Discussion
Early Spring Plankton Development in the Baltic Sea
The Baltic Sea is characterized by annual phytoplankton spring blooms. These occur during late February to early April in the southern parts but may last into May in the northern parts (Groetsch et al., 2016). Sufficient light is essential for the onset of phytoplankton blooms. Diminished vertical mixing and the associated earlier onset of thermal stratification depend on the balance of surface warming and wind energy. In the Baltic Sea, there is a strong inter-annual variation in the springtime water temperature, which ranges between −1° and +5°C (HELCOM, 2013). In the northern Baltic, low water temperatures prevent thermal stratification such that the phytoplankton spring bloom generally does not start before April (Spilling and Markager, 2008). However, short-term warming periods can result in a temporal stratification, warmer surface temperatures, and the initiation of smaller, earlier phytoplankton blooms (e.g., Wasmund et al., 1998). Recent trends of ongoing sea surface warming have already been shown to impact the phytoplankton spring bloom in the Baltic Sea, in the form of earlier bloom onsets and changes in community composition (Klais et al., 2011, 2013; Wasmund et al., 2011). For example, during recent winter periods, the earlier stratification of the water column due to the increasing sea surface temperature frequently led to changes in phytoplankton composition and abundance, such that the proportions of cold-water species, including Diatomophyceae, declined and those of warm-water species, such as Dinophyceae and Cyanophyceae, increased.
Our study missed the beginning of the early spring bloom at the northern Baltic Sea stations, where we encountered pre-bloom conditions, but it did encompass a typical diatom-dominated spring bloom (mainly Skeletonema costatum), which developed in late February and reached a peak in early March (Wasmund et al., 2013; Groetsch et al., 2016), at the sea's southern stations. Hence, this study detected the normal phytoplankton spring succession, whereby stations 5–7 probably covered the initial phase and station 8 the maximum of the spring bloom. Here the diatoms were probably already experiencing nutrient limitation, especially by nitrate availability (Table 1). This high phytoplankton biomass of 347 μg C L−1 at station 8 was in accordance with previous phytoplankton peaks observed during springtime in the Baltic Sea (e.g., Wasmund et al., 2008). The dominance of one or few taxa during the phytoplankton spring blooms in the Baltic Sea, as observed here for Skeletonema costatum (e.g., ~95% of total phytoplankton biomass at station 8) is also a typical feature (Wasmund et al., 2013). Phytoplankton provides labile organic carbon, either directly through the exudation of DOC (which resembles dissolved PP) or indirectly through grazers or viral lysis, thereby activating the bacterioplankton community and triggering the growth of adapted bacterial taxa (Sarmento and Gasol, 2012; Teeling et al., 2012; Wear et al., 2015). However, at very low temperatures bacterial growth can be suppressed or delayed despite organic matter production by the phytoplankton spring blooms (Pomeroy and Deibel, 1986). The latter presumption has been challenged by studies that demonstrated considerable bacterial activity at low temperatures (Yager et al., 2001; Kirchman et al., 2009). Interactive effects between temperature and substrate supply complicate predictions of the response to temperature by bacterioplankton communities (Pomeroy and Wiebe, 2001; Hall and Cotner, 2007; Kritzberg et al., 2010).
In our field study, where the temperature at all stations was in the 0–3°C range, activation of the bacterial communities by blooming phytoplankton was apparent at stations with high PP (Figures 2B, 3B). Here, increases in bacterial abundance and production, as well as in the proportion of HNA bacteria, a common indicator of more active bacterial cells (Gasol et al., 1999), were recorded. A similar temperature experiment revealed that in situ BP and BR were positively related to temperature but BR responded more strongly to temperature than BP, indicating that increased temperature may result in a higher bacterial carbon demand and decreased growth efficiency (Kritzberg et al., 2010). Thus, overall there is considerable evidence that increasing temperature results in a higher carbon turnover in surface waters during phytoplankton spring blooms.
At the level of the main phylogenetic phyla/classes, there were only minor differences in bacterial community composition (BCC) across the stations sampled (Figure 4) whereas larger differences were visible at the genus level based OTU composition (Figure 5). Although salinity is a dominant factor for BCC in the Baltic Sea, major shifts occur when salinities drop to < 3 or rise to >10 (Herlemann et al., 2011). Modest changes in main bacterial phyla in our study area can be explained by the fact that only stations in a salinity range of 5.2–10 were covered. Temperature is another strong factor for the BCC, but since the conditions of the sampled stations were also relatively similar in terms of temperature (0–3°C), we assume that the presence of phytoplankton was probably the main driver for the differences in the dominating OTUs at the different stations. Both bacterial richness (here, the number of different OTUs) and the Shannon index decreased with increasing phytoplankton biomass (Figure S2), indicating that adapted bacteria became more dominant. OTUs belonging to Gammaproteobacteria and Bacteroidetes, which became more dominant at the stations with high phytoplankton biomass, are characteristic for diatom-dominated phytoplankton blooms (Teeling et al., 2012; Buchan et al., 2014; Bunse et al., 2016). For example, at station 8 with the phytoplankton bloom peak, one OTU of the NS3a marine group within the Flavobacteriales dominated. The same taxon occurred in high abundance in a previous mesocosm experiment with a comparable diatom-dominated phytoplankton bloom (clone-157; 16% of all 16S rRNA clones; von Scheibner et al., 2014) as well as in another field study carried out in the same area of the Baltic Sea during a spring bloom (OTU_000022; 16% of all 16S rRNA gene sequences; Bunse et al., 2016). This suggests a key role for this flavobacterial taxa in carbon processing during early diatom spring blooms in the Baltic Sea. Most of the other abundant OTUs in our study differed from those reported by Bunse et al. (2016), perhaps due to the fact that those authors encountered a higher phytoplankton biomass, with a different phytoplankton composition in the northern Baltic than was the case in our study. Generally, the OTUs that were abundant during the phytoplankton bloom at station 8 were more closely related to the OTUs from the southern Baltic Sea in the study of Bunse et al. (2016).
Phyto-Bacterioplankton Coupling and the Impact of Warming
Although bacterial abundance and production were elevated at the southern stations (5–8), characterized by high PP rates, proportionally much stronger increases in phytoplankton and lower BP/PP ratios (1.0%–1.3%) than generally known for phytoplankton blooms were recorded. Despite our measurements of PP are probably only rough estimates of daily production, as changes in light and radiation could not be considered, and PP may change from day to day, we do not think that more extensive PP measurements would have provided very different BP/PP ratios. For example, the incubations at stations 1, 5 and 8 started later in the morning, and higher radiance over midday may have resulted in higher PP values, BP increased equivalent to PP, resulting in comparable BP/PP values. The Q10 values have to be interpreted with caution as the calculation was based on only two temperatures. However, the obtained Q10 values are well within the range of reported values from experimental warming experiments.
According to global observations, water temperature correlates positively with BP/PP ratios, which increase from 2 to 10% in cold and temperate climate zones up to roughly 40% at lower latitudes (Carlson and Ducklow, 1996; Ducklow et al., 1999; Hoppe et al., 2002). This suggests that the low temperature in our study area suppressed bacterial growth and therefore prevented stronger phytoplankton-bacteria coupling. The low BP/PP ratios are comparable to those reported in other studies of low temperature waters, in which ratios < 10% were interpreted as evidence of “uncoupled” BP and PP (Cole et al., 1988; Nielsen and Richardson, 1989). The low water temperatures during early spring blooms in the Baltic Sea probably delay the bacterial degradation of phytoplankton-derived organic matter and may result in the gradual temporal accumulation of DOC. A temporal delay of bacteria-phytoplankton coupling is often recorded at these low water temperatures, since the bacterioplankton peak follows the phytoplankton bloom peak often with a delay of 1–2 weeks and could be a critical factor for the low bacterial abundance and production in this study (e.g., Hoppe et al., 2008). Furthermore, high grazing pressure is often observed for controlling the bacterial growth during phytoplankton spring blooms at low temperatures and could be another factor for the reduction in phytoplankton-bacteria coupling (Lignell et al., 1992; von Scheibner et al., 2014).
Bacterial growth is more dependent on dissolved primary production (DPP) than on particulate primary production (PPP) (e.g., Morán et al., 2002). Data concerning the percent extracellular release (PER) of PP in the Baltic Sea during phytoplankton spring blooms are scarce, but studies from other systems indicate that PER is usually < 20% of total PP (Nagata, 2000; Teira et al., 2001; Marañón, 2005; Morán et al., 2013). At a PER of 20%, the estimated BCD at the northern stations would exceed DPP whereas at the southern stations BCD could be entirely fueled by DPP. However, other mechanisms also contribute to the transfer of phytoplankton carbon to bacteria, such as sloppy feeding by zooplankton or viral lysis. As we did not measure DPP, we used PPP as a proxy for phytoplankton organic carbon production, which can fuel the microbial loop. Although BP/PP ratios are insufficient to completely describe the pelagic carbon flow, they are indicators of the current relation between autotrophic production and heterotrophic consumption of organic matter. Including measurements of DPP, bacterial respiration, and grazing rates in assessments of the carbon flow during the phytoplankton spring bloom would lead to more precise estimates but probably would not change the overall finding of low phytoplankton-bacteria coupling.
Warming (Δ+6°C) stimulated both PP and BP, with distinctly higher average Q10 values for BP (2.1 ± 0.7) than for PP (1.4 ± 0.2) during phytoplankton bloom conditions at the southern stations (Table 2). This positive effect of higher temperature on bacterial production rates is in agreement with many previous studies in the Baltic Sea and other marine locations in this climate zone (Vázquez-Domínguez et al., 2007; Hoppe et al., 2008; Panigrahi et al., 2013). However, in some studies the Q10 for BP even exceeded the normal range of 2–3 known for heterotrophic organisms during phytoplankton spring blooms (Vaquer-Sunyer et al., 2015). PP increased significantly with higher temperatures in all samples, resulting in Q10 values between 1.2 and 8.2 (Table 2). These were within the range reported in other field studies (e.g., Panigrahi et al., 2013) and in marine mesocosm experiments in the Baltic Sea (Hoppe et al., 2008; von Scheibner et al., 2014). In our study, the warming-related increase in PP suggested light saturation of phytoplankton, since light-limited rates of PP are insensitive to temperature changes (Tilzer et al., 1986), as also shown in mesocosm studies of marine plankton (e.g., Lewandowska and Sommer, 2010; Lewandowska et al., 2012; Sommer et al., 2012). Q10 values between 1.2 and 1.7 at stations with high PP rates indicated that short-term warming did not greatly disturb biochemical processes. In addition, these values are in accordance with those of previous studies demonstrating lower temperature-sensitivity of phytoplankton growth and production (Marañón et al., 2014). A more detailed picture would be obtained by measuring not only the response of particulate but also that of dissolved primary production (e.g., Morán et al., 2006) as this is expected to be more related to bacterial substrate utilization. However, other temperature-sensitive factors, such as grazing pressure (Duarte et al., 2005; Aberle et al., 2007), qualitative and quantitative aspects of phytoplankton exudation (Zlotnik and Dubinsky, 1989; Morán et al., 2006), and substrate affinity (Nedwell, 1999), can strongly interfere with phytoplankton-bacteria interactions and will have to be considered for a more comprehensive understanding of the effects of temperature. Their inclusion would also help to explain the absence of a temperature effect on the BCD/PP ratio in samples obtained from the non-bloom conditions of the northern Baltic Sea (stations 1–4). In these waters, temperature more strongly stimulated PP than BP, such that carbon flow to heterotrophic decomposers was reduced by ~1% per 1°C. The high proportion of Dinophyceae (e.g., Scrippsiella sp.), which often establish a high biomass at low temperature in the northern Baltic Sea (Kremp, 2000; Kremp et al., 2008), may have been responsible for the reduced carbon flow to bacterioplankton. However, at these very low PP and BP rates, carbon budget calculations should be interpreted with caution, since small changes, close to the detection limit, nonetheless strongly impact BP/PP ratios.
Comparison of Field Data With Experimental Warming Experiments
Our results from the Baltic Sea are consistent with those of other field studies performed at a similar cold temperature range (Hoppe et al., 2002; Morán et al., 2002; Duarte et al., 2005). They suggest that only a small fraction of the fixed carbon of phytoplankton enters the heterotrophic bacterial community during early bloom conditions at cold temperatures. Previous mesocosm studies of Baltic Sea spring communities, examined at a temperature range similar to that tested in the present work, showed that BP during phytoplankton spring bloom conditions was strongly stimulated by warming (Wohlers et al., 2009; von Scheibner et al., 2014), leading to a closer temporal coupling of autotrophic and heterotrophic processes as the temperature rose (Wohlers-Zöllner et al., 2012). The mean Q10 of 2.2 for the bacterial response in our study was nearly identical to that reported in a previous experimental warming experiment (Q10 = 2.4) (Hoppe et al., 2008).
However, bacterial stimulation by warming was much less in this field study than in previous mesocosm studies mentioned above, as BP/PP ratios were low (0.9–2.1%) even during the diatom blooms at the southern stations (Table 2), implying that BP was largely uncoupled from PP also at the elevated experimental temperatures. By contrast, in the mesocosm experiments the BCD/PP ratio during the bloom peak was ~19% at the in situ temperature (~2°C) and ~24% in response to a warming of +6°C (von Scheibner et al., 2014). Major differences with our field study were the higher phytoplankton biomass (1,000–1,500 μg C L−1) and PP in the mesocosm experiments (Hoppe et al., 2008; Wohlers et al., 2009; Lewandowska and Sommer, 2010), significant grazing by mesozooplankton and a longer duration. This might have provided higher substrate supply from phytoplankton origin for heterotrophic bacteria. Several studies already highlighted that bacterial growth is rather depended on the supply of dissolved PP than to particulate PP. Moreover, in experimental warming study with slight temperature increase of 2°C resulted in massive increase of dissolved PP, whereas the particulate PP nearly stable (Morán et al., 2006). In the more unstable physical conditions of the Baltic Sea (e.g., higher vertical mixing or drifting), phytoplankton biomass is much lower (Table 1). This could explain the differences in the carbon flow patterns between the mesocosm experiments vs. in situ conditions in our study. Another reason for the distinct differences in phyto-bacterioplankton coupling might be that the duration of warming in this study was not sufficient to allow acclimatization of the bacterial communities to a similar extent as in the mesocosm studies, which were generally conducted for several weeks.
Conclusions
Overall, the appearance of diatom-dominated phytoplankton blooms in the southern Baltic Sea led to a strong activation of the bacterial communities, in contrast to the northern Baltic Sea, where the phytoplankton biomass was low and dominated by dinoflagellates. The experimental temperature increase during the phytoplankton bloom significantly enhanced both PP and, especially, BP. This partially confirmed our hypothesis and previously published considerations that warming results in a stronger stimulation of bacterioplankton than of phytoplankton communities under cold-water conditions. However, according to the low BP/PP ratios, bacteria were still relatively uncoupled from phytoplankton growth, a situation that remained essentially unchanged after a temperature increase of 6°C. This is in contrast to results from previous mesocosm experiments and suggests that during the early spring bloom in the Baltic Sea increased sea surface temperatures will not strengthen bacterio-phytoplankton coupling to a similar extent as in mesocosm experiments. The clear differences in carbon flow pattern between field conditions and experimental studies could be due to different factors such as physical conditions, food web structure and acclimation, which remain to be examined. The temporal response patterns of microbial communities to warming as well as possible bacterial adaptations are important avenues for future field and experimental research. By careful comparisons of the results of experimental mesocosm with those of in situ studies, including the different time scales, a more realistic picture of the effects of global warming on the marine carbon budget will be obtained.
Author Contributions
MvS and KJ planned, initiated and conducted the study and wrote the first draft of the manuscript. All authors discussed the results and edited the manuscript. DH analyzed bacterial community composition and AL assessed phytoplankton composition and biomass.
Funding
This work was funded by the German Science Foundation (DFG), grants JU367/7-3 (priority program AQUASHIFT) and JU 367/15-1 to KJ. DH was supported by the grant MOBTT24 (Estonian Research Council, and European Union).
Conflict of Interest Statement
The authors declare that the research was conducted in the absence of any commercial or financial relationships that could be construed as a potential conflict of interest.
Acknowledgments
We thank the crew and captain of the RV Alkor (AL332) for support during the cruise, and Marco Nack for technical assistance during the incubation experiments, as well as Annett Grüttmüller for flow cytometry analysis.
Supplementary Material
The Supplementary Material for this article can be found online at: https://www.frontiersin.org/articles/10.3389/fmars.2018.00231/full#supplementary-material
References
Aberle, N., Lengfellner, K., and Sommer, U. (2007). Spring bloom succession, grazing impact and herbivore selectivity of ciliate communities in response to winter warming. Oecologia 150, 668–681. doi: 10.1007/s00442-006-0540-y
Azam, F. (1998). Microbial control of oceanic carbon flux: the plot thickens. Science 280, 694–696. doi: 10.1126/science.280.5364.694
Boyd, P. W., and Doney, S. C. (2002). Modelling regional responses by marine pelagic ecosystems to global climate change. Geophys. Res. Lett. 29, 53-1–53-4. doi: 10.1029/2001GL014130
Brown, J. H., Gillooly, J. F., Allen, A. P., Savage, V. M., and West, G. B. (2004). Toward a metabolic theory of ecology. Ecology 85, 1771–1789. doi: 10.1890/03-9000
Buchan, A., LeCleir, G. R., Gulvik, C. A., and González, J. M. (2014). Master recyclers: features and functions of bacteria associated with phytoplankton blooms. Nat. Rev. Microb. 12, 686–698. doi: 10.1038/nrmicro3326
Bunse, C., Bertos-Fortis, M., Sassenhagen, I., Sildever, S., Sjoqvist, C., Godhe, A., et al. (2016). Spatio-temporal interdependence of bacteria and phytoplankton during a Baltic Sea spring bloom. Front. Microbiol. 7:517.
Caporaso, J., Kuczynski, J., Stombaugh, J., Bittinger, K., Bushman, F. D., Costello, E. K., et al. (2010). QIIME allows analysis of high-throughput community sequencing data. Nat. Methods 7, 335–336. doi: 10.1038/nmeth.f.303
Carlson, C. A., and Ducklow, H. W. (1996). Growth of bacterioplankton and consumption of dissolved organic carbon in the Sargasso Sea. Aquat. Microb. Ecol. 10, 69–85. doi: 10.3354/ame010069
Cole, J. J., Findlay, S., and Pace, M. L. (1988). Bacterial production in fresh and saltwater ecosystems: a cross-system overview. Mar. Ecol. Prog. Ser. 43, 1–10. doi: 10.3354/meps043001
Degerman, R., Dinasquet, J., Riemann, L., Sjöstedt de Luna, S., and Andersson, A. (2013). Effect of resource availability on bacterial community responses to increased temperature. Aquat. Microb. Ecol. 68, 131–142. doi: 10.3354/ame01609
del Giorgio, P. A., and Cole, J. J. (1998). Bacterial growth efficiency in natural aquatic systems. Annu. Rev. Ecol. Syst. 29, 503–541. doi: 10.1146/annurev.ecolsys.29.1.503
del Giorgio, P. A., Cole, J. J., and Cimbleris, A. (1997). Respiration rates in bacteria exceed phytoplankton production in unproductive aquatic systems. Nature 385, 148–151. doi: 10.1038/385148a0
Duarte, C. M., Agust,í, S., Vaqué, D., Agawin, N. S. R., Felipe, J., Casamayor, E. O., et al. (2005). Experimental test of bacteria-phytoplankton coupling in the Southern Ocean. Limnol. Oceanogr. 50, 1844–1854. doi: 10.4319/lo.2005.50.6.1844
Ducklow, H. W. (2000). “Bacterial production and biomass in the oceans,” in Microbial Ecology of the Oceans, ed D. L. Kirchman (New York, NY: Wiley), 85–120.
Ducklow, H. W., Carlson, C. A., and Smith, W. O. (1999). Bacterial growth in experimental plankton assemblages and seawater cultures from the Phaeocystis antarctica bloom in the Ross Sea, Antarctica. Aquat. Microb. Ecol. 19, 215–227. doi: 10.3354/ame019215
Edwards, K. F., Thomas, M. K., Klausmeier, C. A., and Litchman, E. (2016). Phytoplankton growth and the interaction of light and temperature. Synth. Species Community Level. Limnol. Oceanogr. 61, 1232–1244. doi: 10.1002/lno.10282
Eriksson Wiklund, A. K., Dahlgren, K., Sundelin, B., and Andersson, A. (2009). Effects of warming and shifts of pelagic food web structure on benthic productivity in a coastal marine system. Mar. Ecol. Prog. Ser. 396, 13–25. doi: 10.3354/meps08290
Field, C. B., Behrenfeld, M. J., Randerson, J. T., and Falkowski, P. G. (1998). Primary production of the biosphere. Integr. Terres. Oceanic Compon. Sci. 281, 237–240. doi: 10.1126/science.281.5374.237
Gasol, J. M., and del Giorgio, P. A. (2000). Using flow cytometry for counting natural planktonic bacteria and understanding the structure of planktonic bacterial communities. Sci. Mar. 64, 197–224. doi: 10.3989/scimar.2000.64n2197
Gasol, J. M., Zweifel, U. L., Peters, F., Fuhrman, J. A., and Hagström, Å. (1999). Significance of size and nucleic acid content heterogeneity as measured by flow cytometry in natural planktonic bacteria. Appl. Environ. Microbiol. 65, 4475–4483.
Gragas, E. (1975). A manual for phytoplankton primary production studies in the Baltic. Baltic Marine Biol. 3, 1–88.
Grasshoff, K., Kremling, K., and Ehrhardt, M. (1999). Methods of Seawater Analysis. Weinheim: Wiley-VCH Verlag GmbH.
Groetsch, P. M. M., Simis, S. G. H., Eleveld, M. A., and Peters, S. W. M. (2016). Spring blooms in the Baltic Sea have weakened but lengthened from 2000 to 2014. Biogeoscience 13, 4959–4973. doi: 10.5194/bg-13-4959-2016
Hall, E. K., and Cotner, J. B. (2007). Interactive effect of temperature and resources on carbon cycling by freshwater bacterioplankton communities. Aquat. Microb. Ecol. 49, 35–45. doi: 10.3354/ame01124
Harris, L. A., Duarte, C. M., and Nixon, S. W. (2006). Allometric laws and prediction in estuarine and coastal ecology. Estuar. Coasts 29, 340–344. doi: 10.1007/BF02782002
HELCOM (2013). “Climate change in the Baltic Sea Area: HELCOMthematic assessment in 2013,” in Balt. Sea Environ. Proc. No. 137.
Herlemann, D. P. R., Labrenz, M., Jürgens, K., Bertilsson, S., Waniek, J. J., and Andersson, A. F. (2011). Transitions in bacterial communities along the 2000 km salinity gradient of the Baltic Sea. ISME J. 5, 1571–1579. doi: 10.1038/ismej.2011.41
Herlemann, D. P. R., Lundin, D., Andersson, A. F., Labrenz, M., and Jürgens, K. (2016). Phylogenetic signals of salinity and season in bacterial community composition across the salinity gradient of the Baltic Sea. Front. Microbiol. 7:1883. doi: 10.3389/fmicb.2016.01883
Hoppe, H.-G., Breithaupt, P., Walther, K., Koppe, R., Bleck, S., Sommer, U., et al. (2008). Climate warming in winter affects the coupling between phytoplankton and bacteria during the spring bloom. A mesocosm study. Aquat. Microb. Ecol. 51, 105–115. doi: 10.3354/ame01198
Hoppe, H.-G., Gocke, K., Koppe, R., and Begler, C. (2002). Bacterial growth and primary production along a north-south transect of the Atlantic Ocean. Nature 416, 168–171. doi: 10.1038/416168a
IPCC (2013). Intergovernmental Panel on Climate Change 2013 - The Physical Science Basis. Cambridge: Cambridge University Press.
Keller, A. A., Oviatt, C. A., Walker, H. A., and Hawk, J. D. (1999). Predicted impacts of elevated temperature on the magnitude of the winter-spring phytoplankton bloom in temperate coastal waters. A mesocosm study. Limnol. Oceanogr. 44, 344–356. doi: 10.4319/lo.1999.44.2.0344
Kirchman, D. L. (2001). Measuring bacterial biomass production and growth rates from leucine incorporation in natural aquatic environments. Meth. Microbiol. 30, 227–237. doi: 10.1016/S0580-9517(01)30047-8
Kirchman, D. L., Morán, X. A. G., and Ducklow, H. W. (2009). Microbial growth in the polar oceans - role of temperature and potential impact of climate change. Nat. Rev. Microbiol. 7, 451–459. doi: 10.1038/nrmicro2115
Klais, R., Tamminen, T., Kremp, A., Spilling, K., and Olli, K. (2011). Decadal-scale changes of dinoflagellates and diatoms in the anomalous Baltic Sea spring bloom. PLoS ONE 6:e21567. doi: 10.1371/journal.pone.0021567
Klais, R., Tamminen, T., Kremp, A., Spilling, K., and Woong, A. B. (2013). Spring phytoplankton communities shaped by interannual weather variability and dispersal limitation. Mechanisms of climate change effects on key coastal primary producers. Limnol. Oceanogr. 58, 753–762. doi: 10.4319/lo.2013.58.2.0753
Klindworth, A., Pruesse, E., Schweer, T., Peplies, J., Quast, C., Horn, M., et al. (2013). Evaluation of general 16S ribosomal RNA gene PCR primers for classical and next-generation sequencing-based diversity studies. Nucleic Acids Res. 41, e1. doi: 10.1093/nar/gks808
Kremp, A. (2000). Factors regulating germination of resting cysts of the spring bloom dinoflagellate Scrippsiella hangoei from the northern Baltic Sea. J. Plankton Res. 22, 1311–1327. doi: 10.1093/plankt/22.7.1311
Kremp, A., Tamminen, T., and Spilling, K. (2008). Dinoflagellate bloom formation in natural assemblages with diatoms. Nutrient competition and growth strategies in Baltic spring phytoplankton. Aquat. Microb. Ecol. 50, 181–196. doi: 10.3354/ame01163
Kritzberg, E. S., Duarte, C. M., and Wassmann, P. (2010). Changes in Arctic marine bacterial carbon metabolism in response to increasing temperature. Polar Biol. 33, 1673–1682. doi: 10.1007/s00300-010-0799-7
Lewandowska, A. M., Breithaupt, P., Hillebrand, H., Hoppe, H.-G., Jürgens, K., and Sommer, U. (2012). Responses of primary productivity to increased temperature and phytoplankton diversity. J. Sea Res. 72, 87–93. doi: 10.1016/j.seares.2011.10.003
Lewandowska, A. M., and Sommer, U. (2010). Climate change and the spring bloom. A mesocosm study on the influence of light and temperature on phytoplankton and mesozooplankton. Mar. Ecol. Prog. Ser. 405, 101–111. doi: 10.3354/meps08520
Li, W., and Godzik, A. (2006). Cd-hit: a fast program for clustering and comparing large sets of protein or nucleotide sequences. Bioinformatics 22, 1658–1659. doi: 10.1093/bioinformatics/btl158
Lignell, R., Kaitala, S., and Kuosa, H. (1992). Factors controlling phyto- and bacterioplankton in late spring on a salinity gradient in the northern Baltic. Mar. Ecol. Prog. Ser. 84, 121–131. doi: 10.3354/meps084121
Lindh, M. V., Riemann, L., Baltar, F., Romero-Oliva, C., Salomon, P. S., Granéli, E., et al. (2012). Consequences of increased temperature and acidification on bacterioplankton community composition during a mesocosm spring bloom in the Baltic Sea. Environ. Microbiol. 5, 252–262. doi: 10.1111/1758-2229.12009
López-Urrutia, Á., San Martin, E., Harris, R. P., and Irigoien, X. (2006). Scaling the metabolic balance of the oceans. Proc. Natl. Acad. Sci. U.S.A. 103, 8739–8744. doi: 10.1073/pnas.0601137103
Marañón, E. (2005). Phytoplankton growth rates in the Atlantic subtropical gyres. Limnol. Oceanogr. 50, 299–310. doi: 10.4319/lo.2005.50.1.0299
Marañón, E., Cermeño, P., Huete-Ortega, M., López-Sandoval, D. C., Mouriño-Carballido, B., and Rodríguez-Ramos, T. (2014). Resource supply overrides temperature as a controlling factor of marine phytoplankton growth. PLoS ONE 9:e99312. doi: 10.1371/journal.pone.0099312
Morán, X. A. G., Ducklow, H. W., and Erickson, M. (2013). Carbon fluxes through estuarine bacteria reflect coupling with phytoplankton. Mar. Ecol. Prog. Ser. 489, 75–85. doi: 10.3354/meps10428
Morán, X. A. G., Estrada, M., Gasol, J. M., and Pedrós-Ali,ó, C. (2002). Dissolved primary production and the strength of phytoplankton- bacterioplankton coupling in contrasting marine regions. Microb. Ecol. 44, 217–223. doi: 10.1007/s00248-002-1026-z
Morán, X. A. G., Sebastián, M., Pedrís-Alií, C., and Estrada, M. (2006). Response of Southern Ocean phytoplankton and bacterioplankton production to short-term experimental warming. Limnol. Oceanogr. 51, 1791–1800. doi: 10.4319/lo.2006.51.4.1791
Müren, U., Berglund, J., Samuelsson, K., and Andersson, A. (2005). Potential effects of elevated sea-water temperature on pelagic food webs. Hydrobiologia 545, 153–166. doi: 10.1007/s10750-005-2742-4
Nagata, T. (2000). “Production mechanisms of dissolved organic matter,” in Microbial Ecology of the Oceans, ed D. L. Kirchman (New York, NY: Wiley), 121–152.
Nedwell, D. (1999). Effect of low temperature on microbial growth: lowered affinity for substrates limits growth at low temperature. FEMS Microbiol. Ecol 30, 101–111. doi: 10.1111/j.1574-6941.1999.tb00639.x
Nielsen, T. G., and Richardson, K. (1989). Food chain structure of the North Sea plankton communities: seasonal variations of the role of the microbial loop. Mar. Ecol. Prog. Ser. 56, 75–87. doi: 10.3354/meps056075
O'Connor, M. I., Piehler, M. F., Leech, D. M., Anton, A., and Bruno, J. F. (2009). Warming and resource availability shift food web structure and metabolism. PLoS Biol. 7:e1000178. doi: 10.1371/journal.pbio.1000178
Olenina, I., Hajdu, S., Edler, L., Andersson, A., Wasmund, N., Busch, S., et al. (2006). “Biovolumes and size-classes of phytoplankton in the Baltic Sea, Helsinki,” in HELCOM Balt. Sea Environ. Proc. No. 106, 144pp. hdl:10013/epic.39031.d001
Panigrahi, S., Nydahl, A., Anton, P., and Wikner, J. (2013). Strong seasonal effect of moderate experimental warming on plankton respiration in a temperate estuarine plankton community. Estuar. Coast. Shelf Sci. 135, 269–279. doi: 10.1016/j.ecss.2013.10.029
Pomeroy, L. R., and Deibel, D. (1986). Temperature regulation of bacterial activity during the spring bloom in newfoundland coastal waters. Sci. 233, 359–361. doi: 10.1126/science.233.4761.359
Pomeroy, L. R., and Wiebe, W. J. (2001). Temperature and substrates as interactive limiting factors for marine heterotrophic bacteria. Aquat. Microb. Ecol. 23, 187–204. doi: 10.3354/ame023187
Pruesse, E., Peplies, J., and Glöckner, F. O. (2012). SINA: accurate high-throughput multiple sequence alignment of ribosomal RNA genes. Bioinformatics 28, 1823–1829. doi: 10.1093/bioinformatics/bts252
Raven, J. A., and Geider, R. J. (1988). Temperature and algal growth. N. Phytol. 110, 441–461. doi: 10.1111/j.1469-8137.1988.tb00282.x
Robertson, C. E., Harris, J. K., Wagner, B. D., Granger, D., Browne, K., Tatem, B., et al. (2013). Explicet: graphical user interface software for metadata-driven management, analysis and visualization of microbiome data. Bioinformatics 29, 3100–3101. doi: 10.1093/bioinformatics/btt526
Rivkin, R. B., and Legendre, L. (2001). Biogenic carbon cycling in the upper ocean: effects of microbial respiration. Science 291, 2398–2400. doi: 10.1126/science.291.5512.2398
Sarmento, H., and Gasol, J. M. (2012). Use of phytoplankton-derived dissolved organic carbon by different types of bacterioplankton. Environ. Microbiol. 14, 2348–2360. doi: 10.1111/j.1462-2920.2012.02787.x
Sarmiento, J. L., Slater, R., Barber, R., Bopp, L. P., Doney, S. C., Hirst, A. C., et al. (2004). Response of ocean ecosystems to climate warming. Global Biogeochem. Cycles 18, 1–23. doi: 10.1029/2003GB002134
Segata, N., Izard, J., Waldron, L., Gevers, D., Miropolsky, L., Garrett, W. S., et al. (2011). Metagenomic biomarker discovery and explanation. Genome Biol. 12:R60. doi: 10.1186/gb-2011-12-6-r60
Schlitzer (2011). Ocean Data View. Available online at: https://odv.awi.de/
Simon, M., and Azam, F. (1989). Protein content and protein synthesis rates of planktonic marine bacteria. Mar. Ecol. Prog. Ser. 51, 201–213. doi: 10.3354/meps051201
Sommer, U., Aberle, N., Lengfellner, K., and Lewandowska, A. M. (2012). The Baltic Sea spring phytoplankton bloom in a changing climate. An experimental approach. Mar. Biol. 159, 2479–2490. doi: 10.1007/s00227-012-1897-6
Spilling, K., and Markager, S. (2008). Ecophysiological growth characteristics and modeling of the onset of the spring bloom in the Baltic Sea. J. Mar. Syst. 73, 323–337. doi: 10.1016/j.jmarsys.2006.10.012
Teeling, H., Fuchs, B. M., Becher, D., Klockow, C., Gardebrecht, A., Bennke, C. M., et al. (2012). Substrate-controlled succession of marine bacterioplankton populations induced by a phytoplankton bloom. Science 336, 608–611. doi: 10.1126/science.1218344
Teira, E., José Paz,ó, M., Serret, P., and Fernández, E. (2001). Dissolved organic carbon production by microbial populations in the Atlantic Ocean. Limnol. Oceanogr. 46, 1370–1377. doi: 10.4319/lo.2001.46.6.1370
Thornton, D. C. O. (2014). Dissolved organic matter (DOM) release by phytoplankton in the contemporary and future ocean. Eur. J. Phycol. 49, 20–46. doi: 10.1080/09670262.2013.875596
Tilzer, M. M., Elbrächter, M., Gieskes, W. W., and Beese, B. (1986). Light-temperature interactions in the control of photosynthesis in Antarctic phytoplankton. Polar Biol. 5, 105–111. doi: 10.1007/BF00443382
Utermöhl, H. (1958). “Zur Vervollkommnung der quantitativen Phytoplankton-Methodik,” in Mitteilungen Internationale Vereinigung Theoretische und Angewandte Limnologie, Vol. 9 (German), 1–38.
Vaquer-Sunyer, R., Conley, D. J., Muthusamy, S., Lindh, M. V., Pinhassi, J., and Kritzberg, E. S. (2015). Dissolved organic nitrogen inputs from wastewater treatment plant effluents increase responses of planktonic metabolic rates to warming. Environ. Sci. Technol. 49, 11411–11420. doi: 10.1021/acs.est.5b00674
Vázquez-Domínguez, E., Vaqué, D., and Gasol, J. M. (2007). Ocean warming enhances respiration and carbon demand of coastal microbial plankton. Global Change Biol. 13, 1327–1334. doi: 10.1111/j.1365-2486.2007.01377.x
von Scheibner, M., Dörge, P., Biermann, A., Sommer, U., Hoppe, H.-G., and Jürgens, K. (2014). Impact of warming on phyto-bacterioplankton coupling and bacterial community composition in experimental mesocosms. Environ. Microbiol. 16, 718–733. doi: 10.1111/1462-2920.12195
Wasmund, N., Andrushaitis, A., Łysiak-Pastuszak, E., Müller-Karulis, B., Nausch, G., Neumann, T., et al. (2001). Trophic status of the south-eastern Baltic Sea. A comparison of coastal and open areas. Estuar. Coast Shelf Sci. 53, 849–864. doi: 10.1006/ecss.2001.0828
Wasmund, N., Göbel, J., and Bodungen, B. V. (2008). 100-years-changes in the phytoplankton community of Kiel Bight (Baltic Sea). J. Mar. Syst. 73, 300–322. doi: 10.1016/j.jmarsys.2006.09.009
Wasmund, N., Nausch, G., and Feistel, R. (2013). Silicate consumption. An indicator for long-term trends in spring diatom development in the Baltic Sea. J. Plankton Res. 35, 393–406. doi: 10.1093/plankt/fbs101
Wasmund, N., Nausch, G., and Matthäus, W. (1998). Phytoplankton spring blooms in the southern Baltic Sea - spatio-temporal development and long-term trends. J. Plankton Res. 20, 1099–1117. doi: 10.1093/plankt/20.6.1099
Wasmund, N., Tuimala, J., Suikkanen, S., Vandepitte, L., and Kraberg, A. (2011). Long-term trends in phytoplankton composition in the western and central Baltic Sea. J. Mar. Syst. 87, 145–159. doi: 10.1016/j.jmarsys.2011.03.010
Wear, E. K., Carlson, C. A., James, A. K., Brzezinski, M. A., and Windecker, L. A. (2015). Synchronous shifts in dissolved organic carbon bioavailability and bacterial community responses over the course of an upwelling-driven phytoplankton bloom. Limnol. Oceanogr. 60, 657–677. doi: 10.1002/lno.10042
Weinbauer, M. G., Fritz, I., Wenderoth, D. F., and Höfle, M. G. (2002). Simultaneous extraction from bacterioplankton of total RNA and DNA suitable for quantitative structure and function analyses. Appl. Environ. Microbiol. 68, 1082–1087. doi: 10.1128/AEM.68.3.1082-1087.2002
Wohlers, J., Engel, A., Zöllner, E., Breithaupt, P., Jürgens, K., Hoppe, H.-G., et al. (2009). Changes in biogenic carbon flow in response to sea surface warming. PNAS 106, 7067–7072. doi: 10.1073/pnas.0812743106
Wohlers-Zöllner, J., Biermann, A., Engel, A., Dörge, P., Lewandowska, A. M., von Scheibner, M., et al. (2012). Effects of rising temperature on pelagic biogeochemistry in mesocosm systems. A comparative analysis of the AQUASHIFT Kiel experiments. Mar. Biol. 159, 2503–2518. doi: 10.1007/s00227-012-1958-x
Yager, P. L., Connelly, T. L., Mortazavi, B., Wommack, K. E., Bano, N., Bauer, J. E., et al. (2001). Dynamic bacterial and viral response to an algal bloom at subzero temperatures. Limnol. Oceanogr. 46, 790–801. doi: 10.4319/lo.2001.46.4.0790
Keywords: phytoplankton spring bloom, bacteria, primary production, bacterial production, temperature, global warming, Baltic Sea, bacterial community composition
Citation: von Scheibner M, Herlemann DPR, Lewandowska AM and Jürgens K (2018) Phyto- and Bacterioplankton During Early Spring Conditions in the Baltic Sea and Response to Short-Term Experimental Warming. Front. Mar. Sci. 5:231. doi: 10.3389/fmars.2018.00231
Received: 10 January 2018; Accepted: 14 June 2018;
Published: 13 July 2018.
Edited by:
Riina Klais, University of Tartu, EstoniaReviewed by:
Raquel Vaquer-Sunyer, Instituto Mediterráneo de Estudios Avanzados (IMEDEA), SpainJelena Godrijan, Bigelow Laboratory For Ocean Sciences, United States
Copyright © 2018 von Scheibner, Herlemann, Lewandowska and Jürgens. This is an open-access article distributed under the terms of the Creative Commons Attribution License (CC BY). The use, distribution or reproduction in other forums is permitted, provided the original author(s) and the copyright owner(s) are credited and that the original publication in this journal is cited, in accordance with accepted academic practice. No use, distribution or reproduction is permitted which does not comply with these terms.
*Correspondence: Klaus Jürgens, a2xhdXMuanVlcmdlbnNAaW8td2FybmVtdWVuZGUuZGU=