- 1Department for Marine Biology, Leibniz Institute for Baltic Sea Research, Warnemünde, Germany
- 2Department of Experimental Ecology of Marine Organisms, Institute of Oceanography, University of Gdańsk, Gdańsk, Poland
During three cruises to the Bay of Gdansk, Baltic Sea, the fauna, porewater and bottom water were sampled at stations parallel to the shore and along a transect offshore. Diffusive porewater fluxes were calculated and related to the total net fluxes (TNF) of nutrients. The TNF comprise all nutrients that reach the bottom water from the sediment including diffusive nutrient efflux, discharge from macrozoobenthos and microbial activity. They were determined during in situ incubations using a benthic chamber lander, which is rarely done in coastal research. The lander restricts the physical influence of currents and waves on the sediments and only allows nutrient fluxes due to bioturbation by natural communities. Strong benthic-pelagic coupling in the shallow coastal zone suggested a crucial filter function for the bioturbated coastal sediments, which are separated from muddy deep sediments with little or no fauna at a depth of 50 m; in between is a small intermediate zone. While diffusive fluxes were highest at intermediate and offshore stations, TNF were highest at sandy coastal stations, where reservoirs of dissolved nutrients were small and sediments almost devoid of organic material. The greatest impact of macrofauna on sedimentary fluxes was found at stations whose communities were dominated by deep-burrowing polychaetes. The largest TNF were measured directly at the mouth of the Vistula River, where riverine food and nutrients supplies were highest. Macrofauna communities and sediment variables can thus serve as descriptive indicator to estimate the extent of the coastal filter. Finally, based on the total areal size of the different sediment types, annual efflux for the complete coastal zone of the Gdansk Bay was estimated to be 6.9 kt N, 19 kt Si, and 0.9 kt P. Compared to the muddy offshore area, which is twice as large, these amounts were similar for P and threefold higher for N and Si.
Introduction
Eutrophication and anoxia threaten large stretches of the world's coastal zones, including those of the Baltic Sea (Diaz and Rosenberg, 2008; Conley et al., 2011). Although most nutrients from rivers are sequestered in the coastal zone, as suggested in the Baltic Sea by the δ15N budget (Voss et al., 2005), little is known about the processes that impact the recycling of dissolved and particulate organic matter or the contribution of the related transport processes. The current definition of coastal zones takes into account their high nutrient turnover rates, the product of intensive benthic-pelagic coupling (Dürr et al., 2011). In addition, shallow coastal zones are impacted by wind waves and swell, both of which strengthen benthic-pelagic coupling via sediment resuspension in the breaker zone (Phillips, 1966). Besides physical mixing, the reworking of substrates by macrofauna (bioturbation) modifies natural gradients in the system and alters the exchange of nutrients between sediment and water, which in turn changes the bioavailability of nutrients (e.g., Graf and Rosenberg, 1997; Aller and, 1998). Microbial processes such as denitrification and nitrification are closely related to nutrient availability and are responsible for the efficiency of the coastal filter (Asmala et al., 2017). The highest remineralization potential due to microbes was shown to occur in sediments, and the highest production of particulate organic matter (POM) in the euphotic zone (Andersen and Kristensen, 1992).
The direct impact of macrofauna on nutrient turnover is via detrital food consumption and the predation of secondary consumers on primary consumers. Since most marine invertebrates are ammonotelic, the excreted metabolic waste products are ammonia and ammonium (e.g., Gardner et al., 1993; Wright, 1995; Devine and Vanni, 2002); thus, the benthic ammonium pool is either enlarged or eventually transported into the overlying water. Bioturbation, defined as the mechanical work of macrofauna in or on the sediment, impacts nutrient turnover processes indirectly, through the exchange of solutes and particles across the sediment-water interface through burrows and tubes (bioirrigation), particle mixing, biodeposition, and bioresuspension (Graf and Rosenberg, 1997; Aller and, 1998; Bouma et al., 2009; Laverock et al., 2011).
The impact of fauna on nutrient turnover and release has mainly been studied in lab experiments, which have shown an influence of this process on solute exchange. Higher ammonium concentrations in the bottom water of bioturbated sediments than in sediments with no macrozoobenthic activity were reported by Karlson (2007). However, differences between species have been demonstrated. For example, Corophium volutator and Hediste diversicolor doubled the ammonium efflux while Cerastoderma spp. did not have a positive effect on ammonium release (Pelegri and Blackburn, 1994; Mermillod-Blondin et al., 2004). Reworking by macrofauna increases the availability of nitrate in the sediment and thereby stimulates denitrification, which removes nitrogen from the system (Gilbert et al., 1998). However those effects vary depending on the species (Heisterkamp et al., 2012). The authors showed that the occurrence of the polychaete H. diversicolor increased nitrification rates in the system due to the ammonium supply, resulting in nitrogen retention. To understand the main net effect of fauna on nutrient removal or retention in the environment requires measurements of all related processes in combination with both, information on the structure of the faunal community and efflux data. Furthermore, an accurate investigation of the effect of macrozoobenthos and bioturbation on the change in nutrient availability must include a consideration of the differences between sedimentary nutrient fluxes in the presence and absence of fauna.
Artificial core experiments with rebuilt sediment column or modified infauna can provide information on an animal's effect on nutrient release from the sediment but not on net fluxes, as these involve the natural microbial and macrofauna community. For example, denitrification can only be quantified in naturally layered sediment cores with strong gradients in oxygen and nitrate, which are usually not obtained in cores rebuilt in the lab. Further, the mutual impact on the activity of one benthic species by another can be positive, neutral or negative; accordingly, the effect of bioturbation in a natural benthic community is less investigated and might be never simply the sum of the bioturbation effects of a single species or of a few individuals. Thus, while artificial core experiments yield valuable information on general processes, they do not necessarily allow the quantification of real-life fluxes. Even in situ cores may not include a representative, natural, undisturbed fraction of the benthic community, as a typical core 10 cm in diameter may include a community in which 200 ind/m2 of its residents have been disturbed or damaged during the retrieval. Contrary, only 50 ind/m2 are disturbed using cores 30 cm in diameter (Glud and Blackburn, 2002). Moreover, large individuals, for example bivalves or starfish, are often accidently excluded from measurements performed in small cores, even if in the field they are major contributors to nutrient exchange and oxygen consumption (Glud et al., 1998). Consequently, current knowledge of the impact of entire communities on nutrient cycling in the field remains deficient.
Chamber lander incubations are an appropriate tool to study nutrient release from sediments while excluding the influence of physical advection on vertical nutrient fluxes, but including the effect of a natural benthic community. In this study, a chamber lander system was used to address the following questions: What is the difference between in situ TNF and diffusive fluxes at the same station? How is the flux impacted by different benthic communities from the same site? To answer these questions, we compared data on the classical diffusive fluxes determined in 10-cm diameter cores with chamber lander data by following a holistic approach that considered all potential fluxes. We hypothesized that, due to the presence of fauna, TNF would be higher in near-coastal sediments than offshore and that an effect of deep burrowing animals will be visible in the in situ approach. Finally, we evaluated the coastal filter function of the Bay of Gdansk with respect to the presence of macrozoobenthos.
Materials and Methods
Study Area
The study area is located in the Polish part of the Bay of Gdansk in the southern Baltic Sea. The Vistula River is one of the largest rivers draining into the Baltic Sea. It contributes 90% of the total inflow to the Bay of Gdansk (Witek et al., 2003), with an annual N load of ~98 kt (recalculated from data of Pastuszak and Witek, 2012).
Samples were collected during three 10- to 15-day cruises with the “RV Elisabeth Mann Borgese” and “RV Alkor” in 2014 (July), 2015 (February), and 2016 (March) covering summer, winter, and spring, respectively. Measurements and samples were taken at 19 different stations (10–111 m water depth) (Figure 1). Due to inflows from the Vistula River, the salinity is in the range of 0–12, with salinities of almost 0 directly at the mouth and as 12 in the bottom water of the Gdansk Deep. Water temperatures in summer were between 18°C at the surface and 5°C in the bottom water. In winter and spring, temperatures varied between 3 and 6°C. The Vistula plume is variable and wind-driven, in a complex manner. In a steady state the plume is transported eastwards parallel to the coast.
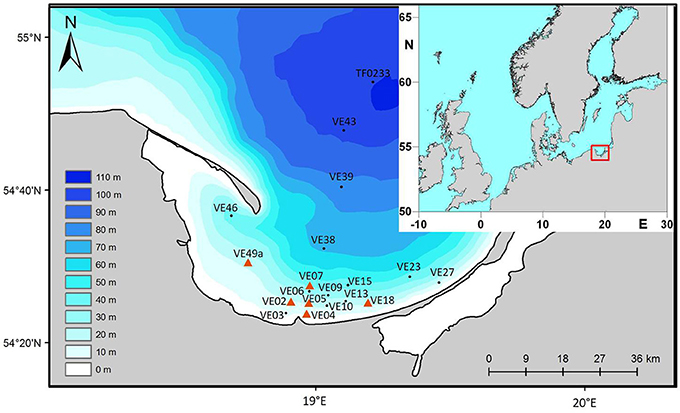
Figure 1. Location of the sampling stations in the Bay of Gdansk, southern Baltic Sea. The inlay shows a map of Northern Europe, the rectangle marks the Bay of Gdansk.
To cover all types of sediments, cores were taken along two transects; one extending directly offshore from the mouth of the river to the Gdansk Deep and the other parallel to the coast. This allowed coastal stations (down to 50 m water depth) to be distinguished from offshore stations (60–110 m water depth). Station VE07 (50 m water depth) differed from the other stations in that the values of the collected data were between those of the other stations; it was therefore considered as an intermediate station. The properties of the sediment at station VE07 suggested the presence of sandy as well as muddy components with a high organic matter content, such that an exact description of the sediment type was not possible.
The Bay of Gdansk has an area of 1,141 km2 where the water depth is <50 m and 2,153 km2 where the depth is >50 m. Its mostly well-sorted sediments are of different types and the entire area is characterized by a distinct patchiness (Figure 1). The three main sediment types were: fine sand (type 1), medium sand (type 2), and mud/silt (type 3). The areas covered by sediment types 1–3 were calculated using the program ArcGIS 10 (ESRI 2011) together with the WGS_1984_UTM_Zone_33N Projected Coordinate System.
Sediment and Porewater
At each sampling station, five sediment cores each 10 cm in diameter were taken using a multicorer. At coarse-grained sandy stations, multicorer sampling was not possible; instead, the sediments were sampled using Haps corers (KC Denmark) and subsampled with the same 10-cm diameter cores used for the multicorer, to ensure the same volumes. All cores had undisturbed surfaces and clear water on top. Until the cores were processed, they were kept cold and dark. Two of the cores were immediately used for porewater retrieval via rhizon sampling (Seeberg- Elverfeldt et al., 2005). The first milliliter was used to rinse the rhizon and 4 ml were stored frozen at −20°C for later nutrient analysis. Only the porewater samples from the winter cruise (2015) were measured onboard directly after sampling. The rhizons from rhizosphere had a membrane 5 cm in length and with a pore size of 0.12–0.18 μm; they were kept in ultra-pure water for 15 min before they were used in the sampling, to obtain suction during the filtration. Three of the cores from each site were sectioned into 0.5-cm slices in the upper 3 cm, 1-cm slices down to 10 cm, and 2-cm slices down to a sediment depth of 20 cm. The samples were kept frozen at −20°C until the analysis. Subsamples of each slice were used for the determination of water content and porosity, organic matter content, and grain size. Samples used to determine the water content were freeze-dried for 2.5 days, at which time they had reached a constant weight, and then weighed to determine the weight loss. The percentage of each sample composed of water is defined as the porosity. The organic matter content of the dry samples was measured as the loss of ignition (LOI) after heating in a muffle furnace at 550°C for 5 h.
The grain size in each slice was analyzed using a Cilas gradistrat 1180 laser granulometer according to a procedure based on a laser multi-angle analysis to measure particle diameter in the range of 0.04–1,000 μm. Particles >1,000 μm were retained by a sieve and not registered quantitatively, but they made up only a small fraction of the sample. The sediment-type designations used herein are based on the median grain size of each sample.
The δ15N values in the upper 3 cm of the sediment were analyzed using a Thermo Scientific Delta V Advantage (Thermo) isotope mass spectrometer combined with a Conflo IV (Thermo) interface and a Flash 2000 (Thermo) elemental analyzer. Each sample was dried for at least 2 days at 50°C and then homogenized with mortar and pestle. For mud and sand samples, 5 and 100 mg were weighed, placed in tin caps, and pressed into pellets. To measure the organic C content, a parallel sample was first acidified by adding ~10 μl of HCl (2N) and then processed as described for the δ15N samples except that silver caps were used.
Benthic Chamber Lander Incubations
In situ incubations to measure benthic nutrient fluxes across the sediment-water interface were done with the automated mini-chamber lander system (Unisense, DK). The first deployment was in February 2015, at station VE02; all other stations (VE04, VE05, VE07, VE49a, and VE18) were sampled in March 2016. The incubation chamber was square, with rounded corners, and Teflon-coated to avoid reactions between ambient oxygen and the stainless steel cover of the chamber. The adjustable feet and changeable weights on the lander frame enabled the chamber to penetrate the sediment to a depth of ~5 cm. The chamber proportions were 30 × 30 × 35 cm (width × length × height) and its sediment penetration depth was 5 cm. The enclosed incubation volume is large enough (27 L) to avoid oxygen depletion. The sediment area covered by the chamber was 900 cm2. The chamber size was favorable toward smaller chambers and cores, as the inaccuracy in measurements of oxygen uptake was 10% (few fauna) or 20% (abundant fauna), in contrast to core incubations in which the inaccuracy is 26 and 41%, respectively (Glud and Blackburn, 2002). The chamber lid was open during launch but autonomously closed after an acclimatization time defined in the program. The water inside the chamber was stirred during the whole sampling period at 15 rpm to ensure sample homogeneity. Preliminary tests showed that a homogenous water sample was obtained after 5 min of stirring. No resuspension effects from the bottom could be detected. Up to 12 water samples of 55 ml each were removed directly from the chamber using polypropylene syringes operated by an integrated water-sampling rosette. During the 12- to 15-h incubation used for each deployment, the first water sample was taken directly at the beginning of the incubation, and subsequent samples every 65–75 min. Oxygen inside the chamber was measured using an optode to monitor the total oxygen uptake and thus the chamber tightness. Only one small port in the chamber lid was kept open to ensure a pressure balance while retrieving the samples. The oxygen concentration profiles showed that the open port was not large enough for environmental water to enter the chamber and thereby disturb the measurements. The samples were incubated overnight, including at least 2 h before dusk and after dawn. The water samples were filtered through a syringe GF filter (Whatman) and frozen at −20°C directly after retrieval of the lander system.
Nutrient Analysis
Nutrients of all samples (porewater, bottom water, lander incubation) were measured colorometrically according to Grasshoff et al. (1999) using a SEAL analytical QuAAtro continuous segmented flow analyzer. Methods for NO-2 and NO-3 determination were adapted for the SEAL based on a procedure developed by NIOZ (Grasshoff et al., 1999). NH+4 levels were measure using sodium salicylate, which results in the formation of the same color complex obtained with the phenol reagent. To address different nutrient concentration ranges six-point- calibrations were done at different quantification limits. For porewater samples, the quantification limits were as follows: 0.25 μmol PO3-4/l, 0.05 μmol NO-2/l, 0.5 μmol NO-3/l, 1.5 μmol NH+4/l, and 2 μmol SiO2/l. For bottom water samples from the field and from the lander incubations, the quantification limits were the following: 0.25 μmol PO3-4/l, 0.05 μmol NO-2/l, 0.5 μmol NO-3/l, 0.5 μmol NH+4/l, and 1 μmol SiO2/l. We did not find significant increase in SiO2 concentrations when samples were filtered through GF/F, which was done for pore waters only. However, we took care to process samples as fast as possible. All nutrient samples were frozen at −20°C directly after sampling until used in the measurements, except the porewater samples of the winter cruise in 2015, which were analyzed onboard immediately after sampling.
Flux Calculations
Diffusive nutrient fluxes were calculated according to Fick's first law of diffusion:
where J is the flux (mmol m−2 d−1), Ds the effective diffusion coefficient, c the concentration of a nutrient, z the sampling depth, and Δz the distance between two sampling depths. Fluxes were calculated using steady porewater nutrient profiles. If the profile shows vertical oscillation, a 1-2-1- filter was used to obtain steady profiles. The effective diffusion coefficient for each nutrient in the respective sediment was calculated according to Equation (2), using the diffusion coefficients for dissolved nutrients in water (D0) (Iversen and Jørgensen, 1993; Schulz and Zabel, 2006). Porosity (Φ) was calculated according to Flemming and Delafontaine (2000) where n reflects a coefficient for the sediment type.
For each diffusive flux calculation, two parallels were considered. The TNF were used to calculate the flux during the incubation. Finally, the fluxes were extrapolated to 1 m2 based on the chamber volume of 27 l.
Macrofauna
At each station, at least four parallel samples were taken with a 0.1 m2 Van Veen grab, sieved with a 1-mm mesh sieve, and preserved in 4% borax-buffered formalin. At least four parallel fauna samples were taken at each site while the ship was remained in a fixed position. The grab samples covered an area of several tens of square meters. It was therefore assumed that the average fauna sample also represented the fauna in the incubation chamber. The fauna was sorted and identified to the lowest taxonomic level using a stereomicroscope. Individuals were counted and weighed to determine their abundance and biomass (biomass data not shown in this study). To relate the fauna to sediment properties and nutrient fluxes. The faunal species were grouped according to their burrowing depth and irrigation behavior (e.g., Davey, 1994; Zettler et al., 1994; Pelegri and Blackburn, 1995), as these are the most relevant features for solute exchange between sediment and water.
Statistics
Principal Component Analyses (PCA) and cluster analysis were applied using the software Statistica (Statistica System Reference, 2012). An agglomerative cluster analysis was computed with the variables sediment surface grain size, LOI and water depth using Euclidian distance norm (Figure 3 right). Functional traits of the macrofauna, sediment properties and nutrient fluxes are subject of a PCA computing the eigenvalues and eigenvectors of the correlation matrix to analyses the dominant relationship in the coastal system (Figure 8).
A canonical correlation analysis (CCA) was performed between the leading eigenvectors of benthic animals, TNFs, and sediment variables (depth, grain size, LOI) using statistical downscaling methods (von Storch et al., 1993). This method has been applied e.g., to macrozoobenthos communities in the German Bight (Kröncke et al., 2013) or in the Gulf of Riga (Dippner and Ikauniece, 2001) and details on the method were given in Heyen and Dippner (1998).
Results
Source of Particulate Organic Nitrogen
The organic material in the sediment was characterized by high δ15N values (8.6 ± 0.8%0) at stations with a water depth of <50 m. The highest values were at the shallowest stations near the river mouth. The δ15N values of the sediments at stations deeper than 50 m, including the intermediate station VE07, were lower (6.3 ± 0.9%0). A comparison of the δ15N values of POM from the Vistula River (8%0) and the surface waters of the offshore station farthest from the river mouth (station TF0233, 5%0) suggested a riverine impact on the organic matter at coastal sediments, but a declining influence offshore (Figure 2 left). This relationship was less obvious in δ13C values of the surface sediment. The δ13C values were higher at coastal stations (−26.8 ± 0.8%0) and slightly lower (−25.8 ± 0.5%0) further offshore (Figure 2 right), a trend typical for coastal sediments. In fine and medium sand the standard deviation for δ15N values was ±0.63%0 and ±0.6%0 for δ13C values. For mud the standard deviation was of ±0.29%0 for δ15N values and of ±0.53%0 for δ13C values.
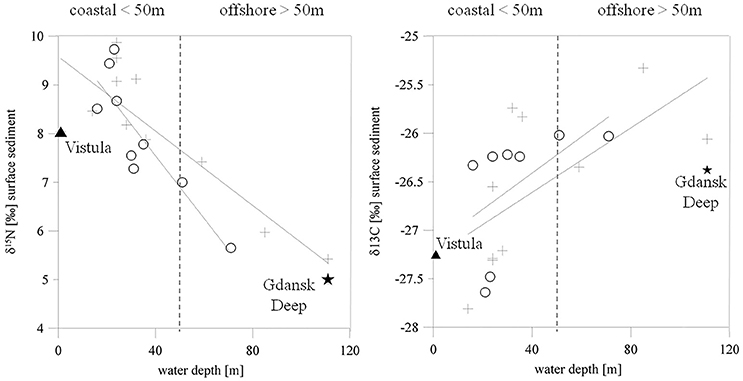
Figure 2. δ15N (left) and δ13C (right) values of the organic matter from the upper surface sediments as a function of water depth. Crosses mark winter 2015 and circles spring 2016 data. The straight lines show the regression. The filled triangle represents Vistula River POM, and the filled star the surface-water POM at the deep-water station TF0233.
Sediment Characteristics and Organic Matter
The properties of the well sorted sediment samples suggested the patchiness of fine and medium sand, with smaller areas covered by medium- and coarse-grained silt emerging in shallower areas. All of the sediments from the offshore stations with a water depth >50 m were muddy, while those from coastal stations had lower organic matter content and higher grain size. Fine and medium sand had an average standard deviation of ±23.15 μm for and mud an average standard deviation of ±4.35 μm. The median grain size and organic matter content of the surface sediments correlated with the water depth, based on R2 values of 0.88 and 0.92 for the winter and spring cruises, respectively. Figure 3A displays surface values only. Further analyses indicated the same structure in the vertical up to a depth of 20 cm (not shown). For the three parallel cores at each station we found an average standard deviation of ±0.89% dry sample at sandy sites and of ±1.6% dry samples at muddy stations. The division in three main group is also supported by a cluster analysis (Figure 3). The cluster analysis underlines that especially the deep muddy stations and the coastal medium sand stations of both years group together, the fine sand stations build the third main cluster. Station VE07_2015 groups to the offshore stations while the same station sampled in 2016 groups to the fine sand stations. That may illustrate its variability and the intermediate character between sands and muds at 50 m depth.
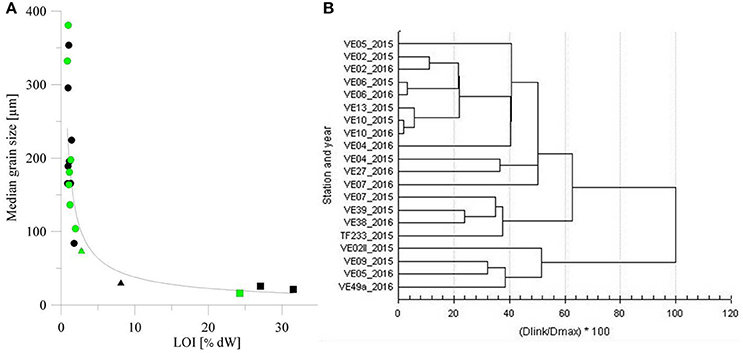
Figure 3. (A) Correlation of the loss on ignition (LOI, in % dW) and the median grain size for samples collected during winter cruise AL449 (black) and spring cruise EMB123 (green). Dots indicate the coastal stations, triangles the intermediate stations, and squares the offshore stations. (B) Cluster analysis of surface grain size, surface LOI, and water depth of all stations against the distance value (D) in percent.
Porewater Nutrient Concentrations and Fluxes in the Cores
The nutrient concentrations did not differ in magnitude during the different seasons. Porewater nutrient concentrations are closely related to the sediment type and, in general, higher concentrations of SiO2, , and are found in the porewaters of muddy sediments than in those of sandy, shallower sediments. The properties of the porewater from the near-surface sediment layers were quite similar and in the lower ranges at all stations (<10 μmol/l for and , <1 μmol/l for , ~5 μmol/l for , and 10–50 μmol/l for SiO2). In deeper sediment layers the differences were more pronounced (see Supplementary Figure 1). The porewater concentrations of SiO2, , and NH4 + were highest at muddy offshore stations, but much lower at coastal stations. The maximum concentration was between 2,000 and 3,000 μmol/l, while the maximum values were as high as 360 μmol/l, and those of SiO2 up to 1,500 μmol/l. At coastal sites, the maximum concentration was in the range of 100–200 μmol/l, measured in deeper waters. The concentrations at all coastal stations were as high as 50 μmol/l, with summer values being slightly higher than winter and spring values. The SiO2 concentration in coastal waters was between 200 and 500 μmol/l. The porewater nutrient concentrations at the intermediate station VE07 were mostly between those of the coastal and offshore stations. Coastal stations VE04 and VE03, located close to the river mouth, showed small deviation from the general patterns whereas their porewater nutrient concentrations were lower than those at the offshore stations. Measurable and concentrations were detected only in sandy sediments; at muddy sites the concentration of both nutrients was below the detection limit (see Supplementary Figure 1).
The concentration gradients were related to different fluxes, with higher diffusive fluxes of SiO2, , and at muddy stations (except stations VE03 and VE04). Most fluxes did not differ in magnitude between seasons, thus indicating a stable condition of the sediment porewater. A significant difference occurred at station VE07, where the diffusive flux values in winter were 3–14 times higher than those in spring. Diffusive SiO2 fluxes at the coastal stations were between 0.01 and 0.14 mmol m−2 d−1, with the exception of station VE03, located close to the river mouth, where the flux was 2.11 mmol m−2 d−1 (0.62 mmol m−2 d−1 for ), and station VE46, with a flux of 0.22 mmol m−2 d−1 (0.21 mmol m−2 d−1 for ). The latter station with muddy sediment in Puck Bay was largely influenced by sediment type and not by depth alone. At the muddy offshore stations, SiO2 fluxes were 0.44–0.66 mmol m−2 d−1. fluxes were in the range of 0.002–0.62 mmol m−2 d−1, with a mean of 0.06 mmol m−2 d−1. At station VE07, the diffusive flux was 0.06–0.18 mmol m−2 d−1 and at the offshore stations 0.21–0.87 mmol m−2 d−1. For , the fluxes were between 0.004 and 0.03 mmol m−2 d−1 (except VE03: 0.06 mmol m−2 d−1, and VE46: 0.01 mmol m−2 d−1) at coastal stations, between 0.005 and 0.07 mmol m−2 d−1 at the intermediate station, and between 0.01 and 0.68 mmol m−2 d−1 at the offshore stations. In muddy sediments, diffusive and fluxes could not be calculated, but the values determined were in the range of −0.01 to −0.006 mmol m−2 d−1 indicating a flux into the sediment (Supplementary Table 1).
Nutrient and Oxygen Fluxes in Chamber Lander Incubations
Chamber lander incubations were only possible in shallow water down to a depth of 50 m.
Continuous oxygen consumption was determined in all sediments but only down to a minimum of 299.63 μmol/l. The most rapid decrease in the oxygen concentration was at station VE04, near the mouth of the Vistula River, where the flux was −31.48 mmol m−2 d−1. The oxygen fluxes at stations VE02 and VE05 were only half as high. At the eastern and western stations VE18 and VE49a, oxygen consumption was slightly lower: 12.43 and 11.26 mmol m−2 d−1, respectively. At station VE07, the oxygen concentration inside the chamber dropped very slowly, as indicated by a flux of −2.37 mmol m−2 d−1 (Figure 4).
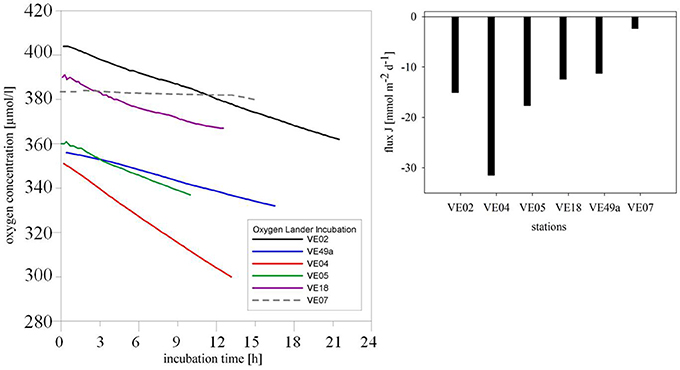
Figure 4. Change in the oxygen concentrations in the chamber over time. Solid lines in black (VE02), blue (VE49a), red (VE04), green (VE05), and violet (VE18) indicate the coastal stations, and the dashed gray line the intermediate station VE07 (left). Oxygen consumption at the different stations is expressed as the flux (mmol m−2 d−1) (right).
Net nutrient fluxes were high at all sandy stations and lower at the intermediate muddy station. At all stations, nutrient concentrations increased over time, except for at station VE04 and at station VE49a, where the respective concentrations decreased over time. Generally, at all stations the highest initial concentrations were those of and SiO2 whereas and concentrations remained low throughout the incubations (Figure 5). The strongest concentration changes were those of at the coastal stations, where initial concentrations were 0.5–2.2 μmol/l but increased to 1.3–6.8 μmol/l by the end of the incubation. At VE07, the concentrations of all nutrients fluctuated during the incubation. Large changes in the and concentrations over time were not detectable at any of the coastal stations. Fluctuations in nutrient concentration during the incubations caused TNF with respect to the start and end values that were lower than the fluxes over the short term, in some cases. A decrease in the concentration of from 8.2 to 7.3 μmol/l after 11 h (Figure 5) was measured at station VE04 (see Supplementary Material).
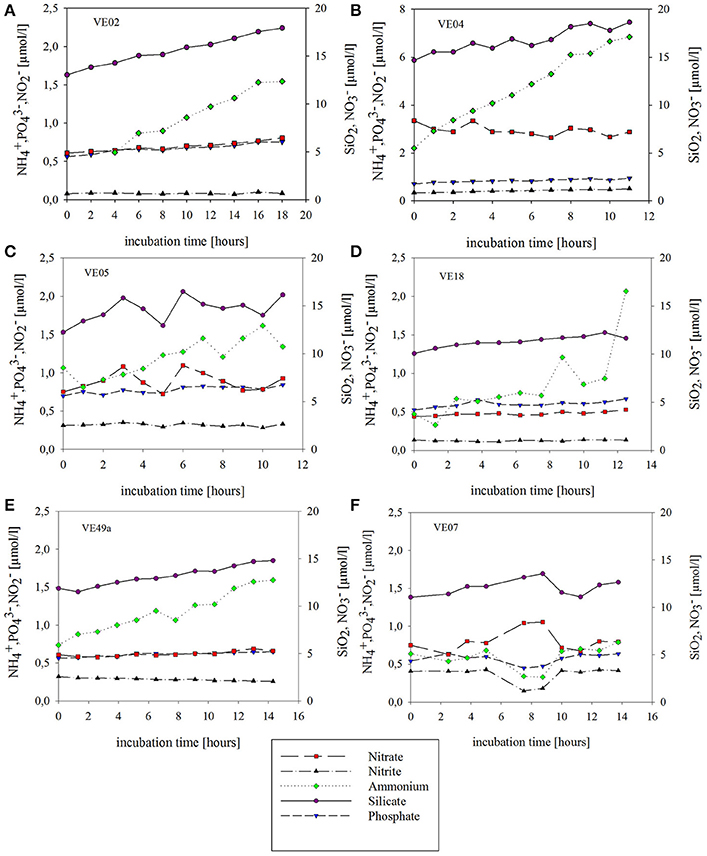
Figure 5. Concentration changes over time during the chamber lander incubations of sediment and bottom water at five sandy coastal stations (A–E) and one muddy intermediate station (F). Symbols indicate the time points at which the water samples were taken (via syringes) and the concentration of each nutrient at each time point. The lines connecting the time points show the tendency of the concentration development. SiO2 is indicated in purple, in green, in red, in black, and in blue.
The data obtained from the chamber Lander incubations were used to calculate the TNF. Based on the nutrient concentrations inside the chamber, all fluxes were positive, with two exceptions, at stations VE04 and VE49a, where the total flux and the total flux, respectively, were negative, which may indicate a flux into the sediment. The TNF was lowest at station VE07 in nearly all cases, while at the sandy stations the TNFs were high. The highest averaged fluxes were those of SiO2, and the lowest positive fluxes those of at all stations. The fluxes at all stations ranged between 0.01 and 0.16 mmol m−2 d−1 for , from −0.77 to 0.91 mmol m−2 d−1 for , and from 0.08 to 3.04 mmol m−2 d−1 for . The maximum flux of all incubations occurred at station VE04, where the flux was 3.04 mmol m−2 d−1; this value exceeded even the SiO2 flux (2.61 mmol m−2 d−1) (Figure 6).
Comparison of TNF and Diffusive Fluxes
Considering all nutrient fluxes, an amplification factor (AF) is defined as the relation of TNF to the diffusive fluxes, which showed a band- width of 1.3–303. The highest AF of 303 occurred in at station VE05. This was the only station where a positive diffusive flux of was measured. The second highest flux was that of SiO2 (AF of 11.9–255). For and , the AF were quite similar at the different stations: 10–16 and 1.3–32.5, respectively. In nearly every case, the AF for the intermediate station VE07 were lower than those of the coastal stations. For the coarse sands at stations VE18 and VE49a, the TNF of SiO2 was lower than at the other stations, but the AF were higher (Figure 6), which may have been related to the presence of fauna (see below).
Macrofaunal Composition
The individual abundances of macrofauna and the species richness decreased with larger distance to the shore and to the mouth of the Vistula River (Figure 7). The highest abundances were measured at stations VE02, VE04, and VE05, closest to the river mouth, followed by stations VE18 and VE49a, east and west of it. The abundance at the intermediate station VE07 was approximately one-third of the average abundances at the coastal stations. At station TF0233 (Table 1), representing the Gdansk Deep, the absence of any fauna was consistent with the lack of oxygen in these waters. Small, surface-living animals, dominated by the surface-grazing snail P. ulvae, contributed numerous individuals but little biomass. Shallow- and deep-burrowing animals accounted for a large percentage of the macrofaunal community at coastal stations. The macrofaunal communities in the Bay of Gdansk were dominated by following species: the amphipod C. volutator at station VE02, the soft- shell clam Mya arenaria at station VE04, and the polychaete Marenzelleria spp. at station VE05. Station VE07 was dominated by shallow-burrowing animals, mostly the small clam M. baltica and the amphipods P. femorata and M. affinis. This station lacked deep-burrowing animals, in contrast to the coastal stations, and harbored only a few individuals of Marenzelleria spp. and P. elegans. Excluding the highly abundant P. ulvae at the coastal stations, the overall abundance of shallow-burrowing individuals at station VE07 was only half or less of that of the sediment infauna at the coastal stations. Only two of the three offshore stations were inhabited by macrofauna: at station VE39 (5 individuals per m2) and at station VE38 (32 individuals per m2). At the latter station, the macrofaunal community comprised M. baltica and B. sarsi, both of which are surface-living and shallow-burrowing species. Generally, mollusks and polychaetes dominated over crustaceans in the waters of the Bay of Gdansk (Table 1).
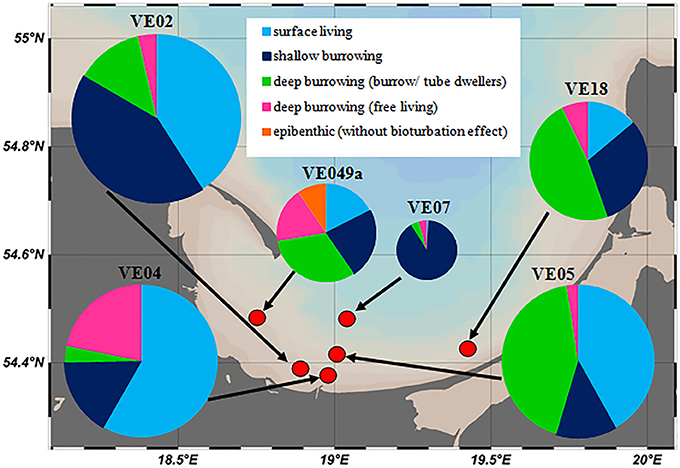
Figure 7. Overview of the proportional occurrence and distribution of functional groups of macrozoobenthos at the six chamber lander incubation stations. The sizes of the pie charts reflect the total individual abundances at the stations, given in Table 1.
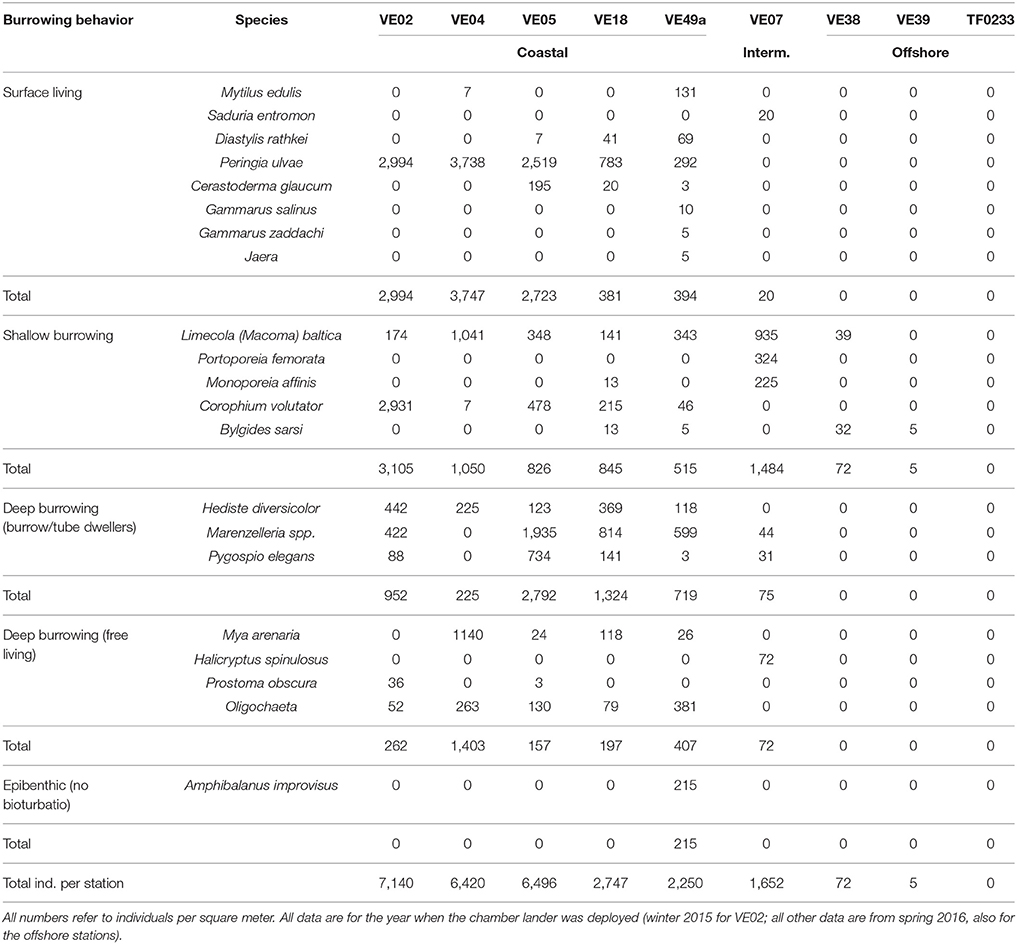
Table 1. Macrofaunal abundances and individual composition at each chamber lander incubation site and the three offshore stations.
Finally, we performed a principal component analysis (PCA) to figure out dependencies between high of SiO22 TNF, SiO2 factors and different variables (Figure 8). The dominant pattern with an amount of explained variance of 78% in the first two modes indicated a clear dependence between AF and deep burrowing tube dwellers as well as a strong negative correlation between AF and SiO2 inventory in the sediment. Further, the high of TNF is depending on more diverse variables such as most of the remaining groups of fauna which built the major portion of the macrozoobenthos community. The water depth is negatively correlated with the TNF.
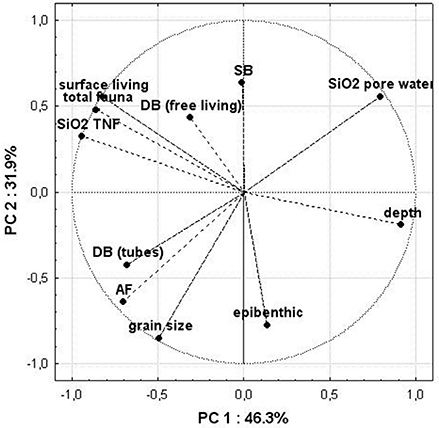
Figure 8. Results of the principle component analysis (PCA). The eigenvectors of the correlation matrix are shown as lines. The variables included in the PCA are DB, deep burrowing fauna; SB, shallow burrowing fauna; epibenthic, epibenthic fauna; depth, water depth at the stations; total fauna, total amount of individuals of all groups at the station.
The CCA pattern of the dominant eigenvectors of sediment variables and TNFs were uncorrelated (r = −0.17) and a negative Brier based skill score indicated a poor potential predictability (Livezey, 1995). In contrast, a significantly high correlation occurred in the CCA between benthic animals and TNFs (Figure 9).
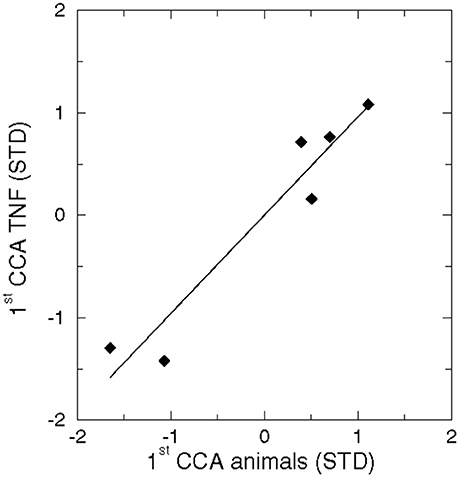
Figure 9. Correlation between the 1st CCA pattern of benthic animals and the 1st CCA pattern of TNFs (r = 0.96, n = 6, p < 0.01).
Extrapolation of the Data to the Whole Bay of Gdansk
Diffusive and total nutrient fluxes were estimated based on the area covered by each representative sediment type (Figure 10), for coastal and for offshore areas. The annual diffusive and total fluxes of -N from the sediment into the bottom water in the coastal zone of the Bay of Gdansk were 0.31 and 6 kt, respectively. For -P, the annual diffusive and total fluxes were 0.11 and 0.84 kt, respectively. The largest annual diffusive and total fluxes were those of SiO2-Si: 0.92 and 18.83 kt, respectively. Adding the total annual -N efflux (0.74 kt) or that of -N (0.16 kt) yielded a balance of 6.9 kt N per year originating from the sediment and entering the bottom water in the Bay of Gdansk's coastal zone. For the offshore area, the total fluxes were consistent with the diffusive fluxes of the muddy stations. Extrapolation of - N, SiO2- Si, and -P to the entire offshore area resulted in annual fluxes of 2.96, 6.8, and 0.9 kt, respectively.
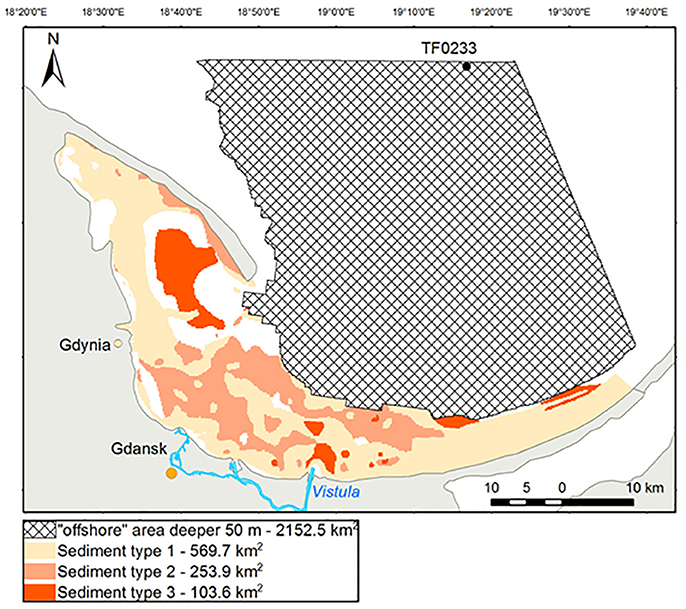
Figure 10. Sediment type distribution. Type 1 = fine sand (VE04 and VE02), type 2 = medium sand (VE05, VE18, and VE49a), type 3 = mud (VE07). The shaded area indicates the offshore region (water depth of 50–100 m) which were excluded from the classification.
Discussion
Characterization of the Coastal Zone: Zonation of the Study Site
In contrast to other studies on coastal zone nutrient cycling, most of which investigated lagoons or semi-enclosed bights, the focus of our study was an open bay with strong environmental gradients. This region was divided into sub-areas based on the grain size, LOI, and stable isotope data (δ 15N, δ 13C) of the sediments and the POM, thus separating an area shallower than 50 m depth, covered mainly by fine and medium sand, from offshore muddy sediments. That is a typical feature of coastal zones, as shown, for example, by Reid et al. (2005) for the US Atlantic coast, Wilson et al. (2008) for various regions in the world ocean and by Leipe et al. (2011) for the southern Baltic Sea. Waters with a depth of <50 m may be the natural border for a close benthic-pelagic coupling. If the impact of wind-induced waves reaches the bottom, fine-grained material is resuspended into the water column and transported parallel to the coast by the long- shore current (Longuet- Higgins, 1970). The consequence is that the shallow areas are covered by coarser- grained sediment. The δ15N and δ13C values suggested a strong impact of Vistula-River-derived organic matter on the sea bottom near the river mouth and dilution of the signal further offshore, while the higher δ 15N values were an indicator of land-derived material (Voss et al., 2005). Even if the Vistula River plume propagated quasi geostrophic balanced, parallel to the coast, the sediments of the entire coastal zone showed an intense δ15N signal. Under oxygen availability, even the fine fraction in sandy sediments may be used more efficiently than the muddy sediments (Leipe et al., 2017). However, in our study the fine fraction was not analyzed separately, such that information on the organic carbon content of sediment <63 μm was not available. At the muddy stations, the grain size of the complete sediment volume could be assigned to the fine fraction. Fauna may play an important role in changing the bioavailability of this organic fraction. Leipe et al. (2017) described an effect of the fine fraction of the sandy sediments of the Oderbank (Germany), showing that sand and mud provide nearly the same amount of organic carbon. Thus, the organic and fine fractions in sandy sediments are readily available to the fauna and microbes and thus potentially fully degraded by them, whereas this is not the case in mud. The offshore macrofaunal abundance showed a pattern similar to that of the sediment characteristics (Figure 7).
Species richness and individual abundances were separated by the 50-m depth contour. Since the sediment type determines the settlement of macrofauna, most species will be found in the sandy sediment or in sand-mud mixtures, with few species and low abundances in pure mud (Künitzer et al., 1992). Denisenko et al. (2003) reported that community structure is highly influenced by the total organic carbon content and the water depth of shallow waters. While at deeper stations the settling of POM from the pelagial may be restricted by stratification, at coastal sites larger amounts of potential food reach the bottom and can lead to the development of a greater infauna biomass. Additionally, biodeposition induced by deposit feeders increases the introduction of carbon and nitrogen to the benthic system (Norkko et al., 2001).
Therefore, in the absence of tides, the factor limiting macrofaunal occurrence is oxygen availability (Warwick and Uncles, 1980), as stable stratification in the deep basins can lead to oxygen deficiency or anoxia at the bottom. Janas et al. (2004) measured H2S concentrations as high as 443 μmol/l in the sediment of a 51-m-deep station. The oxygen supply was lower at this station than at a station with a depth of 37 m, where the maximum H2S concentration was ~13 μmol/l. A stable macrofaunal community, characterized by the relatively high-level reproduction of animals, can develop only under permanent oxygen conditions (Jørgensen, 1980; Rosenberg et al., 1992; Diaz and Rosenberg, 1995). Accordingly, the largest number of individuals was detected at shallow stations VE02, VE04, and VE05, fewer at intermediate station VE07, and none in the hypoxic Gdansk Deep (Figure 7 and Table 1).
Previous studies revealed the dominance of different species in the macrozoobenthos communities of the Bay of Gdansk (e.g., Janas et al., 2004) and their close relationship to sediment type.
The high abundances of the grazing snail P. ulvae at all coastal stations provided an indication that settling POM comprises a major food source. The filter-feeding bivalve M. arenaria was prominent at station VE04, presumably due to the ample food supply near the mouth of the Vistula River. The occurrence of perennial, omnivorous predators such as H. diversicolor and Marenzelleria spp. (data not shown) evidenced the permanent presence of this food supply and the associated prey animals. At the intermediate station VE07, mostly surface-living or shallow-burrowing animals were detected, probably because of the noticeable occurrence of H2S in the upper sediments. M. baltica has a high tolerance of both low oxygen concentrations and the presence of H2S (Long et al., 2008), which together probably account for it being one of the dominant species. Hence, species distribution can also be used to define the extent of the coastal zone.
Patterns of Nutrient Release and the Impact of Macrofauna
Oxygen as a Measure of Benthic Activity
The rates of oxygen consumption in coastal zones of the Bay of Gdansk was in the range of 11.24–31.48 μmol O2 m−2 d−1, indicating the higher biological activity at the coastal stations than at the intermediate station VE07, where the rate was 2.37 μmol O2 m−2 d−1 (Figure 4). Although in terms of their size bacteria use more oxygen than macrozoobenthos and are the largest sink for oxygen, because of their large numbers in the (muddy) sediment (Schwinghamer, 1981), macrofaunal respiration uses more oxygen at once. Our data showed a much higher total net oxygen flux into the sediment at the sandy coastal stations than at the intermediate station (Figure 4). This was caused by intense macrofaunal activity at coastal sites, due to individual abundances and a reworking of the sediment, the rate of which exceeded that of oxygen consumption (Moodley et al., 1998) by bacterial activity at the intermediate, muddy station. The deep-burrowing species H. diversicolor and M. viridis alone caused a doubling of benthic oxygen consumption (Kristensen et al., 2011). Thus, as a measure of the activity of benthic communities, oxygen consumption differentiates the coastal from the intermediate zone (Moodley et al., 1998).
Comparison of Fluxes
The nutrient inventory of the sediments matched that previously described for the porewaters of different sediment types (Devol and Christensen, 1993; Mortimer et al., 1999; Tuominen et al., 1999). While the sands lacked nutrient reservoirs, except of and , in muddy sediments significantly high amounts of SiO2, , and were measured, increasing with depth (Figure 11, Supplementary Figure 1). This accounted for the higher diffusive efflux in muddy than in sandy sediments (Supplementary Table 1).
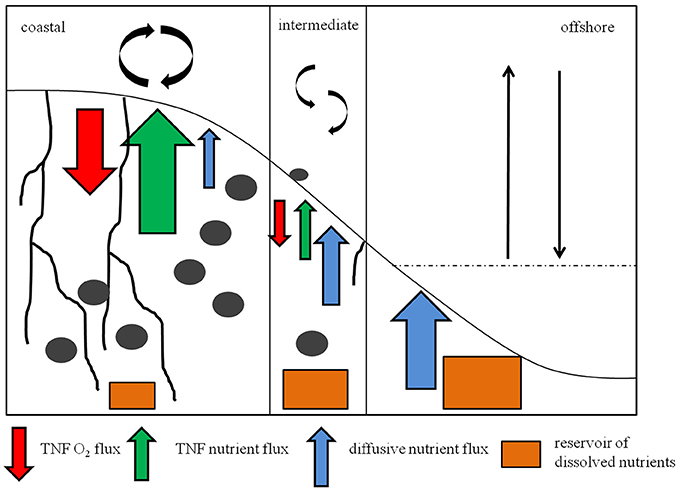
Figure 11. Schematic overview of the sedimentary conditions and trends in fluxes at coastal, intermediate and offshore stations. Black irregular lines in the sediment symbolize deep burrowing tube- dwellers. Gray ellipses symbolize burrowing animals. The red arrows mean the total net flux of O2 to avoid misunderstandings, green arrows mean the TNF of nutrients, blue arrows mean the diffusive flux of nutrients. Orange boxes stand for the nutrient inventory inside the sediment. The size of these symbols stands for the high of the fluxes and the size of the nutrient inventories in the field.
Consistent with our findings regarding O2 consumption, the TNF showed the greater release of nutrients from the coastal zone than from offshore and intermediate stations, attributable to active reworking of the sediments by macrofauna. The efflux of dissolved nutrients was generally higher at sandy sites (Figure 6), even though fewer dissolved nutrients were stored in the sedimentary reservoirs (Figure 11, Supplementary Figure 1). The food supply at the sediment surface is a driving factor in sediment reworking. Duport et al. (2006) reported a positive linear relationship between the density of H. diversicolor and sediment reworking and showed a significant effect of the abundance of animals on particle transport into the sediment similar to our own observations. In addition, the irrigation activity increases with their increasing density. This behavior promotes the transport of particles and organic matter into the sediment (Ouellette et al., 2004). Moreover, irrigation and degradation leave no time for storing the dissolved nutrients in amounts comparable to those at the offshore stations. Produced or released nutrients as well as settled POM cannot accumulate in the sediment because they are used immediately. Rusch et al. (2006) already reported muddy, diffusion dominated sediments to offer the highest amount of POM degeneration only at the surface. By contrast, their experiments showed that the permeable sands of the shelf area are effective biocatalytical filters with high microbial degradation rates due to percolation of POM loaded water down to 10 cm sediment depth.
The mismatch between the output and inventory of the sediments can also be explained by the fine fraction of the sediments itself (Leipe et al., 2017). Deep-burrowing fauna in the Bay of Gdansk, such as Marenzelleria ssp. and H. diversicolor, bury organic matter but also make buried POM available to microbial degradation processes by particle mixing and surface increment. Burrow walls constitute an increased sediment surface. Their ventilation provides habitats for microbes dependent on oxygen for the remineralization of organic matter. Thus, remineralization rates are enhanced in ventilated sediments. For example, a 300% increase of the oxygenated surface was reported for sediments inhabited by H. diversicolor (Davey, 1994) and a 6–11% increase of oxygenated sediment volume was shown for M. viridis (Jovanovic et al., 2014). The latter transports oxygenated water into the sediment most of the time, but the deeper parts of the burrows are oxygen depleted due to intense use of oxygen in aerobic microbial processes (Quintana et al., 2011; Jovanovic et al., 2014). Renz and Forster (2014) also reported the sediment water interface as the largest sink for oxygen in M. viridis and M. neglecta burrows due to increased nitrification activity. By contrast, at muddy, less-bioturbated stations remineralizing microbes can use only the organic material at the sediment surface, where oxygen from the bottom water is present. The higher volume of reworked sandy sediment and the larger bioturbation depth may thus compensate for the low organic carbon content that is due to a dilution of the fine fraction per volume. Also dissolved nutrients are transported by deep burrowing fauna like Marenzelleria spp. (Quintana et al., 2011; Jovanovic et al., 2014; Renz and Forster, 2014). Based solely on the efflux caused by bioirrigation of the reservoirs, higher fluxes from the sediment may be reached by large numbers of animals processing small amounts of nutrients repeatedly (Duport et al., 2006; De Backer et al., 2011) than by only a few animals processing large amounts less often.
Impact of Faunal Communities on Flux Modification
Amplification Factors and the Magnitude of TNF
The effects of physical advection by currents and wave action can be excluded from our TNF calculations because the incubated bottom water was completely enclosed by the chamber. The steadily decreasing oxygen concentrations inside the chamber ruled out the influence of the surrounding water (Figure 4). However, if physical advection as an impact on total fluxes is also considered, then our calculated fluxes may be slightly underestimated. Especially for permeable sands in shelf areas it is known that waves and currents can influence the fluid flow through the bottom. Bottom topography may also play a role, thus it was reported that sand ripple structures caused an increase of the sediment solute volume by a factor of 2.1 and the solute flux down to 2.7 cm sediment depth was enhanced by a factor of 1.9 due to pressure gradients (Huettel et al., 1996). Thus, advective solute flow in permeable sediment water layers is able to control the removal and offer of POM for degradation processes (Rusch et al., 2006; Rocha, 2008). This might be supported by hydraulic wave pumping where pressure gradients caused by wave influence cause an increased porewater flow through the sediment (Santos et al., 2012). Ahmerkamp et al. (2017) additionally showed that the increased oxygen supply caused by current velocities and depending on the sediment grain size requires higher microbial degradation rates in the sediment. They reported an oxygen penetration depth of 1–6 cm in coarse sands (210–540 μm) and an oxygen flux of 8 mmol to 34 mm m−2 d−1 only caused by physical transport and sediment characteristics. For our efflux calculations in the Bay of Gdansk this would mean a doubling in fluxes when physical advection contributes. Therefore, the complete efflux of the nutrient inventory in the sediment would take average only 0.8 days than 1.66 days as calculated from our incubation measurement. The physical advection might enhance the degradation potential of the sediments and cause a leaching of solutes from the permeable sands. Hence, the nutrient cycling in the coastal zone might probably be faster in reality than we estimated.
SiO2 is not governed by any microbial turnover processes; rather, total net SiO2 fluxes are a measure of macrofaunal influence because dissolved SiO2 can be treated as an inert tracer of bioturbation (Boudreau, 1997). All of the other nutrients contributed to turnover processes during the chamber lander incubations. This would explain why the highest AF occurred in the total net SiO2 fluxes, which were larger than the respective diffusive fluxes (Figure 6). Thus, the intrinsic effluxes of nutrients that undergo microbial transformation or use were probably underestimated. Consequently, in estimating the macrofaunal contribution to nutrient exchange, it might be more important to evaluate those factors of the TNF that cause it to exceed the diffusive fluxes than to focus on the fluxes themselves. An examination of the AF and TNF of different nutrients as a function of the dominant species of the benthic communities revealed interesting features related to their individual life strategies as following described. At station VE18, deep-burrowing Marenzelleria spp. and H. diversicolor dominated the community, with 814 and 369 ind. m−2, respectively. The total net SiO2 flux at that station was only a few mmol m−2 d−1 higher than at station VE07, where the abundances of these species were 44 and 0 ind. m−2, respectively. The second largest factor enhancing sedimentary SiO2 fluxes was at station VE18, where M. viridis might have reached a bioturbation depth of ~25 cm (maximum: 30 cm) (Zettler et al., 1994). Davey (1994) showed that the burrow systems of H. diversicolor reached a depth of 8 cm (small individuals) to 35 cm (large individuals). Thus, the establishment of deep reservoirs (1,620 μmol/l; station VE04) in the sediment was possible. Marenzelleria spp. irrigates nutrients dissolved in the porewater even more efficiently than does H. diversicolor (Kristensen et al., 2011), as demonstrated by the AF identified in our study that were especially high at stations where Marenzelleria spp. dominated the polychaete community (VE05, VE18, VE49a; Figure 6).
At station VE02, the large population of C. volutator may have accounted for a total flux that was 12 times higher than the diffusive flux, in contrast to the enhancement at station VE04 (AF 9.5). Together with the large populations of H. diversicolor, C. volutator may be capable of increasing the flux to this extent (Pelegri and Blackburn, 1994; Mermillod-Blondin et al., 2004). Moreover, the different excretion rates of the macrozoobenthos feed the pool (Blackburn and Henriksen, 1983; Gardner et al., 1993). For example, Tuominen et al. (1999) showed that a population of M. affinis can enlarge the flux in a system by up to 10%, due to excretion products. We speculate that at station VE04, where we found the highest TNF of 3.04 mmol m−2 d−1 and where the food supply was likely also high, excretion by the filter-feeding clam M. arenaria and the facultative deposit-feeding clam M. baltica, might also have contributed to the high flux since both were present at high abundances (1,140 and 1,041 ind. m−2, respectively). High excretion rates of Mya arenaria have long been known (Allen and Garrett, 1970) and filter-feeding clams are able to control phytoplankton stocks in shallow bights (Officier et al., 1982)
Additionally, remineralization stimulated by fauna might have increased the ammonium pool (Davey, 1994). At stations VE04, VE05, and VE18, where deep-burrowing polychaetes were found (Table 1) alongside high positive ammonium fluxes (Figure 5 and Supplementary Table 1), an increased oxygenated surface presumably caused ammonium effluxes of 0.65–3.04 mmol m−2 d−1. In studies of deep-sea sediment without fauna, the fluxes of differed from the fluxes at coastal sites. Berelson et al. (1990), for example, found that during a 4-day chamber lander incubation in the deep sea was below the detection limit. By contrast, we found detectable concentration changes (Figure 5) and TNF values measurable in mmol m−2 d−1 (Figure 6) after <1 day of incubation in shallow waters. At station VE04, where the reservoirs of dissolved nutrients were higher than at the other coastal stations (Supplementary Figure 1), deep-burrowing fauna may have created a high flux due to bioirrigation. This would suggest that H. diversicolor (225 ind. m−2 at VE04) was responsible for a doubling of the flux (Mermillod-Blondin et al., 2004) and therefore half of the increase at station VE04 (AF 9.5; Figure 6).
Total net fluxes were quite low and the calculated AF were in a similar range at all stations. This may have been caused by the precipitation reaction of phosphate with Fe(III)- ions to iron phosphate under aerobic conditions in the bottom water (Froehlich et al., 1982). Because of the low concentration of in the bottom water, the amount exiting the sediment might react in the oxygenated water immediately.
The CCA expresses the high influence of fauna on the TNF (Figure 9). In contrast, a second CCA showed that the TNF are uncorrelated to sediment properties and therefore not predictable. That means that the TNF are directly depending on the fauna community, which in turn depends on the sediment characteristics and location as discussed above. Thus, for a prediction of TNF the macrofauna community, the abundances in the functional groups and the availability of food sources must be considered.
Further, only the AF expresses the contribution of the macrofaunal community to flux modification in the coastal filter, not the high nutrient flux itself. This clearly implies that a comparison between total and diffusive fluxes is essential. The results suggest that it is mainly the occurrence and abundance of deep-burrowing polychaetes in a community that account for the enlargement of nutrient fluxes in the sediment, even if other species belong to the community in high abundances. The PCA showed that the depth of the sediments, which reflects the near to the shore and river mouth, and the faunal community are important determinants of the magnitude of the nutrient fluxes (Figure 8). The results further suggest that sediments consisting of well-mixed sand and mud and located in very shallow waters near the mouth of a river create the best conditions for turnover processes whereas sediments of mud alone seem to inhibit them. This might be why according to our data, oxygen consumption, TNF, and infauna occurrences were highest at stations nearest the mouth of the Vistula River and decreased progressively with increasing proximity to the offshore stations. The coastal zone therefore seems to buffer the highest loads of the Vistula River directly at the entry to the bay.
Flux Modification at the Study Site by Different Species According to Microbial Turnover Processes
is produced in the dissimilatory nitrate reduction that occurs in anoxic sediment layers (Laverock et al., 2011) or during microbial degradation of organic matter. Both processes feed the pool, either in the oxic zone of the sediment or bottom water or in the deeper, anoxic regions (Laverock et al., 2011). Conversely, an important -consuming process might be nitrification that occurs in oxygenated bottom water, and oxic sediment layers, or at the oxic-anoxic interfaces of tubes and burrows where ammonium may be converted to nitrate which may then be used during denitrification (e.g., Kristensen and Blackburn, 1987; Jäntti and Hietanen, 2012). In the absence of calculations of the rates of assimilation, remineralization, excretion, or nitrification, the process(es) with the strongest influence on production and transformation could not be determined.
While there was a net efflux from the sediments at all stations except VE04 where a negative flux appeared. This may imply a consumption of during denitrification in the sediments, which was assumed for all stations at the beginning of the incubation. Previous studies showed that H. virens activity stimulated the coupling of nitrification and denitrification by 2.3- to 2.4-fold (Kristensen and Blackburn, 1987). This may also have been due to the high supply, as a substrate for nitrification, at VE04. Karlson et al. (2005) showed that an increased supply from the bottom water supported sediment denitrification in cores inhabited by M. affinis. This was also the case for H. diversicolor in the study of Pelegri and Blackburn, 1995. Karlson et al. (2005) further proposed that, in the absence of appropriate denitrification rates, the high negative total flux in bioturbated cores reflected the high rates of dissimilatory nitrate reduction to ammonium. For all stations where accumulated in the bottom water, we speculated that nitrification was significantly higher than denitrification in the sediments or bottom water. Further, it may be that these processes were more weakly coupled than at station VE04. An example was provided by the large increase in the total net efflux at stations VE05 and VE02, which exceeded the diffusive porewater fluxes by an AF of 303 (VE05) despite the considerable amount of deep-burrowing tube and burrow dwellers inhabiting the sediments of these stations. While at station VE04 the conditions for denitrifiers were potentially good, due to the sediment characteristics near the river mouth, station VE05 lacked substrates for heterotrophs and probably included anoxic sediment areas that hindered the removal of from the system (Hellemann et al., 2017). This result is supported by a study of Henriksen et al. (1983), which showed that is oxidized to in burrow environments before it reaches the water column via bioirrigation. The opposite was probably true at station VE07, where the sedimentary conditions might have favored removal, but deep-burrowing animals were absent (Table 1, Figure 7). At that station, sedimentary denitrification and bottom water nitrification might have been nearly uncoupled, such that the removal processes were not enhanced.
The Coastal Filter Function and Bioturbation
Sediments of the coastal zone are generally a source of nutrients (Figures 5, 6), although variable for nitrate (this study Figure 6; Devol and Christensen, 1993; Sumei et al., 2004). For example, in sediments of the continental margin of the eastern Pacific, the TNF of SiO2 and were up to three times higher than the diffusive fluxes (Devol and Christensen, 1993). The AF determined in this study for sediments of the coastal zone were, in the case of SiO2, much higher (11.9–148, Figure 6), and provide an example of the potential differences in the enhancement of fluxes, as determined in the studies published thus far. Using chamber lander incubations, Berelson et al. (1990) showed that deep-sea sediments are a source of nutrients but the diffusive fluxes of the porewater were considered to be the main source of SiO2 and effluxes. The fluxes measured in their study were, on average, only a small fraction of those measured in the present study at the Bay of Gdansk. Forja et al. (1994) reported SiO2 and fluxes in the Mediterranean that were 6- to 13-fold higher than measured in this work; the difference can perhaps be explained by seasonal temperature changes (Forja et al., 1994). By contrast, in the core incubations in Bohai Sea, Sumei et al. (2004) did not find significant seasonal differences in the and SiO2 fluxes. Our study, however, was limited to winter and spring with the chamber Lander incubations for TNF calculation.
According to our calculations, 10% of the nitrogen in the Bay of Gdansk is introduced by inputs from the Vistula River reaching the bottom water system each year after being filtered and partly removed through the sediment in the coastal zone. The coastal filter is probably not a “one-way street” from the river through the sediments to the open Baltic Sea. More likely, a cycling process takes places as long as nutrients reach the bioturbated sediments and mixed water columns. Each nutrient cycle reduces bioavailability because every turnover process, including ingestion and digestion by fauna and microbial transformation, results in the transformation of nutrients to biomass. The retention of nutrients in the coastal zone would therefore lead to the temporal removal of nutrients bound in biomass. Coastal zones with long residence times and large numbers of bioturbating, deep-burrowing infauna may offer the best nutrient-filter function. Bioturbation is therefore not only a tool for determining the extent of the coastal filter but also an indispensable component thereof.
Summary
In this study, a significant, direct increase in the TNF compared to the diffusive fluxes in bioturbated sediments was determined, thus demonstrating that the sediments were a source of nearly all of the examined nutrients. The smaller increase in the TNF at the intermediate station reflected the lower level of bioturbation activity of infauna in those sediments than in the sediments of the coastal stations. The increase in the TNF differed for the different nutrients, with the highest TNF being that of SiO2. Consistent with the enhancement of fluxes due to bioturbation, stations close to the mouth of the Vistula River had the highest fluxes overall. In contrast, the stations with the largest number of deep-burrowing animals had the largest AF. Thus, the abundance (dominance) of burrow dwellers may determine the bioturbation effect of the whole community on the benthic system (Figures 6, 8, 9).
Our findings suggest that, each year, 6.9 kt N, 0.9 kt P, and 19 kt Si exit coastal sediments in the Bay of Gdansk and enter new transformation cycles in other parts of the marine system. For nitrogen, this is ~10% of the amount annually reaching the bay from the Vistula River.
In the offshore region of the Bay of Gdansk, the area of which is approximately twice that of the coastal zone (<50 m water depth), only 2.96 kt N, 6.8 kt Si, and 0.9 kt P exit the sediments each year. Thus, when extrapolated to the total area, the contribution of the coastal zone is roughly twice that of the offshore area.
Author Contributions
FT designed the study together with MV, did the sediment sampling, chamber lander, and porewater sampling and most of the analysis and the calculations and wrote the paper. MV additionally contributed to the text. UJ and HK did the macrofauna sampling and counting and contributed to the text. IL did the mass-spectrometric analyzes, CB performed the nutrient analyzes. MG conducted the area calculation and map design and contributed to the text. JD run the statistical analysis and contributed to the text.
Conflict of Interest Statement
The authors declare that the research was conducted in the absence of any commercial or financial relationships that could be construed as a potential conflict of interest.
The reviewer JW and handling Editor declared their shared affiliation.
Acknowledgments
We thank David Meyer (Leibniz Institute for Baltic Sea Research Warnemünde) for helpful discussions and comments. Thanks also to the captains and crews of RV Elisabeth Mann Borgese and RV Alkor for their help with sampling. Thanks to all BONUS-COCOA colleagues. The BONUS COCOA project was funded by BMBF grant number FKZ 03F063A. Finally we thank two reviewers for their helpful and constructive comments.
Supplementary Material
The Supplementary Material for this article can be found online at: https://www.frontiersin.org/articles/10.3389/fmars.2018.00201/full#supplementary-material
Abbreviations
TNF, total net fluxes; AF, amplification factor; LOI, loss on ignition.
References
Ahmerkamp, S., Winter, C., Krämer, K., de Beer, D., Janssen, F., Friedrich, J., et al. (2017). Regulation of benthic oxygen fluxes in permeable sediments of the coastal ocean. Limnol. Oceanogr. 62, 1935–1954. doi: 10.1002/lno.10544
Allen, J. A., and Garrett, M. R. (1970). The excretion of ammonia and urea by Mya arenaria L. (Mollusca: Bivialvia). Comp. Biochem. Phys. A 39, 633–642. doi: 10.1016/0300-9629(71)90185-X
Aller, R. C., and Aller, J. Y. (1998). The effect of biogenic irrigation intensity and solute exchange on diagenetic reaction rates in marine sediments. J. Mar. Res. 56, 905–936. doi: 10.1357/002224098321667413
Andersen, F. Ø., and Kristensen, E. (1992). The importance of benthic macrofauna in decomposition of microalgae in a coastal marine sediment. Limnol. Oceanogr. 37, 1392–1403. doi: 10.4319/lo.1992.37.7.1392
Asmala, E., Carstensen, J., Conley, D. J., Slomp, C. P., Stadmark, J., and Voss, M. (2017). Efficiency of the coastal filter: nitrogen and phosphorous removal in the Baltic Sea. Limnol. Oceanogr. 62, 222–238. doi: 10.1002/lno.10644
Berelson, W. M., Hammond, D. E., O'Neill, D., Xu, X.-M., Chin, C., and Zukin, J. (1990). Benthic fluxes and pore water studies from sediments of the central equatorial north Pacific: nutrient diagenesis. Geochim. Cosmochim. Acta 54, 3001–3012. doi: 10.1016/0016-7037(90)90117-4
Blackburn, T. H., and Henriksen, K. (1983). Nitrogen cycling in different types of sediment from Danish waters. Limnol. Oceanogr. 28, 477–493. doi: 10.4319/lo.1983.28.3.0477
Bouma, T. J., Olenin, S., Reise, K., and Ysebaert, T. (2009). Ecosystem engineering and biodiversity in coastal sediments: posing hypothesis. Helgol. Mar. Res. 63, 95–106. doi: 10.1007/s10152-009-0146-y
Conley, D. J., Carstensen, J., Aigars, J., Axell, P., Bonsdorff, E., Eremenia, T., et al. (2011). Hypoxia is increasing in the coastal zone of the Baltic Sea. Environ. Sci. Technol. 45, 6777–6783. doi: 10.1021/es201212r
Davey, J. T. (1994). The architecture of the burrow of Nereis diversicolor and its quantification in relation to sediment-water exchange. J. Exp. Mar. Biol. Ecol. 179, 115–129. doi: 10.1016/0022-0981(94)90020-5
De Backer, A., van Coillie, F., Montserrat, F., Provoost, P., van Colen, C., Vincx, M., et al. (2011). Bioturbation effects of Corophium volutator: importance of density and behavioural activity. Estuar. Coast. Shelf Sci. 91, 306–313. doi: 10.1016/j.ecss.2010.10.031
Denisenko, S. G., Denisenko, N. V., Lehtonen, K. K., Andersin, A.-B., and Laine, A. O. (2003). Macrozoobenthos of the Pechora Sea (SE Barents Sea): community structure and spatial distributions in relation to environmental conditions. Mar. Ecol. Prog. Ser. 258, 109–123. doi: 10.3354/meps258109
Davey, J. T. (1994). The architecture of the burrow of Nereis diversicolor and its quantification in relation to sediment-water exchange. J. Exp. Mar. Bio. Ecol. 179, 115–129.
Devine, J. A., and Vanni, M. J. (2002). Spatial and seasonal variation in nutrient excretion by benthic invertebrates in a eutrophic reservoir. Freshw. Biol. 47, 1107–1121. doi: 10.1046/j.1365-2427.2002.00843.x
Devol, A. H., and Christensen, J. P. (1993). Benthic fluxes and nitrogen cycling in sediments of the continental margin of the eastern North Pacific. J. Mar. Res. 51, 345–372. doi: 10.1357/0022240933223765
Diaz, R. J., and Rosenberg, R. (1995). Marine benthic hypoxia: a review of its ecological effects, and the behavioural responses of benthic macrofauna. Oceanogr. Mar. Biol. 33, 245–303.
Diaz, R. J., and Rosenberg, R. (2008). Spreading dead zones and consequences for marine ecosystems. Science 321, 926–929. doi: 10.1126/science.1156401
Dippner, J. W., and Ikauniece, A. (2001). Long-term zoobenthos variability in the Gulf of Riga in relation to climate variability. J. Mar. Syst. 30, 155–164. doi: 10.1016/S0924-7963(01)00055-0
Duport, E., Stora, G., Tremblay, P., and Gilbert, F. (2006). Effects of population density on the sediment mixing induced by the gallery- diffuser Hediste (Nereis) diversicolor O.F. Müller, 1776. J. Exp. Mar. Biol. Ecol. 336, 33–41. doi: 10.1016/j.jembe.2006.04.005
Dürr, H. H., Laruelle, G. G., van Kempen, C. M., Slomp, C. P., Meybeck, M., and Middelkoop, H. (2011). Worldwide typology of nearshore coastal systems: defining the estuarine filter of river inputs to the oceans. Estuar. Coasts 3, 441–458. doi: 10.1007/s12237-011-9381-y
Flemming, B. W., and Delafontaine, M. T. (2000). Mass physical properties of muddy intertidal sediments: some applications, misapplications and non- applications. Cont. Shelf Res. 20, 1179–1197. doi: 10.1016/S0278-4343(00)00018-2
Forja, J. M., Blasco, J., and Gómez- Parra, A. (1994). Spatial and seasonal variation of in situ benthic fluxes in the Bay of Cadiz (south- west Spain). Estuar. Coast. Shelf Sci. 39, 127–141. doi: 10.1006/ecss.1994.1053
Froehlich, P. N., Bender, M. L., Luedtke, N. A., Heath, G. R., and De Vries, T. (1982). The marine phosphorus cycle. Am. J. Sci. 282, 474–511. doi: 10.2475/ajs.282.4.474
Gardner, W. S., Briones, E. E., Kaegi, E. C., and Rowe, G. T. (1993). Ammonium excretion by benthic invertebrates and sediment-water nitrogen flux in the Gulf of Mexico near the Mississippi River outflow. Estuar. Coast 16, 799–808. doi: 10.2307/1352438
Gilbert, F., Stora, G., and Bonin, P. (1998). Influence of bioturbation on denitrification activity in Mediterranean coastal sediments: an in situ experimental approach. Mar. Ecol. Prog. Ser. 163, 99–107. doi: 10.3354/meps163099
Glud, R. N., and Blackburn, N. (2002). The effects of chamber size on benthic oxygen uptake measurements: a simulation study. Ophelia 56, 23–31. doi: 10.1080/00785236.2002.10409486
Glud, R. N., Holby, O., Hoffmann, F., and Canfield, D. E. (1998). Benthic mineralization and exchange in arctic sediments. Mar. Ecol. Prog. Ser. 173, 237–251. doi: 10.3354/meps173237
Graf, G., and Rosenberg, R. (1997). Bioresuspension and biodeposition: a review. J. Mar. Syst. 11, 269–278. doi: 10.1016/S0924-7963(96)00126-1
Grasshoff, K., Kremling, K., and Ehrhardt, M. (1999). Methods of Seawater Analysis, 3rd Edn. Weinheim: Wiley-VCH.
Heisterkamp, I. M., Kamp, A., Schramm, A. T., de Beer, D., and Stief, P. (2012). Indirect control of the intracellular nitrate pool of intertidal sediment by the polychaete Hediste diversicolor. Mar. Ecol. Prog. Ser. 445, 181–192. doi: 10.3354/meps09464
Hellemann, D., Tallberg, P., Bartl, I., Voss, M., and Hietanen, S. (2017). Denitrification in an oligotrophic estuary: a delayed sink for riverine nitrate. Mar. Ecol. Prog. Ser. 583, 63–80. doi: 10.3354/meps12359
Henriksen, K., Rasmussen, M. B., and Jensen, A. (1983). Effect of bioturbation on microbial nitrogen transformations in the sediment and fluxes of ammonium and nitrate to the overlying water. Environ. Biogeochem. 35, 193–205.
Heyen, H., and Dippner, J. W. (1998). Salinity variability in the German Bight in relation to climate variability. Tellus 50, 545–556. doi: 10.3402/tellusa.v50i4.14532
Huettel, M., Ziebis, W., and Forster, S. (1996). Flow- induced uptake of particulate matter in permeable sediments. Limnol. Oceanogr. 41, 309–322. doi: 10.4319/lo.1996.41.2.0309
Iversen, N., and Jørgensen, B. B. (1993). Diffusion coefficients of sulfate and methane in marine sediments: influence of porosity. Geochim. Cosmochim. Acta 57, 571–578. doi: 10.1016/0016-7037(93)90368-7
Janas, U., Wocial, J., and Szaniawska, A. (2004). Seasonal and annual changes in the macrozoobenthic populations of the Gulf of Gdansk with respect to hypoxia and hydrogen sulphide. Oceanologia 46, 85–102.
Jäntti, H., and Hietanen, S. (2012). The effects of hypoxia on sediment nutrient cycling in the Baltic Sea. Ambio 42, 161–169. doi: 10.1007/s13280-011-0233-6
Jørgensen, B. B. (1980). Seasonal oxygen depletion in the bottom waters of a danish fjord and its effect on the benthic community. Oikos 34, 68–76. doi: 10.2307/3544551
Jovanovic, Z., Larsen, M., Quintana, C. O., Kristensen, E., and Glud, R. N. (2014). Oxygen dynamics and porewater transport in sediments inhabited by the invasive polychaete Marenzelleria viridis. Mar. Ecol. Prog. Ser. 504, 181–192. doi: 10.3354/meps10737
Karlson, K. (2007). Diurnal bioturbating activities of Monoporeia affinis: effects on benthic oxygen and nutrient fluxes. Mar. Ecol. Prog. Ser. 331, 195–205. doi: 10.3354/meps331195
Karlson, K., Hulth, S., Ringdahl, K., and Rosenberg, R. (2005). Experimental recolonisation of Baltic Sea reduced sediments: survival of benthic macrofauna and effects on nutrient cycling. Mar. Ecol. Prog. Ser. 294, 35–49. doi: 10.3354/meps294035
Kristensen, E., and Blackburn, T. H. (1987). The fate of organic carbon and nitrogen in experimental marine sediment systems: influence of bioturbation and anoxia. J. Mar. Res. 45, 231–257. doi: 10.1357/002224087788400927
Kristensen, E., Hansen, T., Delefosse, M., Banta, G. T., and Quintana, C. O. (2011). Contrasting effects of the polychaetes Marenzelleria viridis and Nereis diversicolor on benthic metabolism and solute transport in sandy coastal sediment. Mar. Ecol. Prog. Ser. 425, 125–139. doi: 10.3354/meps09007
Kröncke, I., Reiss, H., and Dippner, J. W. (2013). Effects of cold winters and regime shifts on macrofauna communities in the southern North Sea. Estuar. Coast. Shelf Sci. 119, 79–90. doi: 10.1016/j.ecss.2012.12.024
Künitzer, A., Basford, D., Craeymeersch, J. A., Dewarumez, J. M., Dörjes, J., Duineveld, G. C. A., et al. (1992). The benthic infauna of the North Sea: species distribution and assemblages. J. Mar. Sci. 49, 127–143. doi: 10.1093/icesjms/49.2.127
Laverock, B., Gilbert, J. A., Tait, K., Osborn, A. M., and Widdicombe, S. (2011). Bioturbation: impact on the marine nitrogen cycle. Biochem. Soc. Trans. 39, 315–320. doi: 10.1042/BST0390315
Leipe, T., Naumann, M., Tauber, F., Radtke, H., Friedland, R., Hiller, A., et al. (2017). Regional distribution patterns of chemical parameters in surface sediments of the south- western Baltic Sea and their possible causes. Geo. Mar. Lett. 37, 593–606. doi: 10.1007/s00367-017-0514-6
Leipe, T., Tauber, F., Vallius, H., Virtasalo, J., Uścinowicz, S., Kowalski, N., et al. (2011). Particulate organic carbon (POC) in surface sediments of the Baltic Sea. Geol. Mar. Lett. 31, 175–188. doi: 10.1007/s00367-010-0223-x
Livezey, R. E. (1995). “The evaluation of forecast,” in Analysis of Climate Variability, eds H. Von Storch and A. Navarra(Berlin: Springer Verlag), 177–196.
Long, C. W., Brylawski, B. J., and Seitz, R. D. (2008). Behavioral effects of low dissolved oxygen on the bivalve Macoma balthica. J. Exp. Mar. Biol. Ecol. 359, 34–39. doi: 10.1016/j.jembe.2008.02.013
Longuet- Higgins, M. S. (1970). Longshore currents generated by obliquely incident sea waves 1. J. Geophys. Res. 75, 6778–6789. doi: 10.1029/JC075i033p06778
Mermillod-Blondin, F., Rosenberg, R., François-Carcaillet, F., Norling, K., and Maulaire, L. (2004). Influence of bioturbation by three benthic infaunal species on microbial communities and biogeochemical processes in marine sediment. Aquat. Microb. Ecol. 36, 271–284. doi: 10.3354/ame036271
Moodley, L., Heip, C. H. R., and Middelburg, J. J. (1998). Benthic activity in sediments of the northwestern Adriatic Sea: sediment oxygen consumption, macro- and meiofauna dynamics. J. Sea Res. 40, 263–280. doi: 10.1016/S1385-1101(98)00026-4
Mortimer, R. J. G., Davey, J. T., Krom, M. D., Watson, P. G., Frickers, P. E., and Clifton, R. J. (1999). The effect of macrofauna on porewater profiles and nutrient fluxes in the intertidal zone of the Humber Estuary. Estuar. Coast. Shelf Sci. 48, 683–699. doi: 10.1006/ecss.1999.0479
Norkko, A., Hewitt, J. E., Thrush, S. F., and Funnell, G. A. (2001). Benthic- pelagic coupling and suspension- feeding bivalves: linking site- specific sediment flux and biodeposition to benthic community structure. Limnol. Oceanogr. 46, 2067–2072. doi: 10.4319/lo.2001.46.8.2067
Officier, C. B., Smayda, T. J., and Mann, R. (1982). Benthic filter feeding: a natural eutrophication control. Mar. Ecol. Prog. Ser. 9, 203–210. doi: 10.3354/meps009203
Pastuszak, M., and Witek, Z. (2012). “Discharges of water and nutrients by the Vistula and Oder rivers draining Polish territory,” in Temporal and Spatial Differences in Emission of Nitrogen and Phosphorus from Polish Territory to the Baltic Sea, eds M. Pastuszak and J. Igras (Gdynia: National Marine Fisheries Research Institute; Institute of Soil Science and Plant Cultivation; Fertilizer Research Institute), 309–346.
Pelegri, S. P., and Blackburn, T. H. (1994). Bioturbation effects of the amphipod Corophium volutator on microbial nitrogen transformations in marine sediments. Mar. Biol. 121, 253–258. doi: 10.1007/BF00346733
Pelegri, S. P., and Blackburn, T. H. (1995). Effect of bioturbation by Nereis sp., Mya arenaria and Cerastoderma sp. on nitrification and denitrification in estuarine sediments. Ophelia 42, 289–299. doi: 10.1080/00785326.1995.10431509
Phillips, O. M. (1966). The Dynamic of the Upper Ocean, 2nd Edn. Cambridge, NY: Cambridge University Press.
Ouellette, D., Desrosiers, J.-P. G., Gilbert, F., Poggiale, J.-C., Blier, P. U., and Stora, G. (2004). Effects of temperature on in vitro sediment reworking processes by a gallery diffusor, the polychaete Neanthes virens. Mar. Ecol. Prog. Ser. 266, 185–193. doi: 10.3354/meps266185
Quintana, C. O., Hansen, T., Delefosse, M., Banta, G., and Kristensen, E. (2011). Burrow ventilation and associated porewater irrigation by the polychaete Marenzelleria viridis. J. Exp. Mar. Biol. Ecol. 397, 179–187. doi: 10.1016/j.jembe.2010.12.006
Reid, J. M., Reid, J. A., Jenkins, C. J., Hastings, M. E., Williams, S. J., and Poppe, L. J. (2005). usSEABED: Atlantic Coast Offshore Surficial Sediment Data Release. (Reston, VA: U.S. Geological Survey Data Series 118, version 1.0).
Renz, J., and Forster, S. (2014). Effects of bioirrigation by the three sibling species of Marenzelleria spp. on solute fluxes and porewater nutrient profiles. Mar. Ecol. Prog. Ser. 505, 145–159. doi: 10.3354/meps10756
Rocha, C. (2008). Sandy sediments as active biogeochemical reactors: compound cycling in the fast lane. Aquat. Microb. Ecol. 53, 119–127. doi: 10.3354/ame01221
Rosenberg, R., Loo, L.-O., and Möller, P. (1992). Hypoxia, salinity and temperature as structuring factors for marine benthic communities in a eutrophic area. Neth. J. Sea Res. 30, 121–129. doi: 10.1016/0077-7579(92)90051-F
Rusch, A., Huettel, M., Wild, C., and Reimers, C. (2006). Benthic oxygen consumption and organic matter turnover in organic- poor, permeable shelf sands. Aquat. Geochem. 12, 1–9. doi: 10.1007/s10498-005-0784-x
Santos, I. R., Eyre, B. D., and Huettel, M. (2012). The driving forces of porewater and groundwater flow in permeable coastal sediments: a review. Estuar. Coast Shelf Sci. 98, 1–15. doi: 10.1016/j.ecss.2011.10.024
Schwinghamer, P. (1981). Characteristic size distributions of integral benthic communities. Can. J. Fish. Aquat. Sci. 38, 1255–1263. doi: 10.1139/f81-167
Seeberg- Elverfeldt, J., Schlüter, M., Feseker, T., and Kölling, M. (2005). Rhizon sampling of pore waters near the sediment- water interface of aquatic systems. Limnol. Oceanogr. Methods 3, 361–371. doi: 10.4319/lom.2005.3.361
Sumei, L., Jing, Z., Hongtao, C., and Raabe, T. (2004). Benthic nutrient recycling in shallow coastal waters of the Bohai Sea. Chin. J. Oceanol. Limnogr. 22, 365–372. doi: 10.1007/BF02843630
Tuominen, L., Mäkelä, K., Lehtonen, K. K., Haahti, H., Hietanen, S., and Kuparinen, J. (1999). Nutrient fluxes, porewater profiles and denitrification in sediment influenced by algal sedimentation and bioturbation by Monoporeia affinis. Estuar. Cost. Shelf Sci. 49, 83–97. doi: 10.1006/ecss.1999.0492
von Storch, H., Zorita, E., and Cubasch, U. (1993). Downscaling of global climate change estimates to regional scales: an application to Iberian rainfall in wintertime. J. Clim. 6, 1161–1171. doi: 10.1175/1520-0442(1993)006<1161:DOGCCE>2.0.CO
Voss, M., Liskow, I., Pastuszak, M., Rüβ, D., Schulte, U., and Dippner, J. W. (2005). Riverine discharge into a coastal bay: a stable isotope study in the Gulf of Gdansk, Baltic Sea. J. Mar. Syst. 57, 127–145. doi: 10.1016/j.jmarsys.2005.04.002
Warwick, R. M., and Uncles, R. J. (1980). Distribution of benthic macrofauna associations in the Bristol Channel in relation to tidal stress. Mar. Ecol. Prog. Ser. 3, 97–103. doi: 10.3354/meps003097
Wilson, A. M., Huettel, M., and Klein, S. (2008). Grain size and depositional environment as predictors of permeability in coastal marine sands. Estuar. Coast. Shelf Sci. 80, 193–199. doi: 10.1016/j.ecss.2008.06.011
Witek, Z., Humborg, C., Savchuk, O., Grelowski, A., and Łysiak-Pastuszak, E. (2003). Nitrogen and phosphorus budgets of the Gulf of Gdansk (Baltic Sea). Estuar. Coast. Shelf Sci. 57, 239–248. doi: 10.1016/S0272-7714(02)00348-7
Wright, P. A. (1995). Nitrogen excretion: three end products, many physiological roles. J. Exp. Biol. 198, 273–281.
Keywords: bioturbation, chamber lander, macrofaunal communities, nutrient fluxes, porewater fluxes, coastal filter, coastal sediments, Baltic Sea
Citation: Thoms F, Burmeister C, Dippner JW, Gogina M, Janas U, Kendzierska H, Liskow I and Voss M (2018) Impact of Macrofaunal Communities on the Coastal Filter Function in the Bay of Gdansk, Baltic Sea. Front. Mar. Sci. 5:201. doi: 10.3389/fmars.2018.00201
Received: 07 December 2017; Accepted: 18 May 2018;
Published: 08 June 2018.
Edited by:
Karol Kulinski, Institute of Oceanology (PAN), PolandReviewed by:
Jan Marcin Weslawski, Institute of Oceanology (PAN), PolandNafsika Papageorgiou, Hellenic Centre for Marine Research, Greece
Copyright © 2018 Thoms, Burmeister, Dippner, Gogina, Janas, Kendzierska, Liskow and Voss. This is an open-access article distributed under the terms of the Creative Commons Attribution License (CC BY). The use, distribution or reproduction in other forums is permitted, provided the original author(s) and the copyright owner are credited and that the original publication in this journal is cited, in accordance with accepted academic practice. No use, distribution or reproduction is permitted which does not comply with these terms.
*Correspondence: Franziska Thoms, franziska.thoms@io-warnemuende.de