- 1GEOMAR Helmholtz Centre for Ocean Research Kiel, Kiel, Germany
- 2State Key Laboratory of Tropical Oceanography, South China Sea Institute of Oceanology, Chinese Academy of Sciences, Guangzhou, China
- 3Daya Bay Marine Biology Research Station, Chinese Academy of Sciences, Shenzhen, China
- 4Institute of Physical Chemistry, Kiel University, Kiel, Germany
- 5KMS Kiel Marine Science-Centre for Interdisciplinary Marine Science, Kiel University, Kiel, Germany
The surface microlayer (SML) is the uppermost thin layer of the ocean and influencing interactions between the air and sea, such as gas exchange, atmospheric deposition and aerosol emission. Organic matter (OM) plays a key role in air-sea exchange processes, but studying how the accumulation of organic compounds in the SML relates to biological processes is impeded in the field by a changing physical environment, in particular wind speed and wave breaking. Here, we studied OM dynamics in the SML under controlled physical conditions in a large annular wind wave channel, filled with natural seawater, over a period of 26 days. Biology in both SML and bulk water was dominated by bacterioneuston and -plankton, respectively, while autotrophic biomass in the two compartments was very low. In general, SML thickness was related to the concentration of dissolved organic carbon (DOC) but not to enrichment of DOC or of specific OM components in the SML. Pronounced changes in OM enrichment and molecular composition were observed in the course of the study and correlated significantly to bacterial abundance. Thereby, hydrolysable amino acids, in particular arginine, were more enriched in the SML than combined carbohydrates. Amino acid composition indicated that less degraded OM accumulated preferentially in the SML. A strong correlation was established between the amount of surfactants coverage and γ-aminobutric acid, suggesting that microbial cycling of amino acids can control physiochemical traits of the SML. Our study shows that accumulation and cycling of OM in the SML can occur independently of recent autotrophic production, indicating a widespread biogenic control of process across the air-sea exchange.
Introduction
Wind, waves and temperature are expected to control the exchange of heat, mass and momentum between the surface ocean and the lower atmosphere. Besides these physical factors, the thin interfacial layer between the air and the ocean, referred to as sea surface microlayer (SML), has increasingly gathered attention in modulating air-sea gas exchange (Upstill-Goddard et al., 2003; Frew, 2005; Cunliffe et al., 2013) as well as for the emission of primary organic aerosols that may act as cloud condensation nuclei (CCN) in the atmosphere, and even induce ice-crystal formation (Russell et al., 2010; Quinn and Bates, 2011; Wilson et al., 2015). High wind speed conditions typically induce wave breaking and bubble entrainment. When bubbles burst at the sea surface, sea spray emitted to the atmosphere includes organic matter (OM) from the bubble film as well as from the SML. This material can dry out in air and become nuclei for water vapor condensation, i.e., marine primary organic aerosol. Biogenic compounds released by microorganisms inhabiting the SML, referred to as neuston, or inhabiting the water column below, referred to as plankton, can accumulate in the SML and modify air-sea gas exchange rates in several ways: particulate OM may act as a physical barrier, while dissolved surface active agents (surfactants) may lower the surface tension and thereby damp small capillary waves, subsequently affecting sea surface hydrodynamics (McKenna and McGillis, 2004). Biogenic production or consumption of gaseous compounds within the SML can also directly enhance or reduce the air-sea exchange as demonstrated for CO, H2, CH4, N2O (Conrad and Seiler, 1988; Frost, 1999; Upstill-Goddard et al., 2003; Nakajima et al., 2013). Extracellular enzymes that catalyze chemical reactions, such as extracellular carbonic anhydrase that enhances the interconversion of and CO2, may be enriched in the SML, resulting in air-sea CO2 fluxes higher than expected from surface water carbonate chemistry (Mustaffa et al., 2017).
At present, there is little information about the chemical nature of surfactants in seawater, and how they relate to biological productivity. Laboratory experiments have suggested a strong suppression of gas exchange by exudates that are released by phytoplankton, such as carbohydrates (Goldman et al., 1988; Frew et al., 1990), similar to the effect observed for synthetic surfactants (Bock et al., 1999; Frew, 2005) added to seawater. Other studies have demonstrated that marine bacteria can be potent producers of surfactants (see review by Satpute et al., 2010). In particular under low wind speed, slick-type accumulations of OM in the SML can form and have been hypothesized to reduce gas exchange rates by 15% (Wurl et al., 2016). Quantifying effects of surface films on gas-exchange in the ocean, however, remains challenging due to a high spatial and temporal variability in the total amount of surfactants and in the chemical composition of the SML (Engel et al., 2017). Moreover, surfactant accumulations, visible as slicks, tend to dissipate quickly under turbulent conditions (Frew et al., 1990; Bock et al., 1999).
Organic components in the SML are furthermore expected to chemically interact with compounds entering the ocean via the atmosphere such as nutrients and dust (Astrahan et al., 2016). Organic macromolecules in the SML are known to form complexes with trace metals and may thus bind metal ions from atmospheric depositions, e.g., iron, before these enter deeper into the water column (Hardy et al., 1985). Moreover, heterogeneous oxidation processes as well as surface specific photosensitized reactions can transform OM into volatile compounds (Carpenter and Nightingale, 2015; Ciuraru et al., 2015). Hence, OM in the SML plays a pivotal role in exchange processes between the ocean and atmosphere under a wide range of physical conditions, including low as well as high wind speeds.
Due to frequent variations in environmental conditions, such as in wind speed, precipitation, solar radiation, temperature and waves, the current understanding of processes controlling the chemical and biological composition of the SML in marine environments is rather poor. How accumulations of specific organic components in the SML relate to biological processes in the water column is largely unknown. Despite the fact that the SML is a distinct habitat for neuston species living preferentially at the air-sea interface, it is clearly linked to the chemistry and biology of the underlying water (Kuznetsova and Lee, 2001; Matrai et al., 2008; Lindroos et al., 2011). Previous studies showed that the accumulation of some organic components, such as carbohydrates, transparent exopolymere particles (TEP) and cell abundances in the SML can be directly related to their abundance in the surface water column (Wurl et al., 2011a; Engel and Galgani, 2016; Galgani et al., 2016). A coupling between autotrophic production in the water column and accumulation of OM in the SML could explain high organic aerosol emission over the ocean at times of phytoplankton blooms (O'Dowd et al., 2004). Especially under calm weather conditions, algae can accumulate and influence OM composition in the SML (Joux et al., 2006). Other studies reported that the high organic enrichment in aerosols can be correlated to the abundance of heterotrophic bacteria and to dimethyl sulfide concentration as an indicator for oxidative stress and phytoplankton cell lysis (Bates et al., 2012; Prather et al., 2013). Earlier studies on visible surface films, i.e., slicks in coastal areas suggested that systems with high productivity supported by eutrophic conditions, favor SML formation with consequences for air-sea exchange processes (Frew, 2005). More recent studies instead emphasized a global pattern of OM enrichment in the SML, with surfactant activity in the open ocean being comparable to coastal sites even at high wind speed (Wurl et al., 2011b; Sabbaghzadeh et al., 2017; van Pinxteren et al., 2017). Hence, evidence from field studies on what controls SML formation and composition, and how variations in SML characteristic may influence air-sea exchange processes is sporadic and rather conflicting.
In order to investigate air-sea exchange processes under controlled wind conditions for seawater containing biogenic matter in both dissolved and particulate form, as well as to better understand influences of changes in seawater biology and chemistry on air-sea exchange processes, the SOPRAN Aeolotron study was conducted in 2014. The Aeolotron at the University of Heidelberg, Germany, is a large scale facility that includes an annular wind wave channel, where a wind speed (U10, scaled to the reference height of 10 m) of up to 20 m s−1 can be realized (Nagel et al., 2015). Here, we report on the accumulation of organic components in the SML associated with heterotrophic and autotrophic microbial communities at low wind condition (U10: 1.3–1.5 m s−1) and over several days. Particular attention was paid to biopolymers that have been suggested to affect air-sea exchanges processes, including hydrolysable amino acids, high molecular weight combined carbohydrates and surfactants.
Materials and Methods
Seawater Collection and Transport
22,000 L of sea water were collected in September 2014 by the RV Poseidon: about 14,000 L were collected at 55 m depth near the Sula Reef Complex in the North Atlantic (64° 4,90' N 8° 2,03' E), an additional 8,000 L were collected from a surface water source at 5 m depth near the Island of Sylt in the German Bight, North Sea. The water from both sources was mixed and stored in the ship's seawater tank in the dark until the end of the cruise. In home port, the water was pumped into a clean (“food safe”) road tanker on the 22.09.2014 and was simultaneously filtered through a rough gravel filter to remove larger particles. The water was unloaded at the wind wave facility Aeolotron in Heidelberg the following day and stored in the dark and cool (~10°C) until the start of the experiment.
Experimental Conditions and Treatments
The Aeolotron is a circular tank facility, 8.68 m inner diameter, 0.61 m width, and about 17.9 m3 water volume when filled to 1 m height. Total surface area of still seawater in the tank is 18.4 m2. More detailed description of the facility is given by Krall (2013) and by Nagel et al. (2015). On November 3rd 2014 (day 1) the experiment started. However, due to a break in the bubble generator, seawater was fully drained from the system on November 5th (day 3) and refilled the same day. Sampling of chemical and biological development in the bulk seawater and in the SML was conducted until November 27 (day 25). Seawater temperature over the course of the experiment was about 21°C.
To assess variations in the composition of the SML in response to environmental changes, we modified light, nutrients, and phytoplankton abundance during the study. In addition, SML previously collected during a phytoplankton bloom experiment was added to simulate slick coverage. A timeline of the study and of experimental interventions is given in Figure 1.

Figure 1. Timeline of activities carried out during the Aeolotron experiment in 2014. Filled boxes: no light, open boxes: light, diagonal lines: SML sampling, circles: wind experiments, crossed circles: wind and bubbling experiments. Indicated are also the days when nutrients, a culture of Emiliania huxleyi or a SML sample from a previous phytoplankton bloom experiment were added.
Light sources were operated over the tank between days 9 and 16 and days 20 and 25, with a light:dark cycle of 11 h:13 d. When the light was switched on, Photosynthetically Active Photon Flux Density (PFD) at the water surface was about 115–120 μmol m−2 s−1 over about 20 m of the tank perimeter, the remaining about 20 μmol m−2 s−1. The water inside the tank was well mixed and circulated during daytime throughout the experiment by wind driven currents and bubbling, carried out on days 2, 4, 5, 9, 11, 15, 22, and 23.
At the evening of day 12, inorganic nutrients were added to the tank to increase the concentrations of nitrate (NO3) and silicate (SiO4) and phosphate (PO4) to 14.7, 9.5, and 0.48 μmol L−1, respectively. About 800 mL of a culture of Emiliania huxleyi (cell density: 4.6 × 105 cells ml−1) was added on day 20 to induce phytoplankton growth and exudation. In addition, 6 L of biogenic microlayer from a previous phytoplankton mesocosm experiment, which had been stored frozen at −20° for about 6 months was added on day 21.
Wind in the air space (24.4 m3) above the water was generated by two axial fans mounted diametrically in the ceiling of the air space of the flume. During the Aeolotron study a total of 9 wind experiments were conducted, with stepwise increase in wind speeds (U10) ranging from 1.4 to 23.7 m s−1. Following the highest wind speed, bubbling of seawater was induced for 1 h to simulate wave breaking. Bubbles were generated with a profiO2 oxygen diffuser hose. About 54 meters of this tubing were installed at the bottom of the wind-wave channel and were operated with an over pressure of around 900 mbar with air taken from the air space of the Aeolotron at a flow rate of around 100 L per minute. Results of the wind experiments, e.g., on gas exchange and surface dynamics, will be reported elsewhere.
Sampling
The SML inside the wind wave channel was sampled on 12 days applying the glass plate technique (Harvey and Burzell, 1972), described in more detail by Engel and Galgani (2016) and summarized here briefly. An acid (HCl 10%) and Milli-Q cleaned plate of 500 × 200 × 5 mm made of borosilicate glass and with an effective sampling surface area of 2,000 cm2 (considering both sides) was inserted into the water perpendicular to the surface and withdrawn at a rate of ~20 cm s−1. For each sample, this procedure was repeated between 23 and 48 times and the exact number of dips and total volume of water were recorded. The samples were directly filled into an acid (HCl 10%) and Milli-Q cleaned borosilicate glass bottle, pre-rinsed with about 20 mL of sample.
All SML samples reported in this study were collected in the morning at low wind speed (u10, representing the calculated with speed 10 m above the water surface) of 1.3–1.5 m s−1. Bulk water was sampled at least once a day; i.e., always in the morning and at low wind speed, and occasionally also in the evening, except for day 6. Bulk water samples were collected from an outlet at about 50 cm depth equivalent to half the height of the water column. Samples of about 500 mL were filled into an acid (HCl 10%) and Milli-Q cleaned borosilicate glass bottle, pre-rinsed with about 20 mL of sample.
Analysis of Chemical Parameters
Inorganic Nutrients
Nitrate, silicate and phosphate in bulk seawater were analyzed according to Hansen and Koroleff (1999). Twenty five milliliter samples were filtered in duplicate with 0.2 μm syringe filters and stored frozen at −20°C until analysis on a spectrophotometer.
Particulate Organic Carbon (POC) and Particulate Nitrogen (PN)
Between 200 and 400 mL of bulk seawater were filtered (<200 mbar) in duplicate, onto combusted (8 h at 500°C) GF/F filters (Whatmann, 25 mm) and stored frozen (−20°C) until analysis. To remove carbonate, filters were exposed to fuming hydrochloric acid in a fuming box for ~12 h and dried subsequently (60°C, 12 h). Filters were wrapped in tin cups and measured using an Euro EA elemental analyzer calibrated with an acetanilide standard.
Dissolved Organic Carbon (DOC)
For DOC, samples (20 ml) were collected in duplicate from the SML and bulk water, filtered through combusted (8 h, 500°C) GF/F filters and filled into combusted (8 h, 500°C) glass ampoules. Samples were acidified with 80 μL of 85% phosphoric acid, heat sealed immediately, and stored at 4°C in the dark until analysis. DOC samples were analyzed by high-temperature catalytic oxidation (TOC-VCSH, Shimadzu) modified from Sugimura and Suzuki (1988) and as described in more detail in Engel and Galgani (2016).
Total Dissolved Nitrogen (TDN)
TDN was determined with the TNM-1 detector on the Shimadzu analyzer. Here, nitrogen is combusted and converted to NOx, which chemiluminesces when mixed with ozone and detected using a photomultiplier (Dickson et al., 2007). Calibration was done every 8–10 days with standard solutions of potassium nitrate Suprapur® (Merck 105065) yielding 0, 100, 250, 500, and 800 μg N L−1. On every measurement day deep seawater reference (DSR) material (Consensus Reference Materials Project of RSMAS, University of Miami) was used, yielding values within the certified range of 31–33 μmol N L−1.
Chlorophyll a (Chl a)
Chl a was determined in duplicate from bulk seawater (200–400 mL), filtered onto GF/F filters (25 mm). Filters were placed in the dark immediately after filtrations and stored at−20°C until analysis. Chl a was determined after extraction with 10 mL of acetone (90%) on a Turner fluorimeter after Welschmeyer (1994). Spinach extract standard (Sigma Aldrich) was used for calibration.
Phyto- and Bacterioplankton/ Neuston
Autotrophic cells <20 μm, and heterotrophic bacteria were counted by flow-cytometry on a FACSCalibur (Becton Dickinson) using BD CellQuest Pro-Software as described in more detail by Engel and Galgani (2016). Duplicate 4 mL samples were fixed with 200 μL glutaraldehyde (5% final concentration). Samples for autotrophic cells were stored at −80°C those for bacteria at −20°C until enumeration. Prior to counting, bacteria were stained with SYBR Green I (Molecular Probes). Samples from the SML were collected on days 1, 2, 3, 4, 5, 9, 11, 12, 15, 22, 23, and 24.
Total and Dissolved Hydrolysable Amino Acids
Total hydrolysable amino acids (THAA) and dissolved hydrolysable amino acids (DHAA) were determined from bulk water and on 7 sampling days from the SML using a 1260 HPLC system (Agilent), following the methods described by Lindroth and Mopper (1979) and Dittmar et al. (2009), with modifications as described in Engel and Galgani (2016). Duplicate samples (5 ml) were filled into pre-combusted glass vials (8 h, 500°C) and stored at −20°C until analysis. For DHAA, samples were first filtered through 0.45 μm Millipore Acrodisc® syringe filters. Samples from the SML were collected on days 2, 4, 9, 11, 15, 22, and 24.
A measure for the diagenetic state of OM is the amino acid-based degradation index (DI) (Dauwe and Middelburg, 1998; Dauwe et al., 1999). For the calculation of DI from THAA in this study, mole percentages of amino acid were standardized using averages, and standard deviations and multiplied with factor coefficients as given in Dauwe et al. (1999) based on Principal Component Analysis. DI values often range between +2 and −2, with lower values indicating more degraded, higher values more fresh organic material.
Total and Dissolved Combined Carbohydrates
Total and dissolved hydrolysable carbohydrates >1 kDa (THCHO and DHCHO) were determined from duplicate bulk seawater and on 7 sampling days from SML samples applying high performance anion exchange chromatography coupled with pulsed amperometric detection (HPAEC-PAD) using a Dionex ICS 3000, following the method by Engel and Händel (2011) with modifications as described in Engel and Galgani (2016). Samples of 20 mL were filled into pre-combusted glass vials (8 h, 500°C) and kept frozen at −20°C until analysis. Samples from the SML were collected on days 2, 4, 9, 11, 15, 22, and 24.
Surfactants
Duplicate SML samples of about 50~mL were collected on 8 days, transferred into polypropylene bottles and immediately frozen at −40°C for transport and stored at −79°C prior to analysis by vibrational sum-frequency generation (VSFG) spectroscopy using a commercial picosecond VSFG spectrometer (EKSPLA, 532 nm up-conversion) as described elsewhere (Laß and Friedrichs, 2011). The use of VSFG spectroscopy for environmental surfactant analysis has been introduced by Laß et al. (2010) and the interpretation of the measured spectra in terms of surfactant abundance has been outlined and discussed in some detail in Laß and Friedrichs (2011) and Laß et al. (2013). Non-linear VSFG provides surface-specific infrared spectra of the molecular nanolayer, i.e., the signal arises from the molecules residing directly at the interface. The VSFG signal intensity, , reflects the average molecular hyperpolarizability β of N probed surface oscillators. In general, due to the additional orientation sensitivity of the VSFG signal, β is a parameter that is also dependent on N itself. However, as β has been found in our previous study to be approximately constant for marine water samples (Laß and Friedrichs, 2011), IVSFG can be directly taken as a qualitative measure of surfactant surface concentration cSurfactants, with . As an operational definition of surfactant abundance or coverage it is useful to report the measured intensities relative to the intensity obtained for a well reproducible reference. Here, a densely packed monolayer of the phospholipid DPPC (dipalmitoyl-sn-glycero-3-phosphocholine) at a surface concentration corresponding to 20 Å2 per lipid chain has been used. Surfactant abundance is reported by means of the square root of the ratio of the integral VSFG intensities over the spectral range from 2,750 to 3,000 cm−1. This spectral range corresponds to C-H vibrations mainly related to the CH2 and CH3 groups of lipid alkyl chains. To this ends, the plotted surfactant value can be understood as a measure of the surfactant coverage, e.g., a value of 0.25 simply implies a 25% coverage. However, facing the rough assumptions and approximations made for the data reduction, one should be aware of the still qualitative nature of this measure. Also note that the absolute surfactant signal is dependent on the wet-to-dry surfactant ratio in the sample, with wet surfactants reported to dominate the surfactant pool (Baier, 1972; Laß and Friedrichs, 2011; Cunliffe et al., 2013; Laß et al., 2013). Dry surfactants (such as simple fatty acids, phospholipids, etc.) accumulate at the water-air interface and hence their signal contribution depend on the ratio of the sampled water surface and the used surface area of the spectrometer dish (about 0.12 m2 vs. 37 cm2) as well as on their transfer efficiency which is limited by wall adsorption losses. In contrast, more water-soluble wet surfactants re-establish surface-bulk equilibrium resulting in signal contributions largely reflecting their SML concentration and that are less affected by the sampling procedure. Total measured VSFG intensities are equal to the sum of both contributions from dry and wet surfactants. Samples from the SML were collected on days 2, 4, 5, 9, 11, 15, 22, and 24.
Data Analysis
An operational estimate for the apparent thickness (d) of the SML sampled with the glass plate is given by:
Here, V is the volume collected, i.e., 200–430 mL, A is the sampling area of the glass plate, i.e., 2,000 cm2, and n is the number of dips.
Enrichment or depletion of a substance in the SML was estimated by calculating the enrichment factor (EF):
with x being the concentration of a given parameter in the SML or ULW, respectively (GESAMP, 1995).
Statistical Analysis
Average values are given by the statistical mean and its standard deviation (SD). Averaged values were compared by means of a Mann-Whitney Rank Sum Test. Significance of the Pearson's correlation coefficient (r) was tested by a t-test (two-tailed) with degree of freedom, df = n-2. Significance level was p < 0.05.
Principle component analysis (PCA) was performed to explore differences in individual amino acid and carbohydrate composition between different fractions (dissolved and particulate) of the SML and the bulk water. Data for the relative contribution (%) of individual amino acid and carbohydrate concentrations to total amino acids and carbohydrates concentrations (nmol L−1) was used. The package CRAN:factoMineR (Lê et al., 2008) in the open source software R (version 3.0.2, R Core Team, 2013) was used for the PCA analysis using a correlation matrix.
Results
Microbial Growth and Biomass Build-Up in the Water Column
In general, macronutrients, i.e., NO3, NO2, SiOH4, and PO4 were detectable in the bulk seawater during the whole experiment. Nutrient concentrations were 0.28 μmol L−1 PO4, and 7.01 μmol L−1 NO3, initially and decreased continuously until addition of nutrients on the evening of day 12 (Figure 2). Nitrite was 0.32 μmol L−1 initially, increased slightly during the first 2 days and declined until day 12 as well. After nutrient addition, following a lag phase of about 5 days, NO3, NO2, and PO4 decreased again until the end of the experiment. Silicate was 3.88 SiOH4 μmol L−1 initially and was raised to about 10 μmol L−1 on day 12. A decline in silicate concentration was not observed, neither before nor after nutrient addition, and in compliance with the absence of silicifying organisms throughout the study.
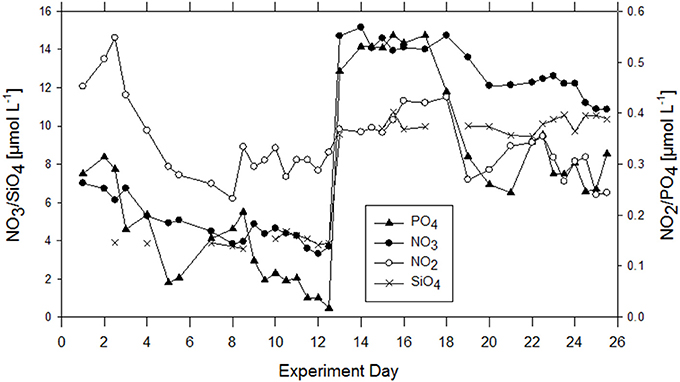
Figure 2. Development of inorganic nutrients over the course of the experiment in the bulk seawater of the wind wave channel. On the evening of day 12, 10 μM of NO3 and PO4 as well as 0.6 μM of PO4 were added.
Autotrophic biomass was low throughout the experiment. Chlorophyll a (Chl a) concentrations were negligible until day 20/ 21, when an E. huxleyi culture and a microlayer from an earlier phytoplankton bloom experiment were added (Figure 3). Chl a increased more strongly after day 23 to yield highest values of 0.042 μg L−1 on day 25. Autotrophic cell abundance in the bulk water was 18 mL−1 before and 220 mL−1 directly after addition of the E. huxleyi culture (data not shown).
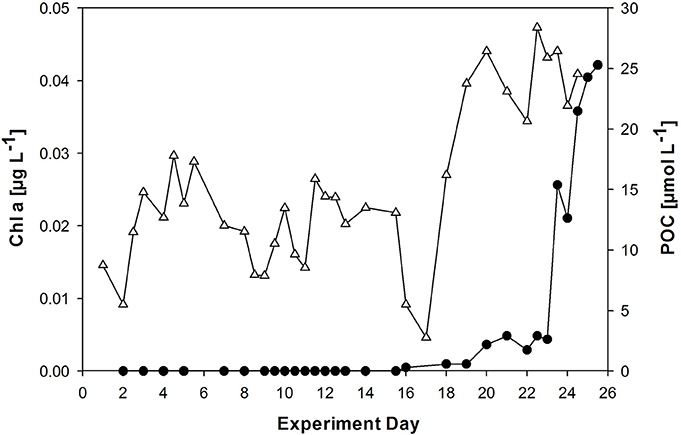
Figure 3. Development of chlorophyll a (Chl a, filled circles) and POC (triangles) concentration in the bulk seawater of the wind wave channel. A culture of the phytoplankton species Emiliania huxleyi was added on day 20, followed by sea surface microlayer (SML) sample from a previous phytoplankton bloom experiment on day 21.
Particulate organic carbon (POC) concentration varied between 4 and 29 μmol L−1 in the course of the experiments (Figure 3). A large fraction of the POC may be attributed to high gel particles abundance, e.g., TEP) and Coomassie stainable particles (CSP) (Sun et al., 2017). A strong increase in POC was observed after day 17, coinciding with decline in phosphate and subsequently DIN concentration (Figure 2). PON concentration followed the development of POC concentration in general (Figure S1). [POC]:[PN] ratios were enriched in nitrogen compared to the Redfield ratio of C:N of 6.6 and yielded on average 4.7 ± 1.2 (n = 33). However, a strong decline in PN concentration was observed at day 15, leading to a temporary rise in [POC]:[PN] at that day (Figure S1).
Organic Matter Accumulation in the SML
A thin SML of 28.3 μm was observed at the beginning of the experiment (day 0) (Table 1). Thereafter, the SML thickness increased gradually in the course of the study to reach highest values of 50 μm on day 23. On 8 days of the Aeolotron study wind experiment were conducted with wind speed increasing stepwise from about 1 to 18 m s−1, and each wind speed lasting for 1–3 h. Following the highest wind speed, bubbling of seawater was induced by aeration to simulate wave breaking. Both wind and bubbling treatments had no long-lasting effects on the thickness of the SML, as similar thicknesses were observed at low wind speed in the mornings before and after increasing the wind velocities, e.g., days 4 and 5 or within 1 week of wind speed changes (days 11–22).
Heterotrophic bacterial cell abundance in the water column started with 1.4 × 105 mL−1, and increased to a first peak of abundance on day 4 with values of 9 × 105 mL−1 (Figure 4A). Between days 17 and 22 a bacterial bloom developed, with the highest abundance of 19 × 105 cells mL−1 determined on day 20. This bacterial bloom coincided with the observed increase in particulate OM (Figure 3) and can partly explain the low C:N ratios in particles, which are typical for heterotrophic marine bacteria. After day 20, a decline in bacterial abundances was observed, following the addition of the E. huxleyi culture.
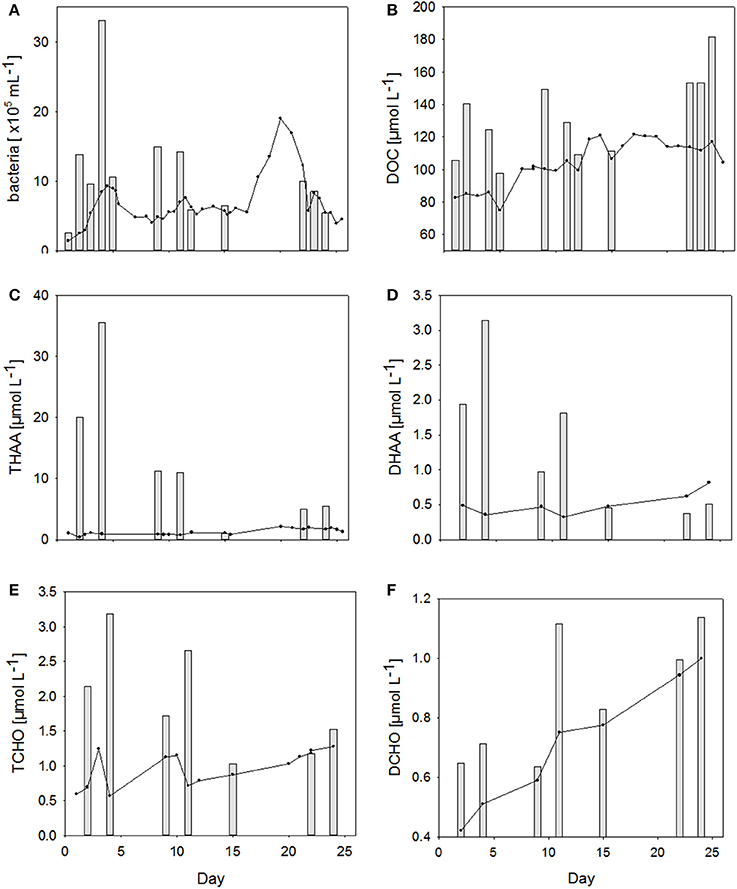
Figure 4. (A–F) Changes in concentrations of different organic matter components in the SML (bars) and in the bulk water (lines) during the Aeolotron experiment.
Bacteria were strongly enriched in the SML during the first 11 days of the experiment with EF's ranging from 1.1 to 5.6. No bacterial enrichment was observed in the SML after nutrient addition on day 12. Abundance of bacteria in the SML was not significantly related to those of the water column.
Dissolved organic carbon (DOC) concentration in the water column started with 85 μmol L−1 and increased steadily over the course of the experiment to reach maximum value of 120 μmol L−1 on the last days of the study (Figure 4B). DOC was enriched in the SML during all sampling days, except for day 15, when the difference between DOC concentration in the SML and underlying water fell within the range of the analytical error. EFDOC ranged between 1.0 and 1.6. DOC concentration in the SML was significantly related to DOC concentration in the water column (r = 0.73, n = 12, p < 0.05) as well as to SML thickness (r = 0.58, n = 12, p < 0.05), indicating that DOM production in the water column directly affected SML properties.
DON concentrations started with 6.8 μmol L−1 initially and increased slowly to a maximum value of 11 at day 20 (data not shown). [DOC]:[DON] ratios showed a clear carbon enrichment with an average of 12.2 ±2.2 (n = 12). Highest [DOC]:[DON] of 16 was observed at day 14, declining thereafter and parallel to inorganic nitrogen draw-down to reach a value of 9.3 at the end of the study.
THAA, including particulate and dissolved hydrolysable amino acids, in the water column varied on the days directly after filling the Aeolotron, but stabilized thereafter around 1 μmol L−1 until day 16. On day 20, highest concentration of 2.31 μmol L−1 was reached for the water column (Figure 4C). Thereafter, THAA declined slowly until the end of the study. THAA in the SML exceeded concentrations in the underlying water by one order of magnitude during the first 12 days of the study, resulting in high enrichment factors (EFs) of 13–48. Enrichment of THAA in the SML was absent on day 15 and again slightly higher after the addition of an organic microlayer (day 21), yielding EFs of ~3 on days 22 and 24. THAA concentrations were tightly related to bacterial abundance in both water column (r = 0.60, n = 20, p < 0.005) and SML (r = 0.94, n = 7, p < 0.005), indicating that heterotrophic bacteria were mainly responsible for amino acid dynamics during this experiment.
Similar to THAA, a strong enrichment in the SML was also observed for dissolved hydrolysable amino acids (DHAA) until day 15 (Figure 4D), yielding EFs between 2.2. and 8.8. However, DHAA concentrations were about one order of magnitude lower than THAA and no enrichment in the SML was observed on days 22 and 24. Thus, DHAA could not explain DOC enrichment in the SML observed at the end of the experiment. In general, DHAA comprised between 1 and 10% of DOC in the SML with the higher values observed during the first 2 weeks of the study. A close direct relationship between DHAA enrichment and the relative DOC fraction of DHAA was observed for the SML, yielding Δ[EFDHAA]:Δ[DHAA-C:DOC] = 0.85 ± 0.06 (r = 0.98, n = 7, p < 0.001; Figure 5). Neither THAA nor DHAA in the SML were significantly related to the respective concentrations in the water column.
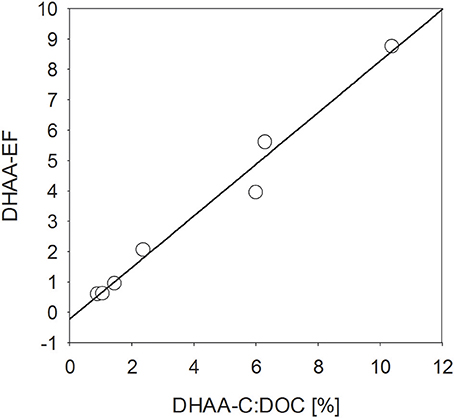
Figure 5. Direct relationship (p < 0.001, n = 7) between the enrichment of dissolved hydrolysable amino acids (DHAA) in the SML and the DHAA fraction of DOC.
Total combined carbohydrates (>1 kDa, TCHO) concentrations in the water column varied during the first 12 days of the study (Figure 4E). Overall concentrations of TCHO were lower than of THAA and increased from an initial value of 0.6–1.2 μmol L−1 on days 9 and 10 and again on day 22. Similar to THAA, a strong enrichment of TCHO in the SML was observed during the first 12 days of the study, albeit EF's for TCHO of 1.5–5.6 were clearly lower than those for THAA. On days 15 and 22, TCHO concentrations in the SML were similar to bulk water. A slight enrichment of TCHO in the SML was again observed on day 24. EF's for TCHO varied between 1 and 5.6 with the latter being observed on day 4. Like for amino acids, TCHO concentration in the SML was significantly related to bacterial abundance (r = 0.85, n = 7, p < 0.05) albeit less strongly. No relationship between SML and bulk water concentrations of TCHO was observed.
DCHO concentration started with 0.4 μmol L−1 and increased continuously in the course of the experiment to reach a value of 1.0 μmol L−1 on day 24 (Figure 4F). EFs of DCHO varied between 1.1 and 1.5, with the highest enrichment observed on day 11. In contrast to TCHO, DCHO concentration in the SML closely corresponded to those of the underlying water (r = 0.84, n = 7, p < 0.05). In general, DCHO contributed between 2.4 and 5.1% to DOC, with slightly higher contributions during the second half of the experiment.
Molecular Composition of the SML
Degradation indices (DI) which were calculated from THAA data were significantly higher for the SML compared to the bulk water (Mann-Whitney Rank Sum Test, t = 20, n = 6, p < 0.01), except for day 15, when no difference between SML and bulk water was visible (Figure 6). This suggests that OM accumulating in the SML was relatively fresh and likely more labile than bulk OM in the water column.
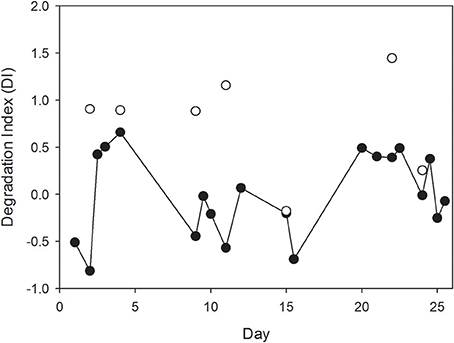
Figure 6. Changes in the degradation index (DI) of organic matter in the SML and in bulk water in the course of the experiment were estimated from THAA composition following Dauwe et al. (1999). Note that no difference in composition was determined for day 15 suggesting complete absence of SML organic enrichment.
Charged, zwitterionic amino acids represent polar blocks of amphiphilic moieties and are known components of biogenic surfactants (Infante et al., 2004). To investigate the role of amino acid charges on SML enrichment, we grouped THAA into different charge classes (Figure 7). The strongly polar, basic amino acid Arg was highly enriched in the SML (Figure 7A), followed by aromatic and acidic amino acids (Figures 7B,C). In general, neutral amino acids, which represented the largest fraction of THAA, were underrepresented in the SML, except for two samplings at the end of the study, after addition of autotrophic cells (Figure 7D). Hydroxylic amino acids were always underrepresented in THAA of SML with a remarkably steady fraction of THAA of 13.6–15.2% Mol (Figure 7E). In general composition of THAA was less variable in the SML than in the bulk water, where strong compositional changes were observed in particular during the first 15 days of the study. In general, THAA enrichment in the SML was significantly related to the relative abundance of the basic amino acid Arg (p < 0.005; Figure 8), following an exponential function. Highest THAA enrichment during this study was obtained for THAA containing about 6.5% Mol Arg.
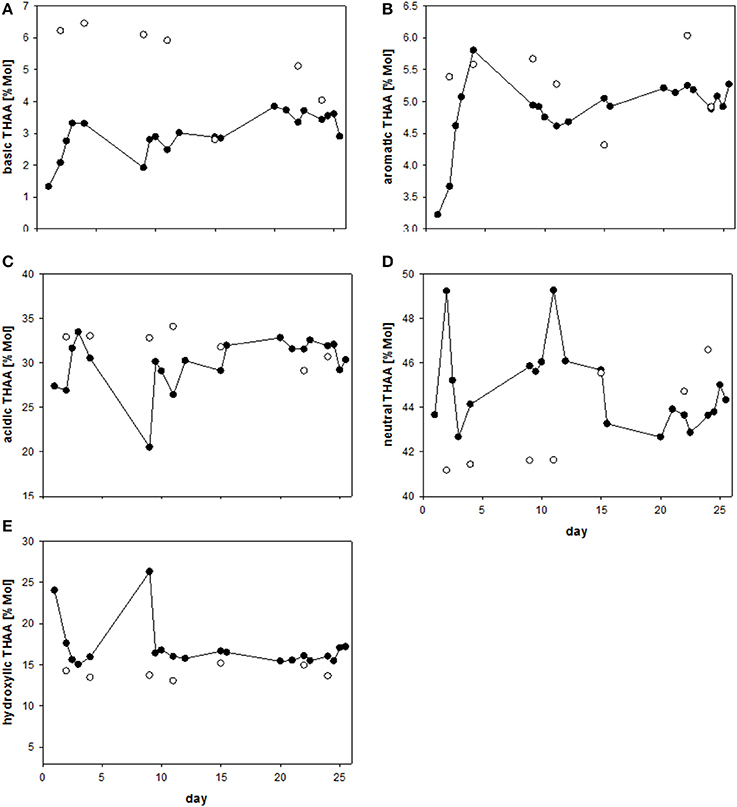
Figure 7. (A–E) Relative changes (%Mol) in different groups of THAA in the SML (solid symbols) and bulk water (open symbols) during the Aeolotron experiment.
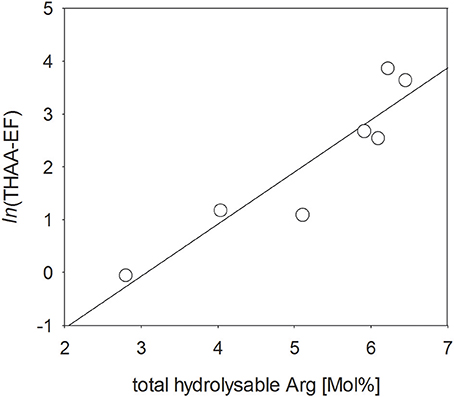
Figure 8. Direct relationship between the enrichment of THAA in the SML and the molar percentage of total arginine (Arg) (p < 0.005).
PCA analysis of individual amino acids revealed distinct differences between DHAA and PHAA, (= THAA-DHAA), and also between the SML and bulk water (Figure 9A). Overall, the first two components explained 61.1% (43.5% + 17.6%) of the variance within the samples. DHAA in the SML showed higher relative contribution of the acidic amino acid GlX, while the DHAA in the bulk water showed higher contribution of Tyr, Ile, Thr, Phe, and Ser. Bulk water PHAA had increased contributions of Gly, Asx, Leu, and Ala. PHAA in the SML clustered around the intersection of the PC1 and PC2 axes, which suggests that the amino acid composition in this fraction was an intermediate from the other three fractions. The enrichment of Arg, as seen in THAA, of the SML was mainly driven by DHAA. Arg was clearly enriched in DHAA of the SML, while depleted in the ULW (Figure 9A).
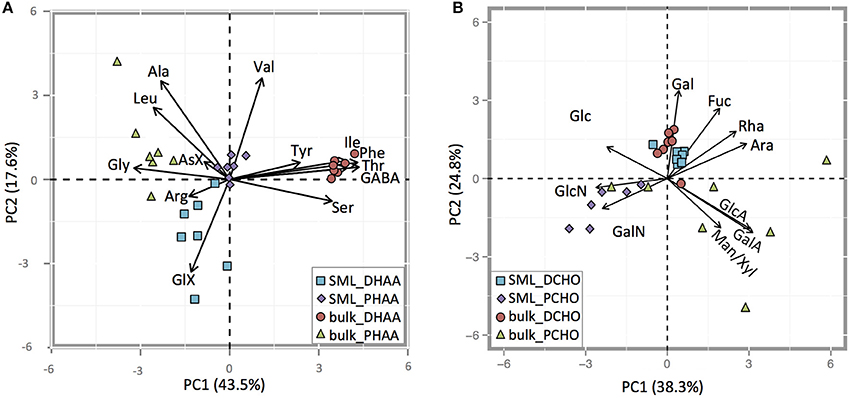
Figure 9. (A,B) PCA biplot of relative contribution of individual AA to dissolved (DHAAS) and to particulate (PHAAS) (A) and of individual CHO to dissolved (DCHO) and to particulate (PCHO) (B) in the SML and the bulk water.
PCA analysis was also conducted for individual CHO in DCHO and in PCHO (= TCHO-DCHO), and also pointed to compositional differences between SML and bulk water (Figure 9B). The first two components explained 63.1% (38.3% + 24.8%) of the variance between the samples. DCHO in the SML and bulk fractions were very similar, and showed increased contributions of Fuc and Gal compared to PCHO, as well as low variations between sampling days. Fuc was slightly more enriched in DCHO samples from the in SML, while Gal was slightly more enriched in the DCHO bulk samples. PCHO samples in the SML were enriched in amino-sugars GlcN and GalN compared to DCHO, while PCHO in bulk water samples had very variable compositions. However, during sampling days 2 and 9 (before the nutrient addition) bulk PCHO samples were also enriched in both amino sugars (GlcN and GalN) and clustered with PCHO from SML samples. On sampling days after nutrient addition, the PCHO bulk samples showed enrichment in Man/Xyl and in the acidic sugars GlcA and GalA. Mur-Ac was below detection in both SML and bulk water throughout the study.
Surfactants
Spectral characteristics of the Aelotron VSFG data are compared with the reference spectrum of a dense monolayer of the phospholipid DPPC in Figure 10A. The shaded areas indicate the range of C-H vibrational signal used to determine the relative CH integral strength and with it the surfactant coverage. The two prominent peaks in the DPPC spectrum are characteristic for the terminal -CH3 group of mutually parallel aligned alkyl chains of well-ordered lipid chains in the monolayer. In contrast, the Aeolotron spectrum reveals broader spectral signatures and an additional pronounced peak around 2,850 cm−1 (typically assigned to -CH2- vibrations) indicating the presence of a more complex surfactant mixture. The spectral range from 3,000 to 3,800 cm−1 largely reflects the contributions from O-H vibrations including that of interfacial water. The two-peak spectral feature with the unusual intense peak at about 3,440 cm−1, though typical for SML samples, has been tentatively assigned by Laß and Friedrichs (2011) to the presence of lipopolysaccharides or other lipid-like compounds embedded in colloidal matrices. However, facing the high SML enrichment factors of amino acids as part of the biogenic surfactant pool observed in this study, it cannot be ruled out that peptide-rich components contribute to the observed VSFG signature as well.
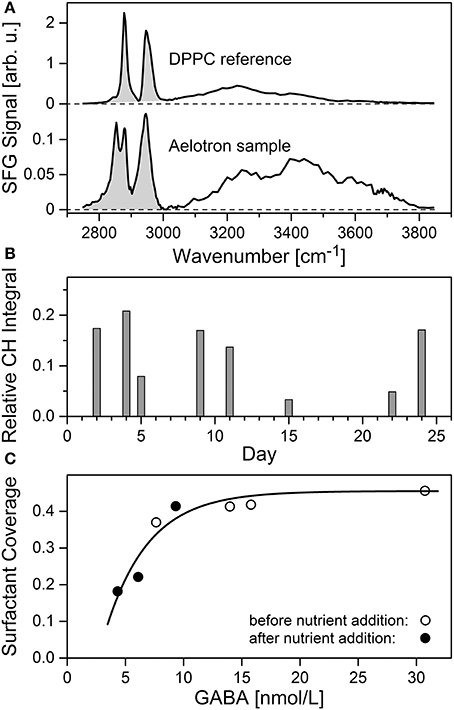
Figure 10. (A–C) Accumulation of surfactants in the SML. (A) Averaged VSFG and DPPC reference spectra with integrated CH spectral range (shaded area), (B) relative CH integral trend (C) correlation of surfactant coverage with γ-aminobutric acid (GABA) during the periods days 2–12 (open circles) and days 13–24 (filled circles) of the experiment. The curve indicates a regression model reflecting the possible signal trend resulting from a saturation of the surface with surfactants.
The measured relative CH integral strength during the course of the experiment is plotted in Figure 10B. During the first 11 days, relatively high values of up to 0.21 (corresponding to a 45% surface coverage) have been observed, hence indicating the presence of a dense surfactant layer. In fact, keeping in mind the presumably less well ordered structure of the natural surfactants compared to the artificial DPPC reference monolayer, these high values may even indicate an interface that is more or less fully covered with surfactants. Nevertheless, the addition of nutrients on day 12 first caused a significant reduction of the relative integral strength to about 3% on day 15, but its high value recovered toward the end of the experiment. A close correlation was observed between the concentrations of surfactants and total γ-aminobutric acid (GABA) (Figure 10C) in the SML. GABA, a non-proteinogenic amino acid and derived from glutamate, has often been used as an indicator for microbial decomposition of OM (Dauwe et al., 1999). Thereby the relationship between GABA and surfactants seems to differ between the periods before (days 2–12) and after (days 13–24) addition of nutrients. During the period before nutrient addition, a decline in GABA concentration was associated with only a slight decline in surfactant concentration (Figure 10C, open circles), whereas during the second half of the experiment, starting from low levels, the surfactant pool in the SML accumulated even stronger than the concentration of GABA (filled circles). The latter dependence may suggest that processes other than microbial degradation of OM fueled the surfactant pool as well. However, as indicated by the curve in Figure 10C, instead of being indicative for different biogeochemical regimes, a saturation of the interface with surface active compounds may serve as a plausible alternative explanation of the observed trend as well. A robust correlation between the surfactant signal and GABA concentration (r = 0.95, n = 7, p < 10−5) indicates a close non-linear relation of surfactant abundance and microbial degradation processes of OM. For a more detailed statistical analysis of the VSFG data we refer to Table S1 in the Supplementary Material.
Actually, the observed high surfactant levels may at least be partly due to a high enrichment of dry surfactants resulting from the sampling procedure. Compared to previous VSFG work (Laß and Friedrichs, 2011), higher ratios of sampling surface to sample dish area have been used in this study, possibly favoring the VSFG detection of dry surfactant fractions. Clearly, more experimental data and repeated future experiments are desirable to verify the observed trend between surfactant signal and GABA concentration.
Discussion
The advantage of the Aeolotron facility is that sampling for SML properties can be conducted repeatedly under controlled physical conditions. Here, data were collected under the same temperature and very similar low wind conditions over the duration of 26 days. Therewith, wind speed or convective mixing as explanation for variations in OM enrichment in the SML can largely be excluded. The advantage of a controlled laboratory system such as the Aeolotron, however, comes with the drawback of absence of biotic and abiotic dynamics and of complexity that are typical for a natural ecosystem such as the surface ocean. The Aeolotron study was not designed to mimic a natural oceanic ecosystem, but to investigate processes at the air-sea interface of a natural seawater body under controlled physics. Due to technical reasons two dark phases, i.e., days 1–8 and 17–19 had to be included and were likely responsible for the failure of a phytoplankton or cyanobacterial bloom, despite addition of nutrients and E. huxleyi seed culture. Thus, the dominant bacterial bloom that developed during this study may not be representative for a surface ocean situation, although bacterial abundance did not exceed typical oceanic values (Stolle et al., 2009; Engel and Galgani, 2015; Zäncker et al., 2017). In particular, phytoplankton blooms can release large amounts of carbohydrates that accumulate in in SML (Jayarathne et al., 2016), a scenario not included in our study. Moreover, manipulations such as the continuous increase in wind speed at several days and the addition of a pure phytoplankton culture and of SML have to be seen in an experimental context rather than an attempt to simulate natural conditions. Nevertheless, several findings gained during this study were in accordance with previous observations from the ocean, indicating in particular that the accumulation of specific OM components in the SML, as well as the relationships between individual constituents can be transferred to the natural system, and may help to give insight into the dynamics of an specific environment, the SML, that is not easily accessible in the open sea.
Identifying ecological drivers of OM accumulation at the air –sea interface is an important task, since organic compounds in the SML are thought to influence a variety of air-sea exchange processes potentially relevant for regional and global climate, marine biogeochemical cycling and ocean physics (Cunliffe et al., 2013; Engel et al., 2017). Our experiment included different ecological phases according to the biology and chemistry of the bulk seawater. Until day 20, biological processes in the tank were clearly dominated by heterotrophic bacteria, as autotrophic biomass was virtually absent. On day 20 a culture of Emiliania huxleyi was added, followed 1 day later by a natural microlayer sample from a previous, phytoplankton dominated, experiment. After this addition a slight increase in Chl a concentration and in SML-thickness were observed. Hence, apart from the last 5 days of the study, changes in OM quantity and quality can clearly be attributed to heterotrophic activity. Thereby, amino acids apparently served as a preferred bacterial substrate since THAA as well as DHAA concentrations in the SML decreased throughout the study. The decline of TCHO in the SML, in contrast, was accompanied by an accumulation of DCHO suggesting solubilization of particulate CHO and delayed uptake of DCHO. Stronger accumulation over time of DCHO compared to DHAA has been observed before, and indicates that amino acids as source of both carbon and nitrogen are a preferred substrate for marine bacteria (Grossart et al., 2007; Engel et al., 2011).
Causes of SML Formation
During this study, the apparent SML thickness ranged between 20 and 50 μm, which fits well to previous observations for the SML when sampled with a glass plate at similar withdrawal rates (Zhang, 2003; Cunliffe et al., 2011; Engel and Galgani, 2016). Using direct pH microelectrode measurements, Zhang (2003) showed that physico-chemical properties at the sea surface changed beyond an in situ thickness of 60 μm corroborating the proposed apparent sampling thickness of 50 ± 10 μm (Zhang et al., 1998). We therefore assume that samples obtained with the glass plate during this study well represented the SML.
SML thickness, in this study, was significantly related to DOC concentration in bulk water, suggesting that the large, bulk reservoir of organic components in the water column is an important source of SML components. Thereby, the thickness of the SML increased with DOC concentration in the ULW, but was not related to DOC enrichment in the SML. This suggests that absolute DOC concentration in the water column, rather than relative differences between the SML and the underlying water are more important for determining SML thickness. The importance of seawater DOC as major source for OM exchange across the air-sea interface has been suggested recently based on organic compound analysis of sea spray, which showed no correlation to autotrophic production but mirrored the more uniform composition of surface water DOM (Quinn et al., 2014).
During the first days of our study, i.e., days 2–11, the SML was characterized by a strong enrichment of bacteria and semi-labile OM, in particular amino acids, coinciding with highest surfactant concentration, while little enrichment of these components was observed during the second part of the experiment, when autotrophic biomass increased. This clearly shows that accumulation of surface active OM in the SML can be derived from the bulk pool of seawater OM and can occur independently of recent autotrophic production.
The thickness of the SML sampled at low wind speed was apparently not influenced by intermittent changes in wind speed, which were applied in between samplings. This implies that the disruption of SML and mixing of organic components between SML and bulk water at high wind speeds have no long-lasting effect and that SML and surface films recover rapidly after disturbance as suggested earlier (Dragcevic and Pravdic, 1981; Laß et al., 2010).
Interestingly, enrichment of amino acids and carbohydrates, as well as surfactant concentration in the SML, did not increase with SML thickness or DOC concentration. On the contrary, highest abundance of surfactants and amino acids were observed when SML thickness and bulk DOC concentration was lowest. Thereby, changes in SML thickness were too small to explain the observed OM enrichment by means of simple dilution. Reduction of surfactant and DOC concentration in the SML with increasing bulk DOC concentration and in systems with high autotrophic productivity and consequently likely higher DOC production has been observed previously (Carlson, 1983; van Pinxteren et al., 2017). Carlson (1983) suggested a chemical process of enhanced solubility of surfactants interacting with subsurface DOC to explain a diminished accumulation of surface active components.
Chemical Enrichment in the SML
During this study, the SML enrichment of amino acids in particular was highest at low DOC concentrations. This observation is in accordance with findings by Galgani et al. (2016) who determined higher amino acids enrichment in Arctic sea ice melt pools at relatively low subsurface DOC concentration compared to adjacent open ocean waters. Since a higher percentage of amino acids in the carbon pool points to fresh and more labile OM (Davis et al., 2009), it can be assumed that it is the less degraded OM that accumulates in the SML. In this study, DHAA-C was >2 Mol% during the first phase of the study, indicating labile OM accumulation at the SML. This assumption is also supported by changes in amino acid composition that yielded a higher degradation index for OM in the SML compared to the bulk water throughout this study.
Amino acids in general showed highest enrichment, up to EF of 48, of all organic components analyzed in this study. This result fits well to field observations showing a specifically high enrichment of amino acids for many coastal as well as open ocean sites (Henrichs and Williams, 1985; Carlucci et al., 1992; Kuznetsova and Lee, 2001; Kuznetsova et al., 2004; Reinthaler et al., 2008; Engel and Galgani, 2016). Kuznetsova et al. (2004) suggested that individual amino acids had higher mole percentages in the SML than in the corresponding subsurface water, which implies selective peptide enrichment in the SML. During this study the basic amino acid arginine was identified as peptide component strongly correlating to total amino acid enrichment in the SML. This suggests that arginine is part of the biogenic polymeric surfactant pool that preferentially absorbs to the air-sea interface.
Interesting in this respect is that bacterial oxidation of arginine can result in nitric oxide (NO) production and also lead to the formation of nitrous oxide (N2O) (Schreiber et al., 2012), one of the most powerful greenhouse gases. So far, bacterioneuston has been suggested to be actively involved in the destruction of N2O in the SML (Conrad and Seiler, 1988). Recurring enrichment of arginine in the SML, however, may give rise to N2O enhanced production, hence it may well be speculated that this process constitutes a hitherto unexploited source of N2O release from the ocean to the atmosphere.
Microbial Control of SML Composition
Loss of chemically labile OM from the SML was observed within a few days during the first part of the experiment, pointing to rapid microbial degradation. The observed close relationship between bacterial abundance, amino acid concentration and surfactants strongly suggest a bacterial control of physicochemical properties of the SML. This finding is in line with the outcome of a previous VSFG field study performed at the Boknis Eck Time Series Station (Western Baltic Sea), where surfactant signals were not directly related to phytoplankton abundance alone (Laß et al., 2013). Instead highest surfactant levels were found in summer months where bacterial abundance (and the associated grazing by protozoans) is enhanced at this site (Hoppe et al., 2013).
Taxa identified for the bacterial community in the SML during this study such as Pseudoalteromonas sp. and Alteromonas sp. as well as taxa inhabiting both SML and bulk water, dominated by Phaeodactylibacter xiamenensis, are typical for natural marine environments (Rahlff et al., 2017). Alteromonas sp. has been shown to efficiently utilize the labile pool of coastal seawater DOM in experimental incubations within a period of days (Pedler et al., 2014). Hence, microbial dynamics as observed during this wind wave channel experiment may be transferrable to the field situation under similar environmental conditions, in particular low wind speed and low light.
It is still unclear what controls bacteria and their activities in the SML. Bacteria in the field bacteria may be enriched (Stolle et al., 2009) or depleted in the SML (Engel and Galgani, 2016; Galgani et al., 2016), or their growth and hence consumption rates of amino acids are reduced (Stolle et al., 2009; Santos et al., 2012). Possible controls of bacterioneuston activity include the prevailing meteorological conditions, UV light (Agogué et al., 2005), incident OM availability (Carlucci et al., 1985, 1992), wind speed (Stolle et al., 2011; Rahlff et al., 2017) and aerosol deposition (Astrahan et al., 2016). While the importance of solar and UV irradiance for controlling neuston activity in the field is debated (Bailey et al., 1983; Carlucci et al., 1985; Santos et al., 2012), the high bacterial enrichment in the SML during the first days of this study suggest, that accumulation of labile OM and at the absence of light potentially favors bacterial growth at the air sea interface. In the ocean, solar irradiation can reduce the bioavailability of fresh DOM or enhance it when refractory DOM undergoes photochemical modifications (Obernosterer et al., 1999; Galgani and Engel, 2016). As a consequence, strong diel variations in bacterial activity have been suggested for the surface lit upper water column (Herndl et al., 2008) and may be even more pronounced within the SML. As a consequence, irradiance dependent (e.g., day-night) variations can be expected for microbial cycling of climate relevant trace gases such as methane (Upstill-Goddard et al., 2003), nitrous oxide, carbon monoxide and hydrogen (Conrad and Seiler, 1988) or carbon dioxide (Calleja et al., 2005).
Overall, our study highlights the close connection between the appearance of heterotrophic bacteria and organic compounds that potentially act as surfactants in the SML. We did not resolve to which extend the bacterial community was a source or a sink of these substances. Experiments tracing the uptake or release of biogenic surfactants, in particular arginine-rich polypeptides would be needed to further unravel linkages between ecology and physico-chemistry of the air-sea interface. This knowledge could help to better constrain air-sea exchange processes and feed-backs in times of environmental change.
Author Contributions
AE designed the study, analyzed the data and wrote the manuscript. MS and CS conducted the study, GF provided and analyzed data, JG analyzed data. All authors contributed to writing of the manuscript.
Conflict of Interest Statement
The authors declare that the research was conducted in the absence of any commercial or financial relationships that could be construed as a potential conflict of interest.
Acknowledgments
We thank Bernd Jähne and Kerstin Krall for hospitality and organization during the Aeolotron study. Many thanks go to the captain and crew of R/V POSEIDON as well as to Armin Form for seawater collection. Thanks to Kristian Laß for making available the VSFG data through the project CP1212 of the Kiel cluster of excellence The Future Ocean. Jon Roa, Ruth Flerus, Katja Laß, and Tania Klüver are greatly acknowledged for technical support. This work was supported by the BMBF project SOPRAN III (Surface Ocean Processes in the Anthropocene 03F0662A-TP2.2). The study is a contribution to the international Surface Ocean Lower Atmosphere Study (SOLAS).
Supplementary Material
The Supplementary Material for this article can be found online at: https://www.frontiersin.org/articles/10.3389/fmars.2018.00182/full#supplementary-material
References
Agogué, H., Joux, F., Obernosterer, I., and Lebaron, P. (2005). Resistance of marine bacterioneuston to solar radiation. Appl. Environ. Microbiol. 71, 5282–5289. doi: 10.1128/AEM.71.9.5282-5289.2005
Astrahan, P., Herut, B., Payton, A., and Rahav, E. (2016). The impact of dry atmospheric deposition on the sea-surface microlayer in the se mediterranean sea: an experimental approach. Front. Mar. Sci. 3:222. doi: 10.3389/fmars.2016.00222
Baier, R. E. (1972), Organic films on natural waters: their retrieval, identification, and modes of elimination, J. Geophys. Res. 77, 5062–5075. doi: 10.1029/JC077i027p05062
Bailey, C. A., Neihof, R. A., and Tabor, P. S. (1983). Inhibitory effect of solar radiation on amino acid uptake in Chesapeake Bay bacteria. Appl. Environ. Microbiol. 46, 44–49.
Bates, T. S., Quinn, P. K., Frossard, A. A., Russell, L. M., Hakala, J., Petäjä, T., et al. (2012). Measurements of ocean derived aerosol off the coast of California. J. Geophys. Res. 117, 1–13. doi: 10.1029/2012JD017588
Bock, E. J., Hara, T., Frew, N. M., and McGillis, W. R. (1999). Relationship between air-sea gas transfer and short wind waves. J. Geophys. Res. 104, 25821–25831. doi: 10.1029/1999JC900200
Calleja, M. L., Duarte, C. M., Navarro, N., and Agustí, S. (2005). Control of air-sea CO2 disequilibria in the subtropical NE Atlantic by planktonic metabolism under the ocean skin. Geophys. Res. Lett. 32:L08606. doi: 10.1029/2004GL022120
Carlson, D. J. (1983). Dissolved organic materials in surface microlayers: temporal and spatial variability in relation to sea state. Limnol. Oceanogr. 28, 415–431. doi: 10.4319/lo.1983.28.3.0415
Carlucci, A., Craven, D., and Henrichs, S. (1985). Surface-film microheterotrophs: amino acid metabolism and solar radiation effects on their activities. Mar. Biol. 85, 13–22. doi: 10.1007/BF00396410
Carlucci, A. F., Wolgast, D. M., and Craven, D. B. (1992). Microbial populations in surface films: amino acid dynamics in nearshore and offshore waters off Southern California. J. Geophys. Res. 97, 5271–5280. doi: 10.1029/91JC02614
Carpenter, L. J., and Nightingale, P. D. (2015). Chemistry and release of gases from the surface ocean. Chem. Rev. 115, 4015–4034. doi: 10.1021/cr5007123
Ciuraru, R., Fine, L., van Pinxteren, M., D'Anna, B., Herrmann, H., and George, C. (2015). Unravelling new processes at interfaces: photochemical isoprene production at the sea surface. Environ. Sci. Technol. 49, 13199–13205. doi: 10.1021/acs.est.5b02388
Conrad, R., and Seiler, W. (1988). Influence of the surface microlayer on the flux of nonconservative trace gases (CO, H2, CH4, N2O) across the ocean-atmosphere interface. J. Atmos. Chem. 6, 83–94. doi: 10.1007/BF00048333
Cunliffe, M., Engel, A., Frka, S., Gašparović, B., Guitart, C., Murrell, J. C., et al. (2013). Sea surface microlayers: a unified physicochemical and biological perspective of the air–ocean interface. Prog. Oceanogr. 109, 104–116. doi: 10.1016/j.pocean.2012.08.004
Cunliffe, M., Upstill-Goddard, R. C., and Murrell, J. C. (2011). Microbiology of aquatic surface microlayers. FEMS Microbiol. Rev. 35, 233–246. doi: 10.1111/j.1574-6976.2010.00246.x
Dauwe, B., and Middelburg, J. J. (1998). Amino acids and hexosamines as indicators of organic matter degradation state in North Sea sediments. Limnol. Oceanogr. 43, 782–798. doi: 10.4319/lo.1998.43.5.0782
Dauwe, B., Middelburg, J. J., Herman, P. M. J., and Heip, C. H. R. (1999). Linking diagenetic alteration of amino acids and bulk organic matter reactivity. Limnol. Oceanogr. 44, 1809–1814. doi: 10.4319/lo.1999.44.7.1809
Davis, J., Kaiser, K., and Benner, R. (2009). Amino acid and amino sugar yields and compositions as indicators of dissolved organic matter diagenesis. Org. Geochem. 40, 343–352. doi: 10.1016/j.orggeochem.2008.12.003
Dickson, A. G., Sabine, C. L., and Christian, J. R. (2007). Guide to Best Practices For Ocean CO2 Measurements. Sidney, BC: PICES.
Dittmar, T., Cherrier, J., and Ludwichowski, K. (2009). “The analysis of amino acids in seawater,” in Practical Guidelines for the Analysis of Seawate, ed O. Wurl (Boca Raton, FL: CRC-Press), 67–78.
Dragcevic, D., and Pravdic, V. (1981). Properties of seawater-air interfaces: rates of surface film formation under steady state conditions. Limnol. Oceanogr. 26, 492–499. doi: 10.4319/lo.1981.26.3.0492
Engel, A., Bange, H. W., Cunliffe, M., Burrows, S. M., Freidrichs, G., Galgani, L., et al. (2017). The ocean's vital skin: towards an integrated understanding of the sea surface microlayer. Front. Mar. Sci. 4:165. doi: 10.3389/fmars.2017.00165
Engel, A., and Galgani, L. (2016). The organic sea-surface microlayer in the upwelling region off the coast of Peru and potential implications for air–sea exchange processes. Biogeosciences 13, 989–1007. doi: 10.5194/bg-13-989-2016
Engel, A., and Händel, N. (2011). A novel protocol for determining the concentration and composition of sugars in particulate and in high molecular weight dissolved organic matter (HMW-DOM) in seawater. Mar. Chem. 127, 180–191. doi: 10.1016/j.marchem.2011.09.004
Engel, A., Händel, N., Wohlers, J., Lunau, M., Grossart, H. P., and Riebesell, U. (2011). Effects of sea surface warming on the production and composition of dissolved organic matter during phytoplankton blooms: results from a mesocosm study. J. Plankton Res. 33, 357–372. doi: 10.1093/plankt/fbq122
Frew, N. M. (2005). “The role of organic films in air-sea gas exchange,” in The Sea Surface and Global Change, eds S. Pliss and R. A. Duce (Cambridge: Cambridge University Press), 121–163.
Frew, N. M., Goldman, J. C., Dennett, M. R., and Johnson, A. S. (1990). Impact of phytoplankton-generated surfactants on air-sea gas exchange. J. Geophys. Res. Oceans 95, 3337–3352. doi: 10.1029/JC095iC03p03337
Frost, T. (1999). Environmental Controls of Air-Water Gas Exchange. University of Newcastle upon Tyne, UK.
Galgani, L., and Engel, A. (2016). Changes in optical characteristics of surface microlayers hint to photochemically and microbially mediated DOM turnover in the upwelling region off the coast of Peru. Biogeosciences 13, 2453–2473. doi: 10.5194/bg-13-2453-2016
Galgani, L., Piontek, J., and Engel, A. (2016). Biopolymers form a gelatinous microlayer at the air-sea interface when Arctic sea ice melts. Sci. Rep. 6:29465. doi: 10.1038/srep29465
GESAMP (1995). The Sea-Surface Microlayer and its Role in Global Change. Reports and Studies No. 59. WMO.
Goldman, J. C., Dennett, M. R., and Frew, N. M. (1988). Surfactant effects on air-sea gas exchange under turbulent conditions. Deep Sea Res. Part A. Oceanograp. Res. Papers 35, 1953–1970. doi: 10.1016/0198-0149(88)90119-7
Grossart, H., Engel, A., Arnosti, C., C., L., De La Rocha, Murray, A. E., and Passow, U. (2007). Microbial dynamics in autotrophic and heterotrophic seawater mesocosms. III. Organic matter fluxes. Aqua. Microb. Ecol. 49, 143–156. doi: 10.3354/ame01140
Hansen, H. P., and Koroleff, F. (1999). “Determination of nutrients,” in Methods of seawater analysis, 3rd Edn. eds K. Grasshoff, K. Kremling, and M. Ehrhardt (Weinheim: Wiley).
Hardy, J. T., Apts, C. W., Crecelius, E. A., and Fellingham, G. W. (1985). The sea-surface microlayer: fate and residence times of atmospheric metals. Limnol. Oceanogr. 30, 93–101. doi: 10.4319/lo.1985.30.1.0093
Harvey, G. W., and Burzell, L. A. (1972). A simple microlayer method for small samples. Limnol. Oceanogr. 11, 608–614. doi: 10.4319/lo.1966.11.4.0608
Henrichs, S. M., and Williams, P. M. (1985). Dissolved and particulate amino acids and carbohydrates in the sea surface microlayer. Mar. Chem. 17, 141–163. doi: 10.1016/0304-4203(85)90070-2
Herndl, G. J., Agogué, H., Baltar, F., Reinthaler, T., Sintes, E., and Varela, M. M. (2008). Regulation of aquatic microbial processes: the ‘microbial loop’ of the sunlit surface waters and the dark ocean dissected. Aqua. Microb. Ecol. 53, 59–68. doi: 10.3354/ame01225
Hoppe, H.-G., Giesenhagen, H. C., Koppe, R., Hansen, H.-P., and Gocke, K. (2013). Impact of change in climate and policy from 1988 to 2007 on environmental and microbial variables at the time series station Boknis Eck, Baltic Sea. Biogeosciences 10, 4529–4546. doi: 10.5194/bg-10-4529-2013
Infante, M. R., Pérez, L., Pinazo, A., Clapés, P., Morán, M. C., and Vinardell, M. T. (2004). Amino acid-based surfactants. C. R. Chimie 7, 583–592. doi: 10.1016/j.crci.2004.02.009
Jayarathne, T., Sultana, C. M., Lee, C., Malfatti, F. K., and Cox, J. L. (2016). Enrichment of saccharides and divalent cations in sea spray aerosol during two phytoplankton blooms. Environ. Sci. Technol. 50, 11511–11520. doi: 10.1021/acs.est.6b02988
Joux, F. J., Agogué, H., Obernosterer, I., Dupuy, C., Reinthaler, T. J., and Lebaron, P. (2006). Microbial community structure in the sea surface microlayer at two contrasting coastal sites in the northwestern Mediterranean Sea. Aqua. Microb. Ecol. 42, 91–104. doi: 10.3354/ame042091
Krall, K. (2013). Laboratory Investigations of Air-Sea Gas Transfer under a Wide Range of Water Surface Conditions. Dissertation, Faculties for the Natural Sciences and for Mathematics of the Ruperto-Carola University of Heidelberg, Germany, 152,
Kuznetsova, M., and Lee, C. (2001). Enhanced extracellular enzymatic peptide hydrolysis in the sea-surface microlayer. Mar. Chem. 73, 319–332. doi: 10.1016/S0304-4203(00)00116-X
Kuznetsova, M., Lee, C., Aller, J., and Frew, N. (2004). Enrichment of amino acids in the sea surface microlayer at coastal and open ocean sites in the North Atlantic Ocean. Limnol. Oceanogr. 49, 1605–1619. doi: 10.4319/lo.2004.49.5.1605
Laß, K., Bange, H., and Friedrichs, G. (2013). Seasonal signatures in SFG vibrational spectra of the sea surface nanolayer at boknis eck time series station (SWBaltic Sea). Biogeosciences 10, 5325–5334. doi: 10.5194/bg-10-5325-2013
Laß, K., and Friedrichs, G. (2011). Revealing structural properties of the marine nanolayer from vibrational sum frequency generation spectra. J. Geophys. Res. 116, 1–15. doi: 10.1029/2010JC006609
Laß, K., Kleber, J., and Friedrichs, G. (2010). Vibrational sum-frequency generation as a probe for composition, chemical reactivity, and film formation dynamics of the sea surface nanolayer. Limnol. Oceanogr. Methods 8, 216–228. doi: 10.4319/lom.2010.8.216
Lê, S., Josse, J., and Husson, F. (2008). FactoMineR: an R package for multivariate analysis. J. Stat. Softw. 25, 1–18. doi: 10.18637/jss.v025.i01
Lindroos, A., Szabo, H. M., Nikinmaa, M., and Leskinen, P. (2011). Comparison of sea surface microlayer and subsurface water bacterial communities in the Baltic Sea. Aqua. Microb. Ecol. 65, 29–42. doi: 10.3354/ame01532
Lindroth, P., and Mopper, K. (1979). High performance liquid chromatographic determination of subpicomole amounts of amino acids by precolumn fluorescence derivatization with o-phthaldialdehyde. Anal. Chem. 51, 1667–1674. doi: 10.1021/ac50047a019
Matrai, P. A., Tranvik, L., Leck, C., and Knulst, J. C. (2008). Are high Arctic surface microlayers a potential source of aerosol organic precursors? Mar. Chem. 108, 109–122. doi: 10.1016/j.marchem.2007.11.001
McKenna, S. P., and McGillis, W. R. (2004). The role of free-surface turbulence and surfactants in air-water gas transfer. Int. J. Heat Mass Transf. 47, 539–553. doi: 10.1016/j.ijheatmasstransfer.2003.06.001
Mustaffa, N. I. H., Striebel, M., and Wurl, O. (2017). Enrichment of extracellular carbonic anhydrase in the sea surface microlayer and its effect on air-sea exchange. Geophys. Res. Lett. 44, 12324–12330. doi: 10.1002/2017GL075797
Nagel, L., Krall, K. E., and Jähne, B. (2015). Comparative heat and gas exchange measurements in the Heidelberg Aeolotron, a large annular wind-wave tank. Ocean Sci. 11, 111–120. doi: 10.5194/os-11-111-2015
Nakajima, R., Tsuchiya, K., Nakatomi, N., Yoshida, T., Tada, Y., Konno, F., et al. (2013). Enrichment of microbial abundance in the sea-surface microlayer over a coral reef: implications for biogeochemical cycles in reef ecosystems. Mar. Ecol. Prog. Ser. 490, 11–22. doi: 10.3354/meps10481
Obernosterer, I., Reitner, B., and Herndl, G. J. (1999). Contrasting effects of solar radiation on dissolved organic matter and its bioavailability to marine Bacterioplankton. Limnol. Oceanogr. 44, 1645–1654. doi: 10.4319/lo.1999.44.7.1645
O'Dowd, C. D., Facchini, M. C., Cavalli, F., Ceburnis, D., Mircea, M., Decesari, S., et al. (2004). Biogenically driven organic contribution to marine aerosol. Nature 431, 676–680. doi: 10.1038/nature02959
Pedler, B. E., Aluwihare, L. I., and Azam, F. F. (2014). Single bacterial strain capable of significant contribution to carbon cycling in the surface ocean. Proc. Natl. Acad. Sci. U.S.A. 111, 7202–7207. doi: 10.1073/pnas.1401887111
Prather, K., Bertram, T. H., Grassian, V. H., Deane, G. B., Stokes, M. D., DeMott, P. J., et al. (2013). Bringing the ocean into the laboratory to probe the chemical complexity of sea spray aerosol. Proc. Natl. Acad. Sci. U.S.A. 110, 7550–7555. doi: 10.1073/pnas.1300262110
Quinn, P. K., Bates, T. S., Schulz, K. S., Coffman, D. J., Frossard, A. A., Russel, L. M., et al. (2014). Contribution of sea surface carbon pool to organic matter enrichment in sea spray aerosol. Nat. Geosci. 7, 228–232. doi: 10.1038/ngeo2092
Quinn, P. K., and Bates, T. S. (2011). The case against climate regulation via oceanic phytoplankton sulphur emissions. Nature 480, 51–56. doi: 10.1038/nature10580
Rahlff, J., Stolle, C., Giebel, H.-A., Brinkhoff, T., Ribas-Ribas, M., Hodapp, D., et al. (2017). High wind speeds prevent formation of a distinct bacterioneuston community in the sea-surface microlayer. FEMS Microbiol. Ecol. 93:fix041. doi: 10.1093/femsec/fix041
R Core Team (2013). R: A Language and Environment for Statistical Computing. R Foundation for Statistical Computing, Vienna, Austria. Available online at: http://www.R-project.org/
Reinthaler, T., Sintes, E., and Herndl, G. J. (2008). Dissolved organic matter and bacterial production and respiration in the sea surface microlayer of the open Atlantic and the western Mediterranean Sea. Limnol. Oceanogr. 53, 122–136. doi: 10.4319/lo.2008.53.1.0122
Russell, L. M., Hawkins, L. N., Frossard, A. A., Quinn, P. K., and Bates, T. S. (2010). Carbohydrate-like composition of submicron atmospheric particles and their production from ocean bubble bursting. Proc. Natl. Acad. Sci. U.S.A. 107, 6652–6657. doi: 10.1073/pnas.0908905107
Sabbaghzadeh, B., Upstill-Goddard, R. C., Beale, R., Pereira, R., and Nightingale, P. D. (2017). The Atlantic Ocean surface microlayer from 50°N to 50°S is ubiquitously enriched in surfactants at wind speeds up to 13 m s−1. Geophys. Res. Lett. 44, 2852–2858. doi: 10.1002/2017GL072988
Santos, A. L., Oliveira, V., Baptista, I., Henriques, I., Gomes, N. C., Almeida, A., et al. (2012). Effects of UV-B radiation on the structural and physiological diversity of bacterioneuston and bacterioplankton. Appl. Environ. Microbiol. 78, 2066–2069. doi: 10.1128/AEM.06344-11
Satpute, S. K., Banat, I. M., Dhakephalkar, P. K., Banpurkar, A. G., and Chopade, B. A. (2010). Biosurfactants, bioemulsifiers and exopolysaccharides from marine microorganisms. Biotechnol. Adv. 28, 436–450. doi: 10.1016/j.biotechadv.2010.02.006
Schreiber, F., Wunderlin, P., Udert, K. M., and Wells, G. F. (2012). Nitricoxide and nitrousoxide turnover in natural and engineered microbial communities: biological pathways, chemical reactions,and novel technologies. Front. Microbiol. 3:372. doi: 10.3389/fmicb.2012.00372
Stolle, C., Labrenz, M., Meeske, C., and Jürgens, K. (2011). Bacterioneuston community structure in the southern baltic sea and its dependence on meteorological conditions. Appl. Environ. Microb. 77, 3726–3733. doi: 10.1128/AEM.00042-11
Stolle, C., Nagel, K., Labrenz, M., and Jürgens, K. (2009). Bacterial activity in the sea-surface microlayer: in situ investigations in the Baltic Sea and the influence of sampling devices. Aqua. Microb. Ecol. 58, 67–78. doi: 10.3354/ame01351
Sugimura, Y., and Suzuki, Y. (1988). A high-temperature catalytic oxidation method for the determination of non-volatile dissolved organic carbon in seawater by direct injection of a liquid sample. Mar. Chem. 24, 105–131. doi: 10.1016/0304-4203(88)90043-6
Sun, C. C., Sperling, M., and Engel, A. (2017). Effect of wind speed on the size distribution of biogenic gel particles in the sea surface microlayer: insights from a wind wave channel experiment. Biogeosci. Discuss. doi: 10.5194/bg-2017-419
Upstill-Goddard, R. C., Frost, T., Henry, G. R., Franklin, M., Murrell, J. C., and Owens, N. J. (2003). Bacterioneuston control of air-water methane exchange determined with a laboratory gas exchange tank. Global Biogeochem. Cycles 17:1108. doi: 10.1029/2003GB002043
van Pinxteren, M., Barthel, S., Fomba, K. W., Müller, K., von Tümpling, W., and Herrmann, H. (2017). The influence of environmental drivers on the enrichment of organic carbon in the sea surface microlayer and in submicron aerosol particles – measurements from the Atlantic Ocean. Elem. Sci. Anth. 5:35. doi: 10.1525/elementa.225
Welschmeyer, N. A. (1994). Fluometric analysis of chlorophyll a in the presence of chlorophyll b and pheopigments. Limnol. Oceanogr. 39, 1985–1992. doi: 10.4319/lo.1994.39.8.1985
Wilson, T. W., Ladino, L. A., Alpert, P. A., Breckels, M. N., Brooks, I. M., Browse, J., et al. (2015). A marine biogenic source of atmospheric ice nucleating particles. Nature 525, 234–238. doi: 10.1038/nature14986
Wurl, O., Miller, L., and Vagle, S. (2011a). Production and fate of transparent exopolymer particles in the ocean. J. Geophys. Res. Oceans 116:C00H13. doi: 10.1029/2011JC007342
Wurl, O., Stolle, C., Van Thuoc, C., The Thu, P., and Mari, X. (2016). Biofilm-like properties of the sea surface and predicted effects on air-sea CO2 exchange. Prog. Oceanogr. 144, 15–24. doi: 10.1016/j.pocean.2016.03.002
Wurl, O., Wurl, E., Miller, L., Johnson, K., and Vagle, S. (2011b). Formation and global distribution of sea-surface microlayers. Biogeosciences 8, 121–135. doi: 10.5194/bg-8-121-2011
Zäncker, B., Bracher, A., Röttgers, R., and Engel, A. (2017). Variations of the organic matter composition in the sea surface microlayer: a comparison between open ocean, coastal, and upwelling sites off the peruvian coast. Front. Microbiol. 8:2369. doi: 10.3389/fmicb.2017.02369
Zhang, Z. (2003). Studies on the sea surface microlayer II. The layer of sudden change of physical and chemical properties, J. Coll. Inter. Sci. 264, 148–159. doi: 10.1016/S0021-9797(03)00390-4
Keywords: air-sea interface, dissolved organic carbon, amino acids, carbohydrates, prokaryotes, surfactants
Citation: Engel A, Sperling M, Sun C, Grosse J and Friedrichs G (2018) Organic Matter in the Surface Microlayer: Insights From a Wind Wave Channel Experiment. Front. Mar. Sci. 5:182. doi: 10.3389/fmars.2018.00182
Received: 02 February 2018; Accepted: 07 May 2018;
Published: 05 June 2018.
Edited by:
Cecile Guieu, Centre National de la Recherche Scientifique (CNRS), FranceReviewed by:
Marta Plavsic, Rudjer Boskovic Institute, CroatiaElvira Pulido-Villena, UMR7294 Institut Méditerranéen d'Océanographie (MIO), France
Janina Rahlff, Universität Duisburg-Essen, Germany
Copyright © 2018 Engel, Sperling, Sun, Grosse and Friedrichs. This is an open-access article distributed under the terms of the Creative Commons Attribution License (CC BY). The use, distribution or reproduction in other forums is permitted, provided the original author(s) and the copyright owner are credited and that the original publication in this journal is cited, in accordance with accepted academic practice. No use, distribution or reproduction is permitted which does not comply with these terms.
*Correspondence: Anja Engel, YWVuZ2VsQGdlb21hci5kZQ==