- 1Institute of Biological and Environmental Science, School of Biological Sciences, University of Aberdeen, Aberdeen, United Kingdom
- 2Surface Chemistry and Catalysis Group, Materials and Chemical Engineering, School of Engineering, University of Aberdeen, Aberdeen, United Kingdom
- 3Marine Scotland Science, Marine Laboratory Aberdeen, Aberdeen, United Kingdom
Deep sea oil exploration is increasing and presents environmental challenges for deep ocean ecosystems. Marine oil spills often result in contamination of sediments with oil; following the Deepwater Horizon (DwH) disaster up to 31% of the released oil entrained in the water column was deposited as oily residues on the seabed. Although the aftermath of DwH was studied intensely, lessons learned may not be directly transferable to other deep-sea hydrocarbon exploration areas, such as the Faroe-Shetland Channel (FSC) which comprises cold temperatures and a unique hydrodynamic regime. Here, transport of hydrocarbons into deep FSC sediments, subsequent responses in benthic microbial populations and effects of dispersant application on hydrocarbon fate and microbial communities were investigated. Sediments from 1,000 m in the FSC were incubated at 0°C for 71 days after addition of a 20-hydrocarbon component oil-sediment aggregate. Dispersant was added periodically from day 4. An additional set of cores using sterilized and homogenized sediment was analyzed to evaluate the effects of sediment matrix modification on hydrocarbon entrainment. Sediment layers were independently analyzed for hydrocarbon content by gas chromatography with flame ionization detection and modeled with linear mixed effects models. Oil was entrained over 4 cm deep into FSC sediments after 42 days and dispersant effectiveness on hydrocarbon removal from sediment to the water column decreased with time. Sterilizing and homogenizing sediment resulted in hydrocarbon transport over 4 cm into sediments after 7 days. Significant shifts in bacterial populations were observed (DGGE profiling) in response to hydrocarbon exposure after 42 days and below 2 cm deep. Dispersant application resulted in an accelerated and modified shift in bacterial communities. Bacterial 16S rRNA gene sequencing of oiled sediments revealed dominance of Colwellia and of Fusibacter when dispersant was applied over oiled sediments. The increased relative abundance of anaerobic hydrocarbon degraders through time suggests creation of anoxic niches due to smothering. The study showed that hydrocarbons can entrain deep sediments to over 4 cm in a short time and that FSC indigenous bacteria are able to respond to a contamination event, even at a low temperature, reflecting the in situ conditions.
Introduction
Oil and gas exploration in the deep sea is increasing as shallow and more accessible sources become depleted (Leffler et al., 2011; Ramirez-Llodra et al., 2011). The Faroe-Shetland Channel (FSC) is an area of deep-water hydrocarbon exploration (Smallwood and Kirk, 2005; Gallego et al., 2018) where hydrocarbon prospecting is occurring at depths of up to 1,500 m (Lagavulin well: 1,567 m depth, 62°39′N, 1°7′W). There are numerous concerns over environmental implications stemming from oil drilling activity, production and potential spillages/release to areas such as the FSC which require further investigation (Cordes et al., 2016).
The exposure of the marine environment to hydrocarbons can cause serious detriment to localized and wide-scale regional ecosystems (Mearns et al., 2010). Oil can be released to the water column directly, as witnessed following the Deepwater Horizon (DwH) well blowout in the Gulf of Mexico (GoM) in 2010 (Atlas and Hazen, 2011; Schrope, 2011; Montagna et al., 2013; Joye et al., 2016). More commonly oil is released to surface waters in shipping accidents such as the Exxon Valdez spill in 1989 (Atlas and Hazen, 2011) and Prestige spill in 2002 (Acosta-González et al., 2015). Following an oil spill in the water column or sea surface hydrocarbons are transported to the seabed, often in large quantities and across vast areas (Valentine et al., 2014). The quantity of oil transported to the seabed during the DwH was estimated to range between 1.8 and 31% of the water column-entrained oil (Lubchenco et al., 2010; Chanton et al., 2014; Valentine et al., 2014). There have been several oil-to-seabed transportation mechanisms proposed (Romero et al., 2015): (1) a combination of advective transport and oceanic currents carried the oil entrained plume into the continental slope leaving a “bathtub” ring of oily residue (Valentine et al., 2014); (2) the formation of oil-mineral aggregates and marine oil snow were deposited to the seafloor (Passow et al., 2012; Ziervogel et al., 2012); (3) a loss of buoyancy of oil droplets and oil-mineral aggregates in the water column resulted in deposition (Gong et al., 2014a) and (4) the ingestion of oil or oil-mineral aggregates by zooplankton and excretion as fecal pellets that settled on the seabed (Muschenheim and Lee, 2002).
The sedimentation pulses that took place following the DwH spill (Chanton et al., 2014; Valentine et al., 2014; Romero et al., 2015) led to the formation of flocculent oily material on the seabed which covered corals (White et al., 2012), reduced natural bioturbation (Brooks et al., 2015) and altered the microbial community (Yang et al., 2016). Although it was determined that the indigenous microflora and fauna were capable of responding to and degrading the influx of hydrocarbons (Kimes et al., 2014), major shifts within sediment microbial populations in the months following the spill had varying effects on geochemical cycling and redox conditions (Kimes et al., 2013; Scott et al., 2014; Hastings et al., 2016). Anaerobic conditions often prevailed in normally aerated sediments due to smothering by floc and rapid oxygen consumption (Yang et al., 2016), yet Mason et al. (2014a) and Kimes et al. (2013) both detected aerobic and anaerobic hydrocarbon degraders in sediments surrounding the Macondo well following DwH. The shift in redox state to anaerobic conditions meant that deposited hydrocarbons would undergo slower degradation compared to aerobic processes (Head et al., 2006; Widdel et al., 2010), leading to persistence of harmful and toxic oil components in the environment (Hylland, 2006; Marini and Frapiccini, 2013). Seabed-bound hydrocarbons are subjected to physical and biological processes which can translocate them (Konovalov et al., 2010; Zuijdgeest and Huettel, 2012). Solutes can be transported into sediments by diffusive and advective pore water fluxes (Huettel et al., 2014) and hydrocarbons may desorb from sediment, dissolve in the water column and be transported to remote locations (Zhao et al., 2015) where they may be degraded within the water column. The environmental conditions in the deep sea vary with location and a greater understanding of how hydrocarbons entrain and are removed from deep sea sediments is required to assess environmental risks in the event of an oil spill similar to DwH in a different location such as the FSC.
Deepwater hydrocarbon exploration in the FSC has been underway for over 20 years (Austin et al., 2014; Gallego et al., 2018) with fields such as Schiehallion (350–450 m) in 1993 and more recently the North Uist prospect (~1300 m) in 2012. Increased exploration in this region at great depths presents a potential risk of oil spills in the FSC and an outcome analogous to the deep sea intrusion layers and sedimentation pulses observed following DwH is certainly possible. Direct application of lessons learned from DwH may, however, be misguided as the environmental conditions differ to those in the GoM (The Energy and Climate Change Committee, 2011). Temperatures at depths > 1000 m in the FSC are ~0°C, thus colder than temperatures in the GoM (~5°C), and are accompanied by an extreme hydrodynamic regime comprising complex multidirectional water masses (Berx et al., 2013). The transport processes of hydrocarbons in the FSC are likely to be more complex than those observed in the GoM and oil would inevitably be dispersed over a vast area (Main et al., 2016). The FSC is an important region hosting diverse benthic habitats and parts of it have been designated as marine protected areas (e.g., the Faroe-Shetland Sponge Belt Nature Conservation Marine Protected Area; Joint Nature Conservation Committee, 2014). The FSC is known to have intense benthic ecological activity and bioturbation (Jones et al., 2007; Gontikaki et al., 2011), potentially enhancing hydrocarbon transport into sediments. Unlike GoM sediments, FSC microflora and bacterial communities have not been pre-exposed to hydrocarbons, a factor which contributed to the efficiency of microbial oil degradation following DwH (Hazen et al., 2010; Joye et al., 2014). Hydrocarbon degradation in the FSC may be slower compared to that in the GoM due to ~0°C temperatures prevailing below 600 m (Ferguson et al., 2017). The FSC is of high environmental interest as a proxy for potential oil spills in deep arctic ecosystems since North Atlantic Deep Water formed in the Arctic flows southwards at depths over 600 m in the channel (Berx et al., 2013). In particular, ~50% of North Atlantic Deep Water flows through the FSC. It follows that an oil spill in this location would entail the risk of contaminating the North Atlantic's deep water supply.
In response to the DwH blowout, 7 million liters of chemical dispersant (mainly Corexit 9500 and 9527A) were applied to both the surface slick and deep sea plume. Dispersant reduces the surface tension of the oil-water interface, transforming large globules of oil into smaller droplets enhancing solubilization and dissolution; this is proposed to enhance biodegradation rates as hydrocarbons become more bioavailable (Kleindienst et al., 2015b). However, there are concerns over the toxicity and degradability of dispersants (Scarlett et al., 2005) and their efficacy is inconclusive with studies reporting both suppression (Kleindienst et al., 2015b) and stimulation of microbial oil degradation (Baelum et al., 2012). The effect of dispersants on oil-sediment interactions is currently under investigation and contrasting effects on hydrocarbon sorption to marine sediments have been observed (Zhao et al., 2015). Oil dispersants selectively enhance sorption of aliphatic and aromatic hydrocarbons to marine sediments at different dispersant concentrations (Zhao et al., 2016). These effects varied with type of oil dispersant used, highlighting that an understanding of the effects of commercial dispersants on oil-sediment-microbe interactions requires further investigation.
The aims of this study were to evaluate the propensity of hydrocarbons to be transported into and out of FSC sediments following oil-sediment particle deposition and the subsequent bacterial community response over time. An additional aim was to determine the effects of a commercial oil dispersant (Superdispersant-25, SD25 hereafter) on these processes, and identify bacteria responding to oil in the presence or absence of SD25 treatments in deep sea sediments. To achieve this, the following hypotheses were developed: (1) post-depositional transport of hydrocarbons in surficial sediments at in situ temperatures triggers a stratified bacterial community response, (2) chemical dispersant increases hydrocarbon mobility and accessibility to microbial communities enhancing shifts to a hydrocarbon-degrading population and (3) sedimentary matrix modification results in enhanced mobility of hydrocarbons through surficial sediments. To our knowledge, this is the first study to evaluate hydrocarbon transport and subsequent bacterial community response in naturally stratified deep sea sediments of subarctic origin at in situ temperatures.
Materials and Methods
Sediment and Seawater Collection, Transportation, and Preparation
Sediment and seawater samples were collected on May 2014 and May 2015 on-board MRV Scotia (cruise numbers: 0514S and 0515S, respectively) in the FSC from two stations (Supplementary Figure 1). Sediments from station A (1000 m deep; 61°35.02′N, 4°14.64′W), collected in 2015 cruise using a maxi-corer (OSIL, UK) fitted with acrylic cores (internal diameter = 10 cm, length = 60 cm), were used in “undisturbed sediments” experiments. Four sub-cores (acrylic, internal diameter = 3.6 cm, length = 30 cm) of ~10 cm sediment depth were collected from each Maxi-corer core on-board. The sub-cores were stored fully submerged in seawater baths inside a temperature-controlled unit at 0°C that allowed water circulation and were individually aerated via air stones attached to an air pump to prevent anoxia. Following transport to the laboratory, the sub-cores were allowed to acclimatize for 10 days at 0°C until initiation of the experiments. Seawater was collected from station A using a rosette equipped with Niskin bottles during the 2015 cruise to be used in the undisturbed experiment reservoir system. Sediments were collected using a van Veen grab from station B at 180 m depth (69° 49.08′N, 5° 21.03′W) during the 2015 cruise and used in the production of oil-sediment pellets (see below).
A second set of sediments, collected using a van Veen grab during the 2014 cruise from station A, were sterilized and homogenized, and used in the transport experiment (“modified sediments,” hereafter). Once transported to the lab modified sediments were mechanically homogenized and autoclaved at 120°C and 100 kPa for 21 min. Thereafter, sub-cores were made up to 10 cm with the modified sediment and filled up with UV-filtered seawater (0.5 μm filter) collected from the Ythan estuary. Sediment and seawater characterization was performed using methods described in (Supplementary Methods 1).
Model Oil and Artificial Oil-Sediment Pellets
A model oil was prepared with 20 hydrocarbons with a resulting density of 880 kg m−3, similar to a medium crude oil (Ferguson et al., 2017). The model oil was composed of 64.9% aliphatic hydrocarbons, 30.0% combined monoaromatic and PAHs and 5.1% resin fractions. The hydrocarbons used to make the model oil, and their characteristics are listed in Supplementary Table 1. Model oil was filter-sterilized through a 0.22 μm PTFE filter (VWR).
Oil-sediment pellets (OSPs) were made using the 300–350 μm fraction of station B sediments after removal of organic carbon (450°C, 12 h). This ensured that the sediment used was homogenous in terms of organic matter and particle size. To make the 3-cm OSPs, 4 g of treated sediment were placed in tin foil and 1 ml of model oil and 1 ml of MilliQ water (18.2 MΩ cm, 25°C) were added. The OSPs were stored at −20°C until use. To evaluate the exact amount of model oil components retained in individual pellets, three replicate OSPs were extracted by Soxhlet. On average, 0.41 ± 0.09 g (error = standard deviation, n = 3) of the oil was retained in the OSPs (Supplementary Table 1).
Incubation of Oiled Seawater-Sediment Systems
Undisturbed sediment sub-cores were removed from the water baths and lined around a rosette fitted with magnets. The cores were subject to three treatments; no oil (control), oil only (O-treatment) and oil and dispersant (OD-treatment). O- and OD-treatments were inoculated with artificial OSPs. All sub-cores were sealed with modified rubber stoppers on the upper end, leaving no headspace (Supplementary Figure 2). The modified stoppers were fitted with magnetic stirrers and two hollow steel pipes ~8 cm above the sediment surface to enable water exchanges to take place throughout the experiment. The rosette rotated to move the magnetic stirrers inside the sub-cores and simulated advection of supernatant water (20 rpm). The system was kept in darkness and held at 0°C for the duration of the experiment. Supernatant water was periodically replaced to emulate replenishment of seawater in the water column and prevent anoxia in surficial sediments. Each sub-core was connected to a water reservoir (225 ml) and water was exchanged between sub-cores and reservoirs using a 520S peristaltic pump (Watson Marlow) at a rate of 25 ml min−1 for 20 min twice per week. SD25 (Oil Technics, UK) was added to OD-treatment reservoirs (33 μl, 1:30 SD25:oil ratio, based on manufacturer's recommendation) when water exchanges were performed to evaluate the effect of SD25 on transport and solubilization of hydrocarbons. Triplicate sub-cores were analyzed per time point, treatment and sediment type.
Hydrocarbon Extraction and Analysis
At specified time intervals (7, 21, 42, and 71 days) hydrocarbons were quantified from each of the 4 depth layers (0–1, 1–2, 2–4, and 4–10 cm). Approximately 10 g of sediment from each section was stored in glass vials with PTFE caps at −20°C prior to hydrocarbon extraction. Supernatant seawater was collected and stored in amber vials with PTFE caps and maintained at −20°C until extraction. Hydrocarbons were extracted from sediment by Soxhlet extraction for ~40 cycles (5 h in total) using 100 ml dichloromethane. Hydrocarbons were liquid-liquid extracted from the total volume of seawater of each core (~150 ml) with 3 × 10 ml dichloromethane and the resulting extractions combined. Extraction recovery rates for all components were performed in triplicate and can be found in Supplementary Table 1. Hydrocarbon extractions were analyzed by gas chromatography fitted with a flame ionization detector (GC-FID) using a previously described system and method (Ferguson et al., 2017). Calibration curves (6-point) were determined for each compound of the model oil. Laboratory control samples were analyzed to establish the effect of the sediment matrix and extraction procedure on the recovery of model oil compounds. Toluene was added as an internal standard to account for injection error (1 μl ml−1). The limits of detection and quantification were defined as chromatographic signal to noise ratios of 3 and 10, respectively.
Bacterial Community Analysis
DNA Extractions
Total genomic DNA was extracted from 0.4 g sediment using the FastDNA™ SPIN Kit for Soil and FastPrep®-24 instrument (both MP Biomedicals, Cambridge, UK), according to manufacturer's instructions. Purified DNA was stored at −20°C until further analysis.
Denaturing Gradient Gel Electrophoresis (DGGE)
DGGE was performed on all sediment layers and shows differences in bacterial profile through depth. The conserved V3-V5 region of bacterial 16S rRNA gene was amplified using the primer pair 341F (with GC clamp) (Muyzer et al., 1993) and 907R (Muyzer and Smalla, 1998). Each PCR reaction contained 1 μl of target DNA extract (diluted to < 20 ng μl−1), 2 μl PCR water, 45 μl Red Taq DNA Polymerase Master Mix with 1.5 mM MgCl2 and 1 μl of each primer (10 μM). Amplification was carried out on a Techne thermal cycler (Techne, UK) using a step-down PCR programme as follows: initial denaturation at 95°C for 4 min, followed by 10 cycles of 94°C for 30 s, 62°C for 45 s, and 72°C for 1 min, followed by 25 cycles of 94°C for 30 s, 57°C for 40 s, and 72°C for 1 min, plus final extension for 10 min at 72°C. The PCR products were checked on a 1.2% (wt/v) agarose gel with gel electrophoresis for 40 min at 90 V. Gels were stained with GelRed™ and visualized on a UV transilluminator (UGenius 3, Syngene, UK).
DGGE using a TV-400 DGGE system (Scie-Plas, UK) was performed on the PCR amplified products. PCR product was loaded onto a 6% (wt/vol) polyacrylamide gel with a denaturant gradient of 30–70% (100% corresponds to 7 M urea and 40% vol/vol formamide). Electrophoresis was performed in 1 × TAE buffer at 60°C for 16 h at 100 V. The gel was stained with GelRed™ for 60 min and visualized on a UV transilluminator. All three incubation replicates for each time point were analyzed by DGGE to ensure reproducibility but a single replicate for each treatment was loaded on a single DGGE gel to evaluate shifts over time between all treatments. DGGE bands that potentially represented different strains were excised with a sterile scalpel and transferred to sterile 1.5 ml micro-centrifuge tubes containing 50 μl sterile molecular water. DNA was eluted from bands over 48 h at 4°C. Eluted DNA was re-amplified using the same primer pair (without GC clamp) and PCR programme. PCR products were then purified (E.Z.N.A cycle purification kit according to manufacturer's instructions; Omega) and quantified using a Genova Nano spectrophotometer (Jenway). Purified DNA was then diluted to a concentration of 6 ng μl−1 using sterile PCR water and sequenced by paired-end Sanger sequencing (Source Bioscience, Bellshill, Scotland). Sequences were quality checked using SeqTrace (Stucky, 2012) and run through the Basic Local Alignment Search Tool (BLASTn) for nucleotide closest match.
Next-Generation Sequencing
Paired-end (2 × 300 bp) Illumina MiSeq sequencing of the 16S rRNA gene V3–V4 variable region was performed on the upper sediment layer as previously described (Ferguson et al., 2017). Average read depth was 39,838 ± 2,206 (SEM) per sample for 39 samples, except for 3 samples (<10,000) which were omitted from downstream analysis. Bioinformatics analysis was carried out on the Maxwell High Performance Computing Cluster at the University of Aberdeen, using Mothur v 1.39.0. Chimera detection was performed using UCHIME (Edgar et al., 2011). OTU clustering was performed at 97% similarity and taxonomic assignment obtained with SILVA (Quast et al., 2012). The raw sequencing data is available in the European Nucleotide Archive (ENA) under the accession number PRJEB25813.
Statistical Analysis and Calculations
Statistical analysis was carried out using the package nlme (Pinheiro et al., 2017) within R environment (R Core Team, 2017). Preliminary data exploration was undertaken to establish the best approach to analyse the sediment profile data. To model the entrainment of hydrocarbons in the sediments, linear mixed effects models were used due to the correlation of hydrocarbon concentrations at each depth interval within individual cores. [Hydrocarbon] was used as the response variable (x0.4-transformed to ensure normality of residuals), core identity was specified as a random effect and hydrocarbon identity (HCID) was nested within core identity to allow for variation in total [hydrocarbon] content between cores and variation in average [hydrocarbon] between hydrocarbons within cores. An autoregressive residual correlation term (AR1) was included in the model, such that the concentration of a hydrocarbon was allowed to depend on the concentration of the same hydrocarbon directly above it in each core. Treatment, HCID, depth and time (both as factors) were included as fixed effects in the model and all possible interactions between them. Model selection was performed by stepwise elimination of non-significant terms (from higher to lower order terms) using the likelihood ratio test and maximum likelihood estimation. Once a minimal adequate fixed effect model structure was determined, it was refitted using restricted maximum likelihood estimation (Zuur et al., 2009). Separate models were fitted for undisturbed and modified sediment incubations.
To evaluate the partitioning of hydrocarbons between sediment and water phases, a distribution coefficient was developed that describes the ratio of total hydrocarbon mass in supernatant water to total hydrocarbon mass in sediment. This is not a true partition coefficient as this typically refers to distribution between two immiscible liquids. It is a distribution coefficient due to the presence of dissolved hydrocarbons in interstitial water but in this work, the sediment “phase” encapsulates the hydrocarbons dissolved and dispersed in the interstitial water as well as those adsorbed to and absorbed into sediment particles, while the supernatant “phase” encapsulates the hydrocarbons dissolved and dispersed in the water column. The distribution coefficient was defined as:
Where mw is the total hydrocarbon mass in supernatant phase and ms the total hydrocarbon mass in the sediment phase. Where no hydrocarbon was detected in either phase, the minimum detected concentration of that hydrocarbon in that phase was used instead of zero. Kws values below and above zero indicate preferential partitioning to the sediment and the supernatant water phase, respectively. The effect of SD25 application and time was evaluated using a linear mixed effects model following the same procedure as for the sediment transport models above with the exception that no autoregressive correlation was implemented into the model because [hydrocarbon] in one core did not depend on [hydrocarbon] in other cores. Separate models were developed for undisturbed and modified sediment incubations. Underlying model assumptions were validated graphically for hydrocarbon transport and Kws models (Supplementary Figures 3–6).
Effective diffusivities (Deff) for model oil compounds were calculated following Thibodeau and Mackay (2011) assuming the sediments were porous and saturated. Briefly, Deff is calculated as a ratio of aqueous diffusivity divided by physical and chemical resistance terms which take into account sediment properties and organic matter content.
Based on the analysis of DGGE images using Phoretix 1D analysis software (version 4.0; TotalLab Ltd), bacterial community analysis was represented by the relative band intensities within lanes as previously performed (McCaig et al., 2001). The statistical analysis of microbial communities was performed using the package vegan (Oksanen et al., 2017) in R. A distance matrix was generated using the Bray-Curtis method from the relative band intensity data (function vegdist) and the treatment effects on the community structure were visualized by non-parametric multi-dimensional scaling (nMDS) (function metaMDS). Hierarchical clustering (function hclust) was performed using the unweighted pair group method with arithmetic mean (UPGMA). Statistical differences between bacterial communities between treatments and times were analyzed using permutational multivariate analysis of variance (PerMANOVA) (function adonis; 999 restarts).
The operational taxonomic unit (OTU) table resulting from the Illumina sequencing analysis was imported into R with the package phyloseq (function import_biom) (Mcmurdie et al., 2013). Singletons from the whole database were removed and samples rarefied to the smallest sample read depth (function rarefy_even_depth). Plots representing the bacterial structure were visualized using the ggplot2 package (Wickham and Chang, 2009). Alpha diversity analysis was performed in package phyloseq (function estimate_richness). To assess differences in alpha diversity over time and between treatments, analysis of variance was performed where diversity estimates was the response variable and the interaction between treatments and day were the explanatory variables after log transformation of the data. For beta diversity analysis, a distance matrix was produced (function vegdist) with the Jaccard index and community structure differences between samples were visualized by nMDS as described above. PerMANOVA was performed to detect significant differences in community composition. In order to identify the taxa whose changes of abundance between treatments are more significant, differential abundance testing (function deseq) was carried out on non-subsampled data as recommended by package developers in package DESeq2 (Love et al., 2014).
Results
Sediment Properties and Hydrocarbon Effective Diffusivities
The sediment from station A was silt dominated (81–82%) and contained relatively low quantities of carbon (1.7–1.9% TOC and 0.9% TIC) (Supplementary Table 2). The BTEX components and naphthalene had the highest effective diffusivities (Deff; > 29 cm2 year−1) (Supplementary Table 1). For aliphatics, Deff decreased with increasing carbon chain length. Similarly, for PAHs, as the number of rings increased, Deff decreased (Supplementary Table 1).
Transport Into Sediments
In undisturbed sediments, hydrocarbon entrainment varied with HCID over time (Supplementary Figures 7–9, Supplementary Table 4, Supplementary Data 1), interaction: HCID × Depth (factor) × Time (factor): LRdf = 271 = 240.74, P = 0.0001). SD25 application significantly affected hydrocarbon entrainment over time (Figures 1, 2, Supplementary Figures 7–9, Supplementary Table 4, Supplementary Data 1, Treatment × Depth (factor) × Time (factor), LRdf = 9 = 162.9, P < 0.0001). Hydrocarbons were detected over 4 cm deep on day 42 for both treatments (Figures 1, 2, Supplementary Figures 7–9, Supplementary Data 1, [TPH]O−Treatment = 73.1 ± 96.0 μg g−1, [TPH]OD−Treatment = 124.0 ± 161.9 μg g−1, error = standard deviation) but not in the OD-treatment on day 71 ([TPH]O−Treatment = 22.3 ± 22.2 μg g−1, error = standard deviation).
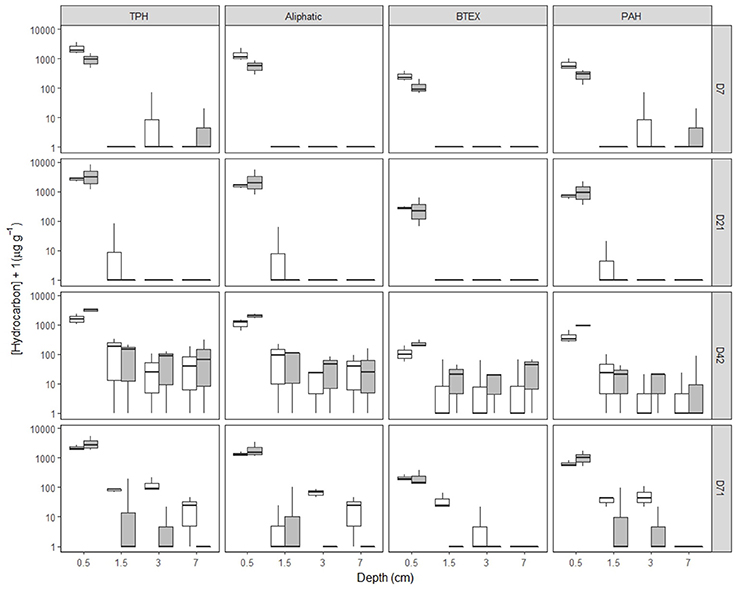
Figure 1. [Hydrocarbon] of hydrocarbon fractions by time, depth and treatment in Faroe-Shetland Channel undisturbed sediments. From top to bottom, days 7, 21, 42, and 71 and left to right total petroleum hydrocarbons (TPH), aliphatic, BTEX and polyaromatic hydrocarbons (PAH) fractions. Box plots represent [hydrocarbon] for oil (white) and oil and dispersant (gray) treatments (n = 3). Depths shown are the average depth of the sections analysis (i.e. 0–1, 1–2, 2–4, and 4–10 cm correspond to 0.5, 1.5, 3, and 7 cm deep, respectively). Note [hydrocarbon] has been log(x+1) scaled.
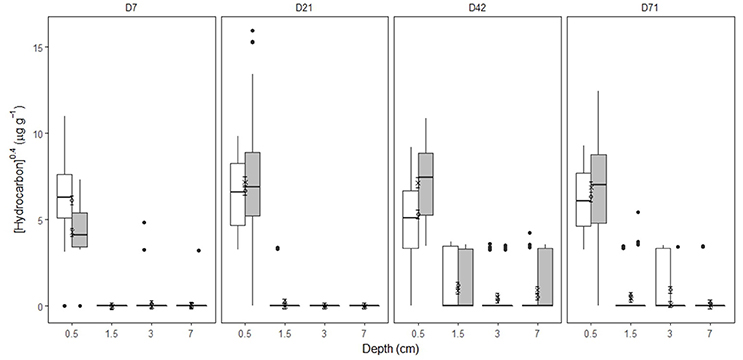
Figure 2. [Hydrocarbon] by time, depth and treatment in Faroe-Shetland Channel undisturbed sediments. From left to right, days 7, 21, 42, and 71. Box plots represent [hydrocarbon] for oil (white) and oil and dispersant (gray) treatments (n = 60). Linear mixed effects predictions are shown for oil (circles) and oil and dispersant (crosses) treatments. Error bars represent standard error. Depths shown are the average depth of the sections analysis (i.e. 0–1, 1–2, 2–4, and 4–10 cm correspond to 0.5, 1.5, 3, and 7 cm deep, respectively). Note [hydrocarbon] has been x0.4- transformed.
In modified sediments, hydrocarbons from all fractions were detected over 4 cm depth in the sediment on day 7 (Supplementary Figures 10–13). As in undisturbed sediments, SD25 application significantly affected [hydrocarbon] by time and depth in modified sediments (Supplementary Figures 10–14, Supplementary Table 3, Supplementary Data 2, Interaction Treatment × Time (factor) × Depth (factor), LRdf = 9 = 167.21, P < 0.0001). However, during model simplification, [hydrocarbon] across hydrocarbons over time was found to be non-significant (Interaction HCID × Treatment × Time (factor): LRdf = 57 = 74.31, P = 0.0615). In contrast to undisturbed sediments, there was no evidence that hydrocarbon entrainment varied across hydrocarbons over time (Interaction HCID × Time (factor) × Depth (factor): LRdf = 171 = 92.59, P = 1) or for the effect of SD25 on hydrocarbon entrainment to vary with hydrocarbon (Interaction HCID × Treatment × Depth (factor): LRdf = 57 = 39.73, P = 0.9603). The two-way interactions of HCID with treatment and time were also found to be not significant in modified sediments (HCID × Treatment: LRdf = 57 = 25.68, P = 0.1392 and HCID × Time (factor): LRdf = 57: 70.25, P = 0.1117).
Water-Sediment Distribution Coefficients (Kws)
In undisturbed sediments, there was no evidence of Kws values changing over time (LR = 4.06, d.f.3, P = 0.2555). However, SD25 application significantly affected Kws values differently between hydrocarbons (Figure 3, Supplementary Table 5, Supplementary Data 3, interaction HCID × Treatment: LRdf = 19 = 80.12, P < 0.0001). SD25 application increased Kws values of all hydrocarbons except BTEX components and naphthalene. In modified sediments, the effect of SD25 application varied with hydrocarbon and time (Supplementary Figure 15, Supplementary Table 6, Supplementary Data 4, Interaction HCID × Time × Treatment, LRdf = 19 = 101.63, P = 0.0001). For the O-treatment, Kws increased in day 21 and decreased thereafter. For the OD-treatment, Kws was lower than in the O-treatment for most components for day 7 but increased in day 21, remained higher than for the O-treatment in day 42 and decreased in day 71 (Supplementary Figure 15).
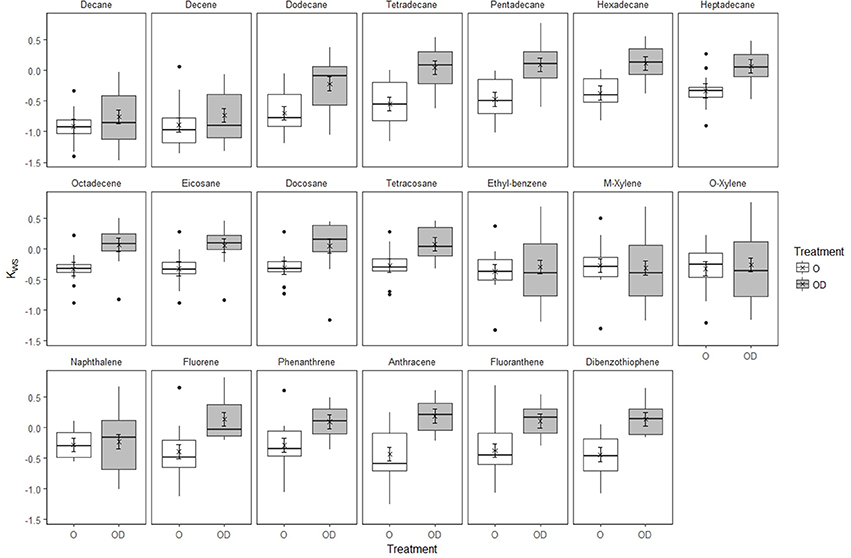
Figure 3. Effect of Superdispersant-25 on Kws by hydrocarbon in undisturbed Faroe-Shetland Channel sediments. Box plots represent the raw data for oil (white) and oil and dispersant (gray) treatments (n = 12). Linear mixed effects model predictions are shown for the oil (circles) and oil and dispersant (crosses) treatments. Error bars represent standard error.
Depth Profile of Bacterial Community Structure in Hydrocarbon and Superdispersant-25 Treated Sediments
According to DGGE analysis the bacterial community structure in the upper layer (0–1 cm) of the controls remained relatively unchanged over time (Supplementary Figures 16A,B). Bacterial communities from layer 1 controls days 0–21 and day 0 O-treatment were grouped together by cluster analysis (Supplementary Figure 16C). Ordination analysis of DGGE patterns of the top layer revealed differences in bacterial community structure between C- and both O- and OD-treatments (Supplementary Figure 16B). The presence of oil (O- and OD-treatments) significantly changed community composition compared to controls (PerMANOVA; R2 = 0.46, P = 0.0009). There were successional shifts in band patterns of O- and OD-treatments from day 7 to 71. The bacterial structure on day 42 in the OD-treatment was similar to O-treatment on day 71. However, this structure had changed in the parallel OD-treatment, indicating similar trajectories but at significantly different rates (PerMANOVA; R2 = 0.93, P = 0.0009).
Bacterial community structure of the control in the upper layer was similar to both C- and O-treatments in the deeper layers (Supplementary Figure 17A). The composition in these treatments was dominated by the presence of a core group of organisms (hereinafter referred to as “core group”). The core group was consistently present regardless of time or treatment. However, within the top layer of O-treatment incubations where [hydrocarbon] was highest, bands decreased in density and abundance in days 21–71. Moreover, there was a shift in bacterial structure at 1–2 cm deep in the O-treatment at days 42–71 (when hydrocarbons had migrated into the sediment) that clustered together with 0–1 cm deep O-treatment days 21–71 (Supplementary Figures 17B,C). Below 2 cm, there was dominance of the core group with no noticeable shifts. There was a significant difference between bacterial communities as a function of depth across both treatments (PerMANOVA; R2 = 0.48, P = 0.0009). Differences between C- and O-treatment communities were only identified within 0–1 cm deep (PerMANOVA; R2 = 0.59, P = 0.0009).
To identify the bacterial members of communities DGGE bands were excised and sequenced. All bands were classified as members of Proteobacteria, except band A, which was part of the Firmicutes phylum (Supplementary Table 7). Bands classified as Proteobacteria were characterized as γ-Proteobacteria, except bands K and L which were categorized as δ-Proteobacteria. Bands B and C showed similar homology to Colwellia rossensis strain ANT9279 (100% identity) and Colwellia psychrerythraea strain 34H (99%), respectively. Colwellia related strains were most prominent in layer 1 in O-treatment incubations but also present in other treatments and depths at a lower density. Bands H and J showed high sequence homology to Pseudoalteromonas sp. K8 (99%) and Pseudoalteromonas translucida KMM 520 (99%), respectively. Pseudoalteromonas related strains appeared to be stimulated by oil exposure within 0–1 cm, particularly band J which was only present on day 71. The core group was mainly represented by bands D to I. Band D diminished in the presence of oil whereas band G was more prominent in the O-treatment; both matched to uncultured γ-Proteobacteria. Band F initially increased in density from days 0–21 but then decreased from days 42–71 showing potential succession to Pseudoalteromonas spp.
Bacterial Community Structure at the Sediment/Water Interface
Illumina sequencing of the top centimeter of sediment was performed to examine the O- and OD-treatments induced bacterial shifts in more detail. The relative abundance of taxa within the bacterial communities was consistent among replicates early in the study but became increasingly divergent over time (Figures 4, 5). Bacterial communities from all treatments were dominated by Proteobacteria across all time points (46–66%) except for OD-treatment day 71 where composition was shared between Proteobacteria (41%) and Firmicutes (28%). Firmicutes was represented in the other two treatments but only with maximum contribution of 9% within the controls and 17% in the O-treatment. The phylum Parcubacteria represented < 1% at all time points and treatments except O-treatment day 71 where it averaged 5%. The phylum Actinobacteria was found at consistent levels throughout all time points within controls. Within Proteobacteria, the majority of organisms were assigned to the orders Alteromonadales and Xanthomonadales.
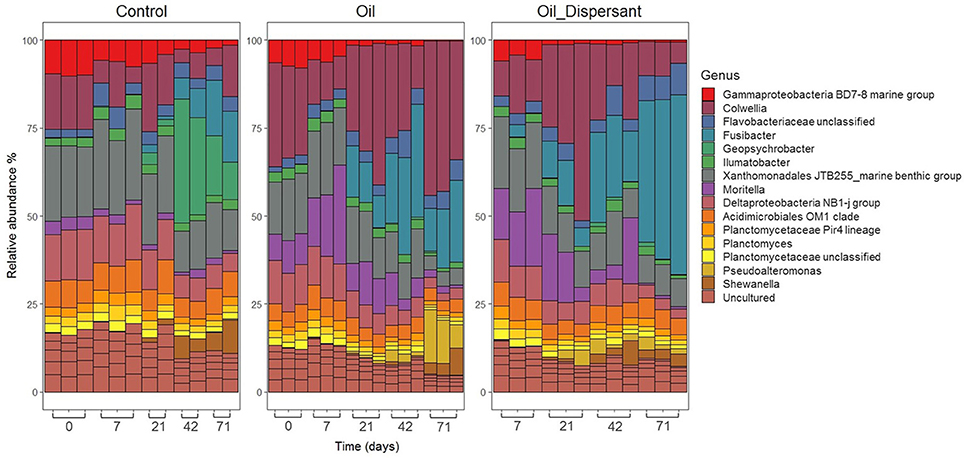
Figure 4. Relative abundance plots visualizing bacterial community composition and dynamics within the top centimeter of Faroe-Shetland Channel sediment over time. Time in days is displayed left to right along the x axis. Treatment is grouped as control (left box), oil (middle) and oil and dispersant (right). Bars represent the top 20 OTUs for each sample grouped at the genus level (clustered at 97%).
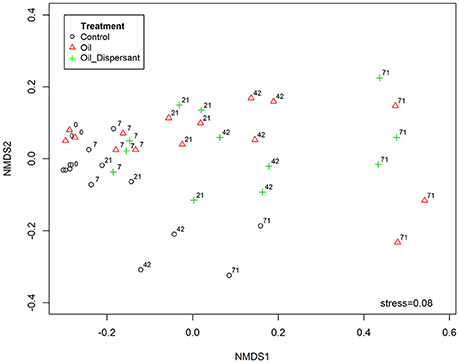
Figure 5. Effect of treatment and time on bacterial community composition within the top centimeter of Faroe-Shetland Channel sediment, assessed using non-metric multidimensional scaling (nMDS), based on Jaccard index following square root transformation of sample data. Treatments are indicated as control (black circles), oil (red triangles) and oil and dispersant (green crosses). Numbers placed next to treatments indicate time points in days.
The presence of oil selected for Alteromonadales, which averaged 14% in day 0 of both C- and O-treatments. By day 71 this reduced to 10% in controls but increased to 39% by day 71 in the O-treatment. In contrast, in the OD-treatment it peaked at 32% on day 21 but decreased to 8% by day 71 indicating succession to other organisms. At the genus level, Colwellia represented the most prominent OTU in both O- and OD-treatments on day 21 (Figure 4). It then decreased in the OD-treatment but increased to 27% in O-treatment. Moritella was present at 3% on day 0 in all treatments. However, by day 7 it had risen to 10% in both O and OD-treatments before decreasing at similar rates down to 1% by day 71. Pseudoalteromonas, Pseudomonas and Oleispira were present in oiled treatments at a lower abundance (1–10%). Oil selected for Candidatus Campbellbacteria, the predominant order within Parcubacteria, which was not present above 0.05% in any treatment other than O-treatment day 71 where it had increased to 6% in two of the three replicates. Application of dispersant resulted in increased abundance of the order Clostridiales, with relative abundance of 1% at day 7 before increasing to 29% at day 71 in the OD-treatment (Supplementary Figure 18). The most prominent member in the OD-treatment by day 71 was Fusibacter (27%), which was also present in O-treatment (11%).
Oil exposure had negative effects on Xanthomonadales which was consistently present in controls but decreased in relative abundance from 10% in both oiled treatments at day 7, to 5% by day 71 (Supplementary Figure 18). Genera which markedly increased in relative abundance in controls include Geopsychrobacter which was < 0.5% at day 0 yet increased to 18% by day 42 and Desulfuromonadales which increased in relative abundance from day 42 onwards in controls. A group of taxa were seen to withstand oil contamination such as Acidimicrobiales OM1_clade and Planctomycetaceae Pir4_lineage and may represent the aforementioned core group (Figure 4).
Statistical Analysis of Illumina Sequenced Bacterial Communities
Ordination analysis revealed dissimilarity of oil treated (both O- and OD-) communities with controls (Figure 5). The effect of treatment significantly explained variation in community structure (PerMANOVA; R2 = 0.16, P = 0.001). There was clustering of day 71 incubations of O- and OD-treatments away from earlier time points. Furthermore, there appeared to be gradual community divergence over time within replicates across all treatments. The overall effect of time was significant in community composition (PerMANOVA; R2 = 0.38, P = 0.001). The richness (observed OTUs) and diversity (Shannon index, Supplementary Figure 19) of the microbial communities across all treatments significantly decreased with time (ANOVA; F = 196.053, P = 0.001; F = 60.586, P = 0.001, respectively). When considering the interacting effects of treatment and time there were significant variations in community richness and diversity (ANOVA; F = 3.776, P = 0.006; F = 5.124, P = 0.001 respectively).
Differential abundance testing using DESeq2 determined which taxa were significantly more abundant between treatments (Figure 6). Pseudoaltermonas, Oleispira, Moritella, Candidatus Campbellbacteria, Pseudomonas, Colwellia and Fusibacter were more significantly abundant (all adjusted p < 0.001) in O- and OD-treatments. Members of the genera Geopsychrobacter and Desulfuromonas were more significantly (adjusted p < 0.001) abundant in controls.
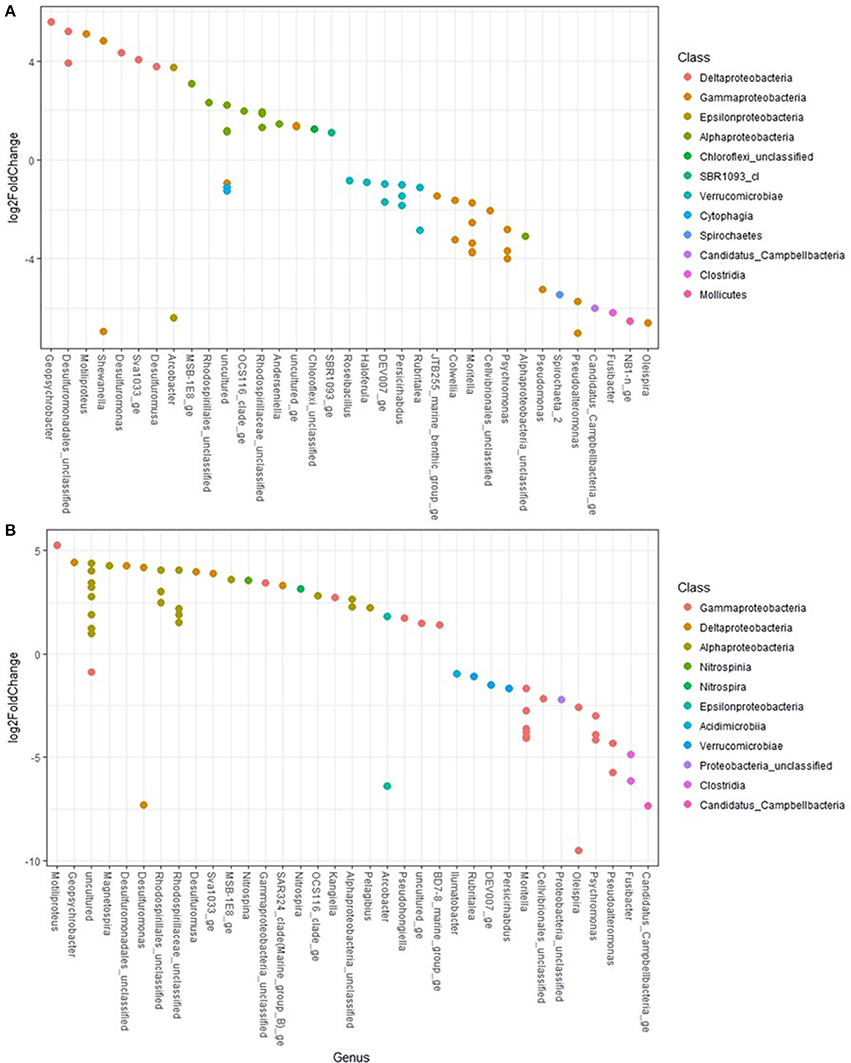
Figure 6. Differential abundance testing of taxa between treatments using DESeq2 found in (A) control (+log2FoldChange) and oil treatments (-log2FoldChange) and (B) control (+) and oil and dispersant treatments (−).
Discussion
The experimental design was unique in the way that it was developed to replicate a scenario following an oil spill in the deep sea where oil residues would be deposited to the seabed. Natural sediment structure was carefully maintained within cores and kept under in situ temperatures, an important factor in understanding and comparing contaminated sediments as was recently stressed (Acosta-González and Marqués, 2016). The use of OSPs enabled retention of oil within the top centimeter of sediment and aimed at replicating heavy oiling of sediments. This likely resulted in the formation of anoxic micro-niches as witnessed at previous spill sites (Yang et al., 2016) and represents a realistic microcosm. SD25 use aimed to simulate continuous application of dispersant at the wellhead as may be performed in a future oil spill response. To replicate appropriate field conditions, whole-core incubations were run and subjected to seawater flushing to ensure an aerobic water column and sediment-water interface, and replenishment of natural seawater nutrients. Flushing would have resulted in mobilization of oil from the sediment and removal of oil from the system, effectively diluting the oil concentration within the water column over time as expected in situ.
Hydrocarbon Transport Into Sediments
Hydrocarbons from all fractions were detected below 4 cm deep from day 7 to day 71 suggesting that the hydrocarbons in the model oil have the capability of entraining undisturbed FSC sediments relatively rapidly (Figures 1, 2, Supplementary Figures 7–9). Limited transport of C20–24 aliphatics and higher ring-number PAHs can be attributed to two key factors: (1) interactions with the minerals and organic matter in the upper centimeters of sediment (Stoffyn-Egli and Lee, 2002) and (2) continuous water replacement of supernatant water promoting the partition to the water column over intra-sediment transport. However, on day 71 C10 aliphatics were not detected below 2 cm in either treatment and C12+ aliphatics were not detected over 3 cm in the OD-treatment suggesting that these hydrocarbons may have been biodegraded in deep FSC sediments within 71 days. Metagenomic profiling of sub-surface sediments at a depth of 1.5–3 cm within 3 km of the well following the DwH spill revealed the dominance of known hydrocarbon degraders from the class δ-Proteobacteria (Kimes et al., 2013). The effect of SD25 was not found to be significant for individual hydrocarbons, it was significant for the Treatment × Depth × Time interaction (Figures 1, 2, Supplementary Figures 7–9). Therefore, the influence of SD25 on post-depositional transport of hydrocarbons in this work was unclear. C22–24 aliphatics were not detected below 2 cm deep at any time point suggesting limited transport of longer chained aliphatics. This is in agreement with the corresponding calculated Deff, which are the lowest of the model oil components (12.8–12.9 cm year−1). As found for aliphatics, BTEX and PAHs were also more abundant in day 42 than in day 71 over 1 cm deep (Supplementary Figures 7–9) and may have been degraded during the experiment. There is evidence for local 1000 m deep sediment microbial communities degrading these hydrocarbons (Ferguson et al., 2017). This is further supported by findings of microbial shifts at 1–2 cm from day 42 (Supplementary Figure 17). The entrainment of hydrocarbons over 4 cm deep into FSC sediments is of significance because it will encourage the consumption of oxygen by aerobic microbes and deplete available oxygen in the sediment rendering it anoxic. This suggests that hydrocarbon biodegradation beyond this will be limited by oxygen availability and dominated by anaerobic microbial communities (Widdel et al., 2010). There was no detected shift in the microbial community structure below 2 cm deep suggesting slower rates of metabolism than in surficial sediments. The half-life of PAHs in the GoM has been shown to be twice as long in sediments over 1000 m deep than at 100–150 m deep (Tansel et al., 2011). Due to the cold deep water temperatures in the FSC, PAH residence times are expected to be higher than in the GoM. Discussion on hydrocarbon transport in modified sediments can be found in the Supplementary discussion.
In undisturbed sediments, the application of SD25 increased Kws values of most hydrocarbons with the exception of BTEX and naphthalene, the most water-soluble components of the model oil, but the interaction was not significant over time (Figure 3, Supplementary 10–12). In contrast, the effect of SD25 application in modified sediments changed significantly over time and followed a similar trend for most hydrocarbons revealing a time lag in Kws which was consistent across hydrocarbons (Supplementary Figure 15). Kws values increased from day 7 to day 21 to equilibrate with the supernatant water phase in both treatments. In 42-day incubations, Kws values decreased for the O- but not for the OD-treatment. The decrease in Kws in the O-treatment can be explained by desorption hysteresis, whereby hydrocarbons adsorbed onto fine particles and organic matter can remain adsorbed despite experiencing conditions which would favor partition to the water column (Gong et al., 2014b). The sustained Kws values in the OD-treatment suggest that SD25 application facilitates the partitioning of hydrocarbons to the water column and reduces the impact of hysteresis. This contradicts the findings of Zhao et al. (2015) where they show that PAH uptake increases with SD25 application. In their experimental setup the [hydrocarbon] used are much lower than those used here (0–6000 mg l−1 for naphthalene and 1-methylnaphthalene, 0–280 mg l−1 for pyrene) suggesting that this effect is only prevalent at low [hydrocarbon].
Bacterial Community Response Following Hydrocarbon Entrainment
Diverse microbial communities inhabit deep sea sediments and their structure and diversity is dependent upon environmental conditions such as depth and organic carbon content (Biddle et al., 2011). These microbes are believed to make up a “seed bank” of taxa which are niche-dependent (Gibbons et al., 2013). Certain bacterial taxa capable of utilizing hydrocarbons are present within the seed bank in very small numbers and bloom once provided with their preferred carbon source (Syutsubo et al., 2001; Head et al., 2006). Differential abundance testing identified certain taxa that were undetectable in controls but were able to respond to oil exposure. Shifts in relative abundance resulted in a modification to the richness, evenness and subsequently diversity of microbial communities. A significant reduction in diversity of oil contaminated samples compared to control samples over time in this study agrees with previous work (Hazen et al., 2010; Baelum et al., 2012; Dubinsky et al., 2013). Yet, the continued presence of a “core” group revealed by both molecular analyses suggests tolerance of selected sediment bacterial communities. This could be caused by the incubation design, whereby the environment/sediment provided a buffering effect, mitigating the impact of oil as opposed to intrusive incubation methods that more readily expose communities to toxic fractions of oil such as slurries and liquid incubations.
Following the sedimentation pulse during DwH, metagenomic analysis of surficial sediments revealed OTU dominance of uncultured γ-Proteobacteria and Colwellia spp. (Mason et al., 2014b). Colwellia was identified here as most responsive to hydrocarbon exposure in oiled treatments. Colwellia is believed to play an active role in hydrocarbon degradation throughout the oceans (Valentine et al., 2010; Redmond and Valentine, 2012; Mason et al., 2014a) and within sediments (Mason et al., 2014b). Strains identified in this study had similarity of 100% to C. rossensis strain ANT9279 isolated from Arctic sea ice (Brinkmeyer et al., 2003) and 99% to C. psychrerythraea isolated from Arctic sea sediment, confirming its ability to function in cold environments. Both of these strains were matched to clones from a library constructed from cold DwH plume waters and linked to short chain alkane degradation (Valentine et al., 2010) and PAH degradation in surface slick and plume samples (Gutierrez et al., 2013). Single cell genomic analysis of a Colwellia strain from the DwH hydrocarbon plume (matching 84% to C. psychrerythraea 34H) revealed the organism has genes for denitrification, adaptations to cold environments, nutrient acquisition and hydrocarbon degradation (Mason et al., 2014a). The presence of denitrification genes suggest anaerobic respiration capability, which would allow growth in areas of oxygen depletion as witnessed within the DwH plume (Joye et al., 2011; Kessler et al., 2011) and in anoxic sediments (Yang et al., 2016). It is plausible to find both aerobic and anaerobic organisms in the same location; Yang et al. (2016) identified both aerobic α-Proteobacteria and anaerobic δ-Proteobacteria within the same site surrounding the Macondo well. This is concurrent with evidence in this study where from days 21 in both O- and OD-treatments over 50% of dominant taxa were a combination of Colwellia and Fusibacter genera which are capable of anaerobic respiration. Yang et al. (2016) showed this may be the result of heterogeneous redox conditions forming within the sediment due to smothering by oil residues and the development of anoxic micro-niches. Fusibacter are generally halotolerant fermentative anaerobes capable of reducing a range of sulfur species and often isolated at mesophilic temperatures (Serrano et al., 2017). Several strains have been isolated from hydrocarbon related environments including the type strain Fusibacter paucivorans isolated from an oil-producing well (Ravot et al., 1999) and Fusibacter bizertensis isolated from a corroded kerosene storage tank (Smii et al., 2015). However, this genus is yet to be directly linked to PAH degradation (Kappell et al., 2014) and the ability of Firmicutes to form endospores may infer an ability to survive when other organisms cannot.
Numerous known hydrocarbon degraders were detected in this study including Pseudoalteromonas, Oleispira, Pseudomonas and Moritella. Pseudoalteromonas is regularly found in oil polluted environments such as surface oil slicks (Yang et al., 2014), the water column (Chakraborty et al., 2012; Gutierrez et al., 2013; Chronopoulou et al., 2014), deep sea sediments (Yang et al., 2016) and coastal ecosystems (Kostka et al., 2011; Kappell et al., 2014). Pseudoalteromonas is commonly linked to PAH degradation and can thrive once aliphatic resources are low or depleted (McKew et al., 2007; Dubinsky et al., 2013) and increased in relative abundance by day 71 in O-treated incubations. Oleispira was significantly more abundant in O-treatment incubations in this study and is active in cold environments (Yakimov et al., 2003; Kube et al., 2013; Gentile et al., 2016). Pseudomonas can degrade various structured hydrocarbons from cold environments (Bacosa et al., 2010; Viggor et al., 2013). Relative abundance data suggests Pseudomonas phylotypes were most prominent toward the end of the incubation period. However, in a simulation study of the DwH spill Pseudomonas were most abundant at 6 days of incubation with crude oil (Hu et al., 2017). Moritella is also capable of hydrocarbon degradation (Bagi et al., 2014) and was dominant by day 7 but then decreased in relative abundance over time. In addition, several Moritella species have been classified as piezophiles (Yanagibayashi et al., 1999; Xu, 2003) as has Colwellia (Oger and Jebbar, 2010) and these physiological traits reflect their sampling environment. Most interesting was the enrichment of Candidatus Campbellbacteria which was undetectable (< 0.05% relative abundance) in controls but rose to 6% by day 71 in the O-treatment. Candidatus Campbellbacteria belongs to Parcubacteria and has been detected in marine sediments and anoxic environments including the Mariana Trench (León-Zayas et al., 2017). Although never cultured, it has been detected in hydrocarbon polluted environments (Salam et al., 2017).
Effects of Dispersant Application on Bacterial Community Structure
Commercial dispersants have long been used in oil spill response efforts and there are a wide variety of US EPA approved dispersants on the market (Kleindienst et al., 2015a) most notably Corexit 9500 and 9527, both used in the DwH clean-up strategy (Seidel et al., 2015). In the UK, one of the approved and most readily available dispersants is SD25 which was found to be less toxic than Corexit varieties (Scarlett et al., 2005). Therefore, it was used in this study as an oil spill remediation treatment to assess the effect on hydrocarbon transport in sediments and bacterial community composition.
The application of SD25 had a significant effect on microbial community composition and selected for a different range of taxa, although there were similarities between O- and OD-treatments. Fusibacter was strongly selected for by SD25 application and this trend has never been witnessed before. The presence of an additional carbon-rich substrate may have allowed Fusibacter to thrive, outcompeting other taxa. Additionally, the increased solubilization of oil in seawater by SD25 may have allowed Fusibacter to utilize hydrocarbons faster than other taxa. Community shifts within the OD-treatment followed a similar pattern to the O-treatment; however, it appeared to occur faster. Moritella was initially selected for, followed by Colwellia, as was apparent in both O- and OD-treatments. Yet, in OD-treatment the decline of Colwellia occurred in day 42 and was succeeded by Fusibacter.
Dispersant has been reported to significantly increase hydrocarbon degradation and enrich the presence of hydrocarbon degrading bacteria (Baelum et al., 2012). SD25 was found to significantly enhance oil degradation rates by communities obtained from 1,000 m in the FSC however, there were no changes in community structure (Ferguson et al., 2017). Conversely, addition of dispersant has been shown to have limited, if any, impact on biodegradation (Foght and Westlake, 1982; Macías-Zamora et al., 2014) and in one case dispersant suppressed the activity of natural oil-degrading microorganisms (Kleindienst et al., 2015b). Thus, the notion that SD25 may have modified the bacterial response compared to oil only treatment may not necessarily mean that it enhanced biodegradation. Dispersants dissolve and disperse oil in water, leading to an increased oil droplet surface area and potential biodegradation stimulation (Kleindienst et al., 2015a). However, sediment particles present an increased surface area for oil adsorption and may reduce dispersant effectiveness (Macías-Zamora et al., 2014). It is often not possible to discern whether the microbial community shift is due to increased oil biodegradation or other influencing factors. Lindstrom and Braddock (2002) observed quicker mineralization of Corexit EC9500A than that of crude oil and dispersed oil implying that Corexit dispersant offered an alternative carbon source and was more selectively degraded. Whether this detracts from oil removal or positively “conditions” the microbial community for oil degradation remains to be answered.
Conclusion
This study assessed the transport of hydrocarbons and subsequent microbial response in FSC sediments following OSP deposition, in the presence and absence of SD25. This is the first study to replicate heavy oiling of naturally stratified deep sea sediments. Conditions were kept representative by maintaining in situ temperatures, replenishing seawater and continuously applying dispersant. A deep water oil spill in the FSC would likely have profound impacts on benthic ecosystems due to its unique oceanographic conditions (Bett, 2012). Biodegradation rates are reduced at low temperatures (Sharma and Schiewer, 2016; Ferguson et al., 2017). Additionally, low temperatures will enhance the extent of adsorption of hydrocarbons to sediments (Delle Site, 2001; Zhao et al., 2015). Adsorption of oil by sediment has been shown to promote biodegradation by capturing hydrocarbons (Yang et al., 2008). However, sequestration of hydrocarbons by sediments may also reduce their bioavailability in the long term through entrainment into organic matter and particle micropores that are inaccessible to microbes (Haritash and Kaushik, 2009).
The results of this study provide realistic data regarding hydrocarbon transport, mobilization and the ability of natural bacterial populations to respond and will aid in oil spill response decision-making in the UK. Key findings were:
(1) Deposited oil was transported to over 4 cm deep into FSC sediments following OSP deposition within the timeframe of the experiment, indicating that hydrocarbons can entrain deep into FSC sediments. Microbial shifts below 2 cm in the sediment were witnessed from 42 days onwards.
(2) The use of modified sediment matrix resulted in enhanced oil transport into FSC sediments with respect to undisturbed sediments. This highlights the importance of considering sediment handling and pre-treatment which may influence patterns of hydrocarbon transport.
(3) Deposition of hydrocarbons on surficial sediments forced bacterial communities to undergo significant shifts. The bacterial community response to oil exposure was related to members of the genera Colwellia, Fusibacter and Pseudoalteromonas. Microbial profiling demonstrated that shifts were primarily taking place within the top centimeter of sediment from day 7.
(4) SD25 application in undisturbed sediments increased the Kws values of all hydrocarbons except C10 aliphatics, BTEX components and naphthalene indicating that SD25 selectively enhances the water-solubility of oil components. In modified sediments, SD25 application had a similar effect for all hydrocarbons.
(5) SD25 application resulted in a significant change in the bacterial community structure with strong dominance of Fusibacter. Shifts at parallel time points suggest an accelerated and modified response at the presence of dispersant.
The results of this study provide insight into hydrocarbon transport and subsequent microbial community shifts in FSC deep sediments following OSP deposition and the role of SD25 on these processes.
Author Contributions
LJP, LDP, EG, UW, and JA: conceived the study; LJP, LDP, and AG: collected the samples; LJP and LDP: conducted the experiments; LJP, LDP, and TC: designed the statistical analysis for hydrocarbon transport and LJP, LDP, and CG-R designed the microbial community analyses. LJP and LDP: wrote the manuscript with input from all authors.
Funding
LJP and hydrocarbon analytics were funded through MarCRF funds for a Ph.D. project designed by UW, JA, and AG and awarded to LJP. LDP and microbiological investigations were funded through NERC award no NE/L00982X/1 to UW, JA, and EG. CG-R is funded by a University Research Fellowship.
Conflict of Interest Statement
The authors declare that the research was conducted in the absence of any commercial or financial relationships that could be construed as a potential conflict of interest.
Acknowledgments
The authors acknowledge Dr. Alan McCue for assistance with GC-FID, the MRV Scotia scientists and crew for assistance with sample collection and Cruikshank Analytical Lab for Carbon content analysis. Amy Bode and Val Johnston are thanked for their assistance with experimental setup and sampling. Dr. Sophie Shaw (CGEBM) is acknowledged for her advice and guidance with molecular analysis.
Supplementary Material
The Supplementary Material for this article can be found online at: https://www.frontiersin.org/articles/10.3389/fmars.2018.00159/full#supplementary-material
References
Acosta-González, A., and Marqués, S. (2016). Bacterial diversity in oil-polluted marine coastal sediments. Curr. Opin. Biotechnol. 38, 24–32. doi: 10.1016/j.copbio.2015.12.010
Acosta-González, A., Martirani-von Abercron, S.-M., Rosselló-Móra, R., Wittich, R.-M., and Marqués, S. (2015). The effect of oil spills on the bacterial diversity and catabolic function in coastal sediments: a case study on the Prestige oil spill. Environ. Sci. Pollut. Res. 22, 15200–15214. doi: 10.1007/s11356-015-4458-y
Atlas, R. R. M. R., and Hazen, T. C. T. (2011). Oil biodegradation and bioremediation: a tale of the two worst spills in US history. Environ. Sci. Technol. 45, 6709–6715. doi: 10.1021/es2013227
Austin, J. A., Cannon, S. J. C., and Ellis, D. (2014). Hydrocarbon exploration and exploitation West of Shetlands. Geol. Soc. Lond. Spec. Publ. 397, 1–10. doi: 10.1144/SP397.13
Bacosa, H. P., Suto, K., and Inoue, C. (2010). Preferential utilization of petroleum oil hydrocarbon components by microbial consortia reflects degradation pattern in aliphatic–aromatic hydrocarbon binary mixtures. World J. Microbiol. Biotechnol. 27, 1109–1117. doi: 10.1007/s11274-010-0557-6
Baelum, J., Borglin, S., Chakraborty, R., Fortney, J. L., Lamendella, R., Mason, O. U., et al. (2012). Deep-sea bacteria enriched by oil and dispersant from the Deepwater horizon spill. Environ. Microbiol. 14, 2405–2416. doi: 10.1111/j.1462-2920.2012.02780.x
Bagi, A., Pampanin, D. M., Lanzén, A., Bilstad, T., and Kommedal, R. (2014). Naphthalene biodegradation in temperate and arctic marine microcosms. Biodegradation 25, 111–125. doi: 10.1007/s10532-013-9644-3
Berx, B., Hansen, B., Østerhus, S., Larsen, K. M., Sherwin, T., and Jochumsen, K. (2013). Combining in situ measurements and altimetry to estimate volume, heat and salt transport variability through the Faroe–Shetland Channel. Ocean Sci. 9, 639–654. doi: 10.5194/os-9-639-2013
Bett, B. J. (2012). Seafloor Biotope Analysis of the Deep Waters of the SEA4 Region of Scotland's Seas. JNCC Rep. No. 472.
Biddle, J. F., White, J. R., Teske, A. P., and House, C. H. (2011). Metagenomics of the subsurface Brazos-Trinity Basin (IODP site 1320): comparison with other sediment and pyrosequenced metagenomes. ISME J. 5, 1038–1047. doi: 10.1038/ismej.2010.199
Brinkmeyer, R., Knittel, K., Jürgens, J., Weyland, H., Amann, R., and Helmke, E. (2003). Diversity and structure of bacterial communities in Arctic versus Antarctic pack ice diversity and structure of bacterial communities in Arctic versus Antarctic pack ice. Appl. Environ. Microbiol. 69, 6610–6619. doi: 10.1128/AEM.69.11.6610-6619.2003
Brooks, G. R., Larson, R. A., Schwing, P. T., Romero, I., Moore, C., Reichart, G.-J., et al. (2015). Sedimentation pulse in the NE Gulf of Mexico following the 2010 DWH Blowout. PLoS ONE 10:e0132341. doi: 10.1371/journal.pone.0132341
Chakraborty, R., Borglin, S. E., Dubinsky, E. A., Andersen, G. L., and Hazen, T. C. (2012). Microbial response to the MC-252 oil and Corexit 9500 in the Gulf of Mexico. Front. Microbiol. 3:357. doi: 10.3389/fmicb.2012.00357
Chanton, J., Zhao, T., Rosenheim, B. E., Joye, S., Bosman, S., Brunner, C., et al. (2014). Using natural abundance radiocarbon to trace the Flux of petrocarbon to the sea floor following the deepwater horizon oil spill. Environ. Sci. Technol. 49, 847–854. doi: 10.1021/es5046524
Chronopoulou, P.-M., Sanni, G. O., Silas-Olu, D. I., van der Meer, J. R., Timmis, K. N., Brussaard, C. P. D., et al. (2014). Generalist hydrocarbon-degrading bacterial communities in the oil-polluted water column of the North Sea. Microb. Biotechnol. 8, 434–447. doi: 10.1111/1751-7915.12176
Cordes, E. E., Jones, D. O. B., Schlacher, T. A., Amon, D. J., Bernardino, A. F., Brooke, S., et al. (2016). Environmental impacts of the Deep-Water Oil and gas industry: a review to guide management strategies. Front. Environ. Sci. 4, 1–54. doi: 10.3389/fenvs.2016.00058
Delle Site, A. (2001). Factors affecting sorption of organic compounds in natural sorbent/water systems and sorption coefficients for selected pollutants. A Review. J. Phys. Chem. Ref. Data 30, 187–439. doi: 10.1063/1.1347984
Dubinsky, E. A., Conrad, M. E., Chakraborty, R., Bill, M., Borglin, S. E., Hollibaugh, J. T., et al. (2013). Succession of hydrocarbon-degrading bacteria in the aftermath of the deepwater horizon oil spill in the Gulf of Mexico. Environ. Sci. Technol. 47, 10860–10867. doi: 10.1021/es401676y
Edgar, R. C., Haas, B. J., Clemente, J. C., Quince, C., and Knight, R. (2011). UCHIME improves sensitivity and speed of chimera detection. Bioinformatics 27, 2194–2200. doi: 10.1093/bioinformatics/btr381
Ferguson, R. M. W., Gontikaki, E., Anderson, J. A., and Witte, U. (2017). The variable influence of dispersant on degradation of oil hydrocarbons in subarctic deep-sea sediments at low temperatures (0–5 °C). Sci. Rep. 7:2253. doi: 10.1038/s41598-017-02475-9
Foght, J. M., and Westlake, D. W. S. (1982). Effect of the dispersant Corexit 9527 on the microbial degradation of Prudhoe Bay oil. Can. J. Microbiol. 28, 117–122. doi: 10.1139/m82-012
Gallego, A., O'Hara Murray, R., Berx, B., Turrell, W. R., Beegle-Krause, C. J., Inall, M., et al. (2018). Current status of deepwater oil spill modelling in the Faroe-Shetland channel, Northeast Atlantic, and future challenges. Mar. Pollut. Bull. 127, 484–504. doi: 10.1016/j.marpolbul.2017.12.002
Gentile, G., Bonsignore, M., Santisi, S., Catalfamo, M., Giuliano, L., Genovese, L., et al. (2016). Biodegradation potentiality of psychrophilic bacterial strain Oleispira antarctica RB-8 T. Mar. Pollut. Bull. 105, 125–130. doi: 10.1016/j.marpolbul.2016.02.041
Gibbons, S. M., Caporaso, J. G., Pirrung, M., Field, D., Knight, R., and Gilbert, J. A. (2013). Evidence for a persistent microbial seed bank throughout the global ocean. Proc. Natl. Acad. Sci. U.S.A. 110, 4651–4655. doi: 10.1073/pnas.1217767110
Gong, Y., Zhao, X., Cai, Z., O'Reilly, S. E., Hao, X., and Zhao, D. (2014a). A review of oil, dispersed oil and sediment interactions in the aquatic environment: influence on the fate, transport and remediation of oil spills. Mar. Pollut. Bull. 79, 16–33. doi: 10.1016/j.marpolbul.2013.12.024
Gong, Y., Zhao, X., O'Reilly, S. E., Qian, T., and Zhao, D. (2014b). Effects of oil dispersant and oil on sorption and desorption of phenanthrene with Gulf Coast marine sediments. Environ. Pollut. 185, 240–249. doi: 10.1016/j.envpol.2013.10.031
Gontikaki, E., Mayor, D. J., Narayanaswamy, B. E., and Witte, U. (2011). Feeding strategies of deep-sea sub-Arctic macrofauna of the Faroe-Shetland Channel: combining natural stable isotopes and enrichment techniques. Deep Sea Res. I Oceanogr. Res. Pap. 58, 160–172. doi: 10.1016/j.dsr.2010.11.011
Gutierrez, T., Singleton, D. R., Berry, D., Yang, T., Aitken, M. D., and Teske, A. (2013). Hydrocarbon-degrading bacteria enriched by the Deepwater Horizon oil spill identified by cultivation and DNA-SIP. ISME J. 7, 2091–2104. doi: 10.1038/ismej.2013.98
Haritash, A. K., and Kaushik, C. P. (2009). Biodegradation aspects of polycyclic aromatic hydrocarbons (PAHs): a review. J. Hazard. Mater. 169, 1–15. doi: 10.1016/j.jhazmat.2009.03.137
Hastings, D. W., Schwing, P. T., Brooks, G. R., Larson, R. A., Morford, J. L., Roeder, T., et al. (2016). Changes in sediment redox conditions following the BP DWH blowout event. Deep Sea Res. II Top. Stud. Oceanogr. 129, 167–178. doi: 10.1016/j.dsr2.2014.12.009
Hazen, T. C., Dubinsky, E. A., DeSantis, T. Z., Andersen, G. L., Piceno, Y. M., Singh, N., et al. (2010). Deep-sea oil plume enriches indigenous oil-degrading bacteria. Science 330, 204–208. doi: 10.1126/science.1195979
Head, I. M., Jones, D. M., and Röling, W. F. M. (2006). Marine microorganisms make a meal of oil. Nat. Rev. Microbiol. 4, 173–182. doi: 10.1038/nrmicro1348
Hu, P., Dubinsky, E. A., Probst, A. J., Wang, J., Sieber, C. M. K., Tom, L. M., et al. (2017). Simulation of deepwater horizon oil plume reveals substrate specialization within a complex community of hydrocarbon degraders. Proc. Natl. Acad. Sci. U.S.A. 114, 7432–7437. doi: 10.1073/pnas.1703424114
Huettel, M., Berg, P., and Kostka, J. E. (2014). Benthic exchange and biogeochemical cycling in permeable sediments. Ann. Rev. Mar. Sci. 6, 23–51. doi: 10.1146/annurev-marine-051413-012706
Hylland, K. (2006). Polycyclic Aromatic Hydrocarbon (PAH) ecotoxicology in marine ecosystems. J. Toxicol. Environ. Heal. A 69, 109–123. doi: 10.1080/15287390500259327
Joint Nature Conservation Committee (2014). North-East Faroe-Shetland Channel MPA. Peterborough. Available online at: http://jncc.defra.gov.uk/page-6483
Jones, D. O. B., Bett, B. J., and Tyler, P. A. (2007). Megabenthic ecology of the deep Faroe–Shetland channel: a photographic study. Deep Sea Res. I Oceanogr. Res. Pap. 54, 1111–1128. doi: 10.1016/j.dsr.2007.04.001
Joye, S. B., Bracco, A., Özgökmen, T. M., Chanton, J. P., Grosell, M., MacDonald, I. R., et al. (2016). The Gulf of Mexico ecosystem, six years after the Macondo oil well blowout. Deep. Res. II Top. Stud. Oceanogr. 129, 4–19. doi: 10.1016/j.dsr2.2016.04.018
Joye, S. B., MacDonald, I. R., Leifer, I., and Asper, V. (2011). Magnitude and oxidation potential of hydrocarbon gases released from the BP oil well blowout. Nat. Geosci. 4, 160–164. doi: 10.1038/ngeo1067
Joye, S. B., Teske, A. P., and Kostka, J. E. (2014). Microbial dynamics following the macondo oil well blowout across Gulf of Mexico environments. Bioscience 64, 766–777. doi: 10.1093/biosci/biu121
Kappell, A. D., Wei, Y., Newton, R. J., van Nostrand, J. D., Zhou, J., McLellan, S. L., et al. (2014). The polycyclic aromatic hydrocarbon degradation potential of Gulf of Mexico native coastal microbial communities after the Deepwater Horizon oil spill. Front. Microbiol. 5:205. doi: 10.3389/fmicb.2014.00205
Kessler, J. D., Valentine, D. L., Redmond, M. C., Du, M., Chan, E. W., Mendes, S. D., et al. (2011). A persistent oxygen anomaly reveals the fate of spilled methane in the deep Gulf of Mexico. Science 331, 312–315. doi: 10.1126/science.1199697
Kimes, N. E., Callaghan, A. V., Aktas, D. F., Smith, W. L., Sunner, J., Golding, B., et al. (2013). Metagenomic analysis and metabolite profiling of deep-sea sediments from the Gulf of Mexico following the deepwater horizon oil spill. Front. Microbiol. 4:50. doi: 10.3389/fmicb.2013.00050
Kimes, N. E., Callaghan, A. V., Suflita, J. M., and Morris, P. J. (2014). Microbial transformation of the deepwater horizon oil spill-past, present, and future perspectives. Front. Microbiol. 5:603. doi: 10.3389/fmicb.2014.00603
Kleindienst, S., Paul, J. H., and Joye, S. B. (2015a). Using dispersants after oil spills: impacts on the composition and activity of microbial communities. Nat. Rev. Microbiol. 13, 388–396. doi: 10.1038/nrmicro3452
Kleindienst, S., Seidel, M., Ziervogel, K., Grim, S., Loftis, K., Harrison, S., et al. (2015b). Chemical dispersants can suppress the activity of natural oil-degrading microorganisms. Proc. Natl. Acad. Sci. U.S.A. 112, 14900–14905.
Konovalov, D., Renaud, P. E., Berge, J., Voronkov, A. Y., and Cochrane, S. K. J. (2010). Contaminants, benthic communities, and bioturbation: potential for PAH mobilisation in Arctic sediments. Chem. Ecol. 26, 197–208. doi: 10.1080/02757541003789058
Kostka, J. E., Prakash, O., Overholt, W., Green, S. J., Freyer, G., Canion, A., et al. (2011). Hydrocarbon-degrading bacteria and the bacterial community response in gulf of Mexico beach sands impacted by the deepwater horizon oil spill. Appl. Environ. Microbiol. 77, 7962–7974. doi: 10.1128/AEM.05402-11
Kube, M., Chernikova, T. N., Al-Ramahi, Y., Beloqui, A., Lopez-Cortez, N., Guazzaroni, M.-E., et al. (2013). Genome sequence and functional genomic analysis of the oil-degrading bacterium Oleispira antarctica. Nat. Commun. 4:2156. doi: 10.1038/ncomms3156
Leffler, W. L., Pattarozzi, R., and Sterling, G. (2011). Deepwater Petroleum Exploration & Production: A Nontechnical Guide, 2nd Edn. Tulsa, OK: PennWell Corp.
León-Zayas, R., Peoples, L., Biddle, J. F., Podell, S., Novotny, M., Cameron, J., et al. (2017). The metabolic potential of the single cell genomes obtained from the Challenger Deep, Mariana Trench within the candidate superphylum Parcubacteria (OD1). Environ. Microbiol. 19, 2769–2784. doi: 10.1111/1462-2920.13789
Lindstrom, J. E., and Braddock, J. F. (2002). Biodegradation of petroleum hydrocarbons at low temperature in the presence of the dispersant Corexit 9500. Mar. Pollut. Bull. 44, 739–747. doi: 10.1016/S0025-326X(02)00050-4
Love, M. I., Huber, W., and Anders, S. (2014). Moderated estimation of fold change and dispersion for RNA-seq data with DESeq2. Genome Biol. 15, 1–21. doi: 10.1186/s13059-014-0550-8
Lubchenco, J., McNutt, M., Lehr, B., Sogge, M., Miller, M., Hammond, S., et al. (2010). BP Deepwater Horizon Oil Budget: What Happened to the Oil?
Macías-Zamora, J. V., Meléndel-Sánchez, A. L., Ramírez-Álvarez, N., Gutiérrez-Galindo, E. A., and Orozco-Borbón, M. V. (2014). On the effects of the dispersant Corexit 9500© during the degradation process of n-alkanes and PAHs in marine sediments. Environ. Monit. Assess. 186, 1051–1061. doi: 10.1007/s10661-013-3438-2
Main, C. E., Yool, A., Holliday, N. P., Popova, E. E., Jones, D. O. B., and Ruhl, H. A. (2016). Simulating pathways of subsurface oil in the Faroe–Shetland Channel using an ocean general circulation model. Mar. Pollut. Bull. 114, 315–326. doi: 10.1016/j.marpolbul.2016.09.041
Marini, M., and Frapiccini, E. (2013). Persistence of polycyclic aromatic hydrocarbons in sediments in the deeper area of the Northern Adriatic Sea (Mediterranean Sea). Chemosphere 90, 1839–1846. doi: 10.1016/j.chemosphere.2012.09.080
Mason, O. U., Han, J., Woyke, T., and Jansson, J. K. (2014a). Single-cell genomics reveals features of a Colwellia species that was dominant during the Deepwater Horizon oil spill. Front. Microbiol. 5:332. doi: 10.3389/fmicb.2014.00332
Mason, O. U., Scott, N. M., Gonzalez, A., Robbins-Pianka, A., Bælum, J., Kimbrel, J., et al. (2014b). Metagenomics reveals sediment microbial community response to deepwater horizon oil spill. ISME J. 8, 1464–1475. doi: 10.1038/ismej.2013.254
McCaig, A. E., Glover, L. A., and Prosser, J. I. (2001). Numerical analysis of grassland bacterial community structure under different land management regimens by using 16S ribosomal DNA sequence data and denaturing gradient gel electrophoresis banding patterns. Appl. Environ. Microbiol. 67, 4554–4559. doi: 10.1128/AEM.67.10.4554-4559.2001
McKew, B. A., Coulon, F., Osborn, A. M., Timmis, K. N., and McGenity, T. J. (2007). Determining the identity and roles of oil-metabolizing marine bacteria from the Thames estuary, UK. Environ. Microbiol. 9, 165–176. doi: 10.1111/j.1462-2920.2006.01125.x
McMurdie, P. J., and Holmes, S. (2013). phyloseq: an R package for reproducible interactive analysis and graphics of microbiome census data. PLoS ONE 8:e61217. doi: 10.1371/journal.pone.0061217
Mearns, A. J., Reish, D. J., Oshida, P. S., and Ginn, T. (2010). Effects of pollution on marine organisms. Water Environ. Res. 82, 2001–2046. doi: 10.2175/106143010X12756668802175
Montagna, P., Baguley, J. G., Cooksey, C., Hartwell, I., Hyde, L. J., Hyland, J. L., et al. (2013). Deep-sea benthic footprint of the deepwater horizon blowout. PLoS ONE 8:e70540. doi: 10.1371/journal.pone.0070540
Muschenheim, D., and Lee, K. (2002). Removal of oil from the sea surface through particulate interactions: review and prospectus. Spill Sci. Technol. Bull. 8, 9–18. doi: 10.1016/S1353-2561(02)00129-9
Muyzer, G., de Waal, E. C., and Uitterlinden, A. G. (1993). Profiling of complex microbial populations by denaturing gradient gel electrophoresis analysis of polymerase chain reaction-amplified genes coding for 16S rRNA. Appl. Environ. Microbiol. 59, 695–700.
Muyzer, G., and Smalla, K. (1998). Application of denaturing gradient gel electrophoresis (DGGE) and temperature gradient gel electrophoresis (TGGE) in microbial ecology. Ant. Van Leeuwen. 73, 127–141. doi: 10.1023/A:1000669317571
Oger, P. M., and Jebbar, M. (2010). The many ways of coping with pressure. Res. Microbiol. 161, 799–809. doi: 10.1016/j.resmic.2010.09.017
Oksanen, J., Blanchet, F. G., Friendly, M., Kindt, R., Legendre, P., McGlinn, D., et al. (2017). Vegan: Community Ecology Package. Available online at: https://cran.r-project.org/package=vegan
Passow, U., Ziervogel, K., Asper, V., and Diercks, A. (2012). Marine snow formation in the aftermath of the deepwater horizon oil spill in the Gulf of Mexico. Environ. Res. Lett. 7:35301. doi: 10.1088/1748-9326/7/3/035301
Pinheiro, J., Bates, D., DebRoy, S., and Sarkar, D., and R Core Team (2017). Linear and Nonlinear Mixed Effects Models. Available online at: https://cran.r-project.org/package=nlme
Quast, C., Pruesse, E., Yilmaz, P., Gerken, J., Schweer, T., Yarza, P., et al. (2012). The SILVA ribosomal RNA gene database project: improved data processing and web-based tools. Nucleic Acids Res. 41, D590–D596. doi: 10.1093/nar/gks1219
Ramirez-Llodra, E., Tyler, P. A., Baker, M. C., Bergstad, O. A., Clark, M. R., Escobar, E., et al. (2011). Man and the last great wilderness: human impact on the deep sea. PLoS ONE 6:e22588. doi: 10.1371/journal.pone.0022588
Ravot, G., Magot, M., Fardeau, M.-L., Patel, B. K. C., Thomas, P., Garcia, J.-L., et al. (1999). Fusibacter paucivorans gen. nov., sp. nov., an anaerobic, thiosulfate-reducing bacterium from an oil-producing well. Int. J. Syst. Bacteriol. 49, 1141–1147. doi: 10.1099/00207713-49-3-1141
R Core Team (2017). R: A Language and Environment for Statistical Computing: The R Foundation for Statistical Computing. Available online at: https://www.r-project.org/
Redmond, M. C., and Valentine, D. L. (2012). Natural gas and temperature structured a microbial community response to the Deepwater Horizon oil spill. Proc. Natl Acad. Sci. U.S.A. 109, 20292–20297. doi: 10.1073/pnas.1108756108
Romero, I. C., Schwing, P. T., Brooks, G. R., Larson, R. A., Hastings, D. W., Ellis, G., et al. (2015). Hydrocarbons in deep-sea sediments following the 2010 Deepwater Horizon blowout in the northeast Gulf of Mexico. PLoS ONE 10:e0128371. doi: 10.1371/journal.pone.0128371
Salam, L. B., Ilori, M. O., Amund, O. O., LiiMien, Y., and Nojiri, H. (2017). Characterization of bacterial community structure in a hydrocarbon-contaminated tropical African soil. Environ. Technol. 39, 939–951. doi: 10.1080/09593330.2017.1317838
Scarlett, A., Galloway, T. S., Canty, M., Smith, E. L., Nilsson, J., and Rowland, S. J. (2005). Comparative Toxicity of two oil dispersants, superdispersant-25 and corexit 9527, to a range of coastal species. Environ. Toxicol. Chem. 24, 1219–1227. doi: 10.1897/04-334R.1
Scott, N. M., Hess, M., Bouskill, N. J., Mason, O. U., Jansson, J. K., and Gilbert, J. A. (2014). The microbial nitrogen cycling potential is impacted by polyaromatic hydrocarbon pollution of marine sediments. Front. Microbiol. 5:108. doi: 10.3389/fmicb.2014.00108
Seidel, M., Kleindienst, S., Dittmar, T., Joye, S. B., and Medeiros, P. M. (2015). Biodegradation of crude oil and dispersants in deep seawater from the Gulf of Mexico: Insights from ultra-high resolution mass spectrometry. Deep Sea Res. II Top. Stud. Oceanogr. 129, 108–118. doi: 10.1016/j.dsr2.2015.05.012
Serrano, A. E., Escudero, L. V., Tebes-Cayo, C., Acosta, M., Encalada, O., Fernández-Moroso, S., et al. (2017). First draft genome sequence of a strain from the genus Fusibacter isolated from Salar de Ascotán in Northern Chile. Stand. Genomic Sci. 12:43. doi: 10.1186/s40793-017-0252-4
Sharma, P., and Schiewer, S. (2016). Assessment of crude oil biodegradation in arctic seashore sediments: effects of temperature, salinity, and crude oil concentration. Environ. Sci. Pollut. Res. 23, 14881–14888. doi: 10.1007/s11356-016-6601-9
Smallwood, J. R., and Kirk, W. J. (2005). Paleocene exploration in the Faroe – Shetland Channel: disappointments and discoveries. Pet. Geol. Conf. Ser. 1, 977–991. doi: 10.1144/0060977
Smii, L., Ben Hania, W., Cayol, J.-L., Joseph, M., Hamdi, M., Ollivier, B., et al. (2015). Fusibacter bizertensis sp. nov., isolated from a corroded kerosene storage tank. Int. J. Syst. Evol. Microbiol. 65, 117–121. doi: 10.1099/ijs.0.066183-0
Stoffyn-Egli, P., and Lee, K. (2002). Formation and characterization of oil–mineral aggregates. Spill Sci. Technol. Bull. 8, 31–44. doi: 10.1016/S1353-2561(02)00128-7
Stucky, B. J. (2012). Seqtrace: a graphical tool for rapidly processing DNA sequencing chromatograms. J. Biomol. Tech. 23, 90–93. doi: 10.7171/jbt.12-2303-004
Syutsubo, K., Kishira, H., and Harayama, S. (2001). Development of specific oligonucleotide probes for the identification and in situ detection of hydrocarbon-degrading Alcanivorax strains. Environ. Microbiol. 3, 371–379. doi: 10.1046/j.1462-2920.2001.00204.x
Tansel, B., Fuentes, C., Sanchez, M., Predoi, K., and Acevedo, M. (2011). Persistence profile of polyaromatic hydrocarbons in shallow and deep Gulf waters and sediments: effect of water temperature and sediment-water partitioning characteristics. Mar. Pollut. Bull. 62, 2659–2665. doi: 10.1016/j.marpolbul.2011.09.026
The Energy and Climate Change Committee (2011). UK Deepwater Drilling—Implications of the Gulf of Mexico Oil Spill. Government Response to the Committee's Second Report of Session 2010–11.
Thibodeau, L. J., and Mackay, D. (eds.). (2011). Handbook of Chemical Mass Transport in the Environment. Boca Raton, FL: Taylor and Francis Group.
Valentine, D. L., Fisher, G. B., Bagby, S. C., Nelson, R. K., Reddy, C. M., Sylva, S. P., et al. (2014). Fallout plume of submerged oil from Deepwater Horizon. Proc. Natl. Acad. Sci. U.S.A. 111, 15906–15911. doi: 10.1073/pnas.1414873111
Valentine, D. L., Kessler, J. D., Redmond, M. C., Mendes, S. D., Heintz, M. B., Farwell, C., et al. (2010). Propane respiration jump-starts microbial response to a deep oil spill. Science 330, 208–211. doi: 10.1126/science.1196830
Viggor, S., Juhanson, J., Jõesaar, M., Mitt, M., Truu, J., Vedler, E., et al. (2013). Dynamic changes in the structure of microbial communities in Baltic Sea coastal seawater microcosms modified by crude oil, shale oil or diesel fuel. Microbiol. Res. 168, 415–427. doi: 10.1016/j.micres.2013.02.006
White, H. K., Hsing, P. Y., Cho, W., Shank, T. M., Cordes, E. E., Quattrini, A. M., et al. (2012). Impact of the Deepwater Horizon oil spill on a deep-water coral community in the Gulf of Mexico. Proc. Natl. Acad. Sci. U.S.A. 109, 20303–20308. doi: 10.1073/pnas.1118029109
Wickham, H., and Chang, W. (2009). ggplot2: Elegant Graphics for Data Analysis. Available online at: http://ggplot2.org
Widdel, K., Knittel, A., and Galushko, F. (2010). “Anaerobic hydrocarbon-degrading microorganisms: an overview,” in Handbook of Hydrocarbon and Lipid Microbiology, ed K. N. Timmis (Berlin; Heidelberg: Springer-Verlag), 1997–2021.
Xu, Y. (2003). Moritella profunda sp. nov. and Moritella abyssi sp. nov., two psychropiezophilic organisms isolated from deep Atlantic sediments. Int. J. Syst. Evol. Microbiol. 53, 533–538. doi: 10.1099/ijs.0.02228-0
Yakimov, M. M., Giuliano, L., Gentile, G., Crisafi, E., Chernikova, T. N., Abraham, W. R., et al. (2003). Oleispira antarctica gen. nov., sp. nov., a novel hydrocarbonoclastic marine bacterium isolated from Antarctic coastal sea water. Int. J. Syst. Evol. Microbiol. 53, 779–785. doi: 10.1099/ijs.0.02366-0
Yanagibayashi, M., Nogi, Y., Li, L., and Kato, C. (1999). Changes in the microbial community in Japan Trench sediment from a depth of 6292 m during cultivation without decompression. FEMS Microbiol. Lett. 170, 271–279. doi: 10.1111/j.1574-6968.1999.tb13384.x
Yang, T., Nigro, L. M., Gutierrez, T., DAmbrosio, L., Joye, S. B., Highsmith, R., et al. (2014). Pulsed blooms and persistent oil-degrading bacterial populations in the water column during and after the Deepwater Horizon blowout. Deep Sea Res. II Top. Stud. Oceanogr. 129, 282–291. doi: 10.1016/j.dsr2.2014.01.014
Yang, T., Speare, K., McKay, L., MacGregor, B. J., Joye, S. B., and Teske, A. (2016). Distinct bacterial communities in surficial seafloor sediments following the 2010 deepwater horizon blowout. Front. Microbiol. 7:1384. doi: 10.3389/fmicb.2016.01384
Yang, Z. F., Xia, X. H., Huang, G. H., Zhou, J. S., and Rong, X. (2008). Effect of sediment on the biodegradation of petroleum contaminants in natural water. Petrol. Sci. Technol. 26, 868–886. doi: 10.1080/10916460701824516
Zhao, X., Gong, Y., O'Reilly, S. E., and Zhao, D. (2015). Effects of oil dispersant on solubilization, sorption and desorption of polycyclic aromatic hydrocarbons in sediment–seawater systems. Mar. Pollut. Bull. 92, 160–169. doi: 10.1016/j.marpolbul.2014.12.042
Zhao, X., Liu, W., Fu, J., Cai, Z., O'Reilly, S. E., Zhao, D., et al. (2016). Dispersion, sorption and photodegradation of petroleum hydrocarbons in dispersant-seawater-sediment systems. Mar. Pollut. Bull. 109, 526–538. doi: 10.1016/j.marpolbul.2016.04.064
Ziervogel, K., McKay, L., Rhodes, B., Osburn, C. L., Dickson-Brown, J., Arnosti, C., et al. (2012). Microbial activities and dissolved organic matter dynamics in oil-contaminated surface seawater from the deepwater horizon oil spill site. PLoS ONE 7:e34816. doi: 10.1371/journal.pone.0034816
Keywords: oil spill, deep-sea sediment, hydrocarbon degradation, hydrocarbon entrainment, bacteria, dispersant, pollution, Faroe-Shetland Channel
Citation: Perez Calderon LJ, Potts LD, Gontikaki E, Gubry-Rangin C, Cornulier T, Gallego A, Anderson JA and Witte U (2018) Bacterial Community Response in Deep Faroe-Shetland Channel Sediments Following Hydrocarbon Entrainment With and Without Dispersant Addition. Front. Mar. Sci. 5:159. doi: 10.3389/fmars.2018.00159
Received: 13 October 2017; Accepted: 23 April 2018;
Published: 17 May 2018.
Edited by:
Ricardo Serrão Santos, University of the Azores, PortugalReviewed by:
Benjamin Harry Gregson, University of Essex, United KingdomGuillermo Ladino Orjuela, Centro Universitário de Votuporanga, Brazil
Conceicao Egas, Universidade de Coimbra, Portugal
Copyright © 2018 Perez Calderon, Potts, Gontikaki, Gubry-Rangin, Cornulier, Gallego, Anderson and Witte. This is an open-access article distributed under the terms of the Creative Commons Attribution License (CC BY). The use, distribution or reproduction in other forums is permitted, provided the original author(s) and the copyright owner are credited and that the original publication in this journal is cited, in accordance with accepted academic practice. No use, distribution or reproduction is permitted which does not comply with these terms.
*Correspondence: Luis J. Perez Calderon, bGoucGVyZXpjYWxkZXJvbkBnbWFpbC5jb20=
Lloyd D. Potts, bGxveWQucG90dHNAYWJkbi5hYy51aw==
†These authors have contributed equally to this work.