- Department of Plant and Environmental Sciences, Weizmann Institute of Science, Rehovot, Israel
Microscopic marine phytoplankton drift freely in the ocean, harvesting sunlight through photosynthesis. These unicellular microorganisms account for half of the primary productivity on Earth and play pivotal roles in the biogeochemistry of our planet (Field et al., 1998). The major groups of microalgae that comprise the phytoplankton community are coccolithophores, diatoms and dinoflagellates. In present oceans, phytoplankton individuals and populations are forced to rapidly adjust, as key chemical and physical parameters defining marine habitats are changing globally. Here we propose that microalgal populations often display the characteristics of a multicellular-like community rather than a random collection of individuals. Evolution of multicellularity entails a continuum of events starting from single cells that go through aggregation or clonal divisions (Brunet and King, 2017). Phytoplankton may be an intermediate state between single cells and aggregates of physically attached cells that communicate and co-operate; perhaps an evolutionary snapshot toward multicellularity. In this opinion article, we journey through several studies conducted in two key phytoplankton groups, coccolithophores and diatoms, to demonstrate how observations in these studies could be interpreted in a multicellular context.
Emiliania huxleyi is the most widespread coccolithophore in modern oceans. Populations of this microalga form massive seasonal blooms that cover thousands of square kilometers and are easily detected by satellites (Holligan et al., 1983; Balch et al., 1991; Balch, 2018). The blooms exhibit unique dynamics whereby they form seasonally over several weeks and then suddenly collapse (Tyrrell and Merico, 2004; Behrenfeld and Boss, 2014; Lehahn et al., 2014). The sudden demise of these blooms is mostly attributed to viral infection (Bratbak et al., 1993; Vardi et al., 2012; Lehahn et al., 2014), and bacteria have also been shown to drive the sudden collapse of E. huxleyi populations (Segev et al., 2016). Algal death, whether due to biotic or abiotic factors, often bears much similarity to Programmed Cell Death (PCD), a process known from higher plants and animals (Bidle, 2016).
PCD has also been reported in diatoms, a key group of phytoplankton. Diatoms are responsible for 50% of the global phytoplankton productivity (Rousseaux and Gregg, 2014). Diatoms, similarly to coccolithophores, grow rapidly over wide areas of ocean, forming blooms that suddenly terminate with death of the vast majority of the population. Autocatalytic death in diatoms was initially reported in response to nutrient limitation (Brussaard et al., 1997; Berges and Falkowski, 1998). Numerous studies have since provided a comprehensive view of the environmental triggers and molecular mechanisms underlying various death mechanisms in diatoms (Bidle, 2015, 2016).
In multicellular organisms, PCD is a process that maintains proper growth and functionality of individuals (Hamburger and Levi-Montalcini, 1949; Glucksmann, 1951; Lockshin and Williams, 1964; Kerr et al., 1972; Milligan and Schwartz, 1996; Jones, 2001; Danial and Korsmeyer, 2004). PCD is an active and highly regulated process that can be undertaken by a subpopulation of cells that are infected or genetically perturbed, in order to eliminate the harmful influence and/or agent and save the other cells. It is considered to be an altruistic act executed by individual cells for the greater good of the entire organism (Glucksmann, 1951; Lockshin and Williams, 1964; Kerr et al., 1972; Milligan and Schwartz, 1996; Jones, 2001; Danial and Korsmeyer, 2004).
Much research has been conducted in recent years to elucidate the eco-physiology of PCD in phytoplankton (Franklin et al., 2006; Bidle, 2015, 2016). While knowledge about mechanisms driving and controlling PCD in phytoplankton expands, it remains unclear why a unicellular organism would execute a highly controlled death process. The paradox of PCD in single-celled organisms has been previously raised and discussed (Ameisen, 1996, 2002; Franklin et al., 2006; Nedelcu et al., 2011; Bayles, 2014; Durand et al., 2016). It has been posited that PCD in single-celled organisms has a different origin and nature than in multicellular organisms (Nedelcu et al., 2011). In addition, it has been proposed that PCD can increase biological complexity in microbial communities (Durand et al., 2016). Faced with the puzzling nature of unicellular PCD, we offer several observations and highlight reports from the literature that encourage a reconsideration of the multicellular features of phytoplankton. We provide several lines of evidence demonstrating multicellular traits of phytoplankton and subsequently discuss the benefits of phytoplankton PCD in analogy to this process in multicellular organisms.
To examine multicellular traits of phytoplankton, we sought to find a definition for multicellularity. Multicellularity has independently evolved at least 16 times within all domains of life (Bonner, 1998; King, 2004; Rokas, 2008; Knoll, 2011). Previous attempts to define multicellularity, especially in the microbial world, have delineated two essential parameters that must be met in a multicellular scenario: intercellular communication that leads to coordinated action, and cell-cell adhesion (Lyons and Kolter, 2015). In the next paragraphs we demonstrate modes of phytoplankton communication and cell-cell adhesion, and we discuss how phytoplankton PCD could be interpreted as a coordinated population action. While clearly phytoplankton cells exist as individual cells, we find ample reports suggesting that microalgae are frequently found as multicellular-like assemblages.
Phytoplankton Communication and Coordinated Behavior
In diatoms, individual cells can communicate warning signals to the entire population, triggering either cell death or community-wide defense mechanisms. A diatom cell experiencing stress conditions will produce the lethal aldehyde (2E,4E/Z)-decadienal (DD) (Miralto et al., 1999; Pohnert, 2002). Diatoms that are exposed to high levels of DD will execute PCD through cellular events that are based on nitric oxide (NO) signaling (Vardi et al., 2006). At the same time, diatoms that are exposed to lower levels of DD initiate an intracellular signaling cascade culminating in resistance to lethal concentrations of DD (Vardi et al., 2006). The first diatoms that are exposed to stress, release DD to the environment before they die, and in their death the hazard is communicated to the rest of the population enabling a coordinated population-wide immunization. DD discharge by the dying diatoms has another benefit for the population; one of the common stresses experienced by diatoms is due to copepod feeders that graze on diatom communities. DD impairs normal development of copepods and other invertebrates (Miralto et al., 1999; Caldwell et al., 2002; Ianora et al., 2004).
Nitric oxide (NO) is another potent signaling molecule with potential roles in phytoplankton cell-cell communication. NO has an established cellular function as a pivotal signaling molecule during PCD in both diatoms and coccolithophores. Diatom cells produce NO under various stresses (Vardi et al., 2008). Production of NO is likely to lead to the formation of reactive oxygen species and ultimately PCD. Upon lysis of the cell and the release of its content, the produced NO may be a cue that triggers PCD in neighboring cells (Vardi, 2008). As a freely diffusing free radical gas, NO from lysing cells easily spreads through the diatom population acting as a signal (Vardi et al., 2006). NO, along with other signaling molecules, has been defined a phytoplankton “infochemical” (Vardi, 2008), due to its capacity to carry information between individual phytoplankton cells.
Significant production of NO was also observed in E. huxleyi populations, both in virus-infected cultures and in natural populations in the ocean (Bidle, 2015; Hirsh et al., 2016). Indeed it appears that phytoplankton communities share information in complex manners facilitating communication between cells. The release of signals from some cells, which are perceived by other cells, culminates in a variety of coordinated actions including death, resilience, and possibly additional modes of behavior yet to be explored. Physical proximity between individuals would facilitate efficient communication within the population, especially when using universal signals as NO.
Phytoplankton Cell-Cell Adhesion
We have found that E. huxleyi cultures regularly aggregate. While clumping seemed no more than a technical nuisance, a closer look revealed that algal aggregates are a complex structure of cells encased within an extracellular matrix (Figure 1). The algal aggregates are held together by at least two structural components: extracellular DNA (Figure 2) and Transparent Exopolymer Particles (TEP) (Figure 2). Recently, proteins were characterized as an additional structural component involved in phytoplankton aggregation (Thornton and Chen, 2017). Interestingly, TEP generation increases under stress conditions, however production of matrix proteins does not (Thornton and Chen, 2017). These observations suggest that the production of the two polymers is differentially regulated, demonstrating the insufficiently studied complexity of phytoplankton aggregates. Further investigation both in the lab and in the environment is needed to determine the precise composition of the phytoplankton extracellular matrix. Current observations hold promise in providing a starting point for studying algal aggregation into multicellular-like assemblages. Both extracellular DNA and exopolysaccharides are structural component of bacterial biofilms (Watnick and Kolter, 2000; Whitchurch et al., 2002; Vlamakis et al., 2013). Bacterial biofilms are multicellular structures where, similar to E. huxleyi aggregates, individual bacterial cells rely on an extracellular matrix to act as a scaffold that holds the community together.
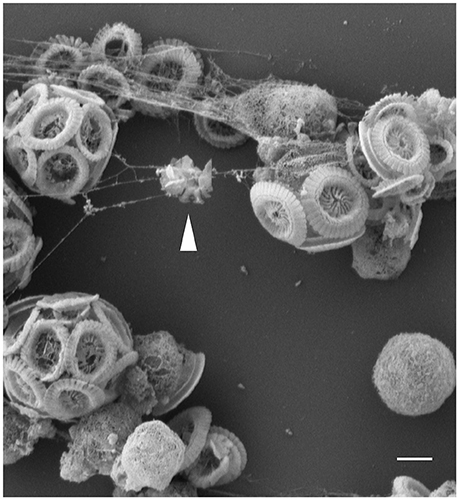
Figure 1. Threads of extracellular matrix connect algal cells. Scanning Electron Microscopy (SEM) image of E. huxleyi cells with threads attaching individual calcified and naked cells. Coccoliths debris seen trapped in the threads (see arrow head), suggestive of the adhesive nature of the filaments. Scale bar corresponds to 1 μm. See Data Sheet 1 for Materials and Methods.
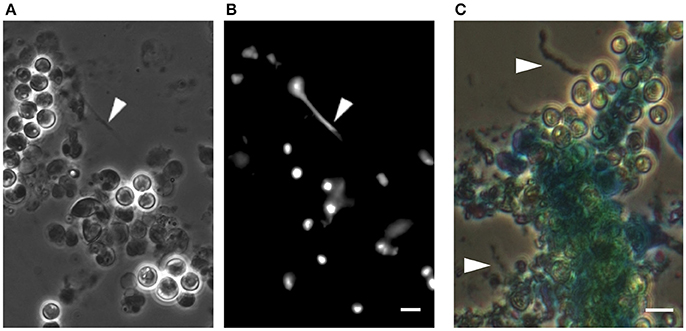
Figure 2. DNA-like threads and polysaccharide fibers in algal aggregates. (A) Phase contrast microscopy of an algal aggregate reveals linear structures looking like extracellular DNA threads (see arrow head). (B) Fluorescence image of the same algal aggregate demonstrating a fluorescent signal from the threads following DNA staining with Sytox green, a dye that cannot penetrate the cell membrane and thus stains extracellular DNA as well as DNA in cells with perturebed membranes. Scale bar corresponds to 4 μm. (C) Image of an algal aggregate stained with the polysaccaride-binding stain alcian blue. Individual fiber-like structures are seen (indicated by arrow heads). The central mass of algal cells appears to be encased in a matrix that absorbed the stain, suggesting an exopolysaccharide composition similar to TEP. Scale bar corresponds to 4 μm. See Data Sheet 1 for Materials and Methods.
The phenomenon of phytoplankton aggregation through TEP has been widely explored. Most reports examined TEP-mediated aggregation as a mechanism facilitating the sinking of particles composed primarily of stressed and dying cells (Nissimov and Bidle, 2017). TEP production indeed increases under various stresses (Chow et al., 2015), but generation of the polymer is also seen in the absence of stress conditions. Diatom production of these polysaccharides was shown to be dynamic, influenced by various environmental conditions such as temperature, acidity and light (Ferreyra et al., 2006; Seebah et al., 2014). TEP formation in diatoms varies between species and was found to change during different growth phases (Passow, 2002; Kahl et al., 2008; Chen and Thornton, 2015). The formation of diatom TEP is increased under turbulent conditions that challenge cell-cell adhesion, and coagulation of TEP-precursors is enhanced (Schuster and Herndl, 1995; Stoderegger and Herndl, 1999; Passow, 2000).
TEP formation by natural E. huxleyi populations was monitored in mesocosm experiments in which TEP production was followed during the course of an induced E. huxleyi bloom (Vardi et al., 2012). In this work, two mesocosms exhibited high and constant basal levels of polysaccharide production. In a third mesocosm TEP increased significantly following viral infection. Microscopy images show algal cells entangled in an extracellular matrix of TEP fibers (Vardi et al., 2012).
Taken together, cells in both coccolithophore and diatom communities appear to be joined together by components of structural and functional complexity. Interestingly, many genes that are necessary for animal multicellularity are involved in cell-cell adhesion and have evolved prior to animal origins (Brunet and King, 2017). Furthermore, clumping has been used as a trait to experimentally evolve multicellularity in yeast and algae (Ratcliff et al., 2012, 2013; Driscoll and Travisano, 2017). Following clumping, improved cooperation was evident resulting in better growth and enhanced ability to compete for resources (Koschwanez et al., 2011). In populations of the freshwater alga Chlorella as well as Chlamydomonas, a unicellular Volvocine alga, clumping occurred in response to predation as a defense mechanism (Sathe and Durand, 2016; Kapsetaki et al., 2017). Volvocine algae have been studied as a model system for the transition from unicellular to multicellular life (Kirk, 1999; Herron, 2016). This group includes species of clumping unicells such as Chlamydomonas, and the complex multicellular species of Volvox exhibiting division of labor into non-reproductive cells (Kirk, 1999; Hanschen et al., 2014; Herron, 2016). Genetic analysis of Volvocine algae revealed the key role of extracellular matrix in the transition to multicellularity (Merchant et al., 2007; Prochnik et al., 2010). It would therefore be fascinating to explore the genetics of phytoplankton exopolymers and modes of attachment and compare them to components with known functions in multicellularity such as cadherins, integrins, and extracellular matrix domains (Brunet and King, 2017). A better characterization of the phytoplankton extracellular polymers is crucial in determining whether these structural components are specific only toward the organisms that produce them, or whether the fibers are generally adhesive thereby promoting multi-species assemblages.
Sea Skin
TEP have long been recognized as crucial components in the upper millimeter of the ocean, a region also known as the sea surface microlayer (SML) (Verdugo et al., 2004; Wurl and Holmes, 2008). In 1983, Sieburth first hypothesized that the SML is a continuous hydrated gelatinous layer formed by complex organic structures, and referred to it as the “sea's skin” (Sieburth, 1983). Later studies have confirmed and further discussed the role of TEP in the formation and gelatinous nature of the SML (Wurl and Holmes, 2008; Cunliffe and Murrell, 2009). The source of the TEP that accumulates and determines the SML properties are the phytoplankton communities beneath it that continuously produce and replenish the reservoir of oceanic extracellular polysaccharides (Cunliffe and Murrell, 2009). The fact that TEP are found at the sea surface, attests to the buoyant nature of these polymers. The floating capacity of TEP would be crucial in mediating multicellular-like assemblages without forcing them to sink. Indeed further study into the composition and structure of TEP in floating versus sinking particles is needed. Floating colonies of the phytoplankton Phaeocystis are composed of cells embedded in a polysaccharidic matrix (Schoemann et al., 2005). The matrix in these colonies maintains the buoyancy of the aggregated cells and keeps them afloat (Schoemann et al., 2005). One could think of the SML as a viscous scaffold holding together a global and probably diverse community of phytoplankton cells that comprise Sieburth's sea skin.
Concluding Remarks
Given the abundance of an oceanic gelatinous SML matrix, the ability of phytoplankton to aggregate, communicate and execute coordinated behaviors, perhaps we should think of the global phytoplankton population as an “oceanic tissue”? An ecosystem of multicellular units, each unit interconnected by an extracellular matrix, acting semi-coherently across kilometer scale. The basic multicellular unit would be of physically attached cells. But what are the physical dimensions of such multicellular units? How big are populations of physically attached phytoplankton cells? These questions should be the subject of further study. Furthermore, once attached through extracellular fibers, do cells keep dividing? This might be a key question in attempts to decipher the onset of phytoplankton aggregates; do individual cells converge or do cells undergo clonal divisions while attached? Both modes of multicellular-like assemblages occur in various microorganisms including choanoflagellates, ciliates, fungi, amoeba, and more (Brunet and King, 2017). Aggregation and clonal division are considered to be ancestral forms of current complex multicellularity, with clonal development being ancestral to all forms (Brunet and King, 2017). Regardless of its mode of formation, physical proximity between phytoplankton cells would make it easier for metabolites to diffuse among individuals, transferring information that enables coordinated behaviors.
The existence and evolution of PCD in unicells is controversial (Deponte, 2008). PCD in unicellular organisms could be explained from an evolutionary point of view using inclusive fitness theory, as long as cells are surrounded by genetic relatives (Hamilton, 1964). Adaptive explanations regarding the benefits conferred by PCD in a population of unicells have been discussed and supported by empirical work (Franklin et al., 2006; Vardi et al., 2007; Durand et al., 2014). Non-adaptive explanations discussing the different evolution and functions of phytoplankton PCD have also been raised and experimentally supported (Segovia et al., 2003; Jiménez et al., 2009; Nedelcu et al., 2011). Here we argue that phytoplankton populations have multicellular features that encourage examination of PCD in phytoplankton similarly to PCD in metazoans.
In analogy to the benefits of PCD in metazoans, a subset of the community members must survive the death of their siblings for a population-wide PCD to be beneficial in a multicellular-like community. Indeed, both coccolithophores and diatoms have such subpopulations with altered and durable phenotypes; in E. huxleyi a shift to a motile cell type occurs in a small part of the population following viral infection (Frada et al., 2017). The motile cells are resistant to the virus. Thus, while the virus triggers the spread of PCD in the population, the infection gives rise to a resistant subpopulation. A similar phenotype-remodeling mechanism is seen in diatoms, where resting spores that are highly resilient cells that endure environmental stresses, can be formed (Sims et al., 2006). These survival strategies demonstrate how the context of multicellularity could facilitate a better understanding of phytoplankton PCD and phenotypic variations.
What areas of research would benefit from acknowledging the multicellular features of phytoplankton? We offer here two examples of testable hypotheses with the hope of sparking discussions that would lead to further ideas:
(i) The algal microenvironment- In E. huxleyi, chemical features of the algal cell and its mineralized shell are used as indicators of environmental and physiological conditions. Examples include boron concentrations and isotopic composition as pH indicators (Stoll et al., 2012) and carbon isotopes as CO2 proxies (Pagani, 2002). In algal clumps, it is likely that the chemical features of microenvironments within aggregates differ from the ambient conditions due to respiration, calcification and altered diffusion imposed by the extracellular matrix. Therefore clumping, as a regular feature of multicellularity, should be considered in efforts to use algal derivatives as indicators of environmental conditions. In line with this idea, in blooms of the dinoflagellate Peridinium gatunense, the pH increases in dinoflagellate patches resulting in CO2 limitation and oxidative stress (Vardi et al., 1999). Further, the chemical regime in the aggregate microenvironment would likely resemble a semi-closed system due to restricted diffusion. In comparison with the open ocean, nutrients would be exhausted more rapidly within aggregates and cells would become isotopically heavier (Kendall and Caldwell, 1998).
(ii) Functional heterogeneity- Phenotypic heterogeneity in isogenic phytoplankton communities is found in enzymatic activities (Dyhrman and Palenik, 2003), structural features (Godoi et al., 2009), biomineralization processes (Znachor et al., 2013), biosynthesis of secreted molecules (Hamilton and Lenton, 1998), motility (Frada et al., 2017) and more. In a multicellular context, the heterogeneity in these populations could be a manifestation of division of labor (Crespi, 2001). Whether disruption of algal aggregates influences the heterogeneity of algal populations should be further tested.
As our oceans change, phytoplankton populations are forced to quickly adapt. How phytoplankton cells adhere to each other, share information, and give rise to coordinated activity merits further study both in the laboratory and in the environment to allow a comprehensive understanding of these key populations under changing conditions.
Author Contributions
AA and ES wrote the manuscript. ES conducted experiments and acquired images.
Conflict of Interest Statement
The authors declare that the research was conducted in the absence of any commercial or financial relationships that could be construed as a potential conflict of interest.
Acknowledgments
We are grateful to Roberto Kolter for inspiring discussions and insightful comments. We thank Assaf Vardi, Daniella Schatz, Assaf Gal, and Or Eliason for thoroughly reading the manuscript and providing valuable input. We are thankful to Jonathan Gressel for his valuable assistance in editing the manuscript. The authors wish to thank Scott Chimileski and Nick Lyons for their feedback on earlier versions of this manuscript. We thank Estelle Kalfon-Cohen for her assistance with electron microscopy and the Harvard Center for Nanoscale Systems for use of its imaging facility. ES is supported by the Estate of Jacqueline Hodes and the Estate of Fannie Sherr.
Supplementary Material
The Supplementary Material for this article can be found online at: https://www.frontiersin.org/articles/10.3389/fmars.2018.00144/full#supplementary-material
References
Ameisen, J. C. (1996). The origin of programmed cell death. Science 272, 1278–1279. doi: 10.1126/science.272.5266.1278
Ameisen, J. C. (2002). On the origin, evolution, and nature of programmed cell death: a timeline of four billion years. Cell 9, 367–393. doi: 10.1038/sj.cdd.4400950
Balch, W. M. (2018). The ecology, biogeochemistry, and optical properties of coccolithophores. Ann. Rev. Mar. Sci. 10, 71–98. doi: 10.1146/annurev-marine-121916-063319
Balch, W. M., Holligan, P. M., Ackleson, S. G., and Voss, K. J. (1991). Biological and optical-properties of mesoscale coccolithophore blooms in the gulf of Maine. Limnol. Oceanogr. 36, 629–643. doi: 10.4319/lo.1991.36.4.0629
Bayles, K. W. (2014). Bacterial programmed cell death: making sense of a paradox. Nat. Rev. Microbiol. 12, 63–69. doi: 10.1038/nrmicro3136
Behrenfeld, M. J., and Boss, E. S. (2014). Resurrecting the ecological underpinnings of ocean plankton blooms. Ann. Rev. Mar. Sci. 6, 167–194. doi: 10.1146/annurev-marine-052913-021325
Berges, J. A., and Falkowski, P. G. (1998). Physiological stress and cell death in marine phytoplankton: induction of proteases in response to nitrogen or light limitation. Limnol. Oceanogr. 43, 129–135. doi: 10.4319/lo.1998.43.1.0129
Bidle, K. D. (2015). The molecular ecophysiology of programmed cell death in marine phytoplankton. Ann. Rev. Mar. Sci. 7, 341–375. doi: 10.1146/annurev-marine-010213-135014
Bidle, K. D. (2016). Programmed cell death in unicellular phytoplankton. Curr. Biol. 26, R594–R607. doi: 10.1016/j.cub.2016.05.056
Bonner, J. T. (1998). The origins of multicellularity. Integr. Biol. 1, 27–36. doi: 10.1002/(SICI)1520-6602(1998)1:1 < 27::AID-INBI4>3.0.CO;2-6
Bratbak, G., Egge, J. K., and Heldal, M. (1993). Viral mortality of the marine alga Emiliania huxleyi (haptophyceae) and termination of algal blooms. Mar. Ecol. Prog. Ser. 93, 39–48. doi: 10.3354/meps093039
Brunet, T., and King, N. (2017). The origin of animal multicellularity and cell differentiation. Dev. Cell 43, 124–140. doi: 10.1016/j.devcel.2017.09.016
Brussaard, C. P. D., Noordeloos, A. A. M., and Riegman, R. (1997). Autolysis kinetics of the marine diatom Ditylum brightwellii (Bacillariophyceae) under nitrogen and phosphorus limitation and starvation. J. Phycol. 33, 980–987. doi: 10.1111/j.0022-3646.1997.00980.x
Caldwell, G. S., Olive, P. J., and Bentley, M. G. (2002). Inhibition of embryonic development and fertilization in broadcast spawning marine invertebrates by water soluble diatom extracts and the diatom toxin 2-trans,4-trans decadienal. Aquat. Toxicol. 60, 123–137. doi: 10.1016/S0166-445X(01)00277-6
Chen, J., and Thornton, D. C. (2015). Transparent exopolymer particle production and aggregation by a marine planktonic diatom (Thalassiosira weissflogii) at different growth rates. J. Phycol. 51, 381–393. doi: 10.1111/jpy.12285
Chow, J. S., Lee, C., and Engel, A. (2015). The influence of extracellular polysaccharides, growth rate, and free coccoliths on the coagulation efficiency of Emiliania huxleyi. Mar. Chem. 175, 5–17. doi: 10.1016/j.marchem.2015.04.010
Crespi, B. J. (2001). The evolution of social behavior in microorganisms. Trends Ecol. Evol. 16, 178–183. doi: 10.1016/S0169-5347(01)02115-2
Cunliffe, M., and Murrell, J. C. (2009). The sea-surface microlayer is a gelatinous biofilm. Isme. J. 3, 1001–1003. doi: 10.1038/ismej.2009.69
Danial, N. N., and Korsmeyer, S. J. (2004). Cell death: critical control points. Cell 116, 205–219. doi: 10.1016/S0092-8674(04)00046-7
Deponte, M. (2008). Programmed cell death in protists. Biochim Biophys. Acta 1783, 1396–1405. doi: 10.1016/j.bbamcr.2008.01.018
Driscoll, W. W., and Travisano, M. (2017). Synergistic cooperation promotes multicellular performance and unicellular free-rider persistence. Nat. Commun. 8:15707. doi: 10.1038/ncomms15707
Durand, P. M., Choudhury, R., Rashidi, A., and Michod, R. E. (2014). Programmed death in a unicellular organism has species-specific fitness effects. Biol. Lett. 10:20131088. doi: 10.1098/rsbl.2013.1088
Durand, P. M., Sym, S., and Michod, R. E. (2016). Programmed cell death and complexity in microbial systems. Curr. Biol. 26, R587–593. doi: 10.1016/j.cub.2016.05.057
Dyhrman, S. T., and Palenik, B. (2003). Characterization of ectoenzyme activity and phosphate-regulated proteins in the coccolithophorid Emiliania huxleyi. J. Plankton Res. 25, 1215–1225. doi: 10.1093/plankt/fbg086
Ferreyra, G. A., Mostajir, B., Schloss, I. R., Chatila, K., Ferrario, M. E., Sargian, P., et al. (2006). Ultraviolet-B radiation effects on the structure and function of lower trophic levels of the marine planktonic food web. Photochem. Photobiol. 82, 887–897. doi: 10.1562/2006-02-23-RA-810
Field, C. B., Behrenfeld, M. J., Randerson, J. T., and Falkowski, P. (1998). Primary production of the biosphere: integrating terrestrial and oceanic components. Science 281, 237–240. doi: 10.1126/science.281.5374.237
Frada, M. J., Rosenwasser, S., Ben-Dor, S., Shemi, A., Sabanay, H., and Vardi, A. (2017). Morphological switch to a resistant subpopulation in response to viral infection in the bloom-forming coccolithophore Emiliania huxleyi. PLoS Pathog. 13:e1006775. doi: 10.1371/journal.ppat.1006775
Franklin, D. J., Brussaard, C. P., and Berges, J. A. (2006). What is the role and nature of programmed cell death in phytoplankton ecology? Eur. J. Phycol. 41, 1–14. doi: 10.1080/09670260500505433
Glucksmann, A. (1951). Cell deaths in normal vertebrate ontogeny. Biol. Rev. Camb. Philos. Soc. 26, 59–86. doi: 10.1111/j.1469-185X.1951.tb00774.x
Godoi, R. H. M., Aerts, K., Harlay, J., Kaegi, R., Ro, C.-U., Chou, L., et al. (2009). Organic surface coating on Coccolithophores - Emiliania huxleyi: its determination and implication in the marine carbon cycle. Microchem. J. 91, 266–271. doi: 10.1016/j.microc.2008.12.009
Hamburger, V., and Levi-Montalcini, R. (1949). Proliferation, differentiation and degeneration in the spinal ganglia of the chick embryo under normal and experimental conditions. J. Exp. Zool. 111, 457–501. doi: 10.1002/jez.1401110308
Hamilton, W. D. (1964). The genetical evolution of social behaviour II. J. Theor. Biol. 7, 17–52. doi: 10.1016/0022-5193(64)90039-6
Hamilton, W. D., and Lenton, T. M. (1998). Spora and Gaia: how microbes fly with their clouds. Ethol. Ecol. Evol. 10, 1–16. doi: 10.1080/08927014.1998.9522867
Hanschen, E. R., Ferris, P. J., and Michod, R. E. (2014). Early evolution of the genetic basis for soma in the Volvocaceae. Evolution 68, 2014–2025. doi: 10.1111/evo.12416
Herron, M. D. (2016). Origins of multicellular complexity: volvox and the volvocine algae. Mol. Ecol. 25, 1213–1223. doi: 10.1111/mec.13551
Hirsh, D. J., Schieler, B. M., Fomchenko, K. M., Jordan, E. T., and Bidle, K. D. (2016). A liposome-encapsulated spin trap for the detection of nitric oxide. Free Radic. Biol. Med. 96, 199–210. doi: 10.1016/j.freeradbiomed.2016.04.026
Holligan, P. M., Viollier, M., Harbour, D. S., Camus, P., and Champagne-Phillippe, M. (1983). Satellite and ship studies of coccolithophore production along a continental shelf edge. Nature 304, 339–342. doi: 10.1038/304339a0
Ianora, A., Miralto, A., Poulet, S. A., Carotenuto, Y., Buttino, I., Romano, G., et al. (2004). Aldehyde suppression of copepod recruitment in blooms of a ubiquitous planktonic diatom. Nature 429, 403–407. doi: 10.1038/nature02526
Jiménez, C., Capasso, J. M., Edelstein, C. L., Rivard, C. J., Lucia, S., Breusegem, S., et al. (2009). Different ways to die: cell death modes of the unicellular chlorophyte Dunaliella viridis exposed to various environmental stresses are mediated by the caspase-like activity DEVDase. J. Exp. Bot. 60, 815–828. doi: 10.1093/jxb/ern330
Jones, A. M. (2001). Programmed cell death in development and defense. Plant Physiol. 125, 94–97. doi: 10.1104/pp.125.1.94
Kahl, L. A., Vardi, A., and Schofield, O. (2008). Effects of phytoplankton physiology on export flux. Mar. Ecol. Prog. Ser. 354, 3–19. doi: 10.3354/meps07333
Kapsetaki, S. E., Tep, A., and West, S. A. (2017). How do algae form multicellular groups? Evol. Ecol. Res. 18, 663–675.
Kendall, C., and Caldwell, E. A. (1998). Isotope Tracers in Catchment Hydrology Place. Amsterdam: Elsevier Science.
Kerr, J. F., Wyllie, A. H., and Currie, A. R. (1972). Apoptosis: a basic biological phenomenon with wide-ranging implications in tissue kinetics. Br. J. Cancer 26, 239–257. doi: 10.1038/bjc.1972.33
King, N. (2004). The unicellular ancestry of animal development. Dev. Cell 7, 313–325. doi: 10.1016/j.devcel.2004.08.010
Kirk, D. L. (1999). Evolution of multicellularity in the Volvocine algae. Curr. Opin. Plant Biol. 2, 496–501. doi: 10.1016/S1369-5266(99)00019-9
Knoll, A. H. (2011). The multiple origins of complex multicellularity. Annu. Rev. Earth Planet. Sci. 39, 217–239. doi: 10.1146/annurev.earth.031208.100209
Koschwanez, J. H., Foster, K. R., and Murray, A. W. (2011). Sucrose utilization in budding yeast as a model for the origin of undifferentiated multicellularity. PLoS Biol. 9:e1001122. doi: 10.1371/annotation/0b9bab0d-1d20-46ad-b318-d2229cde0f6f
Lehahn, Y., Koren, I., Schatz, D., Frada, M., Sheyn, U., Boss, E., et al. (2014). Decoupling physical from biological processes to assess the impact of viruses on a mesoscale algal bloom. Curr. Biol. 24, 2041–2046. doi: 10.1016/j.cub.2014.07.046
Lockshin, R. A., and Williams, C. M. (1964). Programmed cell death. II. Endocrine potentiation of the breakdown of the intersegmental muscles of silkmoths. J. Insect Physiol. 10, 643–649.
Lyons, N. A., and Kolter, R. (2015). On the evolution of bacterial multicellularity. Curr. Opin. Microbiol. 24, 21–28. doi: 10.1016/j.mib.2014.12.007
Merchant, S. S., Prochnik, S. E., Vallon, O., Harris, E. H., Karpowicz, S. J., Witman, G. B., et al. (2007). The Chlamydomonas genome reveals the evolution of key animal and plant functions. Science 318, 245–250. doi: 10.1126/science.1143609
Milligan, C. E., and Schwartz, L. M. (1996). “Programmed cell death during development of animals,” in Cellular Aging and Cell Death, eds N. J. Holbrook, G. R. M. Martin, and R. A. Lockshin (New York, NY: Wiley-Liss), 181–208.
Miralto, A., Barone, G., Romano, G., Poulet, S. A., Ianora, A., Russo, G. L., et al. (1999). The insidious effect of diatoms on copepod reproduction. Nature 402, 173–176. doi: 10.1038/46023
Nedelcu, A. M., Driscoll, W. W., Durand, P. M., Herron, M. D., and Rashidi, A. (2011). On the paradigm of altruistic suicide in the unicellular world. Evolution 65, 3–20. doi: 10.1111/j.1558-5646.2010.01103.x
Nissimov, J. I., and Bidle, K. D. (2017). Stress, death, and the biological glue of sinking matter. J. Phycol. 53, 241–244. doi: 10.1111/jpy.12506
Pagani, M. (2002). The alkenone–CO2 proxy and ancient atmospheric carbon dioxide. Philos. Trans. A Math. Phys. Eng. Sci. 360, 609–632. doi: 10.1098/rsta.2001.0959
Passow, U. (2000). Formation of transparent exopolymer particles, TEP, from dissolved precursor material. Mar. Ecol. Prog. Ser. 192, 1–11. doi: 10.3354/meps192001
Passow, U. (2002). Transparent exopolymer particles (TEP) in aquatic environments. Prog. Oceanogr. 55, 287–333. doi: 10.1016/S0079-6611(02)00138-6
Pohnert, G. (2002). Phospholipase A2 activity triggers the wound-activated chemical defense in the diatom Thalassiosira rotula. Plant Physiol. 129, 103–111. doi: 10.1104/pp.010974
Prochnik, S. E., Umen, J., Nedelcu, A. M., Hallmann, A., Miller, S. M., Nishii, I., et al. (2010). Genomic analysis of organismal complexity in the multicellular green alga Volvox carteri. Science 329, 223–226. doi: 10.1126/science.1188800
Ratcliff, W. C., Denison, R. F., Borrello, M., and Travisano, M. (2012). Experimental evolution of multicellularity. Proc. Natl. Acad. Sci. U.S.A. 109, 1595–1600. doi: 10.1073/pnas.1115323109
Ratcliff, W. C., Herron, M. D., Howell, K., Pentz, J. T., Rosenzweig, F., and Travisano, M. (2013). Experimental evolution of an alternating uni- and multicellular life cycle in Chlamydomonas reinhardtii. Nat. Commun. 4:2742. doi: 10.1038/ncomms3742
Rokas, A. (2008). The origins of multicellularity and the early history of the genetic toolkit for animal development. Annu. Rev. Genet. 42, 235–251. doi: 10.1146/annurev.genet.42.110807.091513
Rousseaux, S. C., and Gregg, W. W. (2014). Interannual variation in phytoplankton primary production at a global scale. Remote Sens. 6, 1–19. doi: 10.3390/rs6010001
Sathe, S., and Durand, P. M. (2016). Cellular aggregation in Chlamydomonas (Chlorophyceae) is chimaeric and depends on traits like cell size and motility. Eur. J. Phycol. 51, 129–138. doi: 10.1080/09670262.2015.1107759
Schoemann, V., Becquevort, S., Stefels, J., Rousseau, W., and Lancelot, C. (2005). Phaeocystis blooms in the global ocean and their controlling mechanisms: a review. J. Sea Res. 53, 43–66. doi: 10.1016/j.seares.2004.01.008
Schuster, S., and Herndl, G. J. (1995). Formation and significance of transparent exopolymeric particles in the northern adriatic sea. Mar. Ecol. Prog. Ser. 124, 227–236. doi: 10.3354/meps124227
Seebah, S., Fairfield, C., Ullrich, M. S., and Passow, U. (2014). Aggregation and sedimentation of Thalassiosira weissflogii (diatom) in a warmer and more acidified future ocean. PLoS ONE 9:e112379. doi: 10.1371/journal.pone.0112379
Segev, E., Wyche, T. P., Kim, K. H., Petersen, J., Ellebrandt, C., Vlamakis, H., et al. (2016). Dynamic metabolic exchange governs a marine algal-bacterial interaction. Elife 5:e17473. doi: 10.7554/eLife.17473
Segovia, M., Haramaty, L., Berges, J. A., and Falkowski, P. G. (2003). Cell death in the unicellular chlorophyte Dunaliella tertiolecta. A hypothesis on the evolution of apoptosis in higher plants and metazoans. Plant Physiol. 132, 99–105. doi: 10.1104/pp.102.017129
Sieburth, J. M. (1983). “Microbiological and organic–chemical processes in the surface and mixed layers,” in Air–Sea Exchange of Gases and Particles, eds P. S. Liss and W. G. N. Slinn (Hingham, MA: Reidel Publishers Co.), 121–172.
Sims, P. A., Mann, D. G., and Medlin, L. K. (2006). Evolution of the diatoms: insights from fossil, biological and molecular data. Phycologia 45, 361–402. doi: 10.2216/05-22.1
Stoderegger, K. E., and Herndl, G. J. (1999). Production of exopolymer particles by marine bacterioplankton under contrasting turbulence conditions. Mar. Ecol. Prog. Ser. 189, 9–16. doi: 10.3354/meps189009
Stoll, H. M., Langer, G., Shimizu, N., and Kanamaru, K. (2012). B/Ca in coccoliths and relationship to calcification vesicle pH and dissolved inorganic carbon concentrations. Geochim. Cosmochim. Ac. 80, 143–157. doi: 10.1016/j.gca.2011.12.003
Thornton, D. C. O., and Chen, J. (2017). Exopolymer production as a function of cell permeability and death in a diatom (Thalassiosira weissflogii) and a cyanobacterium (Synechococcus elongatus). J. Phycol. 53, 245–260. doi: 10.1111/jpy.12470
Tyrrell, T., and Merico, A. (2004). “Emiliania huxleyi: bloom observations and the conditions that induce them,” in Coccolithophores: From Molecular Processes to Global Impact, eds H. R. Thierstein and J. R. Young (Berlin; Heidelberg: Springer), 75–97.
Vardi, A. (2008). Cell signaling in marine diatoms. Commun. Integr. Biol. 1, 134–136. doi: 10.4161/cib.1.2.6867
Vardi, A., Berman-Frank, I., Rozenberg, T., Hadas, O., Kaplan, A., and Levine, A. (1999). Programmed cell death of the dinoflagellate Peridinium gatunense is mediated by CO2 limitation and oxidative stress. Curr. Biol. 9, 1061–1064. doi: 10.1016/S0960-9822(99)80459-X
Vardi, A., Bidle, K. D., Kwityn, C., Hirsh, D. J., Thompson, S. M., Callow, J. A., et al. (2008). A diatom gene regulating nitric-oxide signaling and susceptibility to diatom-derived aldehydes. Curr. Biol. 18, 895–899. doi: 10.1016/j.cub.2008.05.037
Vardi, A., Eisenstadt, D., Murik, O., Berman-Frank, I., Zohary, T., Levine, A., et al. (2007). Synchronization of cell death in a dinoflagellate population is mediated by an excreted thiol protease. Environ. Microbiol. 9, 360–369. doi: 10.1111/j.1462-2920.2006.01146.x
Vardi, A., Formiggini, F., Casotti, R., De Martino, A., Ribalet, F., Miralto, A., et al. (2006). A stress surveillance system based on calcium and nitric oxide in marine diatoms. PLoS Biol 4:e60. doi: 10.1371/journal.pbio.0040060
Vardi, A., Haramaty, L., Van Mooy, B. A., Fredricks, H. F., Kimmance, S. A., Larsen, A., et al. (2012). Host-virus dynamics and subcellular controls of cell fate in a natural coccolithophore population. Proc. Nat. Acad. Sci. U.S.A. 109, 19327–19332. doi: 10.1073/pnas.1208895109
Verdugo, P., Alldredge, A. L., Azam, F., Kirchman, D. L., Passow, U., and Santschi, P. H. (2004). The oceanic gel phase: a bridge in the DOM-POM continuum. Mar. Chem. 92, 67–85. doi: 10.1016/j.marchem.2004.06.017
Vlamakis, H., Chai, Y., Beauregard, P., Losick, R., and Kolter, R. (2013). Sticking together: building a biofilm the Bacillus subtilis way. Nat. Rev. Microbiol. 11, 157–168. doi: 10.1038/nrmicro2960
Watnick, P., and Kolter, R. (2000). Biofilm, city of microbes. J Bacteriol. 182, 2675–2679. doi: 10.1128/JB.182.10.2675-2679.2000
Whitchurch, C. B., Tolker-Nielsen, T., Ragas, P. C., and Mattick, J. S. (2002). Extracellular DNA required for bacterial biofilm formation. Science 295:1487. doi: 10.1126/science.295.5559.1487
Wurl, O., and Holmes, M. (2008). The gelatinous nature of the sea-surface microlayer. Mar. Chem. 110, 89–97. doi: 10.1016/j.marchem.2008.02.009
Keywords: phytoplankton, coccolithophore, Emiliania huxleyi, diatoms, multicellularity, programmed cell death, infochemical communication
Citation: Abada A and Segev E (2018) Multicellular Features of Phytoplankton. Front. Mar. Sci. 5:144. doi: 10.3389/fmars.2018.00144
Received: 30 January 2018; Accepted: 10 April 2018;
Published: 24 April 2018.
Edited by:
Matthias Wietz, University of Oldenburg, GermanyReviewed by:
Nick A. Lyons, Harvard Medical School, United StatesMatthew‘ David Herron, University of Montana, United States
Pierre Durand, University of the Witwatersrand, South Africa
Copyright © 2018 Abada and Segev. This is an open-access article distributed under the terms of the Creative Commons Attribution License (CC BY). The use, distribution or reproduction in other forums is permitted, provided the original author(s) and the copyright owner are credited and that the original publication in this journal is cited, in accordance with accepted academic practice. No use, distribution or reproduction is permitted which does not comply with these terms.
*Correspondence: Einat Segev, ZWluYXQuc2VnZXZAd2Vpem1hbm4uYWMuaWw=