- Marine Biological Section, Department of Biology, University of Copenhagen, Copenhagen, Denmark
Biological dinitrogen fixation (BNF) represents a major input of reduced nitrogen (N) to the oceans. Accurate direct measurements of BNF rates are crucial for reliably determining the biogeochemical significance of diazotrophy at local and global scales. Traditionally, borosilicate glass fiber filters (GF/F, Whatman) with a nominal pore size of 0.7 μm are used to collect suspended particles by filtration after incubations with added 15N2 tracer. We carried out BNF experiments in the Baltic Sea, Danish coastal waters, and the Pacific Ocean comparing the retentive characteristics of precombusted GF/F filters with newer Advantec glass fiber filters which have a smaller nominal pore size of 0.3 μm. Where BNF was detected, rates were nearly always higher, and sometimes even exclusively detectable, when using Advantec filters. In the majority of samples across tested habitats, significantly more cells were lost to GF/F filtrate (average = 51%, range = 10–70% of cells) than to Advantec filtrate (average = 40%, range = 10–54%). Using Illumina sequencing of nitrogenase (nifH) gene amplicons, we show that diazotroph communities can markedly differ between bulk water and filtrates from GF/F and Advantec filtrations, suggesting that different diazotrophs can pass through the filter types. In order to reduce the potential underestimations of BNF due to filtration loss of diazotrophs, we recommend using Advantec filters or alternatively silver membranes with 0.2 μm pore size, especially in waters expected to be inhabited by relatively small, unicellular diazotrophs.
Introduction
Biological nitrogen (N2) fixation (BNF) in the pelagic ocean amounts to up to 200 × 1012 g of nitrogen (N) globally per year, and thereby greatly influences oceanic primary production and CO2 sequestration (Capone et al., 2005; Gruber and Galloway, 2008; Canfield et al., 2010). Cyanobacteria in the upper water column of the tropical and subtropical ocean have traditionally been considered to be the only quantitatively important N2 fixers (diazotrophs) (Zehr, 2011). However, nitrogenase (nifH) gene sequencing has uncovered an almost ubiquitous distribution of diverse and presumably heterotrophic diazotrophs (Zehr et al., 1998; Farnelid et al., 2011). Notably, these organisms are even found in high-latitude, deep, cold, or coastal waters where cyanobacteria are few or absent (e.g., Fernandez et al., 2011; Blais et al., 2012; Bonnet et al., 2013) and their lifestyles and how they impact biogeochemical element cycling seems fundamentally different from photoautotrophic diazotrophs (Rahav et al., 2013; Farnelid et al., 2014; Moisander et al., 2014; Benavides et al., 2015; Bentzon-Tilia et al., 2015a). This has inspired many investigators to hypothesize that global N inputs by BNF could potentially be much higher than previously thought. However, the ecology and actual contribution of heterotrophic diazotrophs to oceanic BNF is still largely elusive (Bombar et al., 2016).
Aside from geochemical approaches which can help to quantify BNF on the scale of ocean basins (Gruber and Sarmiento, 1997; Deutsch et al., 2007), direct rate measurements are an important and widely used tool for studying the ecology and biogeochemical impact of diazotrophs. The most commonly applied method for obtaining BNF rate measurements involves adding 15N2 tracer gas to water samples followed by filtration of suspended plankton and measurement of 15N tracer incorporation into their biomass, typically after 24 h of incubation (Montoya et al., 1996; Luo et al., 2012). However, recent studies show that slow equilibration between the added 15N2 gas and the water sample can lead to an underestimation of rates, requiring a modified protocol in which the gas is pre-dissolved in aliquots of water (Mohr et al., 2010; Grosskopf et al., 2012). Further, some widely used commercially available 15N2 gas stocks were shown to be contaminated with 15N- labeled nitrate and ammonium, placing doubt on measurements for which the purity of the used gas stock is not explicitly confirmed (Dabundo et al., 2014). Regrettably, to date there is no clear consensus about how the 15N2 enriched seawater should be prepared, how trace metal and other contamination can be avoided, and how incubations should best be carried out (Wilson et al., 2012; Bentzon-Tilia et al., 2015a; Klawonn et al., 2015), leaving it somewhat unclear whether rates from different studies are really comparable and whether observed magnitudes of BNF are accurate.
Clearly, an optimized and accorded protocol for how to best carry out BNF measurements is much needed. In this context, we believe that an additional potential source of error in BNF rate measurements needs to be considered, namely the traditional use of glass fiber filters (Whatman GF/F®) with a nominal pore size of 0.7 μm in post-incubation filtrations. It has long been known that significant shares of recently fixed N can be exuded from diazotroph cells as ammonium or dissolved organic nitrogen (DON), giving the obtained BNF rates the operational definition of a “net rate” (Glibert and Bronk, 1994; Mulholland et al., 2004; Berthelot et al., 2017). However, if actual diazotroph cells would also be lost to the filtrate during filtration, as suspected by other investigators (Konno et al., 2010), the resulting BNF rates would simply be operational underestimates. This kind of loss would arguably affect heterotrophic diazotrophs from the picoplankton size class (0.2–2 μm diameter) more than e.g., large Trichodesmium colonies, which can be up to 5 mm in length. Although investigators have tested the performance of different filters for pigment and productivity measurements (Søndergaard and Middelboe, 1993; Moran et al., 1999; Nayar and Chou, 2003 and references therein) and of GF/F filters for retention of bacterioplankton (Lee et al., 1995), there is no assessment of how specific functional groups such as different diazotroph species are retained by GF/F filters. In fall 2013 we carried out an initial test incubation in the Sargasso Sea in which we compared BNF rates obtained from filtering incubations over GF/F filters (0.7 μm pore size) with replicates filtered over different filters (Advantec® glass fiber filters, 0.3 μm pore size), with the surprising result that the “Advantec rates” were twice as high as those obtained from GF/F filters (2.95 ± 0.53 nmol N L−1 d−1 vs. 1.4 ± 0.17 nmol N L−1 d−1, respectively; n = 3; Dziallas and Severin, unpublished). These results motivated the current study, in which we tested the overall retentive characteristics of these filters and whether BNF rate differences between them would be reproducible in contrasting marine environments. Further, we identified diazotrophs in bulk water and in cells passing through to the filtrates by Illumina amplicon sequencing of nifH genes. In the light of our results we aim to provide information and guidance on choice of filters associated with the 15N2 tracer assay in order to obtain better measurements of BNF rates in the future.
Materials and Methods
Sampling Overview and BNF Rate Experiments
We carried out BNF experiments with natural seawater to determine potential differences in the retentive characteristics of conventional glass fiber filters (“GF/F”, 0.7 μm nominal pore size, Whatman®) and Advantec ® filters (Advantec, GF75, 25 mm diameter, Toyo Roshi Kaisha, Japan via Sterlitech Corp.) with a smaller nominal pore size (0.3 μm), and to test whether these differences in pore size would affect resulting rates of BNF. Experiments were carried out with water from three different marine sites between 2013 and 2015, in order to assess whether the retentive characteristics of the filters would differ among fundamentally different diazotroph communities (Supplemental Table S1). One station in the narrow Strait of Øresund between Denmark and Sweden (55.974 N, 12.694 E) was sampled on 28. April 2015, and experiments were carried out with water from the surface (originating from the Baltic Sea, salinity 11) as well as from 25 m depth, below the halocline (originating from Skagerrak, salinity 33). Between March and May 2015, one experiment per month was carried out with surface water from several stations along Roskilde Fjord (Denmark), which is a eutrophic estuary with a steep trophic and salinity gradient (inner buoy, salinity 14; outer buoy, salinity 20; boundary station, salinity 22; Supplemental Table S1). Previous sampling at the inner buoy showed that BNF peaked in spring and diverse heterotrophic and photoheterotrophic diazotrophs were present and active (Bentzon-Tilia et al., 2015a). Lastly, in February 2015 experiments were carried out with water from 100, 50 m, and the surface at one station off Baja California in the subtropical Pacific (23.0 N, −111.253 W).
In all experiments, BNF rates were measured using the 15N2 tracer approach (Montoya et al., 1996). 15N2 tracer gas was pre-dissolved in artificial seawater (Boström et al., 2007); adjusted to local salinities), using methods modified after Mohr et al. (2010). Following sterile filtration, the artificial seawater was degassed for 1 h by heating (50°C), magnet stirring, and applying vacuum (approximately 950 mbar below atmospheric pressure). Thereafter, the water was quickly distributed bubble-free into 50 mL borosilicate serum vials, which were immediately crimp-sealed using sterile butyl rubber septa. One mL of 15N2 tracer gas (Campro Scientific, Veenendaal, The Netherlands; 98% enrichment of 15N) was then injected through the septum into each vial using a gas-tight syringe, and equilibration of the gas in the artificial seawater was achieved by shaking overnight (150 r.p.m) and subsequent storage of inverted vials at 5°C for at least 48 h. In order to avoid potential contamination with trace elements in this degassing protocol, all materials, bottles and tubings were acid-washed prior to use. At each experimental site, triplicate acid-washed and MilliQ-rinsed 1.2 L polycarbonate bottles were rinsed three times with local seawater and then filled close to capacity. We then quickly added one vial of 15N2 enriched water before immediately closing the bottles with screw caps to obtain a theoretical initial N2 substrate label of approximately 10 atom% 15N. Bottles were incubated under simulated in situ conditions including flowing surface seawater for cooling and neutral density screening to approximately mimic light levels at the surface or at particular depths (i.e., 75% of sea surface irradiance for Øresund or Roskilde Fjord surface samples, 5% at 25 m in Øresund, and 75, 1, and 0% for 5, 53, and 100 m samples in the Pacific). Incubations were terminated after 24 h by gentle vacuum filtration (≤25 cm Hg) onto 25 mm pre-combusted (450°C, 5 h) GF/F or Advantec filters (Figure 1). Suspended material in bulk water as well as in filtrate from both types of filters was filtered onto 0.2 μm pore size, 47 mm diameter Supor membranes (Pall Corporation) to collect biomass for DNA extraction in order to analyze diazotroph communities via nifH gene sequencing (see below). Further aliquots of bulk water and filtrate were also preserved for flow cytometric abundance estimations and qualitative microscopic analysis (see below).
All glass fiber filters were dried at 60°C for 12 h and packed in tin cups (IVA, Meerbusch, Germany). Filters were analyzed by isotope ratio mass spectrometry for 15N and 14N at the Laboratory of Applied Physical Chemistry, Gent, Belgium, including empty tin cups, blank filters (duplicates of each for every sampling campaign) and time-zero samples (no 15N2 added) from each replicate at every station/depth, to obtain natural abundance δ15N measurements of suspended particulate N. BNF rates were calculated following Montoya et al. (1996), and detectable activity was defined as when the difference between δ15N in the incubated and initial samples was >3x the standard deviation among all time-zero samples at a given station/depth.
Microscopy and Flow Cytometry
For bacterial enumeration, triplicate bulk and filtrate 2 mL samples were fixed with glutaraldehyde (1% final) and stored at −80°C. After defrosting, samples were stained with SYBR green nucleic acid gel stain I (1% final concentration) and analyzed on a FACSCanto II flow cytometer (BD Biosciences, Franklin Lakes, NJ, USA) (Gasol and Del Giorgio, 2000; Supplemental Figure S1). Fluorescent beads (True count beads, BD Biosciences, Albertslund, Denmark) were used to calibrate the flow rate.
Microscopy was exclusively used for qualitative analysis of samples, i.e., to check for the presence of particles, cell aggregates, etc. in bulk samples and filtrates. Fixed subsamples (formaldehyde 1% final) from experiments were filtered onto black polycarbonate filters (0.2 μm pore size; 25 mm diameter; Whatman) and stained for 2 min with 4′,6-diamidino-2-phenylindole (DAPI; 0.01 mg ml−1). Samples were examined at 1000x magnification under a Zeiss Axioskop 2 mot plus epifluorescence microscope (Carl Zeiss, Jena, Germany) using filter set U-FUW (Olympus Co., Japan).
DNA Extractions, Polymerase Chain Reaction (PCR) and nifH Illumina Sequencing
DNA was extracted using a phenol/chloroform protocol (Boström et al., 2004), and quantified using PicoGreen (Molecular Probes, Invitrogen, Eugene, OR, USA). nifH gene fragments (359 bp) were amplified by nested PCR using degenerate primers (Zehr and Mcreynolds, 1989; Zani et al., 2000). PCR reactions were prepared in a sterile workflow bench after 30 min of UV treatment, and DNA templates were added in a separate bench. PCR amplifications were carried out in 25 μL reactions using Pure Taq Ready-To-Go PCR Beads (GE Healthcare). The first round of PCR was carried out with the nifH3 and nifH4 primers (Zehr and Turner, 2001) followed by another 30-cycle-PCR in triplicate for each sample, using 1 μL PCR product from the first round as template, and custom Illumina primers consisting of gene-specific sites nifH1 and nifH2 (Zehr and Turner, 2001) dual-indexed, sample specific barcodes, and four random nucleotides at the start (Supplementary Table 2). Extracted DNA from a marine diazotroph isolate BAL286 (AY97287; Boström et al., 2007) served as positive control. All reactions were cycled at 95°C for 3 min, followed by 30 cycles of 95°C for 45 s, 54°C for 1 min, 72°C for 1:30 min, and a final elongation step of 72°C for 7 min.
Amplicons from triplicate PCR reactions from each sample were pooled, cleaned (Agencourt AMPure XP kit; Beckman Coulter, Indianapolis, USA), and quantified (PicoGreen). All samples were then pooled in equimolar amounts (approximately 10 ng DNA per sample). Negative control PCR reactions were included in the sequencing pool despite the absence of visual gel bands after amplification. Library preparation and sequencing were performed at NGI Sweden (Royal Institute of Technology) with Illumina Truseq PCR-free library preparation and the MiSeq v2 2 × 250 bp sequencing protocol. Sequences were uploaded to the Sequencing Read Archive (SRA) database on NCBI (Accession number SRP125896). Sequence reads were trimmed, merged, quality-checked (scores <20 eliminated) and demultiplexed following Qiime protocols (Caporaso et al., 2010). Further processing and quality control was performed using Mothur (Schloss et al., 2009), discarding reads with ambiguities and homopolymers (>8 bp). Operational taxonomic units (OTUs) were clustered at 97% nucleotide sequence similarity, and a de novo chimera check was performed using Uchime (Edgar et al., 2011). OTUs containing chimeras, frameshifts, and non-nifH sequences were removed. For non-metric multidimensional scaling (NMDS) plots, rarefied OTU tables were used by subsampling to 2,000 reads per sample, and Bray Curtis similarity matrixes were calculated and designed using PRIMER 6 (Clarke and Gorley, 2006).
Statistics
Significant differences in BNF rates and filtrate bacterial abundances between GF/F and Advantec were detected by means of t-tests (SigmaPlot 12.5, Systat Software Inc.). To reveal differences in nifH community composition among bulk and filtrate samples, Bray–Curtis similarities were calculated in PRIMER 6.1.15 based on rarefied (n = 2,000) nifH genes and visualized by non-metric multidimensional scaling (NMDS). Permutational multivariate analysis of variance (PERMANOVA) was carried out to test for significant differences of nifH communities among bulk samples and GF/F and Advantec filtrates.
Results and Discussion
Different Retention Characteristics of GF/F and Advantec Filters
Recent attempts to improve the 15N2 tracer assays for BNF measurements (Mohr et al., 2010; Dabundo et al., 2014; Klawonn et al., 2015) reemphasize that the goal of this methodology must be to obtain accurate BNF rates which are comparable between fundamentally different habitats and can be used for N budget calculations (Montoya et al., 2004; Voss et al., 2004; Capone et al., 2005; Benavides et al., 2015). Overcoming the methodological biases of 15N2 gas dissolution and dissolved inorganic 15N contamination are important steps, but they can only lead to an acceptably error-free method if other significant biases are excluded. Oceanic diazotrophs can be found in a range of sizes and exhibit different lifestyles. They encompass millimeter-sized, filamentous cyanobacteria, as well as auto- and heterotrophic prokaryotes falling into the picoplankton size class (0.2–2 μm), which includes key players such as the cyanobacterial symbiont “UCYN-A” (Farnelid et al., 2010; Thompson et al., 2012). The present study shows that glass fiber filters indeed do not catch the entire diazotroph community and therefore might commonly lead to underestimates of BNF rates. Thus, amendments to the filtration step of BNF experimental assays seem necessary.
Our assessment of the retentive characteristics of precombusted GF/F and Advantec filters in three different marine environments shows for both filter types that large shares of total bacteria can be lost to the filtrate (Figure 2). These results are similar to earlier tests of un-combusted GF/F filters (Lee et al., 1995; up to around 50% of total bacterioplankton cells lost to the filtrate). However, Advantec filters overall performed better than GF/F, as shown by significantly fewer cells passing through Advantec filters in 6 out of 12 samples across all tested habitats (Figure 2) and by the overall averages for all samples (for GF/F: average = 51%, range = 10–70% of cells; and for Advantec filters: average = 40%, range = 10–54%). Differences in cell retention between GF/F and Advantec filters varied across different samples, which is most clear for different depths at the Øresund and the Pacific stations. This suggests that the filtration bias varies depending on what kind of plankton/bacterial community is present, and also on whether, for example, lots of suspended debris or sediment is present. For example, microscopy showed that in the Øresund the sample from below the halocline was characterized by abundant, large particles densely colonized by bacteria, which probably explains why they were retained comparably on both filter types. At 2 m depth, such particles were scarce, and here bacterial abundances were clearly higher in the GF/F filtrate (Figure 2). In rare cases cells larger than the nominal pore size of glass fiber filters were found in the filtrates (Figure 2, Supplemental Figures S1 D–F), suggesting that they can somehow break through the pores at times. This is likely related to the matrix structure of overlapping glass fibers in these filters, which form pores of nominal size, in contrast to absolute-rated filters having pores with a defined shape and size. Interestingly, Lee et al. (1995) observed that once-retained particles can get lost to GF/F filtrate when filtering large volumes of seawater (several liters, as is rather common for studies in oligotrophic waters).Taken together, our data suggest that while both filters are suboptimal for retaining all bacteria, Advantec filters retain comparatively more cells and thus appear to be the better choice for BNF assays. Optimally, measurements of isotope signatures such as in the 15N2 assay would be carried out by using 0.2 μm pore size silver membrane filters, but these carry a significantly increased cost considering their price (e.g., Sterlitech, approximately $6.90 per filter vs. $0.6 per filter for glass fiber filters) and the common requirement for replicate filters across multiple sampling stations, depths, or experimental time points.
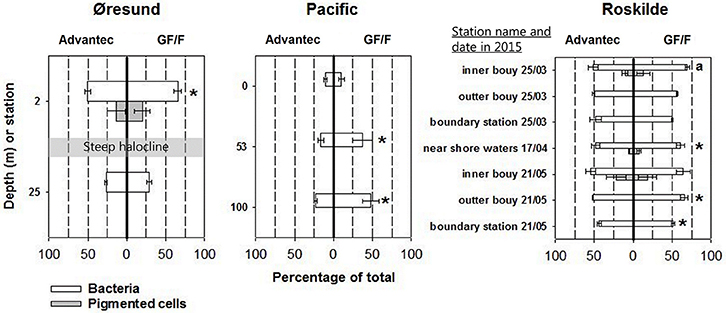
Figure 2. Cell retention characteristics of Advantec and GF/F filters, illustrated as the percentages of cells found in filtrates relative to the cell abundances in bulk water samples (averages ± standard deviation, n = 3). The actual cell abundances can be found in Supplemental Table S1. Where gray bars are lacking, pigmented cells (which included some cells larger than the nominal pore size of the glass fiber filters) were completely retained by the filters or too low in abundance for reliable quantification. Cases where abundances were significantly different between the two filtrates are marked with asterisks (t-test, p < 0.05). In Øresund, the halocline separates surface waters with a salinity of 11 and deep waters with a salinity of 33. For the inner buoy sample on 25th March 2015 (marked with an a) statistical differences could not be calculated since only two replicates were available for flow cytometry.
Our finding that precombusted glass fiber filters fail to retain significant numbers of bacterioplankton is puzzling in the light of earlier claims of precombusted GF/F filters performing comparably as 0.2 μm pore size membrane filters in productivity and pigment measurements (Chavez et al., 1995; Nayar and Chou, 2003). While Chavez et al. (1995) focused solely on Chlorophyll a containing cells (prochlorophytes with 0.54–0.67 μm equivalent spherical diameter) and primary production measurements, Nayar and Chou (2003) used scanning electron microscopy and showed that the borosilicate glass microfibers get compacted during combustion, which effectively downsizes the nominal pore size. The only obvious explanation for the discrepancy to our results is that Nayar and Chou (2003) combusted their filters at 600°C for 1 h, while most studies carrying out BNF measurements (including our present one) combusted filters at 450°C for 2–12 h (e.g., Montoya et al., 1996; Capone et al., 2005; White et al., 2007; Farnelid et al., 2013; Shiozaki et al., 2017). While the lower temperature of 450°C is enough to eliminate carbon or nitrogen contamination from filters, it apparently compacts the filter fiber structure less than at 600°C. Manufacturers advise maximum temperatures of 500°C for borosilicate membranes, and Nayar and Chou (2003) observed melting of the filters when exposing them to >600°C or to longer durations of combustion. Thus, combustion like described by Nayar and Chou (2003) can potentially further reduce the large bacterial cell loss during filtration and should be more widely adapted for measuring bacterial process rates. The current application of only 450°C together with highly variable combustion times in BNF studies adds to the uncertainty about which effective pore sizes may have actually been applied in published BNF studies.
Some investigators opt for not precombusting glass fiber filters prior to using them in BNF experiments (e.g., Fernández et al., 2010). This is due to the concern that the effective combustion temperature could vary for different filters inside the furnace (depending e.g., on how they are positioned and how close they are to the walls), and due to the belief that contamination of purchased filters is negligible and that the additional handling associated with precombustion could add further contamination. While these concerns are valid, we believe that precombustion is an essential part of the BNF protocol. In our experience, significant trace contamination of filters can exist, and thus using uncombusted filters would introduce additional uncertainty about obtained BNF rates, given the mentioned potential contamination of 15N2 gas stocks (Dabundo et al., 2014) and the difficulties with generating contamination-free 15N2 enriched seawater (Klawonn et al., 2015). Further, as mentioned, the effects of combustion on the filter matrix structure seem to be desirable since they increase the retention capacity of the filters (Nayar and Chou, 2003). However, a consensus protocol of preparing and carrying out BNF measurements should include reviewed instructions on how to correctly combust glass fiber filters, including recommendations on combustion times, temperatures, and positioning of the filters inside the furnace.
The Effect of Different Retention by GF/F and Advantec Filters on Obtained BNF Rates
Apart from the initial filter test in the Sargasso Sea (2.95 ± 0.53 nmol N L−1 d−1 for Advantec vs. 1.4 ± 0.17 nmol N L−1 d−1 for GF/F) all measured BNF rates in this study were below 2 nmol N L−1 d−1, falling into the lower range of volumetric BNF measured in oceanic waters (Luo et al., 2012). At the station in the Pacific, BNF even remained undetectable. The low rates in Øresund and Roskilde (Figure 3) are possibly explained by the abiotic conditions encountered, including rather low temperatures measured throughout our samplings, peaking at around sampling in Roskilde Fjord. Such temperatures are below what is traditionally diazotroph growth, even for temperate cyanobacterial species like Aphanizomenon flos aquae or Anabaena spp. (Laamanen and Kuosa, 2005; Sohm et al., 2011). However, not much is known about temperature constraints on field populations of non-cyanobacterial diazotrophs. BNF rates for Roskilde Fjord were overall much lower in 2015 than in 2012 (Bentzon-Tilia et al., 2015a) although water temperatures were comparable, pointing to a high year-to-year variability. It could be speculated that our low BNF rates falsely represent uptake of 15NH4 contaminant (Dabundo et al., 2014) by small bacteria which were subsequently retained better by Advantec filters. However, in this case it would be difficult to explain why BNF remained completely undetectable in some samples, and more importantly, our batch of 15N2 gas from Cambridge Isotopes (lot # I-16727) was described to contain only minute levels of contaminant, theoretically triggering maximal “false” rates of ca. 0.02 nmol N L−1 d−1 (Dabundo et al., 2014). We therefore believe that our measurements represent true diazotroph activity. diazotroph activity.
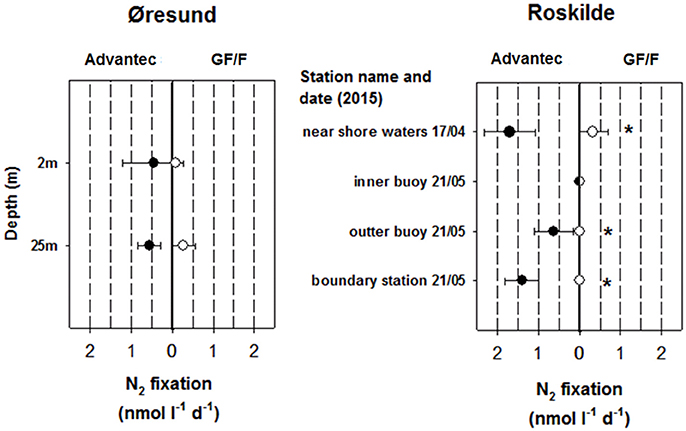
Figure 3. N2 fixation rates obtained from using Advantec or GF/F filters in Øresund and Roskilde Fjord (averages ± standard deviation, n = 3). N2 fixation was undetectable in all Pacific samples and therefore a graph is not shown for that habitat. For Roskilde Fjord, N2 fixation was not measured in March 2015. Cases where rates were significantly different between the two filter types are marked with asterisks (t-test, p < 0.05). In Øresund, the halocline separates surface waters with a salinity of 11 and deep waters with a salinity of 33.
Importantly, in most cases where we detected BNF (including the initial test in the Sargasso Sea), rates were higher when using Advantec filters compared to GF/F filters, and in two cases rates remained undetectable with GF/F filters while they were detectable in Advantec replicates (Roskilde Fjord outer—and boundary station, May 2015; Figure 3). In all cases where BNF rates were significantly higher in Advantec replicates, we also found significantly lower bacterial abundances in Advantec filtrates (Figure 2 vs. Figure 3), suggesting that cells responsible for BNF were lost via GF/F filtration but not via filtration over Advantec. Taken together, our data suggest that by using Advantec filters, detection of low BNF rates is more likely. This finding is important because BNF rates in mesopelagic waters and other “unusual” habitats are often equal to or even lower than our rates (Fernandez et al., 2011; Bonnet et al., 2013; Dekaezemacker et al., 2013; Rahav et al., 2013; Benavides et al., 2015; Löscher et al., 2016). Consequently, it would be desirable to test whether rates determined for these environments (and the biogeochemical significance derived from such rates) are underestimates due to the use of 0.7 μm pore size GF/F filters.
Diazotroph Community Composition in Bulk Samples and Filtrates of GF/F and Advantec Filters
In order to analyze and compare the presence and composition of diazotrophs in bulk and filtrate material, we attempted to PCR-amplify the nifH gene from a total of 62 samples (36 GF/F /Advantec filtrates, 25 bulk samples, plus one blank sample). nifH was successfully amplified from 58 samples, except for one bulk sample from 5 m at the Pacific station, a bulk sample from outer buoy in March 2015, and both filtrate samples at outer buoy in May 2015. The successful amplification in most filtrate samples suggests that across different habitats, glass fiber filters do not catch the entire diazotroph community.
The Illumina sequencing yielded a total of 1,489,321 reads clustering into 464 OTUs after quality control and deletion of OTUs with <100 reads across all samples or OTUs that occurred in <2 per sample ranged from 554 to 89,532, with an average of 25,678. The blank sample did not return any nifH sequences. Sequences accounting for at least 1% of reads in a single sample comprised 89 OTUs and were mainly affiliated with diverse Proteobacteria and a few Cyanobacteria in nifH cluster I, as well as putatively anaerobic prokaryotes in nifH cluster III (Zehr et al., 2003) (Supplemental Figure S2). As in every study sequencing nifH genes, some of the retrieved phylotypes potentially are contaminants, which needs to be kept in mind especially for novel sequences lacking closely related, cultured representatives. The most common OTU_1 was most closely related to Paenibacillus wynnii sp., a facultatively anaerobic bacterium isolated from Alexander Island, Antarctica (Rodríguez-Díaz et al., 2005). However, OTU_2 was closely related to a sequence retrieved from bottled mineral water (França et al., 2016) and was found across the different habitats, indicating that it was possibly a contaminant. In the following discussion on retentive characteristics of the filters, we therefore focus on well-studied phylotypes which undoubtedly are of marine origin. Further, to reduce complexity, from here we mainly focus on bulk and filtrate samples for which BNF was measured.
The nifH community composition differed significantly between habitats (Figure 4A; PERMANOVA p = 0.001), offering the opportunity to test whether retentive characteristics of GF/F and Advantec filters would vary depending on the composition of diazotrophs. We mainly compare the types of diazotrophs detected in bulk and filtrate samples in a qualitative way, since Illumina sequencing of PCR amplicons does not yield truly quantitative information due to biases such as preferential amplification of some phylotypes (e.g., Turk et al., 2011). As shown in Figures 4B–D, nifH communities often differed between bulk- and filtrate samples, and between GF/F—and Advantec filtrate samples, although these differences were not significant overall (PERMANOVA, p = 0.48 for Øresund; p = 0.17 for Pacific samples, and p = 0.26 for Roskilde Fjord samples). While amplicon sequencing can hardly be used to infer how well the different filters retained specific diazotrophs, our data suggest that only parts of the diazotroph community potentially pass through the filters, and that there can be differences in composition between diazotrophs passing through GF/F and Advantec filters, respectively.
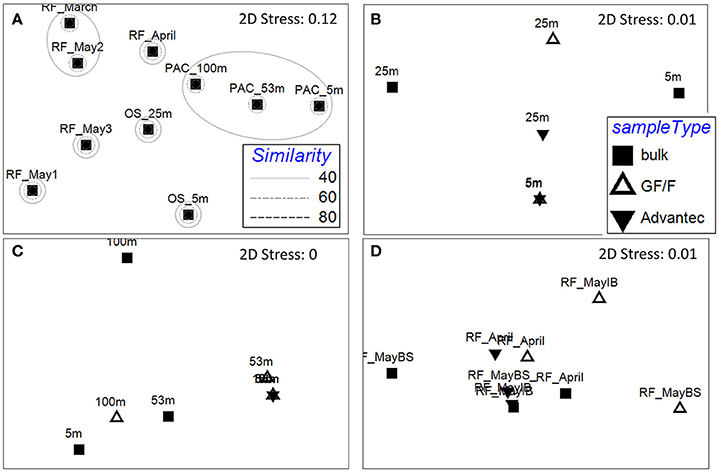
Figure 4. Non-metric multidimensional scaling (NMDS) plots of the diazotroph community composition based on rarefied (n = 2,000) nifH genes, calculated by Bray-Curtis similarity. (A) Resemblance between bulk samples at the different habitats. Rings mark different similarities (%) between samples, as shown in the legend. RF: Roskilde Fjord; OS: Øresund; PAC: Pacific. B–D) Resemblance between bulk and filtrate samples in samples from Øresund, Pacific, and Roskilde Fjord, respectively. IB, Inner Buoy; BS, Boundary Station.
An important example are the few cyanobacterial OTUs found in this study (green cluster in Supplemental Figure S2), which were exclusively retrieved from bulk samples (screening all ≥1% OTUs of all 58 successfully amplified samples), except for OTU_4, which had 99% nucleotide sequence similarity to Candidatus Atelocyanobacterium thalassa (“UCYN-A1”). OTU_4 was only recovered in the Pacific, even though Bentzon-Tilia et al. (2015b) previously found a close relative (UCYN-A2) to be an active diazotroph in Roskilde Fjord. UCYN-A is a symbiotic unicellular cyanobacterium that lives in a fragile association with its host (Thompson et al., 2012), and this small (1–2 μm diameter) diazotroph was also clearly detected in GF/F filtrate at 100 m, although not at the shallower depths (Figure 5). This example documents that small, unicellular diazotroph key players can get lost to filtrate in BNF assays. In contrast, cyanobacterial OTU_10 (Hyella sp.) and OTU_52 (Cyanothece sp.) only occurred in bulk samples. This is likely explained by the morphology and size of these organisms, as Hyella sp. is a pseudofilamentous, slime-generating and relatively large (10s of μm long colonies) cyanobacterium (Brito et al., 2017), and Cyanothece sp. is a larger unicellular cyanobacterium (≥3 μm diameter) which sometimes also forms aggregates (Reddy et al., 1993). OTU_18 is a noteworthy example for a heterotrophic diazotroph found in filtrate. This phylotype is closely related to a Pseudomonas stutzeri (BAL376/BAL410) isolated from Baltic Sea surface waters (Farnelid et al., 2014) and is thought to be able to fix N2 in oxygenated water by forming aggregates. These Pseudomonas types are likely important diazotrophs in the Baltic Sea and Danish coastal waters, and it is notable that we also detected OTU_18 in GF/F filtrate in Roskilde Fjord (Figure 5). Together, our results suggest that the morphology and life style of different diazotrophs influence whether they get lost to glass fiber filtrate or not.
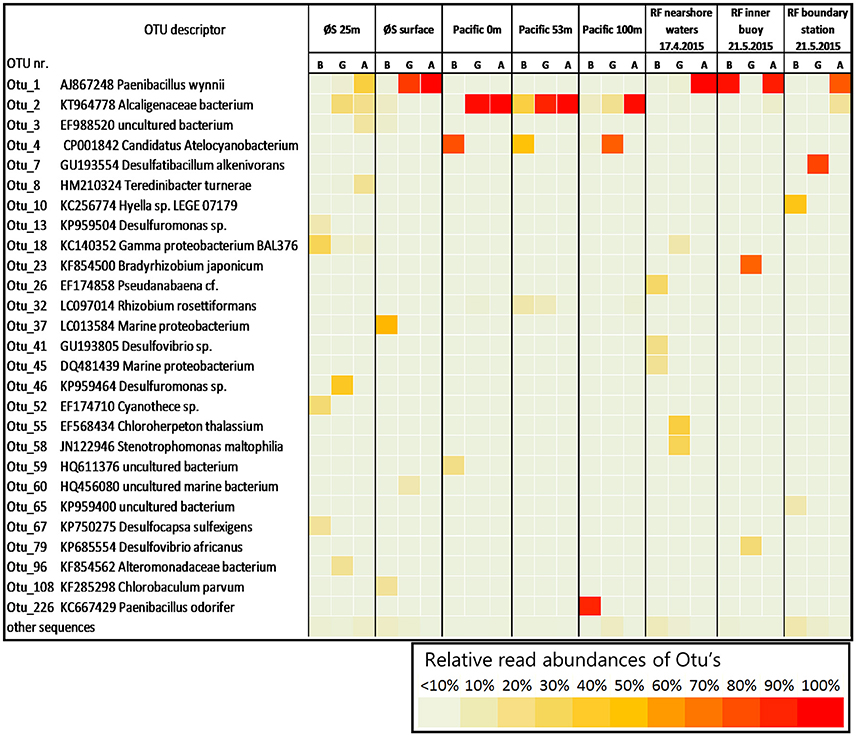
Figure 5. Relative read abundances (in %) of operational taxonomic units (OTU's) in bulk water and filtrates for the 24 samples in which BNF was measured. For clarity, only OTUs accounting for ≥10% of reads in a single sample are shown. Abbreviations B, G, and A refer to bulk, GF/F, and Advantec samples, respectively. The description lists accession numbers of closest BLAST hits and, where available, organism names of closest cultured or genome-sequenced organisms.
Conclusions
The experiments carried out in this study document the significant loss of bacterial cells to filtrates of both GF/F and Advantec filters, but with the latter performing clearly better in retaining cells. While such losses have been described previously, with little or no effect on productivity or pigment measurements, we show that better retention by Advantec filters can at times result in higher BNF rates compared to GF/F filters. It is important to note that although we sampled multiple locations, our dataset is small and detected rates were rather low. We therefore explicitly encourage other investigators to test Advantec filters in 15N2 tracer assays for other areas, including “hotspots” of diazotrophy.
Advantec filters seem generally advisable for BNF measurements, and especially in habitats expected to host small and/or heterotrophic diazotrophs fixing N2 at low rates. Further, BNF activity of cells filtered onto GF/F is often compared with relative or absolute (qPCR determined) abundances of certain nifH phylotypes analyzed from nucleic acid samples filtered onto 0.2 μm pore size membrane filters. Our data shows that nifH containing organisms pass through the glass fiber filters, apparently including organisms with significant contributions to BNF, and thus it is advisable to use Advantec or even silver membrane filters in 15N2 assays in order to obtain better comparable data. In general, our results highlight the importance of the filtration step in BNF measurements and suggest that choosing filters with < 0.7 μm pore size helps to gain more inclusive measurements of diazotroph activity in aquatic ecosystems.
Author Contributions
DB designed and did the research, wrote the paper. RP did part of the experiments, wrote the paper. RA did the flow cytometry, wrote the paper. LR helped with designing the idea and experiments, wrote the paper.
Funding
This work resulted from the BONUS BLUEPRINT project supported by BONUS (Art 185), funded jointly by the EU and the Danish Agency for Science, Technology and Innovation.
Conflict of Interest Statement
The authors declare that the research was conducted in the absence of any commercial or financial relationships that could be construed as a potential conflict of interest.
Acknowledgments
We thank Eero Asmala, Hans Jakobsen, and Jacob Carstensen for providing ship time and for assistance in sampling in Roskilde Fjord, as well as Francisco Chavez, Jason Smith, and the crew of the R/V Western Flyer for ship-time and assistance with experimental incubations in the Pacific. Claudia Dziallas and Ina Severin are acknowledged for providing data from the initial filter comparison in the Sargasso Sea, and Mar Benavides for proofreading the manuscript.
Supplementary Material
The Supplementary Material for this article can be found online at: https://www.frontiersin.org/articles/10.3389/fmars.2018.00006/full#supplementary-material
References
Benavides, M., Moisander, P. H., Berthelot, H., Dittmar, T., Grosso, O., and Bonnet, S. (2015). Mesopelagic N2 fixation related to organic matter composition in the solomon and Bismarck Seas (Southwest Pacific). PLoS ONE 10:e0143775. doi: 10.1371/journal.pone.0143775
Bentzon-Tilia, M., Severin, I., Hansen, L. H., and Riemann, L. (2015b). Genomics and ecophysiology of heterotrophic nitrogen-fixing bacteria isolated from estuarine surface water. mBio 6:e00929. doi: 10.1128/mBio.00929-15
Bentzon-Tilia, M., Traving, S. J., Mantikci, M., Knudsen-Leerbeck, H., Hansen, J. L. S., Markager, S., et al. (2015a). Significant N2 fixation by heterotrophs, photoheterotrophs and heterocystous cyanobacteria in two temperate estuaries. ISME J. 9, 273–285. doi: 10.1038/ismej.2014.119
Berthelot, H., Benavides, M., Moisander, P. H., Grosso, O., and Bonnet, S. (2017). High-nitrogen fixation rates in the particulate and dissolved pools in the Western Tropical Pacific (Solomon and Bismarck Seas). Geophys. Res. Lett. 44, 8414–8423. doi: 10.1002/2017GL073856
Blais, M., Tremblay, J.-É., Jungblut, A. D., Gagnon, J., Martin, J., Thaler, M., et al. (2012). Nitrogen fixation and identification of potential diazotrophs in the Canadian Arctic. Global Biogeochem. Cycles 26:GB3022. doi: 10.1029/2011GB004096
Bombar, D., Paerl, R. W., and Riemann, L. (2016). Marine Non-Cyanobacterial Diazotrophs: moving beyond Molecular Detection. Trends Microbiol. 24, 916–927. doi: 10.1016/j.tim.2016.07.002
Bonnet, S., Dekaezemacker, J., Turk-Kubo, K. A., Moutin, T., Hamersley, R. M., Grosso, O., et al. (2013). Aphotic N2 fixation in the Eastern Tropical South Pacific Ocean. PLoS ONE 8:e81265. doi: 10.1371/journal.pone.0081265
Boström, K. H., Riemann, L., Kühl, M., and Hagström, Å. (2007). Isolation and gene quantification of heterotrophic N2-fixing bacterioplankton in the Baltic Sea. Environ. Microbiol. 9, 152–164. doi: 10.1111/j.1462-2920.2006.01124.x
Boström, K. H., Simu, K., Hagström, Å., and Riemann, L. (2004). Optimization of DNA extraction for quantitative marine bacterioplankton community analysis. Limnol. Oceanogr. 2, 365–373. doi: 10.4319/lom.2004.2.365
Brito, Â., Ramos, V., Mota, R., Lima, S., Santos, A., Vieira, J., et al. (2017). Description of new genera and species of marine cyanobacteria from the Portuguese Atlantic coast. Mol. Phylogenet. Evol. 111, 18–34. doi: 10.1016/j.ympev.2017.03.006
Canfield, D. E., Glazer, A. N., and Falkowski, P. G. (2010). The Evolution and Future of Earth's Nitrogen Cycle. Science 330, 192–196. doi: 10.1126/science.1186120
Capone, D. G., Burns, J. A., Montoya, J. P., Subramaniam, A., Mahaffey, C., Gunderson, T., et al. (2005). Nitrogen fixation by Trichodesmium spp.: An important source of new nitrogen to the tropical and subtropical North Atlantic Ocean. Global Biogeochem. Cycles 19:GB2024. doi: 10.1029/2004GB002331
Caporaso, J. G., Kuczynski, J., Stombaugh, J., Bittinger, K., Bushman, F. D., Costello, E. K., et al. (2010). QIIME allows analysis of high-throughput community sequencing data. Nat. Meth. 7, 335–336. doi: 10.1038/nmeth.f.303
Chavez, F. P., Buck, K. R., Bidigare, R. R., Karl, D. M., Hebel, D., Latasa, M., et al. (1995). On the chlorophyll a retention properties of glass-fiber GF/F filters. Limnol. Oceanogr. 40, 428–433. doi: 10.4319/lo.1995.40.2.0428
Dabundo, R., Lehmann, M. F., Treibergs, L., Tobias, C. R., Altabet, M. A., Moisander, P. H., et al. (2014). The Contamination of Commercial 15N2− Gas Stocks with 15N–Labeled Nitrate and Ammonium and Consequences for Nitrogen Fixation Measurements. PLoS ONE 9:e110335. doi: 10.1371/journal.pone.0110335
Dekaezemacker, J., Bonnet, S., Grosso, O., Moutin, T., Bressac, M., and Capone, D. (2013). Evidence of active dinitrogen fixation in surface waters of the eastern tropical South Pacific during El Ni-o and La Ni-a events and evaluation of its potential nutrient controls. Global Biogeochem. Cycles 27, 768–779. doi: 10.1002/gbc.20063
Deutsch, C., Sarmiento, J. L., Sigman, D. M., Gruber, N., and Dunne, J. P. (2007). Spatial coupling of nitrogen inputs and losses in the ocean. Nature 445, 163–167. doi: 10.1038/nature05392
Edgar, R. C., Haas, B. J., Clemente, J. C., Quince, C., and Knight, R. (2011). UCHIME improves sensitivity and speed of chimera detection. Bioinformatics 27, 2194–2200. doi: 10.1093/bioinformatics/btr381
Farnelid, H., Andersson, A. F., Bertilsson, S., Al-Soud, W. A., Hansen, L. H., Sørensen, S., et al. (2011). Nitrogenase gene amplicons from global marine surface waters are dominated by genes of non-cyanobacteria. PLoS ONE 6:e19223. doi: 10.1371/journal.pone.0019223
Farnelid, H., Bentzon-Tilia, M., Andersson, A. F., Bertilsson, S., Jost, G., Labrenz, M., et al. (2013). Active nitrogen-fixing heterotrophic bacteria at and below the chemocline of the central Baltic Sea. ISME J. 7, 1413–1423. doi: 10.1038/ismej.2013.26
Farnelid, H., Harder, J., Bentzon-Tilia, M., and Riemann, L. (2014). Isolation of heterotrophic diazotrophic bacteria from estuarine surface waters. Environ. Microbiol. 16, 3072–3082. doi: 10.1111/1462-2920.12335
Farnelid, H., Tarangkoon, W., Hansen, G., Hansen, P. J., and Riemann, L. (2010). Putative N2-fixing heterotrophic bacteria associated with dinoflagellate–Cyanobacteria consortia in the low-nitrogen Indian Ocean. Aquat. Microb. Ecol. 61, 105–117. doi: 10.3354/ame01440
Fernández, A., Mouri-o-Carballido, B., Bode, A., Varela, M., and Mara-ón, E. (2010). Latitudinal distribution of Trichodesmium spp. and N2 fixation in the Atlantic Ocean. Biogeosciences 7, 3167–3176. doi: 10.5194/bg-7-3167-2010
Fernandez, C., Farías, L., and Ulloa, O. (2011). Nitrogen fixation in denitrified marine waters. PLoS ONE 6:e20539. doi: 10.1371/journal.pone.0020539
França, L., Albuquerque, L., Sánchez, C., Fareleira, P., and Da Costa, M. S. (2016). Ampullimonas aquatilis gen. nov., sp. nov. isolated from bottled mineral water. Int. J. Syst. Evol. Microbiol. 66, 1459–1465. doi: 10.1099/ijsem.0.000903
Gasol, J. M., and Del Giorgio, P. A. (2000). Using flow cytometry for counting natural planktonic bacteria and understanding the structure of planktonic bacterial Communities 64:28. doi: 10.3989/scimar.2000.64n2197
Glibert, P. M., and Bronk, D. A. (1994). Release of dissolved organic nitrogen by marine diazotrophic cyanobacteria Trichodesmium spp. Appl. Environ. Microbiol. 11, 3996–4000.
Großkopf, T., Mohr, W., Baustian, T., Schunck, H., Gill, D., Kuypers, M. M., et al. (2012). Doubling of marine dinitrogen-fixation rates based on direct measurements. Nature 488, 361–364. doi: 10.1038/nature11338
Gruber, N., and Galloway, J. N. (2008). An Earth-system perspective of the global nitrogen cycle. Nature 451, 293–296. doi: 10.1038/nature06592
Gruber, N., and Sarmiento, J. L. (1997). Global patterns of marine nitrogen fixation and denitrification. Global Biogeochem. Cycles 11, 235–266. doi: 10.1029/97GB00077
Klawonn, I., Lavik, G., Böning, P., Marchant, H. K., Dekaezemacker, J., Mohr, W., et al. (2015). Simple approach for the preparation of 15−15N2-enriched water for nitrogen fixation assessments: evaluation, application and recommendations. Front. Microbiol. 6:769. doi: 10.3389/fmicb.2015.00769
Konno, U., Tsunogai, U., Komatsu, D. D., Daita, S., Nakagawa, F., Tsuda, A., et al. (2010). Determination of total N2 fixation rates in the ocean taking into account both the particulate and filtrate fractions. Biogeosciences 7, 2369–2377. doi: 10.5194/bg-7-2369-2010
Laamanen, M., and Kuosa, H. (2005). Annual variability of biomass and heterocysts of the N2-fixing cyanobacterium Aphanizomenon flos-aquae in the Baltic Sea with reference to Anabaena spp. and Nodularia spumigena. Boreal Env. Res. 10, 19–30.
Lee, S., Kang, Y.-C., and Fuhrman, J. A. (1995). Imperfect retention of natural bacterioplankton cells by glass fiber filters. Mar. Ecol. Prog. Ser. 19, 285–290. doi: 10.3354/meps119285
Löscher, C. R., Bourbonnais, A., Dekaezemacker, J., Charoenpong, C. N., Altabet, M. A., Bange, H. W., et al. (2016). N2 fixation in eddies of the eastern tropical South Pacific Ocean. Biogeosciences 13, 2889–2899. doi: 10.5194/bg-13-2889-2016
Luo, Y. W., Doney, S. C., Anderson, L. A., Benavides, M., Berman-Frank, I., Bode, A., et al. (2012). Database of diazotrophs in global ocean: abundance, biomass and nitrogen fixation rates. Earth Syst. Sci. Data 4, 47–73. doi: 10.5194/essd-4-47-2012
Mohr, W., Grosskopf, T., Wallace, D. W. R., and Laroche, J. (2010). Methodological underestimation of oceanic nitrogen fixation rates. PLoS ONE 5:12583. doi: 10.1371/journal.pone.0012583
Moisander, P. H., Serros, T., Paerl, R. W., Beinart, R. A., and Zehr, J. P. (2014). Gammaproteobacterial diazotrophs and nifH gene expression in surface waters of the South Pacific Ocean. ISME J. 8, 1962–1973. doi: 10.1038/ismej.2014.49
Montoya, J. P., Holl, C. M., Zehr, J. P., Hansen, A., Villareal, T. A., and Capone, D. G. (2004). High rates of N2 fixation by unicellular diazotrophs in the oligotrophic Pacific Ocean. Nature 430, 1027–1031. doi: 10.1038/nature02824
Montoya, J. P., Voss, M., Kahler, P., and Capone, D. G. (1996). A simple, high-precision, high-sensitivity tracer assay for N2 fixation. Appl. Environ. Microbiol. 62, 986–993.
Moran, X. a. G., Gasol, J. M., Arin, L., and Estrada, M. (1999). A comparison between glass fiber and membrane filters for the estimation of phytoplankton POC and DOC production. Mar. Ecol. Prog. Ser. 187, 31–41. doi: 10.3354/meps187031
Mulholland, M. R., Bronk, D., and Capone, D. G. (2004). Dinitrogen fixation and release of ammonium and dissolved organic nitrogen by Trichodesmium IMS101. Aquat. Microb. Ecol. 37, 85–94. doi: 10.3354/ame037085
Nayar, S., and Chou, L. M. (2003). Relative efficiencies of different filters in retaining phytoplankton for pigment and productivity studies. Estuar. Coast. Shelf Sci. 58, 241–248. doi: 10.1016/S0272-7714(03)00075-1
Rahav, E., Bar-Zeev, E., Ohayion, S., Elifantz, H., Belkin, N., Herut, B., et al. (2013). Dinitrogen fixation in aphotic oxygenated marine environments. Front. Microbiol. 4:227. doi: 10.3389/fmicb.2013.00227
Reddy, K. J., Haskell, J. B., Sherman, D. M., and Sherman, L. A. (1993). Unicellular, aerobic nitrogen-fixing cyanobacteria of the genus Cyanothece. J. Bacteriol. 175, 1284–1292. doi: 10.1128/jb.175.5.1284-1292.1993
Rodríguez-Díaz, M., Lebbe, L., Rodelas, B., Heyrman, J., De Vos, P., and Logan, N. A. (2005). Paenibacillus wynnii sp. nov., a novel species harbouring the nifH gene, isolated from Alexander Island, Antarctica. Int. J. Syst. Evol. Microbiol. 55, 2093–2099. doi: 10.1099/ijs.0.63395-0
Schloss, P. D., Westcott, S. L., Ryabin, T., Hall, J. R., Hartmann, M., Hollister, E. B., et al. (2009). Introducing mothur: Open-source, platform-independent, community-supported software for describing and comparing microbial communities. Appl. Environ. Microbiol. 75, 7537–7541. doi: 10.1128/AEM.01541-09
Shiozaki, T., Bombar, D., Riemann, L., Hashihama, F., Takeda, S., Yamaguchi, T., et al. (2017). Basin scale variability of active diazotrophs and nitrogen fixation in the North Pacific, from the tropics to the subarctic Bering Sea. Global Biogeochem. Cycles 31, 996–1009. doi: 10.1002/2017GB005681
Sohm, J. A., Webb, E. A., and Capone, D. G. (2011). Emerging patterns of marine nitrogen fixation. Nat. Rev. Microbiol. 9, 499–508. doi: 10.1038/nrmicro2594
Søndergaard, M., and Middelboe, M. (1993). Measurements of particulate organic carbon: a note on the use of glass fiber (GF/F) and Anodisc R filters. Pol. Arch. Hydrobiol. 127, 73–85.
Thompson, A. W., Foster, R. A., Krupke, A., Carter, B. J., Musat, N., Vaulot, D., et al. (2012). Unicellular cyanobacterium symbiotic with a single-celled eukaryotic alga. Science 337, 1546–1550. doi: 10.1126/science.1222700
Turk, K., Rees, A. P., Zehr, J. P., Pereira, N., Swift, P., Shelley, R., et al. (2011). Nitrogen fixation and nitrogenase (nifH) expression in tropical waters of the eastern North Atlantic. ISME J. 5, 1201–1212. doi: 10.1038/ismej.2010.205
Voss, M., Croot, P., Lochte, K., Mills, M., and Peeken, I. (2004). Patterns of nitrogen fixation along 10N in the tropical Atlantic. Geophys. Res. Lett. 31:L23S09. doi: 10.1029/2004GL020127
White, A. E., Prahl, F. G., Letelier, R. M., and Popp, B. N. (2007). Summer surface waters in the Gulf of California: prime habitat for biological N-2 fixation. Global Biogeochem. Cycles 21:GB2017. doi: 10.1029/2006GB002779
Wilson, S. T., Böttjer, D., Church, M. J., and Karl, D. M. (2012). Comparative assessment of nitrogen fixation methodologies, conducted in the oligotrophic North Pacific Ocean. Appl. Environ. Microbiol. 78, 6516–6523. doi: 10.1128/AEM.01146-12
Zani, S., Mellon, M. T., Collier, J. L., and Zehr, J. P. (2000). Expression of nifH genes in natural microbial assemblages in Lake George, NY detected with RT-PCR. Appl. Environ. Microbiol. 66, 3119–3124. doi: 10.1128/AEM.66.7.3119-3124.2000
Zehr, J. P. (2011). Nitrogen fixation by marine cyanobacteria. Trends Microbiol. 19, 162–173. doi: 10.1016/j.tim.2010.12.004
Zehr, J. P., Jenkins, B. D., Short, S. M., and Steward, G. F. (2003). Nitrogenase gene diversity and microbial community structure: a cross-system comparison. Environ. Microbiol. 5, 539–554. doi: 10.1046/j.1462-2920.2003.00451.x
Zehr, J. P., and Mcreynolds, L. A. (1989). Use of degenerate oligonucleotides for amplification of the nifH gene from the marine cyanobacterium Trichodesmium thiebautii. Appl. Environ. Microbiol. 55, 2522–2526.
Zehr, J. P., Mellon, M. T., and Zani, S. (1998). New nitrogen fixing microorganisms detected in oligotrophic oceans by the amplification of nitrogenase (nifH) genes. Appl. Environ. Microbiol. 64, 3444–3450.
Keywords: oceanic nitrogen fixation, diazotrophs, cyanobacteria, glass fiber filter, bias, filtration
Citation: Bombar D, Paerl RW, Anderson R and Riemann L (2018) Filtration via Conventional Glass Fiber Filters in 15N2 Tracer Assays Fails to Capture All Nitrogen-Fixing Prokaryotes. Front. Mar. Sci. 5:6. doi: 10.3389/fmars.2018.00006
Received: 03 October 2017; Accepted: 10 January 2018;
Published: 30 January 2018.
Edited by:
Sophie Rabouille, UMR7093 Laboratoire d'océanographie de Villefranche (LOV), FranceReviewed by:
Ulisse Cardini, Stazione Zoologica Anton Dohrn, ItalyAntonio Bode, Instituto Español de Oceanografía (IEO), Spain
Copyright © 2018 Bombar, Paerl, Anderson and Riemann. This is an open-access article distributed under the terms of the Creative Commons Attribution License (CC BY). The use, distribution or reproduction in other forums is permitted, provided the original author(s) and the copyright owner are credited and that the original publication in this journal is cited, in accordance with accepted academic practice. No use, distribution or reproduction is permitted which does not comply with these terms.
*Correspondence: Deniz Bombar, ZGJvbWJhckBiaW8ua3UuZGs=
†Present Address: Ryan W. Paerl, Marine, Earth, and Atmospheric Sciences Department, NC State University, Raleigh, NC, United States