- 1Climate Change Cluster, University of Technology, Sydney, NSW, Australia
- 2School of Earth Sciences, UWA Oceans Institute and ARC Centre of Excellence for Coral Reef Studies, University of Western Australia, Perth, WA, Australia
- 3Marine Spatial Ecology Lab, School of Biological Sciences, University of Queensland, St Lucia, QLD, Australia
- 4Institut de Recherche pour le Développement, Centre IRD de Nouméa, ENTROPIE, Nouméa, New Caledonia
- 5Coral Reef Research Unit, School of Biological Sciences, University of Essex, Colchester, United Kingdom
Global climate change and localized anthropogenic stressors are driving rapid declines in coral reef health. In vitro experiments have been fundamental in providing insight into how reef organisms will potentially respond to future climates. However, such experiments are inevitably limited in their ability to reproduce the complex interactions that govern reef systems. Studies examining coral communities that already persist under naturally-occurring extreme and marginal physicochemical conditions have therefore become increasingly popular to advance ecosystem scale predictions of future reef form and function, although no single site provides a perfect analog to future reefs. Here we review the current state of knowledge that exists on the distribution of corals in marginal and extreme environments, and geographic sites at the latitudinal extremes of reef growth, as well as a variety of shallow reef systems and reef-neighboring environments (including upwelling and CO2 vent sites). We also conduct a synthesis of the abiotic data that have been collected at these systems, to provide the first collective assessment on the range of extreme conditions under which corals currently persist. We use the review and data synthesis to increase our understanding of the biological and ecological mechanisms that facilitate survival and success under sub-optimal physicochemical conditions. This comprehensive assessment can begin to: (i) highlight the extent of extreme abiotic scenarios under which corals can persist, (ii) explore whether there are commonalities in coral taxa able to persist in such extremes, (iii) provide evidence for key mechanisms required to support survival and/or persistence under sub-optimal environmental conditions, and (iv) evaluate the potential of current sub-optimal coral environments to act as potential refugia under changing environmental conditions. Such a collective approach is critical to better understand the future survival of corals in our changing environment. We finally outline priority areas for future research on extreme and marginal coral environments, and discuss the additional management options they may provide for corals through refuge or by providing genetic stocks of stress tolerant corals to support proactive management strategies.
Introduction
Coral reef ecosystems and the services they provide sustain half a billion people on Earth, but are deteriorating under the effects of climate change (Cinner et al., 2016; Hughes et al., 2017). Accumulation of greenhouse gases in the atmosphere (primarily carbon dioxide, CO2) is resulting in warmer sea surface temperatures (SST) and rising seawater acidity; processes that are predicted to intensify under current climate change projections (van Hooidonk et al., 2014). Even if carbon emissions were to stabilize today, the world's oceans will experience prolonged warming and inevitable change in carbonate chemistry, due to the lag time that oceans re-equilibrate with the atmosphere (IPCC, 2014). Further changes in other physicochemical conditions, such as reduced oxygen levels (Schmidtko et al., 2017), are accompanying ocean warming, creating a complex suite of environmental factors that coral reefs will need to overcome in order to persist into the future. Understanding whether and how corals can physiologically or genetically adjust to keep pace with environmental change, has therefore become a global research priority.
A key challenge for researchers studying how future projected climates will impact reef form and functioning, is to consider the influence of local scale environmental conditions, including natural diel and seasonal fluctuations (Guadayol et al., 2014). It is imperative to understand the ecological limits to coral distributions by resolving their fundamental niche (the range of conditions an organism can physiologically survive and reproduce under in the absence of biotic interactions, Hutchinson, 1959), relative to their realized niche [determined by other biological pressures, such as competition, (Morin and Thuiller, 2009)], thereby addressing the impact of climate stressors on community reorganization in response to the prevailing conditions (Doney et al., 2012). Natural environments where corals live at the periphery of their environmental tolerance, such as CO2 vent sites, have therefore become increasingly popular to study corals free of the constraints imposed by in vitro experimentation. Such natural extreme and marginal environments have conditions close to the perceived thresholds of coral growth (Kleypas et al., 1999), but support corals that have clearly acclimated and/or adapted to more extreme environments (e.g., Palumbi et al., 2014). Thus, an important role of these environments is that they can offer insight into how corals and coral reefs may survive into the future (Perry and Larcombe, 2003), as they are continuously subjected to more extreme environmental conditions through climate change. The insight provided by these environments will depend in part on the dispersal of adults and juveniles, with sessile brooding species more likely to exhibit adaptation to extreme environments over multiple generations, compared to broadcast spawning species that may recruit into the extreme environment as progeny from “parents” in less stressful environments. Ultimately, no single site studied to date provides a “perfect” analog to future environmental conditions projected for coral reefs. However, a collective assessment of such sites can provide essential information on the ability of corals and coral reefs to persist according to different scenarios, in terms of intensity of stressor and/or combination of stressors at play. Until now, no such collective assessment has been undertaken.
Here, we review and synthesize information across a range of extreme and marginal coral environments, to: (i) demonstrate the extent of abiotic scenarios under which corals can persist, (ii) explore whether there are commonalities in coral taxa able to persist in such extremes, (iii) provide evidence for key mechanisms required to support survival and/or persistence under sub-optimal environmental conditions and, (iv) evaluate the potential of current sub-optimal coral environments to act as potential refugia under changing environmental conditions. We undertake a synthesis of the abiotic variability documented for the different extreme environments, to provide a collective assessment of these systems. Finally, we draw on the findings from the review to explore how these systems may support corals tolerant of stress that could be utilized in proactive reef management, and outline key areas for future research.
Materials and Methods
To begin, we review the current state of knowledge of coral environments that are considered marginal or extreme in relation to one or more abiotic factor, notably, seawater pH, temperature, low light (including highly turbid environments), and oxygen. We use case studies of some well-documented sites within each environment to quantify the extreme abiotic conditions experienced and provide examples of the mechanisms that have been reported to support coral survival. We also consider the ability of these different environments to act as potential coral refugia. Refuge environments are areas that maintain favorable conditions being lost elsewhere (sensu Keppel and Wardell-Johnson, 2012). These could vary across temporal scale, with some environments providing short-term refuge against acute stress (e.g., turbid reefs reducing irradiance during a bleaching event) vs. long term chronic changes (e.g., the potential buffering ability of seagrass systems). As coral reefs globally are forced to acclimatize to changing environmental conditions, utilization of refuge environments becomes an important alternative solution to aid survival.
We then conduct a quantitative synthesis of information from 285 studies (Supplementary Table 1) that have considered extreme, marginal or refuge coral environments. To parameterize the literature included in the quantitative synthesis, only studies that considered scleractinian corals or the physicochemical conditions of coral systems were included. Studies were collected for analysis until the 3rd August 2017. The collection of studies was identified from ISI Web of Science database or Google Scholar searching relevant keywords, e.g., the type of extreme coral environments (high-latitudinal, CO2 vent, mangrove system, seagrass, macro-tidal, ojos (low pH springs), upwelling, turbid reefs, mesophotic, deep reefs, hot seas, tide-pools) with the following words: refuge, coral, thermal tolerance, bleaching, ocean acidification. We also searched the literature cited in all studies identified during that search. Review of this current literature quickly demonstrated that there were not enough data available to compare biological metrics (e.g., calcification rates) across these environments, and as such, we used the quantitative synthesis to: (i) identify knowledge gaps, and (ii) compare the range of abiotic conditions corals are currently able to persist under.
To identify knowledge gaps, the work undertaken in each study was grouped into eight categories: community composition, experimentation, health, holobiont community, physicochemical conditions, physiology, population biology and structural properties, each of which had sub-categories that are illustrated in a heat-map (Figure 1). The heat-map is based on the proportion of studies within a given environment that measured any given parameter.
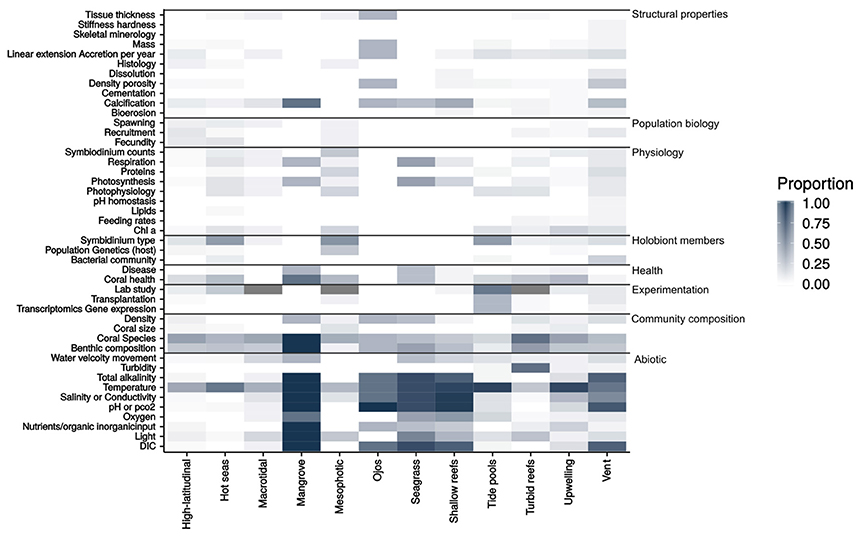
Figure 1. A heat-map showing the research parameters measured within the 285 studies reviewed for the quantitative synthesis. The heat-map is based off the proportion of studies for each environment that researched the given parameter. Number of studies contained in the synthesis per environment: High-latitudinal = 70, Hot seas = 34, Macrotidal = 17, Mangrove = 3, Mesophotic reef = 15, Ojos = 3, Seagrass = 6, Shallow reefs = 21, Tide pools = 21, Turbid reefs = 40, Upwelling = 35, Vent = 20.
To compare the abiotic parameters (pH, temperature, oxygen, total alkalinity (AT) and salinity) of sites within different environments, studies that included the maximum and minimum abiotic values for a minimum of one seasonal period (dry season/wet season) were included. Due to the limited data available across temporal scales (e.g., day, week, month, season, years), data were used as long as a minimum of one diurnal cycle was available for that season. Once additional high-resolution abiotic datasets are available for these environments, comparisons across different temporal scales should be conducted (Table 1). Raw data were used or extracted from primary literature using WebPlot Digitizer (Rohatgi, 2017). Unfortunately, the synthesis quickly revealed that the comparison of multiple abiotic variables across all extreme and marginal coral environments would not be possible due to a lack of data. As such, abiotic comparisons were made across environments on the most commonly reported abiotic variables: pH and temperature. A further Principle Component Analysis (PCA) was conducted on pH (total scale), temperature (°C), total alkalinity (AT; μmol kg−1 SW), salinity and oxygen (mg L−1) for 14 studies, spanning five environments to demonstrate the need to consider multiple abiotic variables within these systems. The PCA was performed in R (R Core Team, 2017) using the prcomp function. To avoid scale change sensitivity in the PCA (Everitt and Hothorn, 2011) variables were rescaled (Härdle and Simar, 2012) to have a mean of zero and a standard deviation of one.
Review of the Current State of Knowledge
Low pH/high CO2 Environments
Absorption of excess atmospheric CO2 by the world's oceans lowers seawater pH and re-organizes the composition of carbonate species of seawater, resulting in reduced saturation states of calcium carbonate minerals [e.g., aragonite (Ωarag)]. This phenomenon, termed ocean acidification, threatens to impact many marine processes (Kroeker et al., 2013) notably through reduced growth and physical integrity of marine calcifiers (Guinotte and Fabry, 2008). CO2 vent sites and ojos (low pH springs) are natural extreme environments where the seawater carbonate chemistry (low pH and low Ωarag) provides comparative conditions to ocean acidification scenarios.
CO2 Vents
Underwater volcanic vents emit CO2 (along with other trace gases, e.g., N2, O2, CH4, Figure 2) into the adjacent seawater, reducing the Ωarag and creating seawater conditions similar to those predicted to occur under ocean acidification. Local hydrology and variations in gas release can create large diel oscillations in pCO2 at vent sites relative to adjacent control sites (Enochs et al., 2015). Even so, vent sites offer a unique natural environment to study ecosystem changes in response to frequent, low pH (Hall-Spencer et al., 2008). In the Mediterranean, the release of CO2 at vent sites has been shown to alter a variety of ecosystems, including seagrass beds (Martin et al., 2008), rocky-reef systems (Hall-Spencer et al., 2008), and vermetid reefs (Milazzo et al., 2014). These temperate studies all report adverse effects of elevated pCO2, including loss of diversity of marine calcifiers (Martin et al., 2008; Kroeker et al., 2011), reduced calcification (Milazzo et al., 2014), shifts from calcareous dominated systems to a state lacking scleractinian corals (Hall-Spencer et al., 2008) and reductions in trophic groups (Kroeker et al., 2011).
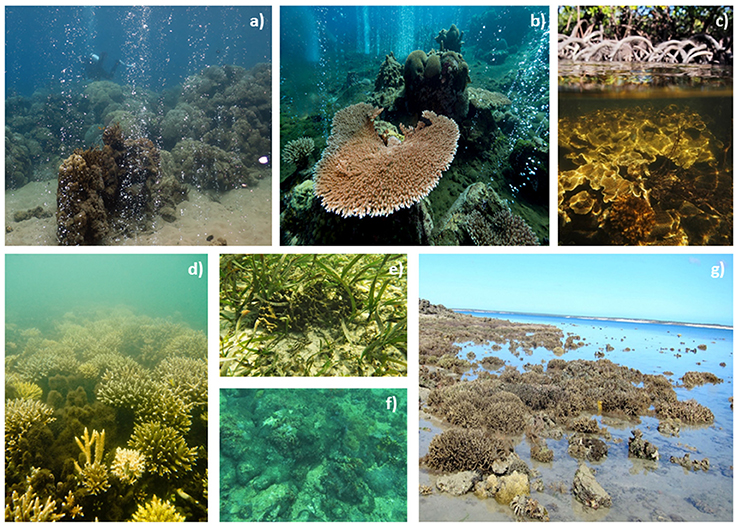
Figure 2. Photographs from different extreme and marginal coral environments. (a,b) Vent sites in Papua New Guinea (photo credit R. Rodolfo-Metalpa and J.M. Boré), (c) mangrove system Great Barrier Reef (photo credit E. Camp), (d) mangrove system New Caledonia (photo credit E. Camp), (e) seagrass bed the Cayman Islands (photo credit E. Camp), (f) turbid reef Salvador Brazil (photo credit E. Camp), (g) Kimberley reef flat (photo credit V. Schoepf).
To date, there have only been three regions where vent systems have been described adjacent to coral reefs: Iwotorishima Island, Japan (Inoue et al., 2013), Papua New Guinea (PNG; Fabricius et al., 2011) and Maug, in the Commonwealth of the Northern Mariana Islands (Enochs et al., 2015). Findings from these vent sites have been variable, likely due to site-specific pH gradients, hydrology and conditions such as nutrient availability (Enochs et al., 2015). For example, in Iwotorishima, communities shifted from hard coral to soft coral as seawater acidified, with all corals absent from the highest pCO2 zone (1,465 μatm, Inoue et al., 2013). In contrast, coral dominated sites at Maug (mean pH = 8.04 ± 0.016, min pH = 7.98) transitioned to macroalgal dominance (mean pH = 7.94 ± 0.051, min pH 7.72).
A common feature across the various vent sites are changes in community structure, where only a few acidification-tolerant species thrive under high pCO2 (e.g., Hall-Spencer et al., 2008; Fabricius et al., 2011, 2015; Kroeker et al., 2011, 2013). There is also evidence that the structural integrity of any reef framework is compromised due to a loss of architectural complexity (Fabricius et al., 2011), increased rates of bioerosion (Enochs et al., 2016a,b), reductions in coral growth (Fabricius et al., 2011) and reduced skeletal density (Fantazzini et al., 2015; Strahl et al., 2015b).
The ability of corals around vent sites to maintain calcification under elevated pCO2 appears to be species-specific (Rodolfo-Metalpa et al., 2011; Inoue et al., 2013). Both in laboratory work (Venn et al., 2011; McCulloch et al., 2012) and field investigations (Wall et al., 2016) species-specific abilities to buffer internal-pH have been recorded. There do appear to be defined physiological limits under which calcification can occur (McCulloch et al., 2012; Wall et al., 2016), and physiological plasticity appears important in supporting calcification at high pCO2. For example, increased rates of calcification (Rodolfo-Metalpa et al., 2011), or the utilization of elevated external dissolved inorganic carbon (DIC) can fuel photosynthesis (Inoue et al., 2013; Strahl et al., 2015a) and offset the higher energetic cost of calcification under acidification. For soft corals (Inoue et al., 2013), sea anemones (Suggett et al., 2012a), and scleractinian corals (Strahl et al., 2015a), success at high pCO2 seems to be driven by their capacity to enhance, or at least sustain, photosynthesis under elevated pCO2. Other physiological processes such as higher cell protective capacities, total lipid content, tissue biomass (Strahl et al., 2015b) and changes in fatty acid metabolism (Kenkel et al., 2017) may also promote resistance to environmental stress at low pH.
Alongside physiological plasticity, live-tissue coverage (Rodolfo-Metalpa et al., 2011), and changes in microbial taxa (Morrow et al., 2014) can support species survival under elevated pCO2. Variable changes in gene expression of Symbiodinium at the PNG vent system suggest population-specific responses to low pH (Kenkel et al., 2017). The collective response of the animal host, symbiotic algae and other microbial taxa demonstrate that fully considering the holobiont (i.e., the coral host, algal symbionts and microbiome) is important in determining success and survival under high pCO2.
Low pH Springs (ojos)
Underwater seeps (ojos) of the Yucatan Peninsula (Mexico) have physicochemical conditions entirely unique to the local area. Here, water that has associated with soil and limestone mixes with high CO2 groundwater resulting in seawater that has a low pH (6.70–7.30), low Ωarag (0.3–0.97 at the center of the seep), and high DIC, AT, Ca2+ and nutrients (Crook et al., 2012). Although the combined physicochemical conditions differ from potential future ocean acidification scenarios and vent environments (e.g., low pH accompanied by high DIC, AT, and Ca2+), the site provides insight into how coral diversity and growth are impacted when corals are exposed to low pH for an extended period of time [high CO2 water has discharged for millennia, (Beddows et al., 2002)]. Crook et al. (2012) found that only three coral species (Porites astreoides, P. divaricata, and Siderastrea radians) persisted close to the ojos where the Ωarag was <2.5. Further analysis of the growth rates of P. astreoides showed no change in linear extension rates, but a fall in skeletal density (ca. 40%) and calcification (ca. 30%) when Ωarag was below 2.0, relative to an adjacent control site where Ωarag was >3.5 (Crook et al., 2013). The ojos sites can experience changes in temperature, salinity and light that may in part explain the reduced calcification rates (Iglesias-Prieto et al., 2014), although the collective assessment from the laboratory and field experimentation suggests low pH is largely responsible for the reductions in calcification measured (Paytan et al., 2014). To date for this site, no molecular data or information on other life-history traits (e.g., metabolic properties) have been published, making it difficult to assess whether this reduction in density does indeed demonstrate a lack of acclimation ability for P. astreoides (Crook et al., 2013) or rather highlights a potential survival process to persist in adverse conditions (e.g., phenotypic or metabolic plasticity, see Pigliucci et al., 2006). Either way, these findings corroborate results from the vent sites that coral calcification will likely be compromised under low pH.
Highly Variable pH/CO2 Environments
Similar to corals, seagrasses (Figure 2) are ecosystem engineers given that they modify their local physical and geochemical conditions (Duarte et al., 2013). During daylight hours, seagrass photosynthesis removes CO2 lowering DIC, while at night, respiration dominates in the absence of photosynthesis, increasing DIC. This metabolic activity in seagrass meadows has been documented to cause large diurnal variation in local seawater carbonate chemistry conditions that can alter the saturation state of carbonate minerals (Schmalz and Swanson, 1969). For example in the Cayman Islands, pH varied by 0.3 units over a diel-cycle (Camp et al., 2016b). Daytime drawdown of CO2 has resulted in seagrass meadows having elevated pH during the day across several regional locations, e.g., Spain (Invers et al., 1997), Florida (Manzello et al., 2012), the Cayman Islands, Indonesia, and the Seychelles (Camp et al., 2016a) that can create an inshore-to-offshore carbonate chemistry gradient (Manzello et al., 2012; Camp et al., 2016a). The capability of seagrasses to elevate local mean pH has led to them being described as potential refugia under ocean acidification (Manzello et al., 2012), since they buffer resident species from rising seawater acidity (Hofmann et al., 2011; Manzello et al., 2012; Hendriks et al., 2014). Unsworth et al. (2012) proposed that the photosynthetic activity of seagrass meadows in the Indo-Pacific could enhance seawater physicochemical conditions to increase coral calcification downstream by as much as 18%. However at night, high respiration rates can lead to seagrass meadows becoming a source of CO2 (Schmalz and Swanson, 1969), causing large drops in local seawater pH, that at several locations have caused pH to fall below 7.8 (Camp et al., 2016a,b), creating conditions predicted under future ocean acidification (Feely et al., 2004).
The ability of seagrasses to modify the local environment may result in non-genetic ecological inheritance (Bonduriansky and Day, 2009), influencing the evolutionary selection pressure on themselves and other local taxa (Mumby and Van Woesik, 2014). Seagrass meadows have not typically been considered important coral habitats due to a lack of solid substrate (Jackson, 1986; Manzello et al., 2012). However, corals can recruit into seagrass systems; for example in the Cayman Islands where 14 coral taxa (primarily P. astreoides and Siderastrea sp.) were found to recruit into seagrass beds (Lohr et al., 2017). The ability of seagrasses to moderate the local seawater carbonate chemistry has resulted in an increased interest in their role as a coral habitat and potential coral refugia, although, the latter role is still unclear. Increased availability of CO2 and under ocean acidification is predicted to enhance photosynthetic rates of carbon limited seagrasses (Durako, 1994; Buapet et al., 2013; Hendriks et al., 2014) and increase the growth and biomass of seagrass beds (Zimmerman et al., 1997). Research from high CO2 systems has provided compelling evidence that seagrasses will flourish (Hall-Spencer et al., 2008; Fabricius et al., 2011; Duarte et al., 2013), sustaining their ability to modulate pH (Duarte et al., 2013). Furthermore, low night-time pH could result in chemical conditions where carbonate-sediment dissolution occurs (Yamamoto et al., 2012), increasing local AT and pH, thus creating a self-buffered system (Andersson et al., 2014). However the shallow nature of seagrass systems means they also experience variability in other parameters such as oxygen (Hendriks et al., 2014), salinity (Manzello et al., 2012), temperature (Manzello et al., 2012; Camp et al., 2016a,b), and light (Hendriks et al., 2014). Work from seagrass habitats in the Cayman Islands suggests that if future diel-oscillations in pH and temperature change at an equivalent magnitude as predicted for the open-ocean, seagrass systems could be limited in their ability to buffer resident species, due to the extreme low night-time pH and elevated daytime temperatures they experience (Camp et al., 2016b). Consequently, the interactive effect of local physicochemical conditions, and the magnitude of diurnal and seasonal variance, will likely control whether any protective services are provided by seagrass systems in the future.
High Temperature Environments
Tropical corals live close to their upper thermal limits and are therefore highly sensitive to periods of elevated SST and ocean warming (Heron et al., 2016). Coral bleaching occurs when corals lose a significant portion of their algal endosymbionts and/or photosynthetic pigments in response to heat stress, and can result in widespread coral mortality on regional-to-global scales. Global interest in elevated temperature effects on corals has peaked recently due to the increasing frequency and intensity of thermally-induced mass bleaching events (Hughes et al., 2017). This has led to research efforts that focus on identifying maximum, and variations in thermal tolerance across coral species and regions, as well as identifying potential coral refugia to future ocean warming.
During the summer season, the Persian-Arabian Gulf (PAG) experiences the highest sea temperatures in the world, yet still houses a significant number of coral species (55–60 species) despite the most extreme temperature conditions ever recorded for extant coral reefs (Sheppard, 2009; Riegl and Purkis, 2012). Shallow (generally <30 m) water depth and restricted water exchange with the Indian Ocean, requires that resident corals must not only endure the highest maximum SSTs (>35°C in summer) recorded for extant coral reefs, but also the largest seasonal range of >20°C (Sheppard, 2009; Riegl and Purkis, 2012). In addition, high evaporation rates in the PAG result in unusually high salinity, regularly exceeding 40 psu (Sheppard, 2009; Riegl and Purkis, 2012). Since the PAG was only formed about 15,000 years ago, and shifted toward the present temperature regime only 3–6,000 years ago (Sheppard, 2009; Purkis et al., 2010), Gulf corals have adapted to these extreme environmental conditions over only the last few millennia. As such, the PAG has become a popular area to better understand the mechanisms corals have evolved to thrive under warmer climates.
Corals within the PAG have the highest upper temperature thresholds for bleaching (~35–36°C; Riegl and Purkis, 2012; Coles and Riegl, 2013), but still remain highly susceptible to bleaching when temperatures exceed their local maximum summer temperatures (Coles and Riegl, 2013; Kavousi et al., 2014). For example, five times from 1995 to 2010, temperatures exceeded normal summer temperatures, reaching 35–37°C for extended periods of time, which resulted in extensive bleaching and mortality (Coles and Riegl, 2013; Kavousi et al., 2014). These disturbances resulted in a decline of branching species and rise of massive favid corals. It is well-known that massive coral species are typically more tolerant to light and heat stress, and such shifts from more heat-sensitive, branching coral communities to more heat-tolerant, massive communities are commonly observed after bleaching (e.g., Loya et al., 2001). In contrast to branching corals, massive corals have skeletons that are less efficient in enhancing the internal light field of the coral (Enríquez et al., 2017), which appears to be a key factor in enabling their higher tolerance to light and heat stress. Although less diverse and spatially complex, the new poritid- and faviid-dominated communities in the PAG appear to be relatively resilient to ongoing disturbances in the region (e.g., Bento et al., 2016) and could represent future coral communities under continued climate change and increasing anthropogenic stressors.
Extensive research into the biology of corals surviving in the warm waters of the PAG has revealed mechanisms for survival, such as metabolic trade-offs (e.g., reduced fecundity Howells et al., 2016a), and unique physiological and genetic signatures, notably a regionally prevalent heat-specialist algal endosymbiont, Symbiodinium thermophilum (Hume et al., 2015; Smith et al., 2017a) belonging to a highly diverse ancient group of symbionts cryptically distributed outside the PAG (Hume et al., 2016). S. thermophilum occurs in high temperature, high saline environments, and as such, the benefit of a temperature stress-resistant phenotype, comes with a fitness-trade off of existing in high saline systems (D'Angelo et al., 2015). In addition to the algal endosymbiont species, PAG corals appear to have evolved genetic hardware at both the level of host and host associated bacteria in heat stress resistance, for example, the coral Platygyra daedalea exhibits superior ability to cope with oxidative stress in the PAG compared to conspecifics from the cooler Gulf of Oman (Howells et al., 2016b). Unusual patterns in the coral microbiome of PAG corals were also recently observed, as bacterial microbiome composition was found to be similar in bleached and healthy Porites lobata corals (Hadaidi et al., 2017), which is in contrast to studies from other coral reef regions (e.g., Bourne et al., 2007).
The PAG is arguably the world's hottest sea supporting coral reefs; however, other hot seas exist (e.g., the Red Sea) that support corals potentially more tolerant of thermal stress events (Fine et al., 2013; Grottoli et al., 2017; Krueger et al., 2017). The hot, southernmost end of the Red Sea acts as a selective thermal barrier favoring heat-resistant genotypes; once these genotypes spread to the northern, cooler Red Sea, they live well below their bleaching threshold (Fine et al., 2013). Consequently, elevated temperatures of 2°C above average thermal maxima did not result in any coral bleaching in northern Red Sea corals in laboratory studies (Fine et al., 2013; Krueger et al., 2017), and for three coral species, Stylophora pistillata, Pocillopora damicornis, and Favia favus, coral bleaching did not occur until 6°C above average thermal maxima (Krueger et al., 2017). Furthermore, the coral S. pistillata has shown resistance to the combined effects of ocean acidification (pH 7.8) and elevated temperatures (Krueger et al., 2017). The unique evolutionary history of northern Red Sea corals highlights the key role of broad latitudinal temperature gradients in promoting adaptation to high temperatures and providing genetic rescue via the exchange of heat-resistant genotypes across latitudes. However, high connectivity across latitudes is critical for the establishment of high temperature high-latitude coral reef environments as refugia from climate change.
Highly Variable Temperature Environments
Alongside environments that experience persistently high temperatures, environments that have variable temperature regimes can provide information on the heat-tolerance, and acclimation/adaptation potential of coral species. Variations in temperature regimes can occur due to local (e.g., tide pools) conditions, or can result from temporal differences (e.g., high-latitudinal corals).
Back Reef Tide Pools
Elevated temperatures become particularly pronounced as reefs shallow and flushing becomes restricted. One key system that has been extensively studied since the early 2000s is the back reef tide pools on Ofu Island, American Samoa. The back reef pools harbor diverse coral communities (Craig et al., 2001) and feature distinct thermal regimes, governed by the thermal dynamics of pools of different sizes (Smith et al., 2007; Oliver and Palumbi, 2011b; Koweek et al., 2015); specifically, smaller high-variance (HV) pools where SST can briefly reach 35°C during strong noontime low tides (exceeding the local critical bleaching temperature of 30°C) and fluctuate by up to 6°C daily, vs. larger moderately variable (MV) pools, where SSTs rarely exceed 32°C and exhibit a more stable thermal environment.
Corals within the HV pool appear to have acquired putative heat tolerance through genetic, physiological, and ecological adaptations. For example, phenotypic plasticity of skeletal characteristics (Smith et al., 2007) that could potentially be linked to optimization of skeletal optical properties (Enríquez et al., 2017), a switch in symbiont type that is considered more heat-tolerant (Smith et al., 2008; Oliver and Palumbi, 2009, 2011a,b), enhanced thermal tolerance when exposed to heat stress (Oliver and Palumbi, 2011b; Palumbi et al., 2014), higher transcription of heat responsive genes than MV corals (Barshis et al., 2013) and a reservoir of alleles pre-adapted to high temperatures (Bay and Palumbi, 2014). Changes in the microbiome have also been demonstrated with bacterial communities changing in response to transplantation between the MV and HV pools (Ziegler et al., 2017). Higher heat tolerance of HV corals was found to be the result of both local acclimatization and fixed effects such as adaptation, with acclimatization in <2 years achieving the same heat tolerance that would be expected from strong natural selection over multiple generations (Palumbi et al., 2014). This unique system has thus provided critical insights into the mechanisms underlying coral heat tolerance, particularly of host-based contributions to holobiont heat tolerance. Nevertheless, it is currently unclear whether even naturally heat tolerant corals can continue to improve their heat tolerance fast enough to keep pace with global warming, with locations within American Samoa recently experiencing severe bleaching (Eakin et al., 2016).
High-Latitude Environments
Corals from high-latitude environments are functionally different to tropical corals, having traits that may facilitate their survival under climate change (Beger et al., 2014). High-latitude, cooler water (sub-tropical) coral environments have received increasing attention as potential refugia from climate change and ocean warming for tropical coral reef organisms (Beger et al., 2014; Muir et al., 2015b). They currently exist at the environmental limits for growth, in terms of temperature, salinity and Ωarag, where conditions are also highly seasonally variable (Kleypas et al., 1999; Guinotte et al., 2003). Well-studied locations include: Lord Howe Island and the Solitary Islands (30–32°S), Sydney Harbour (34°S) in eastern Australia, the Abrolhos Islands (28°S) and Rottnest Island (32°S) in Western Australia, Sodwana Bay in South Africa (28°S), several islands in temperate Japan (32–34°N), and Bermuda (32°N). Due to the marginal environmental conditions (particularly at more extreme high-latitudes), they can be considered natural extreme coral reef environments which differ from their tropical counterparts in many aspects. For example, the unique diversity of high-latitude reefs is due to the overlap of tropical and temperate species ranges, species that are rare or absent at lower latitudes and species endemic to these environments (Beger et al., 2014 and references therein). Species temporal turnover can be high depending on larval supply and recruitment from lower latitudes and fluctuations in environmental conditions. Whilst coral cover (Harriott et al., 1994; Thomson and Frisch, 2010; Denis et al., 2013) and even coral growth rates can be high (e.g., Ross et al., 2015), reef accretion and development are nevertheless often limited, with corals sometimes only forming living veneers on rocky substrate (Beger et al., 2014 and references therein). Furthermore, future reef accretion could become increasingly challenged with the percent changes in Ωarag predicted to be greater at high-latitude reefs relative to their tropical counterparts (Hooidonk et al., 2014).
Greater fluctuations in environmental conditions at high-latitude coral reefs have been hypothesized to increase the environmental tolerance of high-latitude species as observed in tropical corals (Oliver and Palumbi, 2011b; Schoepf et al., 2015), thus potentially increasing their ability to cope with climate change. In high-latitude corals, phenotypic plasticity may support their survival, through diverse coral symbiont communities (Wicks et al., 2010b), enhanced symbiont tolerance to extreme (low) temperatures (Wicks et al., 2010a), enhanced heterotrophic plasticity (Bessell-Browne et al., 2014) and evidence of shifted thermal optima for calcification at cooler temperatures (Ross et al., 2015). High-latitude coral communities around the world have also been found to reproduce sexually and are therefore not solely dependent on recruitment from tropical region coral stocks (e.g., Babcock et al., 1994; van Woesik, 1995; Wilson and Harrison, 2003; Miller and Ayre, 2004; Madsen et al., 2014).
As predicted, corals have already extended their latitudinal range pole-wards in several locations such as Japan and eastern Australia (Booth et al., 2007; Yamano et al., 2011; Baird et al., 2012), although range expansion can be accompanied by reduced symbiont diversity (Grupstra et al., 2017). The ability and rate of this range expansion will be key in determining the ability of high-latitude locations to act as refugia. Furthermore, high-latitudinal corals themselves are increasingly threatened by climate change and ocean warming, as bleaching events have also occurred in many high-latitude locations in recent years (Celliers and Schleyer, 2002; Harrison et al., 2011; Thomson et al., 2011; Abdo et al., 2012; Smale and Wernberg, 2012). In addition, several other factors specific to high-latitude coral reefs, such as latitudinal light attenuation (Muir et al., 2015a), geographic isolation and small population sizes combined with greater predicted ocean warming and generally lower Ωarag of cooler waters, could make high-latitude coral communities particularly susceptible to climate change (Beger et al., 2014). It is therefore currently unclear whether and to what extent high-latitude corals can in fact serve as refugia from continued climate change, despite evidence for some adaptations to their marginal, more extreme environments.
Broad Temperature Gradients
Reef systems that extend across broad latitudes and temperature gradients have provided evidence of juvenile (Woolsey et al., 2015) and adult corals with enhanced thermal tolerance (Ulstrup et al., 2006; van Oppen et al., 2011; Howells et al., 2012, 2013). On the Great Barrier Reef (GBR), mean summer maximum temperatures have been reported to have nearly 2°C difference at reefs 250 km apart (South Molle Island to Magnetic Island; Howells et al., 2013). Different thermal histories on the GBR have been found to influence bleaching susceptibility (Ulstrup et al., 2006; Ainsworth et al., 2016) and the physiological performance of a generalist coral symbiont (Howells et al., 2012). Warmer latitudes have also been shown to enhance heritable thermal tolerance, up to 10-fold in Acropora millepora from the GBR (Dixon et al., 2015). Such latitudinal gradients thus provide important information over the spatial scales through which thermal tolerance can be achieved, and opens discussion on the ability for more “tolerant” species to provide “genetic rescue” through the exchange of more tolerant larvae across latitudes (Ingvarsson, 2001). That said, studies of the coral Oculina patagonica in the Mediterranean across locations with different thermal temperature regimes demonstrated limited capacity to acclimate to conditions comparable to future warming (Rodolfo-Metalpa et al., 2014). Thus, the ability for corals to inherit thermal tolerance is likely to be species-specific, and highly-dependent on the dynamics of local temperature regimes. Costs may also be associated with greater thermal tolerance, for example, a thermo-tolerant symbiont, Symbiodinium trenchii (D1a), has been shown to reduce host mortality rates by 30% under thermal stress; however, that is accompanied by a reduction in growth rates of 50–60% (Little et al., 2004; Smith and Iglesias-Prieto, 2010; Pettay et al., 2015). Energetic trade-offs need to be considered alongside the benefit of tolerance to assess whether coral reef form and function can be maintained.
Low Light Environments
Limitations in light availability can be fundamental in restricting scleractinian coral distribution (Yentsch et al., 2002; Muir et al., 2015a). However, during thermal stress events, solar radiation can act synergistically to intensify the impact on corals (Mumby et al., 2001; Anthony et al., 2007). Consequently, shaded (Coelho et al., 2017) and low-light environments (e.g., turbid reefs and mesophotic reefs) that are typically considered marginal for coral growth, could become increasingly valuable for corals under climate change and ocean warming.
Turbid Reefs
Inshore turbid environments (Figure 2) often have a suite of extreme conditions, such as low light and elevated water temperatures, and thus have historically been overlooked as important coral environments. Corals employ physiological plasticity to persist in turbid waters, including flexible photosynthetic properties, which may include a change of algal endosymbiont genetic type, and/or enhanced host heterotrophy (Anthony, 2000; Anthony and Fabricius, 2000; Hennige et al., 2008, 2010; Suggett et al., 2012b). Morgan et al. (2016) recently described over 21 genera of coral surviving in muddy waters on the inshore GBR suggesting that turbid systems may afford important range (niche) extension beyond that currently considered for many key “blue water” tropical oligotrophic reef-forming coral taxa.
High irradiance exacerbates susceptibility to coral bleaching, and thus turbid reef environments where irradiance is reduced could act as potential refugia during bleaching events. Cacciapaglia and van Woesik (2015) predicted that 9% of coral environments previously considered uninhabitable under ocean warming, due to the combination of elevated temperatures and high irradiance, would be protected by the mitigating effect of shading in high turbid sites. Studies in Palau (van Woesik et al., 2012), Florida (van Woesik and McCaffrey, 2017), and on the GBR (Morgan et al., 2017) have all provided compelling evidence that coral bleaching can be reduced at high-turbidity sites. Consequently, as the oceans warm and bleaching events become more frequent, there could be a direct selection of corals able to survive in inshore turbid environments (van Woesik and McCaffrey, 2017). Potts and Jacobs (2000) propose that turbid inshore habitats have provided ecological and evolutionary continuity and refugia for scleractinian corals during non-reefal periods when environmental conditions have been too extreme for reef growth.
Mesophotic Reefs
High-anthropogenic stress experienced by shallow reefs has seen deeper reefs proposed as potential refugia (Glynn and Morales, 1997; Riegl and Piller, 2003; Armstrong et al., 2006). Under this hypothesis, deeper reefs are able to: (i) protect or reduce the disturbances faced on shallower reefs, and (ii) provide a genetic stock of corals that can re-seed shallower reefs after a disturbance. Supporting this theory are studies evidencing reduced bleaching on deeper coral reefs (e.g., Riegl and Piller, 2003; Bridge et al., 2014). However, this bleaching pattern is not always observed; for example, with deeper corals in the Seychelles bleaching first, and more severely, than shallow corals during the 1997–1998 bleaching event (Spencer et al., 2000). Smith et al. (2016) demonstrated that deep (30–75 m) coral reefs have lower bleaching threshold temperatures than shallow-reefs, and that any increase in temperature above the local mean can result in stress and bleaching. Furthermore, deepwater generally has lower pH and Ωarag than shallow-water, complicating the ability of these systems to act as refugia.
Capacity for deep reefs to act as potential refugia remains debated and depends in part on the connectivity of shallow and deep coral populations (Bongaerts et al., 2011). van Oppen et al. (2011) found evidence of deep-to-shallow coral recruitment for some reefs (Scott's Reef) on the GBR, but not others (Yonge Reef). Similarly, Serrano et al. (2014) found geographic differences in vertical connectivity due to local geophysical conditions. Species-specific differences in population connectivity with depth have also been reported in the Caribbean (Bongaerts et al., 2017).
Low-light conditions of deeper reefs are considered sub-optimal, and may select against some corals that do not have the physiological plasticity to survive. For example, Smith et al. (2017b) identified the importance of photoconvertible red fluorescent proteins (pcRFPs) to support coral adaptation/acclimation to deeper waters, while Cooper et al. (2011) showed that both the host and symbiont needed to have photobiological flexibility to survive across light regimes. Similarly, Lesser et al. (2010) found for the coral Montastrea cavernosa in the Bahamas that both physiological and morphological adaptation was required to survive in depths down to 91 m. Changes in the endosymbiont community (e.g., Frade et al., 2008; Lesser et al., 2010; Bongaerts et al., 2015) and density (Frade et al., 2008) have also been reported with depth. Specialization of deeper coral communities suggests plasticity will be required for species to survive across depths and ultimately utilize these systems as potential refugia.
Multiple Stressor Environments
Under climate change, multiple environmental factors such as temperature, pH, and oxygen, are predicted to co-vary (Bijma et al., 2013). Understanding how these changes will co-occur and influence biological processes is one of the hardest challenges facing researchers. Natural extreme environments where multiple factors co-vary, e.g., mangrove systems (Yates et al., 2014; Camp et al., 2016a), shallow reef habitats (e.g., Price et al., 2012), macrotidal reef environments (e.g., Schoepf et al., 2015) and upwelling environments (Riegl and Piller, 2003) offer natural laboratories to address these more complex environmental questions. Notably in these systems it can be difficult to assess the relative importance of individual factors for the observed response; thus, a collective assessment with systems where one abiotic variable primarily changes is essential to advancing our understanding of how corals will survive into the future.
Mangrove Habitats
Mangroves are widespread reef-associated environments that have recently received attention for their potential services to corals threatened by climate change (e.g., Yates et al., 2014; Camp et al., 2016a). Mangrove systems have dynamic temperature, pH and oxygen profiles, and as such, expose resident corals to very different physicochemical conditions than neighboring reefs (Camp et al., 2017). Mangroves are highly heterogeneous in nature, with freshwater input, organic content, system size and consequently water residency time, along with other benthic components (e.g., seagrass composition) influencing the local physicochemical conditions. As such, mangrove systems appear to offer a range of potential services to corals, dependent on local spatial and temporal biogeochemical conditions. For example, work in the Caribbean (Yates et al., 2014) suggested that mangroves could act as a potential refuge for corals, through physical shading and the elevation of downstream AT as a result of carbonate-sediment dissolution (i.e., buffering potential similar to seagrass habitats), as well as frequent exposure to more variable temperatures. In other locations [e.g., the Seychelles, Indonesia (Camp et al., 2016a) and New Caledonia (Camp et al., 2017)] mangrove systems have exposed corals to simultaneously low pH, low oxygen and warmer waters relative to adjacent reefs. As such, these mangrove systems may precondition corals to future climates (Camp et al., 2016a) or house corals more resilient to future climate conditions (Figure 2, Camp et al., 2017). In New Caledonia, over 20 coral species were regularly exposed to pH as low as 7.3, temperature 1–2°C higher than adjacent reefs, and oxygen levels below 3 mg L−1 (Camp et al., 2017). Initial research at this site suggests that physiological plasticity (e.g., enhanced respiration indicative of increased heterotrophy) is facilitating survival of diverse coral communities, which include numerous species typically thought to be more sensitive to stress, e.g., Acropora spp.
Shallow In-shore Variable Reef Habitats
Physicochemical conditions of shallow reef systems are dynamic and highly influenced by their coastal interface, ecosystem structures and local metabolic activity. Across global locations, there are reports of shallow in-shore reef systems being regularly exposed (albeit for different durations) to conditions predicted for the open-ocean under climate change by the end of the century (e.g., Hofmann et al., 2011; Price et al., 2012; Albright et al., 2013; Guadayol et al., 2014; Kline et al., 2015). The shallow water depth and reduced residency time of inshore waters can create an inshore to offshore carbonate chemistry gradient (Manzello et al., 2012; Shamberger et al., 2014; Camp et al., 2016a). Nutrient run-off and freshwater input can influence AT, while the metabolic activity of local benthos can change pCO2 levels. Sites in Palau (Shamberger et al., 2014; Barkley et al., 2015), Puerto Rico (Gray et al., 2012), Florida (Millero et al., 2001; Yates et al., 2007), and the GBR (Santos et al., 2011; Shaw et al., 2012, 2015; Kline et al., 2015) have all been characterized as having extreme in-shore low pH reef systems. In the in-shore low pH bays of Palau, coral cover and richness remained similar to control areas, but community composition shifted, with replacement of Acropora, Montipora, and Pocillopora with Porites (Barkley et al., 2015). For the abundant Porites sp. skeletal extension and density remained unchanged but macrobioerosion increased at the lower Ωarag sites (Barkley et al., 2015). Interestingly, one of the low pH Palau communities was not dominated by Porites but instead, Leptastrea, Platygyra, Favites, and Dipsastraea, contributing to the emerging evidence that a range of species are tolerant to low pH conditions. These findings suggest that acidification conditions are not deterministic, and that other local factors are also important in shaping coral community composition under low pH and low Ωarag (Barkley et al., 2015).
Along with environments that have persistently low pH (e.g., Palau), there are highly variable pH habitats that routinely, through tidal and diurnal cycles, experience low pH beyond what is predicted for coastal systems by 2100 (Duarte et al., 2013). For example, the Heron Island reef flat can experience a seasonal range in temperature of 8.1°C (17.9–26°C) and pH of 1.04 (7.57–8.61; Kline et al., 2015). Daytime photosynthesis, and night-time respiration in the absence of photosynthesis, create large diel cycles in pH (0.75 units, Santos et al., 2011) and Ωarag (5.4 units, Shaw et al., 2012). At these highly variable in-shore sites, carbonate chemistry conditions can be optimal (elevated pH and Ωarag) or sub-optimal (low pH and Ωarag), and the presence of diverse and abundant coral communities at these sites indicates that corals can tolerate, at least periodic, short-term exposure to low pH. It remains unclear whether exposure to periodic low pH and low Ωarag enhances resistance to future ocean acidification. In the Cayman Islands, corals from a naturally variable inshore pH environment experienced no enhanced tolerance when exposed to conditions predicted for 2100 (Camp et al., 2016b). Similarly, three corals species in Florida Bay regularly exposed to seasonal and diel variance in pCO2 and Ωarag showed no reduced sensitivity to low Ωarag, with calcification rates equivalent to corals from a more stable physicochemical environment (Okazaki et al., 2013). However, the natural oscillations of pCO2 in these in-shore environments may reduce the negative effects of elevated pCO2, by ensuring at least periodic conditions for optimal calcification. For example, Comeau et al. (2014) observed calcification rates for Acropora hyacinthus that were increased by 21% under 400 to 2,000 μatm oscillatory pCO2 vs. 1,000 μatm constant pCO2 treatment. Such heterogeneity of in-shore reef systems, means that some locations dominated by photoautotrophs could in fact see an elevation in mean pH relative to offshore, due to the daytime consumption of CO2 elevating pH and local Ωarag, as observed for the back reefs of the Cayman Islands (Camp et al., 2016a) and in seagrass dominated environments.
Macrotidal Coral Reef Environments
Coral reefs subjected to large tidal ranges are similarly exposed to extreme environmental conditions. The most extreme macrotidal reef site examined to date is the Kimberley region in northwest Australia, which features the world's largest tropical tides (up to 12 m). Corals on the reef flat of the Kimberley region have to cope with extended aerial exposure during spring low tides (up to several hours, Figure 2) and periods of stagnant water alternating with strong tidal currents (>10 knots). These physical conditions create extreme fluctuations in environmental parameters, such as temperature (e.g., the well-studied Shell Island where temperature can fluctuate by up to 7°C daily, Dandan et al., 2015; Schoepf et al., 2015), pH (e.g., Tallon Island where pH can fluctuate from 7.6 to 8.8 units over a spring low tide, Pedersen et al., 2016), dissolved oxygen (Pedersen et al., 2016; Gruber et al., 2017), unusually turbid waters, and monthly SST exceeding 30°C for several months a year. Despite these extreme conditions, highly diverse coral reefs exist throughout the Kimberley of up to 225 species (Rosser and Veron, 2011; Richards et al., 2015), which are comparable to inshore reefs in the central GBR ~2 decades ago (Richards et al., 2015).
Research is just beginning to uncover the biological properties that allow corals to thrive in this extreme macrotidal environment. Firstly, algal endosymbionts appear to be locally adapted with clade C dominating northern Kimberley Acropora assemblages (Thomas et al., 2014) and southern Acropora and Dipsastraea corals (Schoepf et al., 2015); however, further research is required to determine how Symbiodinium diversity within clade C is linked to environmental gradients in this region. Furthermore, thermal history has been shown to influence bleaching susceptibility of corals harboring the same symbiont type, with more variable temperature environments enhancing heat resistance (Schoepf et al., 2015). During the first documented regional-scale bleaching event in the Kimberley in the austral summer of 2016 (Hughes et al., 2017), corals were more severely bleached (Le Nohaïc et al., 2017) and experienced much greater mortality (V. Schoepf, unpublished data) at the less-variable subtidal site on Shell Island.
Reef flat communities of the Kimberley appear to have adapted their physiology to maintain metabolic balance of photosynthesis-to-respiration (P:R) despite the extreme physicochemical conditions of the reef-flat (Gruber et al., 2017). Furthermore, a seasonal calcification study revealed high resilience of coral calcification to both intertidal and subtidal temperature conditions as branching and massive corals calcified at rates that were comparable to those of similar species at a more typical coral reef 1,200 km to the southwest (Ningaloo Reef; Dandan et al., 2015). Given that rising sea levels are expected to reduce extreme temperature variation on macrotidal reef flats such as Tallon Island (Lowe et al., 2016), reef flat communities might have more time than other reef types to acclimatize and/or adapt to ongoing ocean warming. However, further research is needed on these systems to disentangle the complex interactions of acclimation/adaptation processes that support coral survival under such extreme physicochemical conditions.
Upwelling Environments
Dispersed across the world's oceans are sites where deep, nutrient-rich, cooler ocean water wells-up to the surface through local water circulation. Such waters have been proposed as potential localized coral refugia under times of thermal stress (Riegl and Piller, 2003); however, the cool water is also high in CO2 and low in Ωarag; consequently, upwelling environments have the potential to be ocean acidification hot-spots (Feely et al., 2008; Manzello, 2010). Upwelling sites clearly comprise a complex mix of abiotic stressors, with any refuge potentially limited by upwelling time (Chollett et al., 2010). In regions such as the Eastern Tropical Pacific (ETP) and Arabian Sea, marginal coral reef formation has been attributed to the cool upwelled waters creating a “pseudo-high-latitude effect” (Sheppard and Salm, 1988) by reducing reef-building processes (Benzoni et al., 2003). Here, corals do not form a true reef structure, but instead form low-relief coral carpets (often monospecific) or remain as isolated coral colonies that are generally small in size. These locations can illustrate the changes in coral community composition when reef formation ceases, as is predicted to occur in a high CO2 world.
A unique upwelling system exists in the ETP where high CO2 water upwells to the surface, resulting in coral reef and coral communities off the coast of the Galápagos Islands having some of the lowest Ωarag documented for modern coral reefs (<2.5, (Manzello et al., 2008)). Corals of the Galápagos Islands and neighboring Panamá reefs also contend with frequent exposure to the El Niño-Southern Oscillation (ENSO, + 3–4°C during ENSO events for >2 months), creating a unique opportunity to study the long-term effects of low pH and thermal stress events on corals (Manzello et al., 2014). Only one coral reef exists within the low Ωarag waters of the Galápagos archipelago and it is dominated by P. lobata (Manzello et al., 2014). High bioerosion rates (>25 kg CaCO3 m−2 yr−1) and low reef cementation are characteristic of sites within the Galápagos region (Manzello et al., 2008).
Upwelled waters of the ETP are also high in nutrients, which may in part have enhanced coral growth rates for four coral species of the ETP (Jiménez and Cortés, 2003). Elevated nutrient levels have the potential to alleviate some of the negative impacts of decreased coral calcification associated with the low Ωarag of the upwelled waters, by potentially enhancing heterotrophy (Langdon and Atkinson, 2005; Cohen and Holcomb, 2009), or increasing inorganic nutrients that can enhance cell growth and proliferate abundance of the algal symbiont (see review by Fabricius, 2005). However, enhanced heterotrophy may not always be an advantage for corals around cooler upwelling sites, with zooplankton feeding rates of three coral species reduced under cooler upwelling conditions in Panamá (Palardy et al., 2005). High nutrients, in particular phosphates, can also reduce skeletal density (Dunn et al., 2012), and may explain why corals around the Galápagos Islands maintain their linear extension rates, but experience reduced skeletal density (Manzello et al., 2014). Increased exposure to nitrogen levels has also been documented to enhance bleaching susceptibility when exposed to heat and light stress (Wiedenmann et al., 2013) and could add to the potential bleaching susceptibility of corals at upwelling sites (D'Croz and Maté, 2004). Clearly the net-gain or cost of elevated nutrients for corals around upwelling sites remains debated and is an area for further investigation. An important consideration with upwelling sites is that the severity or even presence of upwelling is often seasonal (as occurs for sites of the ETP), meaning the physicochemical conditions representative of future scenarios may be limited in duration. Timing of assessment at these sites is therefore important, with seasonal differences having large impacts on the biodiversity recorded (e.g., Diaz-Pulido and Garzón-Ferreira, 2002; Witman and Smith, 2003).
Discussion
What Are the Extreme Abiotic Scenarios Extant Corals Persist under?
Review of the current literature on extreme and marginal coral environments demonstrated that coral populations presently thrive under different combinations of extreme abiotic conditions that are comparable to, or even exceed, predictions for the open-ocean under future climate change (Figures 3, 4). For example, corals around ojos experienced persistently low pH below 7.7 (Crook et al., 2013), while corals of the PAG in summer experienced temperatures exceeding 35°C (Sheppard, 2009); these are conditions that are not predicted for the tropical open-ocean to well beyond 2100 (IPCC, 2014). The predictability and duration of the extreme environmental conditions experienced by corals are important considerations when assessing the role these environments can play in coral reef research and management. For example, regular exposure, rather than periodic pulses of extreme conditions may be required to facilitate acclimation and/or adaptation (e.g., Oliver and Palumbi, 2011a; Howells et al., 2012). Alternatively, fluctuating abiotic conditions, such as pH, may offer periods of time when physiological processes (e.g. calcification) can occur at less of an energetic cost. A key question is therefore how species will respond to stress according to different exposure time-frames (Mumby and Van Woesik, 2014). To better resolve how environmental history influences the survival of extreme and marginal corals, continuous, multi-physicochemical data sets are required that show both the mean and range of environmental conditions. As demonstrated in Figure 4, clustering of sites varied depending on the metric considered, e.g., mean or range. When reporting environmental conditions, there is also a need to provide both the immediate and historical context. As such, it is recommended that actual abiotic measurements (both mean and variance) are reported alongside historical datasets.
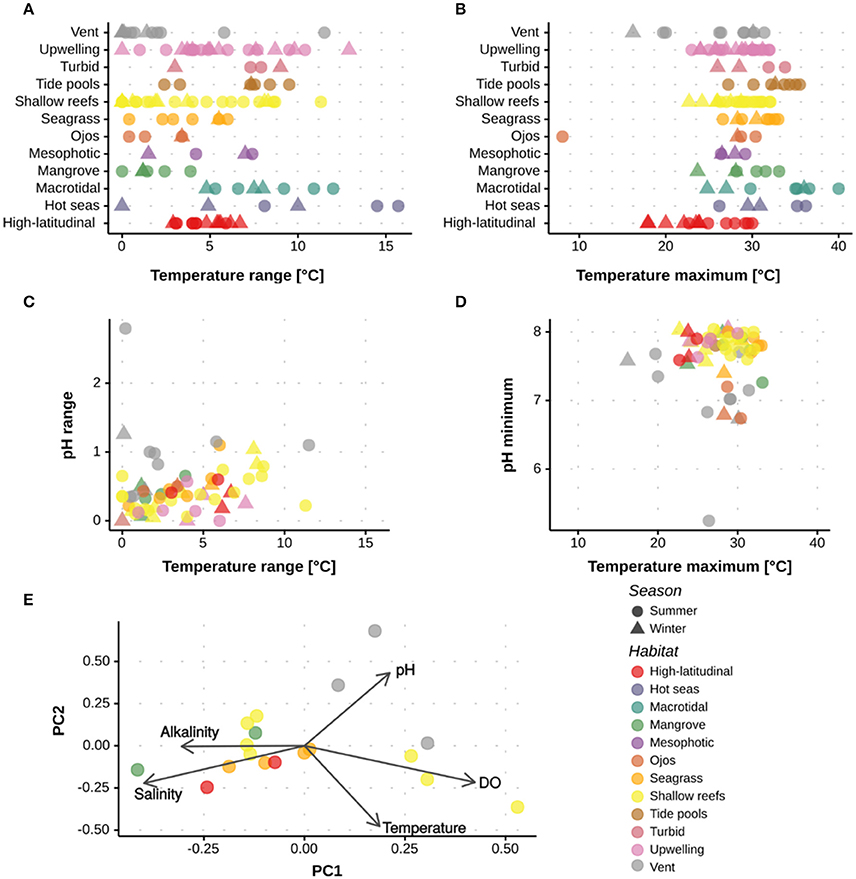
Figure 4. Quantitative assessment of the abiotic conditions experienced at the different extreme and marginal environments. Data are shown for studies out of the 285 assessed that reported the maximum, minimum or range in (A,B) temperature, (C,D) temperature and pH, and (E) temperature, pH, AT, salinity and oxygen. Data are shown per season and environment. Supplementary Table 1 and Supplementary Figure 1 have the references for the data used.
The current review highlighted that few studies reported multiple physicochemical conditions. For example, a seasonal comparison of pH, temperature, AT, salinity, and oxygen was only possible at a total of 14 sites spanning five environments (Figure 4). The limited data collected on multiple abiotic parameters fundamentally restricts our ability to: (i) determine how extreme some coral environments are, and (ii) understand the different biogeochemical responses reported across sites. The PCA highlights that for many environments multiple parameters interact within these extreme systems (Figure 4), thus emphasizing the need to collect a full suite of abiotic parameters to provide context for biological assessments (Table 1). Whilst such a recommendation is not trivial, it becomes increasingly important as we begin to resolve the complex abiotic interactions that regulate many biological processes.
Are There Commonalities in Coral Taxa of Extreme Environments?
Review of the current literature suggests that the capacity for coral taxa to persist in sub-optimal environments is species-specific, and largely dependent on local factors, e.g., nutrient availability. Despite a lack in common coral taxa across extreme environments, critical thresholds in community structure do appear to exist as abiotic conditions become increasingly less optimal: (i) an initial reduction in species diversity (e.g., Craig et al., 2001; Fabricius et al., 2011; Enochs et al., 2015) with specialist species often able to survive and potentially even thrive within the new environmental niche (e.g., Kroeker et al., 2011; Fabricius et al., 2014; Dandan et al., 2015; Schoepf et al., 2015), followed by, (ii) a “lethal” threshold beyond which survival ceases (e.g., Inoue et al., 2013). For example, in the vent sites of Japan, hard corals dominate at 225 μatm, become limited at 831 μatm and are absent at the highest pCO2 sites (1,465 μatm, Inoue et al., 2013). Similarly, in the Kimberley region, diverse coral communities dominated by branching Acropora exist in tide pools characterized by large diurnal temperature but not pH fluctuations (Dandan et al., 2015), whereas coral cover drops to <10% and Acropora ceases to exist on reef flats where extreme temperature, pH and oxygen fluctuations create a much more extreme environment (Gruber et al., 2017).
Changes in the ecological success of coral taxa can result in shifts in coral community composition that can change the entire reef function. For example, the reduction in coral diversity and dominance by a single taxa, Porites of the PNG vent site have reduced architectural complexity compared to adjacent reefs, where multiple coral species with a range of growth forms are present. A loss of reef structure and architectural complexity will compromise the ecological services provided (Rogers et al., 2015). The ability for coral taxa to provide reef accretion is a clear difference between several of the extreme and marginal coral environments; some have diverse coral reef systems e.g., the reef flats of Kimberley (Richards et al., 2015) and American Samoa (Craig et al., 2001), deep-reefs (Glynn and Morales, 1997) and reefs of the PAG (Sheppard, 2009). These environments demonstrate that the realized niche for many corals can in fact span a broad range of abiotic conditions (Schoepf et al., 2015). However, in some environments, non-framework building coral populations are described, e.g., in seagrass habitats of the Cayman Islands and the mangroves of the Seychelles and Indonesia (Camp et al., 2016a,b).
Understanding the interaction of physicochemical factors that result in critical thresholds is required to better predict, and ultimately manage changes in coral community structure, e.g., from accreting reef structures to isolated coral populations. Greater environmental characterization, along with information on the fitness trade-offs for coral populations to survive in sub-optimal environments (see Figure 2), will be needed to better resolve the thresholds and patterns underpinning survival (Table 1). Ultimately, it is likely that moving to a framework that considers the key functional traits conferring fitness (Madin et al., 2016; Suggett et al., 2017) may be required to overcome the limitations and complexities in attempting to reconcile commonalities based on taxonomic identity. Understanding mechanisms that confer survival will be necessary to identify the traits of interest (Suggett et al., 2017).
Mechanisms That Support Corals Surviving in Extremes
Some naturally extreme environments are well-studied with regards to the mechanisms that support survival, while other sites have only recently been discovered (Figure 2). Nevertheless, across environments various adaptive mechanisms seem evident. For example, genetic and physiological characterization across environments where corals have acquired high heat tolerance have shown it is typically the result of acclimatization and/or adaptation in both the coral host and algal symbionts. For example, many PAG corals host an endemic Symbiodinium species with high heat tolerance (Hume et al., 2015), but the coral host also contributes to heat tolerance via a superior ability to cope with oxidative stress (Howells et al., 2016b). Similarly, prevalence of clade D Symbiodinium is linked to heat tolerance in corals from American Samoa (Oliver and Palumbi, 2011b), but follow-up genomic and transcriptomic work has again highlighted the critical role of the coral host in terms of higher transcription of heat responsive genes and alleles pre-adapted to high temperatures (Barshis et al., 2013; Palumbi et al., 2014; Bay and Palumbi, 2015). Around the vent sites of PNG, changes in gene expression of Symbiodinium were highly variable across populations of A. millepora, whereas the host-response remained consistent (Kenkel et al., 2017). These findings suggest population-specific responses may exist, driven by differences in the holobiont community.
Capacity for phenotypic plasticity is also clearly critical in response to naturally extreme environmental conditions. For example, corals across high temperature, low pH, and multi-stressor sites were able to maintain high calcification rates (e.g., Dandan et al., 2015; Wall et al., 2016), enabled via mechanisms such as unimpaired pH-homeostasis at the site of calcification (Wall et al., 2016) or increased photosynthesis rates to supply the required energy demand (Strahl et al., 2015a). Although this is sometimes associated with trade-offs such as lower skeletal density and increased bioerosion (Smith et al., 2007; Crook et al., 2013), other corals are able to do so without indication of compromised energy budgets (Strahl et al., 2015a). Similarly, corals in mangrove habitats appear to enhance respiration rates indicative of increased heterotrophy, to facilitate survival in this challenging habitat (Camp et al., 2017). Traits that can facilitate energy acquisition appear beneficial across environments as corals persist toward the tails of their fundamental niche (Sommer et al., 2014). Currently it is still being resolved how the long-term ability to cope with one stressor could impact the ability of corals to deal with other chronic stressors. For example, in the coral A. millepora at the PNG vent site, a downregulation of chaperones could increase its vulnerability to temperature stress (Kenkel et al., 2017). Understanding the interaction of multiple-stressors, across varying temporal scales, should be a research priority (Table 1).
For many environments significant knowledge gaps exist regarding the adaptive capacity of their coral population (Figure 2), and further research is required to identify how widespread phenotypic plasticity is across these extreme systems. In particular, it is poorly understood whether high physiological plasticity is ultimately an inheritable trait and thus reflects adaptation of specialist populations. In the PAG for example, the algal endosymbiont S. thermophilum demonstrated superior heat tolerance but only within the high saline waters of the PAG (D'Angelo et al., 2015), thus demonstrating that adaptation can constrain the acclimatization potential of well-adapted populations. Similar evidence comes from heat tolerant corals in American Samoa, where the genotype of the coral host limits the plasticity of physiological responses when faced with new environmental conditions (Barshis et al., 2010). Such “specialist” restrictions carry critical implications for the ability of naturally stress-resistant corals to cope with future climate change superimposed on already extreme physicochemical conditions. As a consequence, protecting existing biodiversity by maintaining the largest possible pool of potentially stress-tolerant genotypes, rather than solely the best adapted genotypes, will be crucial to provide sufficiently diverse genetic material for evolution to act on, and thus promote rapid adaptation to climate change (Hume et al., 2016).
The Potential of Refuge Environments
Refuge environments are likely to become increasingly important, particularly for species that are less capable of acclimating to environmental change. The current review shows that there are systems that could off set single stressors; for example, turbid reefs can provide refuge for corals during thermal stress events (van Woesik et al., 2012; Morgan et al., 2017; van Woesik and McCaffrey, 2017) whereas high-latitude reefs can provide refuge from rising ocean temperatures (Beger et al., 2014; Muir et al., 2015a). However, with the exception of the northern Red Sea (Fine et al., 2013), most refuge environments are highly debated, with inconsistent findings across geographic locations. This, in part, results from the heterogeneity of coral environments on the scale of individual reefs. For example, local hydrography likely explains why some deep reefs, but not others, are capable of seeding shallow reefs (van Oppen et al., 2011; Serrano et al., 2014). Similarly, differences in local physicochemical conditions see mangroves of the U.S. Virgin Islands considered a coral refuge, while mangrove systems in the Seychelles, Indonesia and in New Caledonia are not (Camp et al., 2016a, 2017).
Timing of buffering is also an important consideration at these potential refuge environments. Upwelling sites, for example, can only offset thermal stress if the pulse of cool upwelled water coincides with a stress-event, yet this is frequently not the case (Chollett et al., 2010). Similarly, seagrass environments can only positively enhance local seawater pH and Ωarag during the day, with the opposite occurring at night due to the dominance of respiration in the absence of photosynthesis (Schmalz and Swanson, 1969). Furthermore, environments that operate as a refuge against one abiotic variable may also enhance stress from another; for example, cool upwelled water often has a lower Ωarag, which can increase the threat of ocean acidification (Manzello, 2010). Similarly, lower light levels and Ωarag characteristic of high-latitude reefs, particularly during winter, could potentially become limiting for many physiological variables (Beger et al., 2014; Muir et al., 2015a,b).
A further complication of refuge environments is that we currently have only limited information on the ability of non-native coral populations to move into these systems. Although poleward range expansion of tropical coral has been documented in several geographically different locations (e.g., Yamano et al., 2011; Baird et al., 2012), such information for other potential refuge environments is currently lacking. Thus, we do not know if all corals could utilize potential refuge services as they may lack the traits needed to survive. For example, there is evidence that corals surviving on deep reefs require physiological plasticity to survive (Lesser et al., 2010; Cooper et al., 2011; Smith et al., 2017b). An ultimate unknown on the potential of refuge environments is how they will themselves change in the future, and ultimately whether favorable physicochemical conditions can be sustained (Shaw et al., 2013). What we do know is that our understanding of conditions optimal for refugia is increasing, but that the heterogeneous nature of reef systems challenges the ability for environments to act as refuge against multiple abiotic parameters. Effective refugia have recently been described by Kavousi and Keppel (2017) as needing to provide long-term buffering to multiple-stressors. As such, consideration could be given to environmental engineering to facilitate the suite of conditions required for effective refugia, e.g., artificial shading (Coelho et al., 2017).
Conclusions
The future structure and composition of coral communities will be shaped by the vulnerability of different species to climate change and local stressors. Protection of existing biodiversity must be a priority to help maintain the greatest pool of stress-resistant genotypes (Hume et al., 2016). Critical thresholds appear to be a commonality for coral populations within extreme coral environments, which could provide valuable information on the tipping-points beyond which coral reefs or coral populations cease to exist. Importantly, corals will need to be ecologically competitive despite the extreme prevailing conditions to maintain their form and function. This could be increasingly challenging if they experience reductions in growth, while other taxa they compete for space with flourish, e.g., photoautotrophs (Durako, 1994; Buapet et al., 2013; Hendriks et al., 2014). Slower growth not only limits reef formation, but it can also compromise recruitment success (Ortiz et al., 2014). The interaction between taxa will be important in shaping the realized niche of corals into the future as environmental conditions change. As such, caution should be taken in making simple projections on future reef form and function when only a limited number of species are considered (Mumby and Van Woesik, 2014).
Our collective assessment of extreme and marginal coral environments highlights that multiple factors appear important to coral survival in sub-optimal conditions, particularly phenotypic plasticity and ecological fitness traits that can facilitate energy acquisition. Consequently, unless corals can be protected by refuge environments, it will be the collective suite of available traits of the coral holobiont that determine its adaptive capabilities and ultimate survival. As such, understanding the full suite of traits available for corals to trade-off for survival is imperative to assessing the future vulnerability of species, and ultimately shifts in community structure and function (Table 1) (van Woesik et al., 2012). Caution must therefore be taken in assessing single traits and using these to make statements on acclimation and/or adaptive potential (e.g., Suggett et al., 2017).
While no single environment is a perfect analog to projected future climates (which themselves are uncertain), they consistently provide evidence of corals tolerant to stress conditions that could become the “norm” in the future, and that plasticity is often required to survive. Extreme coral populations provide key additional tools for researchers to use alongside laboratory studies and modeling, to better understand the future survival of coral reefs. Beside the value extreme coral environments offer for research, they may also provide additional management options for corals through refuge systems or by providing genetic stocks of corals preconditioned to extreme physicochemical conditions (Camp et al., 2016a). Current information on coral refuge environments remains highly-debated, but evidence suggests the northern Red Sea could provide some refuge for corals in the future (Fine et al., 2013). Reduction of emissions, in particular carbon dioxide, is undoubtedly required to preserve the future form, function and ecosystem services of coral reefs (Gattuso et al., 2015; Hughes et al., 2017). But there is also an increasing debate over the need to facilitate coral survival through proactive reef management, e.g., reef restoration and/or assisted evolution processes (e.g., Coles and Riegl, 2013; van Oppen et al., 2015; Oppen et al., 2017). The use of proactive reef management is highly debated and beyond this synthesis (see van Oppen et al., 2015; Oppen et al., 2017), but what is evident from these extreme environments is the presence of corals that have traits highly favorable for future survival. For many environments we still do not know how these traits may be conserved or lost as corals are moved to new environments, however evidence of heritable heat-tolerance (Palumbi et al., 2014) and conserved thermal tolerance (Hume et al., 2013) has been reported.
Despite current knowledge gaps, extreme coral environments may have an unrecognized (and disproportionally) high conservation value, due to the trait selection of resident coral populations adapted to extremes. Furthermore, extreme environments may offer genetic rescue (e.g., heat-tolerant genotypes across latitudes), which again gives them a high research and conservation value. As we move to identifying areas of high conservation priority to better target limited management resources, encompassing areas that have extreme elements may be crucial in maximizing diverse genetic populations.
Author Contributions
EC, DSu, and RR-M conceived the manuscript. EC and VS led the review and quantitative synthesis. LH conducted the data analysis and figure production. EC and VS led the writing of the manuscript with all authors contributing to the final manuscript preparation.
Funding
The contribution of EC and DSu to manuscript writing was through the Australian Research Council (ARC) Discovery grant DP160100271. EC, DSu and VS were also supported through Fonds Pacific Project No. 1722 (SPP 43-1-2017).
Conflict of Interest Statement
The authors declare that the research was conducted in the absence of any commercial or financial relationships that could be construed as a potential conflict of interest.
Supplementary Material
The Supplementary Material for this article can be found online at: https://www.frontiersin.org/articles/10.3389/fmars.2018.00004/full#supplementary-material
Supplementary Table 1. Literature used in the quantitative synthesis for each environment.
Supplementary Figure 1. Quantitative assessment of the abiotic conditions experienced at the different extreme and marginal environments. Data is shown for studies out of the 285 assessed that reported the maximum, minimum, or range in (A,B) temperature, (C,D) temperature and pH, and (E) temperature, pH, AT, salinity, and oxygen. Data is shown per season and environment. Numbers correspond to the studies where data was obtained (detailed in Supplementary Table 1).
References
Abdo, D. A., Bellchambers, L. M., and Evans, S. N. (2012). Turning up the heat: increasing temperature and coral bleaching at the high latitude coral reefs of the Houtman Abrolhos Islands. PLoS ONE 7:e43878. doi: 10.1371/journal.pone.0043878
Ainsworth, T. D., Heron, S. F., Ortiz, J. C., Mumby, P. J., Grech, A., Ogawa, et al. (2016). Climate change disables coral bleaching protection on the Great Barrier Reef. Science 352, 338–342. doi: 10.1126/science.aac7125
Albright, R., Langdon, C., and Anthony, K. R. N. (2013). Dynamics of seawater carbonate chemistry, production, and calcification of a coral reef flat, central Great Barrier Reef. Biogeoscience 10, 6747. doi: 10.5194/bg-10-6747-2013
Andersson, A. J., Yeakel, K. L., Bates, N. R., and De Putron, S. J. (2014). Partial offsets in ocean acidification from changing coral reef biogeochemistry. Nat. Clim. Change 4, 56. doi: 10.1038/nclimate2050
Anthony, K. R. N. (2000). Enhanced particle-feeding capacity of corals on turbid reefs (Great Barrier Reef, Australia). Coral Reefs 19, 59–67. doi: 10.1007/s003380050227
Anthony, K. R., and Fabricius, K. E. (2000). Shifting roles of heterotrophy and autotrophy in coral energetics under varying turbidity. J. Exp. Mar. Biol. Ecol. 252, 221–253. doi: 10.1016/S0022-0981(00)00237-9
Anthony, K. R., Connolly, S. R., and Hoegh-Guldberg, O. (2007). Bleaching, energetics, and coral mortality risk: effects of temperature, light, and sediment regime. Limnol. Oceanogr. 52, 716–726. doi: 10.4319/lo.2007.52.2.0716
Armstrong, R. A., Singh, H., Torres, J., Nemeth, R. S., Can, A., Roman, C., et al. (2006). Characterizing the deep insular shelf coral reef habitat of the Hind Bank marine conservation district (US Virgin Islands) using the Seabed autonomous underwater vehicle. Cont. Shelf Res. 26, 194–205. doi: 10.1016/j.csr.2005.10.004
Babcock, R. C., Wills, B. L., and Simpson, C. J. (1994). Mass spawning of corals on a high latitude coral reef. Coral Reefs 13, 161–169. doi: 10.1007/BF00301193
Baird, A. H., Sommer, B., and Madin, J. S. (2012). Pole-ward range expansion of Acropora spp. along the east coast of Australia. Coral Reefs 31, 1063–1063. doi: 10.1007/s00338-012-0928-6
Barkley, H. C., Barkley, H. C., Cohen, A. L., Golbuu, Y., Starczak, V. R., DeCarlo, T. M., et al. (2015). Changes in coral reef communities across a natural gradient in seawater pH. Sci. Adv. 1:e1500328. doi: 10.1126/sciadv.1500328
Barshis, D. J., Ladner, J. T., Oliver, T. A., Seneca, F. O., Traylor-Knowles, N., and Palumbi, S. R. (2013). Genomic basis for coral resilience to climate change. Proc. Natl. Acad. Sci. U.S.A. 110, 1387–1392. doi: 10.1073/pnas.1210224110
Barshis, D. J., Stillman, J. H., Gates, R. D., Toonen, R. J., Smith, L. W., and Birkeland, C. (2010). Protein expression and genetic structure of the coral Porites lobata in an environmentally extreme Samoan back reef: does host genotype limit phenotypic plasticity? Mol. Ecol. 19, 1705–1720. doi: 10.1111/j.1365-294X.2010.04574.x
Bay, R. A., and Palumbi, S. R. (2015). Rapid acclimation ability mediated by transcriptome changes in reef-building corals. Genome Biol. Evol. 7, 1602–1612. doi: 10.1093/gbe/evv085
Bay, R. A., and Palumbi, S. R. (2014). Multilocus adaptation associated with heat resistance in reef-building corals. Curr. Biol. 24, 2952–2956. doi: 10.1016/j.cub.2014.10.044
Beddows, P. A., Smart, P. L., Whitaker, F. F., and Smith, S. L. (2002). Density stratified groundwater circulation on the Caribbean coast of the Yucatan peninsula, Mexico. Front. Karst Res. 7, 129–134.
Beger, M., Sommer, B., Harrison, P. L., Smith, S. D., and Pandolfi, J. M. (2014). Conserving potential coral reef refuges at high latitudes. Divers. Distrib. 20, 245–257. doi: 10.1111/ddi.12140
Bento, R., Hoey, A. S., Bauman, A. G., Feary, D. A., and Burt, J. A. (2016). The implications of recurrent disturbances within the world's hottest coral reef. Mar. Poll. Bull. 105, 466–472. doi: 10.1016/j.marpolbul.2015.10.006
Benzoni, F., Bianchi, C. N., and Morri, C. (2003). Coral communities of the northwestern Gulf of Aden (Yemen): variation in framework building related to environmental factors and biotic conditions. Coral Reefs 22, 475–484. doi: 10.1007/s00338-003-0342-1
Bessell-Browne, P., Stat, M., Thomson, D., and Clode, P. L. (2014). Coscinaraea marshae corals that have survived prolonged bleaching exhibit signs of increased heterotrophic feeding. Coral Reefs 33, 795–804. doi: 10.1007/s00338-014-1156-z
Bijma, J., Pörtner, H. O., Yesson, C., and Rogers, A. D. (2013). Climate change and the oceans–What does the future hold? Mar. Poll. Bull. 74, 495–505. doi: 10.1016/j.marpolbul.2013.07.022
Bonduriansky, R., and Day, T. (2009). Nongenetic inheritance and its evolutionary implications. Annu. Rev. Ecol. Evol. Syst. 40, 103–125. doi: 10.1146/annurev.ecolsys.39.110707.173441
Bongaerts, P., Frade, P. R., Hay, K. B., Englebert, N., Latijnhouwers, K. R., Bak, R. P., et al. (2015). Deep down on a Caribbean reef: lower mesophotic depths harbor a specialized coral-endosymbiont community. Sci. Rep. 5:7652. doi: 10.1038/srep07652
Bongaerts, P., Riginos, C., Brunner, R., Englebert, N., Smith, S. R., and Hoegh-Guldberg, O. (2017). Deep reefs are not universal refuges: reseeding potential varies among coral species. Sci. Adv. 3:e1602373. doi: 10.1126/sciadv.1602373
Bongaerts, P., Sampayo, E. M., Bridge, T. C., Ridgway, T., Vermeulen, F., Englebert, N., et al. (2011). Symbiodinium diversity in mesophotic coral communities on the Great Barrier Reef: a first assessment. Mar. Ecol. Prog. Ser. 439, 117–126. doi: 10.3354/meps09315
Booth, D. J., Figueira, W. F., Gregson, M. A., Brown, L., and Beretta, G. (2007). Occurrence of tropical fishes in temperate southeastern Australia: role of the East Australian Current. Estuar. Coast Shelf Sci. 72, 102–114. doi: 10.1016/j.ecss.2006.10.003
Bourne, D., Iida, Y., Uthicke, S., and Smith-Keune, C. (2007). Changes in coral-associated microbial communities during a bleaching event. ISME J. 2, 350–363. doi: 10.1038/ismej.2007.112
Bridge, T. C., Ferrari, R., Bryson, M., Hovey, R., Figueira, W. F., Williams, S. B., et al. (2014). Variable responses of benthic communities to anomalously warm sea temperatures on a high-latitude coral reef. PLoS ONE 9:e113079. doi: 10.1371/journal.pone.0113079
Buapet, P., Rasmusson, L. M., Gullström, M., and Björk, M. (2013). Photorespiration and carbon limitation determine productivity in temperate seagrasses. PLoS ONE 8:e83804. doi: 10.1371/journal.pone.0083804
Cacciapaglia, C., and van Woesik, R. (2015). Reef-coral refugia in a rapidly changing ocean. Global Change Biol. 21, 2272–2282. doi: 10.1111/gcb.12851
Camp, E. F., Nitschke, M. R., Rodolfo-Metalpa, R., Houlbreque, F., Gardner, S. G., Smith, D. J., et al. (2017). Reef-building corals thrive within hot-acidified and deoxygenated waters. Sci. Rep. 7:2434. doi: 10.1038/s41598-017-02383-y
Camp, E. F., Smith, D. J., Evenhuis, C., Enochs, I., Manzello, D., Woodcock, S., et al. (2016b). Acclimatization to high-variance habitats does not enhance physiological tolerance of two key Caribbean corals to future temperature and pH. Proc. R. Soc. B 283:20160442. doi: 10.1098/rspb.2016.0442
Camp, E. F., Suggett, D. J., Gendron, G., Jompa, J., Manfrino, C., and Smith, D. J. (2016a). Mangrove and seagrass beds provide different biogeochemical services for corals threatened by climate change. Front. Mar. Sci. 3:52. doi: 10.3389/fmars.2016.00052
Celliers, L., and Schleyer, M. H. (2002). Coral bleaching on high-latitude marginal reefs at Sodwana Bay, South Africa. Mar. Poll. Bull. 44, 1380–1387. doi: 10.1016/S0025-326X(02)00302-8
Chollett, I., Mumby, P. J., and Cortés, J. (2010). Upwelling areas do not guarantee refuge for coral reefs in a warming ocean. Mar. Ecol. Prog. Ser. 416, 47–56. doi: 10.3354/meps08775
Cinner, J. E., Huchery, C., MacNeil, M. A., Graham, N. A., McClanahan, T. R., Maina, J., et al. (2016). Bright spots among the world's coral reefs. Nature 535, 416–419. doi: 10.1038/nature18607
Coelho, V. R., Fenner, D., Caruso, C., Bayles, B. R., Huang, Y., and Birkeland, C. (2017). Shading as a mitigation tool for coral bleaching in three common Indo-Pacific species. J. Exp. Mar. Biol. Ecol. 497, 152–163. doi: 10.1016/j.jembe.2017.09.016
Cohen, A. L., and Holcomb, M. (2009). Why corals care about ocean acidification: uncovering the mechanism. Oceanography 22, 118–127. doi: 10.5670/oceanog.2009.102
Coles, S. L., and Riegl, B. M. (2013). Thermal tolerances of reef corals in the Gulf: a review of the potential for increasing coral survival and adaptation to climate change through assisted translocation. Mar. Poll. Bull. 72, 323–332. doi: 10.1016/j.marpolbul.2012.09.006
Comeau, S., Edmunds, P. J., Spindel, N. B., and Carpenter, R. C. (2014). Diel pCO2 oscillations modulate the response of the coral Acropora hyacinthus to ocean acidification. Mar. Ecol. Prog. Ser. 501, 99–111. doi: 10.3354/meps10690
Cooper, T. F., Ulstrup, K. E., Dandan, S. S., Heyward, A. J., Kühl, M., Muirhead, A., et al. (2011). Niche specialization of reef-building corals in the mesophotic zone: metabolic trade-offs between divergent Symbiodinium types. Proc. R. Soc. B. 278, 1840–1850. doi: 10.1098/rspb.2010.2321
Craig, P., Birkeland, C., and Belliveau, S. (2001). High temperatures tolerated by a diverse assemblage of shallow-water corals in American Samoa. Coral Reefs 20, 185–189. doi: 10.1007/s003380100159
Crook, E. D., Cohen, A. L., Rebolledo-Vieyra, M., Hernandez, L., and Paytan, A. (2013). Reduced calcification and lack of acclimatization by coral colonies growing in areas of persistent natural acidification. Proc. Natl. Acad. Sci. U.S.A. 110, 11044–11049. doi: 10.1073/pnas.1301589110
Crook, E. D., Potts, D., Rebolledo-Vieyra, M., Hernandez, L., and Paytan, A. (2012). Calcifying coral abundance near low-pH springs: implications for future ocean acidification. Coral Reefs 31, 239–245. doi: 10.1007/s00338-011-0839-y
Dandan, S. S., Falter, J. L., Lowe, R. J., and McCulloch, M. T. (2015). Resilience of coral calcification to extreme temperature variations in the Kimberley region, northwest Australia. Coral Reefs 34, 1151–1163. doi: 10.1007/s00338-015-1335-6
D'Angelo, C., Hume, B. C., Burt, J., Smith, E. G., Achterberg, E. P., and Wiedenmann, J. (2015). Local adaptation constrains the distribution potential of heat-tolerant Symbiodinium from the Persian/Arabian Gulf. ISME J. 9, 2551–2560. doi: 10.1038/ismej.2015.80
D'Croz, L., and Maté, J. L. (2004). Experimental responses to elevated water temperature in genotypes of the reef coral Pocillopora damicornis from upwelling and non-upwelling environments in Panama. Coral Reefs 23, 473–483. doi: 10.1007/s00338-004-0397-7
Denis, V., Mezaki, T., Tanaka, K., Kuo, C. Y., De Palmas, S., Keshavmurthy, S., et al. (2013). Coverage, diversity, and functionality of a high-latitude coral community (Tatsukushi, Shikoku Island, Japan). PLoS ONE 8:e54330. doi: 10.1371/journal.pone.0054330
Diaz-Pulido, G., and Garzón-Ferreira, J. (2002). Seasonality in algal assemblages on upwelling-influenced coral reefs in the Colombian Caribbean. Botan. Mar. 45, 284–292. doi: 10.1515/BOT.2002.028
Dixon, G. B., Davies, S. W., Aglyamova, G. V., Meyer, E., Bay, L. K., and Matz, M. V. (2015). Genomic determinants of coral heat tolerance across latitudes. Science 348, 1460–1462. doi: 10.1126/science.1261224
Doney, S. C., Ruckelshaus, M., Duffy, J. E., Barry, J. P., Chan, F., English, C. A., et al. (2012). Climate change impacts on marine ecosystems. Annu. Rev. Mar. Sci. 4, 11–37. doi: 10.1146/annurev-marine-041911-111611
Duarte, C. M., Hendriks, I. E., Moore, T. S., Olsen, Y. S., Steckbauer, A., Ramajo, L., et al. (2013). Is ocean acidification an open-ocean syndrome? Understanding anthropogenic impacts on seawater pH. Estuar. Coast. 36, 221–236. doi: 10.1007/s12237-013-9594-3
Dunn, J. G., Sammarco, P. W., and LaFleur, G. (2012). Effects of phosphate on growth and skeletal density in the scleractinian coral Acropora muricata: a controlled experimental approach. J. Exp. Mar. Biol. Ecol. 411, 34–44. doi: 10.1016/j.jembe.2011.10.013
Durako, M. J. (1994). Seagrass die-off in Florida Bay (USA): changes in shoot demographic characteristics and population dynamics in Thalassia testudinum. Mar. Ecol. Prog. Ser. 59–66. doi: 10.3354/meps110059
Eakin, C. M., Liu, G., Gomez, A. M., De La Cour, J. L., Heron, S. F., Skirving, W. J., et al. (2016). Global coral bleaching 2014–2017: status and an appeal for observations. Reef Encounter 31, 20–26.
Enochs, I. C., Manzello, D. P., Donham, E. M., Kolodziej, G., Okano, R., Johnston, L., et al. (2015). Shift from coral to macroalgae dominance on a volcanically acidified reef. Nat. Clim. Change 5, 1083–1088. doi: 10.1038/nclimate2758
Enochs, I. C., Manzello, D. P., Kolodziej, G., Noonan, S. H., Valentino, L., and Fabricius, K. E. (2016a). Enhanced macroboring and depressed calcification drive net dissolution at high-CO2 coral reefs. Proc. R. Soc. B 283:20161742. doi: 10.1098/rspb.2016.1742
Enochs, I. C., Manzello, D. P., Tribollet, A., Valentino, L., Kolodziej, G., Donham, E. M., et al. (2016b). Elevated colonization of microborers at a volcanically acidified coral reef. PLoS ONE 11:e0159818. doi: 10.1371/journal.pone.0159818
Enríquez, S., Méndez, E. R., Hoegh-Guldberg, O., and Iglesias-Prieto, R. (2017). Key functional role of the optical properties of coral skeletons in coral ecology and evolution. Proc. R. Soc. B 284:20161667. doi: 10.1098/rspb.2016.1667
Everitt, B., and Hothorn, T. (2011). An Introduction to Applied Multivariate Analysis with R. New York, NY: Springer.
Fabricius, K. E. (2005). Effects of terrestrial runoff on the ecology of corals and coral reefs: review and synthesis. Mar. Poll. Bull. 50, 125–146. doi: 10.1016/j.marpolbul.2004.11.028
Fabricius, K. E., De'ath, G., Noonan, S., and Uthicke, S. (2014). Ecological effects of ocean acidification and habitat complexity on reef-associated macroinvertebrate communities. Proc. R. Soc. B 281:20132479. doi: 10.1098/rspb.2013.2479
Fabricius, K. E., Kluibenschedl, A., Harrington, L., Noonan, S., and De'Ath, G. (2015). In situ changes of tropical crustose coralline algae along carbon dioxide gradients. Sci. Rep. 5:9537. doi: 10.1038/srep09537
Fabricius, K. E., Langdon, C., Uthicke, S., Humphrey, C., Noonan, S., De'ath, G., et al. (2011). Losers and winners in coral reefs acclimatized to elevated carbon dioxide concentrations. Nat. Clim. Change 1, 165. doi: 10.1038/nclimate1122
Fantazzini, P., Mengoli, S., Pasquini, L., Bortolotti, V., Brizi, L., Mariani, M., et al. (2015). Gains and losses of coral skeletal porosity changes with ocean acidification acclimation. Nat. Commun. 6:7785. doi: 10.1038/ncomms8785
Feely, R. A., Sabine, C. L., Hernandez-Ayon, J. M., Ianson, D., and Hales, B. (2008). Evidence for upwelling of corrosive “acidified” water onto the continental shelf. Science 320, 1490–1492. doi: 10.1126/science.1155676
Feely, R. A., Sabine, C. L., Lee, K., Berelson, W., Kleypas, J., Fabry, V. J., et al. (2004). Impact of anthropogenic CO2 on the CaCO3 system in the oceans. Science 305, 362–366. doi: 10.1126/science.1097329
Fine, M., Gildor, H., and Genin, A. (2013). A coral reef refuge in the Red Sea. Global Change Biol. 19, 3640–3647. doi: 10.1111/gcb.12356
Frade, P. R., Bongaerts, P., Winkelhagen, A. J. S., Tonk, L., and Bak, R. P. M. (2008). In situ photobiology of corals over large depth ranges: a multivariate analysis on the roles of environment, host, and algal symbiont. Limnol. Oceanogr. 53, 2711–2723. doi: 10.4319/lo.2008.53.6.2711
Gattuso, J. P., Magnan, A., Billé, R., Cheung, W. W., Howes, E. L., Joos, F., et al. (2015). Contrasting futures for ocean and society from different anthropogenic CO2 emissions scenarios. Science 349:aac4722. doi: 10.1126/science.aac4722
Glynn, P. W., and Morales, G. E. L. (1997). Coral reefs of Huatulco, West Mexico: reef development in upwelling Gulf of Tehuantepec. Rev. Biol. Trop. 45, 1033–1047
Gray, S. E., DeGrandpre, M. D., Langdon, C., and Corredor, J. E. (2012). Short-term and seasonal pH, pCO2 and saturation state variability in a coral-reef ecosystem. Glob. Biogeochem. Cycles 26:GB3012. doi: 10.1029/2011GB004114
Grottoli, A. G., Tchernov, D., and Winters, G. (2017). Physiological and biogeochemical responses of super-corals to thermal stress from the Northern Gulf of Aqaba, Red Sea. Front. Mar. Sci. 4:215. doi: 10.3389/fmars.2017.00215
Gruber, R. K., Lowe, R. J., and Falter, J. L. (2017). Metabolism of a tide-dominated reef platform subject to extreme diel temperature and oxygen variations. Limnol. Oceanogr. 62, 1701–1717. doi: 10.1002/lno.10527
Grupstra, C. G. B., Coma, R., Ribes, M., Leydet, K. P., Parkinson, J. E., McDonald, K., et al. (2017). Evidence for coral range expansion accompanied by reduced diversity of Symbiodinium genotypes. Coral Reefs 36, 981–985. doi: 10.1007/s00338-017-1589-2
Guadayol, Ò., Silbiger, N. J., Donahue, M. J., and Thomas, F. I. (2014). Patterns in temporal variability of temperature, oxygen and pH along an environmental gradient in a coral reef. PLoS ONE 9:e85213. doi: 10.1371/journal.pone.0085213
Guinotte, J. M., and Fabry, V. J. (2008). Ocean acidification and its potential effects on marine ecosystems. Ann. N. Y. Acad. Sci. 1134, 320–342. doi: 10.1196/annals.1439.013
Guinotte, J. M., Buddemeier, R. W., and Kleypas, J. A. (2003). Future coral reef habitat marginality: temporal and spatial effects of climate change in the Pacific basin. Coral Reefs 22, 551–558. doi: 10.1007/s00338-003-0331-4
Hadaidi, G., Röthig, T., Yum, L. K., Ziegler, M., Arif, C., Roder, C., et al. (2017). Stable mucus-associated bacterial communities in bleached and healthy corals of Porites lobata from the Arabian Seas. Sci. Rep. 7:45362. doi: 10.1038/srep45362
Hall-Spencer, J. M., Rodolfo-Metalpa, R., Martin, S., Ransome, E., Fine, M., Turner, S. M., et al. (2008). Volcanic carbon dioxide vents show ecosystem effects of ocean acidification. Nature 454, 96–99. doi: 10.1038/nature07051
Harriott, V. J., Smith, D. A. S., and Harrison, P. L. (1994). Patterns of coral community structure of subtropical reefs in the Solitary Islands Marine Reserve, Eastern Australia. Mar. Ecol. Prog. Ser. 67–76. doi: 10.3354/meps109067
Harrison, P. L., Dalton, S. J., and Carroll, A. G. (2011). Extensive coral bleaching on the world's southernmost coral reef at Lord Howe Island, Australia. Coral Reefs 30, 775. doi: 10.1007/s00338-011-0778-7
Hendriks, I. E., Olsen, Y. S., Ramajo, L., Basso, L., Steckbauer, A., Moore, T. S., et al. (2014). Photosynthetic activity buffers ocean acidification in seagrass meadows. Biogeoscience 11, 333. doi: 10.5194/bg-11-333-2014
Hennige, S. J., Smith, D. J., Perkins, R., Consalvey, M., Paterson, D. M., and Suggett, D. J. (2008). Photoacclimation, growth and distribution of massive coral species in clear and turbid waters. Mar. Ecol. Prog. Ser. 369, 77–88. doi: 10.3354/meps07612
Hennige, S. J., Smith, D. J., Walsh, S. J., McGinley, M. P., Warner, M. E., and Suggett, D. J. (2010). Acclimation and adaptation of scleractinian coral communities along environmental gradients within an Indonesian reef system. J. Exp. Mar Biol. Ecol. 391, 143–152. doi: 10.1016/j.jembe.2010.06.019
Heron, S. F., Maynard, J. A., van Hooidonk, R., and Eakin, M. C. (2016). Warming trends and bleaching stress of the World's coral reefs 1985–2012. Sci. Rep. 6:38402. doi: 10.1038/srep38402
Hofmann, G. E., Smith, J. E., Johnson, K. S., Send, U., Levin, L. A., Micheli, F., et al. (2011). High-frequency dynamics of ocean pH: a multi-ecosystem comparison. PLoS ONE 6:e28983. doi: 10.1371/journal.pone.0028983
Hooidonk, R., Maynard, J. A., Manzello, D., and Planes, S. (2014). Opposite latitudinal gradients in projected ocean acidification and bleaching impacts on coral reefs. Global Change Biol. 20, 103–112.
Howells, E. J., Abrego, D., Meyer, E., Kirk, N. L., and Burt, J. A. (2016b). Host adaptation and unexpected symbiont partners enable reef-building corals to tolerate extreme temperatures. Global Change Biol. 22, 2702–2714. doi: 10.1111/gcb.13250
Howells, E. J., Beltran, V. H., Larsen, N. W., Bay, L. K., Willis, B. L., and Van Oppen, M. J. H. (2012). Coral thermal tolerance shaped by local adaptation of photosymbionts. Nat. Clim. Change 2, 116–120. doi: 10.1038/nclimate1330
Howells, E. J., Berkelmans, R., van Oppen, M. J., Willis, B. L., and Bay, L. K. (2013). Historical thermal regimes define limits to coral acclimatization. Ecology 94, 1078–1088. doi: 10.1890/12-1257.1
Howells, E. J., Ketchum, R. N., Bauman, A. G., Mustafa, Y., Watkins, K. D., and Burt, J. A. (2016a). Species-specific trends in the reproductive output of corals across environmental gradients and bleaching histories. Mar. Poll. Bull. 105, 532–539. doi: 10.1016/j.marpolbul.2015.11.034
Hughes, T. P., Kerry, J. T., Álvarez-Noriega, M., Álvarez-Romero, J. G., Anderson, K. D., Baird, A. H., et al. (2017). Global warming and recurrent mass bleaching of corals. Nature 543, 373–377. doi: 10.1038/nature21707
Hume, B. C., Voolstra, C. R., Arif, C., D'Angelo, C., Burt, J. A., Eyal, G., et al. (2016). Ancestral genetic diversity associated with the rapid spread of stress-tolerant coral symbionts in response to Holocene climate change. Proc. Natl. Acad. Sci. U.S.A. 113, 4416–4421. doi: 10.1073/pnas.1601910113
Hume, B., D'angelo, C., Burt, J., Baker, A. C., Riegl, B., and Wiedenmann, J. (2013). Corals from the Persian/Arabian Gulf as models for thermotolerant reef-builders: prevalence of clade C3 Symbiodinium, host fluorescence and ex situ temperature tolerance. Mar. Poll. Bull. 72, 313–322. doi: 10.1016/j.marpolbul.2012.11.032
Hume, B., D'Angelo, C., Smith, E. G., Stevens, J. R., Burt, J., and Wiedenmann, J. (2015). Symbiodinium thermophilum sp. nov., a thermotolerant symbiotic alga prevalent in corals of the world's hottest sea, the Persian/Arabian Gulf. Sci. Rep. 5:562. doi: 10.1038/srep08562
Hutchinson, G. E. (1959). Homage to Santa Rosalia, or Why are there so many kinds of animals? Am. Nat. 93, 145–159. doi: 10.1086/282070
Iglesias-Prieto, R., Galindo-Martínez, C. T., Enríquez, S., and Carricart-Ganivet, J. P. (2014). Attributing reductions in coral calcification to the saturation state of aragonite, comments on the effects of persistent natural acidification. Proc. Natl. Acad. Sci. U.S.A. 111, E300–E301. doi: 10.1073/pnas.1318521111
Ingvarsson, P. K. (2001). Restoration of genetic variation lost–the genetic rescue hypothesis. Trends Ecol. Evol. 16, 62–63. doi: 10.1016/S0169-5347(00)02065-6
Inoue, S., Kayanne, H., Yamamoto, S., and Kurihara, H. (2013). Spatial community shift from hard to soft corals in acidified water. Nat. Clim. Change 3, 683. doi: 10.1038/nclimate1855
Invers, O., Romero, J., and Pérez, M. (1997). Effects of pH on seagrass photosynthesis: a laboratory and field assessment. Aquat. Bot. 59, 185–194. doi: 10.1016/S0304-3770(97)00072-7
IPCC (2014). Climate Change 2014: Synthesis Report. Contribution of Working Groups I, II and III to the Fifth Assessment Report of the Intergovernmental Panel on Climate Change, eds Core Writing Team, R. K. Pachauri and L. A. Meyer (Geneva: IPCC), 151.
Jackson, J. B. C. (1986). “Distribution and ecology of clonal and aclonal benthic invertebrates,” in Population Biology and Evolution of Clonal Organisms, eds J. B. C. Jackson, L. W. Buss, R. E. Cook (New Haven, CT: Yale University Press), 297–356.
Jiménez, C., and Cortés, J. (2003). Growth of seven species of scleractinian corals in an upwelling environment of the eastern Pacific (Golfo de Papagayo, Costa Rica). Bull. Mar. Sci. 72, 187–198.
Kavousi, J., and Keppel, G. (2017). Clarifying the concept of climate change refugia for coral reefs. ICES J. Mar. Sci. doi: 10.1093/icesjms/fsx124
Kavousi, J., Tavakoli-Kolour, P., Mohammadizadeh, M., Bahrami, A., and Barkhordari, A. (2014). Mass coral bleaching in the Northern Persian Gulf, 2012. Sci. Mar. 78, 397–404. doi: 10.3989/scimar.03914.16A
Kenkel, C. D., Moya, A., Strahl, J., Humphrey, C., and Bay, L. K. (2017). Functional genomic analysis of corals from natural CO2-seeps reveals core molecular responses involved in acclimatization to ocean acidification. Global Change Biol. 24, 158–171. doi: 10.1101/112045
Keppel, G., and Wardell-Johnson, G. W. (2012). Refugia: keys to climate change management. Global Change Biol. 18, 2389–2391. doi: 10.1111/j.1365-2486.2012.02729.x
Kleypas, J. A., McManus, J. W., and Me-ez, L. A. (1999). Environmental limits to coral reef development: where do we draw the line? Am. Zool. 39, 146–159. doi: 10.1093/icb/39.1.146
Kline, D. I., Teneva, L., Hauri, C., Schneider, K., Miard, T., Chai, A., et al. (2015). Six month in situ high-resolution carbonate chemistry and temperature study on a coral reef flat reveals asynchronous pH and temperature anomalies. PLoS ONE 10:e0127648. doi: 10.1371/journal.pone.0127648
Koweek, D. A., Dunbar, R. B., Monismith, S. G., Mucciarone, D. A., Woodson, C. B., and Samuel, L. (2015). High-resolution physical and biogeochemical variability from a shallow back reef on Ofu, American Samoa: an end-member perspective. Coral Reefs 34, 979–991. doi: 10.1007/s00338-015-1308-9
Kroeker, K. J., Kordas, R. L., Crim, R., Hendriks, I. E., Ramajo, L., Singh, G. S., et al. (2013). Impacts of ocean acidification on marine organisms: quantifying sensitivities and interaction with warming. Global Change Biol. 19, 1884–1896. doi: 10.1111/gcb.12179
Kroeker, K. J., Micheli, F., Gambi, M. C., and Martz, T. R. (2011). Divergent ecosystem responses within a benthic marine community to ocean acidification. Proc. Natl. Acad. Sci. U.S.A. 108, 14515–14520. doi: 10.1073/pnas.1107789108
Krueger, T., Horwitz, N., Bodin, J., Giovani, M. E., Escrig, S., Meibom, A., et al. (2017). Common reef-building coral in the Northern Red Sea resistant to elevated temperature and acidification. R. Soc. Open Sci. 4:170038. doi: 10.1098/rsos.170038
Langdon, C., and Atkinson, M. J. (2005). Effect of elevated pCO2 on photosynthesis and calcification of corals and interactions with seasonal change in temperature/irradiance and nutrient enrichment. J. Geophy. Res. Oceans 110:C09S07. doi: 10.1029/2004JC002576
Le Nohaïc, M., Ross, C. L., Cornwall, C. E., Comeau, S., Lowe, R., McCulloch, M. L., et al. (2017). Marine heatwave causes unprecedented regional mass bleaching of thermally resistant corals in northwest Australia. Sci. Rep. 7:14999. doi: 10.1038/s41598-017-14794-y
Lesser, M. P., Slattery, M., Stat, M., Ojimi, M., Gates, R. D., Grottoli, A., et al. (2010). Photoacclimatization by the coral Montastrea cavernosa in the mesophotic zone: light, food, and genetics. Ecology 91, 990–1003. doi: 10.1890/09-0313.1
Little, A. F., Van Oppen, M. J., and Willis, B. L. (2004). Flexibility in algal endosymbioses shapes growth in reef corals. Science 304, 1492–1494. doi: 10.1126/science.1095733
Lohr, K. E., Smith, D. J., Suggett, D. J., Nitschke, M. R., Dumbrell, A. J., Woodcock, S., et al. (2017). Coral community structure and recruitment in seagrass meadows. Front. Mar. Sci. 4:388. doi: 10.3389/fmars.2017.00388
Lowe, R. J., Pivan, X., Falter, J., Symonds, G., and Gruber, R. (2016). Rising sea levels will reduce extreme temperature variations in tide-dominated reef habitats. Sci. Adv. 2:e1600825. doi: 10.1126/sciadv.1600825
Loya, Y., Sakai, K., Yamazato, K., Nakano, Y., Sambali, H., and van Woesik, R. (2001). Coral bleaching: the winners and the losers. Ecol. Lett. 4, 122–131. doi: 10.1046/j.1461-0248.2001.00203.x
Madin, J. S., Hoogenboom, M. O., Connolly, S. R., Darling, E. S., Falster, D. S., Huang, D., et al. (2016). A trait-based approach to advance coral reef science. Trends Ecol. Evol. 31, 419–428. doi: 10.1016/j.tree.2016.02.012
Madsen, A., Madin, J. S., Tan, C. H., and Baird, A. H. (2014). The reproductive biology of the scleractinian coral Plesiastrea versipora in Sydney Harbour, Australia. SEDAO 1, 25–33. doi: 10.3354/sedao00004
Manzello, D. P. (2010). Ocean acidification hotspots: spatiotemporal dynamics of the seawater CO2 system of eastern Pacific coral reefs. Limnol. Oceanogr. 55, 239–248. doi: 10.4319/lo.2010.55.1.0239
Manzello, D. P., Enochs, I. C., Bruckner, A., Renaud, P. G., Kolodziej, G., Budd, D. A., et al. (2014). Galápagos coral reef persistence after ENSO warming across an acidification gradient. Geophys. Res. Lett. 41, 9001–9008. doi: 10.1002/2014GL062501
Manzello, D. P., Enochs, I. C., Melo, N., Gledhill, D. K., and Johns, E. M. (2012). Ocean acidification refugia of the Florida Reef Tract. PLoS ONE 7:e41715. doi: 10.1371/journal.pone.0041715
Manzello, D. P., Kleypas, J. A., Budd, D. A., Eakin, C. M., Glynn, P. W., and Langdon, C. (2008). Poorly cemented coral reefs of the eastern tropical Pacific: possible insights into reef development in a high-CO2 world. Proc. Natl. Acad. Sci. U.S.A. 105, 10450–10455. doi: 10.1073/pnas.0712167105
Martin, S., Rodolfo-Metalpa, R., Ransome, E., Rowley, S., Buia, M. C., Gattuso, J. P., et al. (2008). Effects of naturally acidified seawater on seagrass calcareous epibionts. Biol. Lett. 4, 689–692. doi: 10.1098/rsbl.2008.0412
McCulloch, M., Falter, J., Trotter, J., and Montagna, P. (2012). Coral resilience to ocean acidification and global warming through pH up-regulation. Nat. Clim. Change 2, 623. doi: 10.1038/nclimate1473
Milazzo, M., Rodolfo-Metalpa, R., San Chan, V. B., Fine, M., Alessi, C., Thiyagarajan, et al. (2014). Ocean acidification impairs vermetid reef recruitment. Sci. Rep. 4:4189. doi: 10.1038/srep04189
Miller, K. J., and Ayre, D. J. (2004). The role of sexual and asexual reproduction in structuring high latitude populations of the reef coral Pocillopora damicornis. Heredity 92, 557–568. doi: 10.1038/sj.hdy.6800459
Millero, F. J., Hiscock, W. T., Huang, F., Roche, M., and Zhang, J. Z. (2001). Seasonal variation of the carbonate system in Florida Bay. Bull. Mar. Sci. 68, 101–123.
Morgan, K. M., Perry, C. T., Johnson, J. A., and Smithers, S. G. (2017). Nearshore turbid-zone corals exhibit high bleaching tolerance on the Great Barrier Reef following the 2016 ocean warming event. Front. Mar. Sci. 4:224. doi: 10.3389/fmars.2017.00224
Morgan, K. M., Perry, C. T., Smithers, S. G., Johnson, J. A., and Daniell, J. J. (2016). Evidence of extensive reef development and high coral cover in nearshore environments: implications for understanding coral adaptation in turbid settings. Sci. Rep. 6:29616. doi: 10.1038/srep29616
Morin, X., and Thuiller, W. (2009). Comparing niche-and process-based models to reduce prediction uncertainty in species range shifts under climate change. Ecology 90, 1301–1313. doi: 10.1890/08-0134.1
Morrow, K. M., Bourne, D. G., Humphrey, C., Botté, E. S., Laffy, P., Zaneveld, J., et al. (2014). Natural volcanic CO2 seeps reveal future trajectories for host–microbial associations in corals and sponges. ISME J. 9, 894. doi: 10.1038/ismej.2014.188
Muir, P. R., Wallace, C. C., Done, T., and Aguirre, J. D. (2015a). Limited scope for latitudinal extension of reef corals. Science 348, 1135–1138. doi: 10.1126/science.1259911
Muir, P. R., Wallace, C., Bridge, T. C., and Bongaerts, P. (2015b). Diverse staghorn coral fauna on the mesophotic reefs of north-east Australia. PLoS ONE 10:e0117933. doi: 10.1371/journal.pone.0117933
Mumby, P. J., and Van Woesik, R. (2014). Consequences of ecological, evolutionary and biogeochemical uncertainty for coral reef responses to climatic stress. Curr. Biol. 24, R413–R423. doi: 10.1016/j.cub.2014.04.029
Mumby, P. J., Chisholm, J. R., Edwards, A. J., Andrefouet, S., and Jaubert, J. (2001). Cloudy weather may have saved Society Island reef corals during the 1998 ENSO event. Mar. Ecol. Prog. Ser. 222, 209–216. doi: 10.3354/meps222209
Okazaki, R. R., Swart, P. K., and Langdon, C. (2013). Stress-tolerant corals of Florida Bay are vulnerable to ocean acidification. Coral Reefs 32, 671–683. doi: 10.1007/s00338-013-1015-3
Oliver, T. A., and Palumbi, S. R. (2009). Distributions of stress-resistant coral symbionts match environmental patterns at local but not regional scales. Mar. Ecol. Prog. Ser. 378, 103. doi: 10.3354/meps07871
Oliver, T. A., and Palumbi, S. R. (2011a). Do fluctuating temperature environments elevate coral thermal tolerance? Coral Reefs 30, 429–440. doi: 10.1007/s00338-011-0721-y
Oliver, T. A., and Palumbi, S. R. (2011b). Many corals host thermally resistant symbionts in high-temperature habitat. Coral Reefs 30, 241–250. doi: 10.1007/s00338-010-0696-0
Oppen, M. J., Gates, R. D., Blackall, L. L., Cantin, N., Chakravarti, L. J., Chan, W. Y., et al. (2017). Shifting paradigms in restoration of the world's coral reefs. Global Change Biol. 23, 3437–3448. doi: 10.1111/gcb.13647
Ortiz, J. C., González-Rivero, M., and Mumby, P. J. (2014). An ecosystem-level perspective on the host and symbiont traits needed to mitigate climate change impacts on Caribbean coral reefs. Ecosystems 17, 1–13. doi: 10.1007/s10021-013-9702-z
Palardy, J. E. E., Grottoli, A. G., and Matthews, K. A. (2005). Effects of upwelling, depth, morphology and polyp size on feeding in three species of Panamanian corals. Mar. Ecol. Prog. Ser. 300, 79–89. doi: 10.3354/meps300079
Palumbi, S. R., Barshis, D. J., Traylor-Knowles, N., and Bay, R. A. (2014). Mechanisms of reef coral resistance to future climate change. Science 344, 895–898. doi: 10.1126/science.1251336
Paytan, A., Crook, E. D., Cohen, A. L., Martz, T. R., Takashita, Y., Rebolledo-Vieyra, M., et al. (2014). Combined field and laboratory approaches for the study of coral calcification. Proc. Natl. Acad. Sci. U.S.A. 111, E302–E303. doi: 10.1073/pnas.1319572111
Pedersen, O., Colmer, T. D., Borum, J., Zavala-Perez, A., and Kendrick, G. A. (2016). Heat stress of two tropical seagrass species during low tides – impact on underwater net photosynthesis, dark respiration and diel in situ internal aeration. New Phytol. 210, 1207–1218. doi: 10.1111/nph.13900
Perry, C. T., and Larcombe, P. (2003). Marginal and non-reef-building coral environments. Coral Reefs 22, 427–432. doi: 10.1007/s00338-003-0330-5
Pettay, D. T., Wham, D. C., Smith, R. T., Iglesias-Prieto, R., and LaJeunesse, T. C. (2015). Microbial invasion of the Caribbean by an Indo-Pacific coral zooxanthella. Proc. Natl. Acad. Sci. U.S.A.112, 7513–7518. doi: 10.1073/pnas.1502283112
Pigliucci, M., Murren, C. J., and Schlichting, C. D. (2006). Phenotypic plasticity and evolution by genetic assimilation. J. Exp. Biol. 209, 2362–2367. doi: 10.1242/jeb.02070
Potts, D. C., and Jacobs, J. R. (2000). “Evolution of reef-building scleractinian corals in turbid environments: a paleo-ecological hypothesis,” in Proceedings 9th International Coral Reef Symposium (Bali), 249–254.
Price, N. N., Martz, T. R., Brainard, R. E., and Smith, J. E. (2012). Diel variability in seawater pH relates to calcification and benthic community structure on coral reefs. PLoS ONE 7:e43843. doi: 10.1371/journal.pone.0043843
Purkis, S. J., Rowlands, G. P., Riegl, B. M., and Renaud, P. G. (2010). The paradox of tropical karst morphology in the coral reefs of the arid Middle East. Geology 38, 227–230. doi: 10.1130/G.30710.1
R Core Team (2017). R: A Language and Environment for Statistical Computing. Vienna: R Foundation for Statistical Computing. Available online at://www.R-project.org/
Richards, Z. T., Garcia, R. A., Wallace, C. C., Rosser, N. L., and Muir, P. R. (2015). A diverse assemblage of reef corals thriving in a dynamic intertidal reef setting (Bonaparte archipelago, Kimberley, Australia). PLoS ONE 10:e0117791. doi: 10.1371/journal.pone.0117791
Riegl, B., and Piller, W. E. (2003). Possible refugia for reefs in times of environmental stress. Int. J. Earth Sci. 92, 520–531. doi: 10.1007/s00531-003-0328-9
Riegl, B., and Purkis, S. J. (2012). Coral Reefs of the Gulf: Adaptation to Environmental Extremes. Dordrecht: Springer.
Rodolfo-Metalpa, R., Hoogenboom, M. O., Rottier, C., Ramos-Esplá, A., Baker, A. C., Fine, M., et al. (2014). Thermally tolerant corals have limited capacity to acclimatize to future warming. Global Change Biol. 20, 3036–3049. doi: 10.1111/gcb.12571
Rodolfo-Metalpa, R., Houlbrèque, F., Tambutté, É., Boisson, F., Baggini, C., Patti, F. P., et al. (2011). Coral and mollusc resistance to ocean acidification adversely affected by warming. Nat. Clim. Change 1, 308. doi: 10.1038/nclimate1200
Rogers, A., Harborne, A. R., Brown, C. J., Bozec, Y. M., Castro, C., Chollett, I., et al. (2015). Anticipative management for coral reef ecosystem services in the 21st century. Global Change Biol. 21, 504–514. doi: 10.1111/gcb.12725
Rohatgi, A. (2017). Web Plot Digitizer V3.12, Texas, USA. Available online at: http://arohatgi.info/WebPlotDigitizer
Ross, C. L., Falter, J. L., Schoepf, V., and McCulloch, M. T. (2015). Perennial growth of hermatypic corals at Rottnest Island, Western Australia (32 S). Peer J. 3:e781. doi: 10.7717/peerj.781
Rosser, N. L., and Veron, J. E. N. (2011). Australian corals thriving out of water in an extreme environment. Coral Reefs 30, 21–21. doi: 10.1007/s00338-010-0689-z
Santos, I. R., Glud, R. N., Maher, D., Erler, D., and Eyre, B. D. (2011). Diel coral reef acidification driven by porewater advection in permeable carbonate sands, Heron Island, Great Barrier Reef. Geophys. Res. Lett. 38:L03604. doi: 10.1029/2010GL046053
Schmalz, R. F., and Swanson, F. J. (1969). Diurnal variations in the carbonate saturation of seawater. J. Sedim. Res. 39, 255–267.
Schmidtko, S., Stramma, L., and Visbeck, M. (2017). Decline in global oceanic oxygen content during the past five decades. Nature 542, 335–339. doi: 10.1038/nature21399
Schoepf, V., Stat, M., Falter, J. L., and McCulloch, M. T. (2015). Limits to the thermal tolerance of corals adapted to a highly fluctuating, naturally extreme temperature environment. Sci. Rep. 5:17639. doi: 10.1038/srep17639
Serrano, X., Baums, I. B., O'Reilly, K., Smith, T. B., Jones, R. J., Shearer, T. L., et al. (2014). Geographic differences in vertical connectivity in the Caribbean coral Montastrea cavernosa despite high levels of horizontal connectivity at shallow depths. Mol. Ecol. 23, 4226–4240. doi: 10.1111/mec.12861
Shamberger, K. E., Cohen, A. L., Golbuu, Y., McCorkle, D. C., Lentz, S. J., and Barkley, H. C. (2014). Diverse coral communities in naturally acidified waters of a Western Pacific reef. Geophys. Res. Lett. 41, 499–504. doi: 10.1002/2013GL058489
Shaw, E. C., McNeil, B. I., and Tilbrook, B. (2012). Impacts of ocean acidification in naturally variable coral reef flat ecosystems. J. Geophys. Res. Oceans 117:C03038. doi: 10.1029/2011JC007655
Shaw, E. C., Munday, P. L., and McNeil, B. I. (2013). The role of CO2 variability and exposure time for biological impacts of ocean acidification. Geophys. Res. Lett. 40, 4685–4688. doi: 10.1002/grl.50883
Shaw, E. C., Phinn, S. R., Tilbrook, B., and Steven, A. (2015). Natural in situ relationships suggest coral reef calcium carbonate production will decline with ocean acidification. Limnol. Oceanogr. 60, 777–788. doi: 10.1002/lno.10048
Sheppard, C. (2009). Large temperature plunges recorded by data loggers at different depths on an Indian Ocean atoll: comparison with satellite data and relevance to coral refuges. Coral Reefs 28, 399–403. doi: 10.1007/s00338-009-0476-x
Sheppard, C. R. C., and Salm, R. V. (1988). Reef and coral communities of Oman, with a description of a new coral species (Order Scleractinia, genus Acanthastrea). J. Nat. History 22, 263–279. doi: 10.1080/00222938800770201
Smale, D. A., and Wernberg, T. (2012). Ecological observations associated with an anomalous warming event at the Houtman Abrolhos Islands, Western Australia. Coral Reefs 31, 441–441. doi: 10.1007/s00338-012-0873-4
Smith, E. G., D'Angelo, C., Sharon, Y., Tchernov, D., and Wiedenmann, J. (2017a). Acclimatization of symbiotic corals to mesophotic light environments through wavelength transformation by fluorescent protein pigments. Proc. R. Soc. B 284:20170320. doi: 10.1098/rspb.2017.0320
Smith, E. G., Hume, B. C., Delaney, P., Wiedenmann, J., and Burt, J. A. (2017b). Genetic structure of coral-Symbiodinium symbioses on the world's warmest reefs. PLoS ONE 12:e0180169. doi: 10.1371/journal.pone.0180169
Smith, L. W., Barshis, D., and Birkeland, C. (2007). Phenotypic plasticity for skeletal growth, density and calcification of Porites lobata in response to habitat type. Coral Reefs 26, 559–567. doi: 10.1007/s00338-007-0216-z
Smith, L. W., Wirshing, H. H., Baker, A. C., and Birkeland, C. (2008). Environmental versus genetic influences on growth rates of the corals Pocillopora eydouxi and Porites lobata (Anthozoa: Scleractinia). Pac. Sci. 62, 57–69. doi: 10.2984/1534-6188(2008)62[57:EVGIOG]2.0.CO;2
Smith, R., and Iglesias-Prieto, R. (2010). A significant physiological cost to Caribbean corals infected with an opportunistic Symbiodinium. ISRS2010 Reefs in a changing environment. Book Abst. 102.
Smith, T. B., Gyory, J., Brandt, M. E., Miller, W. J., Jossart, J., and Nemeth, R. S. (2016). Caribbean mesophotic coral ecosystems are unlikely climate change refugia. Global Change Biol. 22, 2756–2765. doi: 10.1111/gcb.13175
Sommer, B., Harrison, P. L., Beger, M., and Pandolfi, J. M. (2014). Trait-mediated environmental filtering drives assembly at biogeographic transition zones. Ecology 95, 1000–1009. doi: 10.1890/13-1445.1
Spencer, T., Teleki, K. A., Bradshaw, C., and Spalding, M. D. (2000). Coral bleaching in the southern Seychelles during the 1997–1998 Indian Ocean warm event. Marine Poll. Bull. 40, 569–586. doi: 10.1016/S0025-326X(00)00026-6
Strahl, J., Francis, D. S., Doyle, J., Humphrey, C., and Fabricius, K. E. (2015b). Biochemical responses to ocean acidification contrast between tropical corals with high and low abundances at volcanic carbon dioxide seeps. ICES J. Mar. Sci. 73, 897–909. doi: 10.1093/icesjms/fsv194
Strahl, J., Stolz, I., Uthicke, S., Vogel, N., Noonan, S. H. C., and Fabricius, K. E. (2015a). Physiological and ecological performance differs in four coral taxa at a volcanic carbon dioxide seep. Comp. Biochem. Physiol. A Physiol. 184, 179–186. doi: 10.1016/j.cbpa.2015.02.018
Suggett, D. J., Hall-Spencer, J. M., Rodolfo-Metalpa, R., Boatman, T. G., Payton, R., Tye Pettay, D., et al. (2012a). Sea anemones may thrive in a high CO2 world. Global Change Biol. 18, 3015–3025. doi: 10.1111/j.1365-2486.2012.02767.x
Suggett, D. J., Kikuchi, R. K., Oliveira, M. D., Spanó, S., Carvalho, R., and Smith, D. J. (2012b). Photobiology of corals from Brazil's near-shore marginal reefs of Abrolhos. Mar. Biol. 159, 1461–1473. doi: 10.1007/s00227-012-1925-6
Suggett, D. J., Warner, M. E., and Leggat, W. (2017). Symbiotic dinoflagellate functional diversity mediates coral survival under ecological crisis. Trends Ecol. Evol. 32, 735–745. doi: 10.1016/j.tree.2017.07.013
Thomas, L., Kendrick, G. A., Kennington, W. J., Richards, Z. T., and Stat, M. (2014). Exploring Symbiodinium diversity and host specificity in Acropora corals from geographical extremes of Western Australia with 454 amplicon pyrosequencing. Mol. Ecol. 23, 3113–3126. doi: 10.1111/mec.12801
Thomson, D. P., and Frisch, A. J. (2010). Extraordinarily high coral cover on a nearshore, high-latitude reef in south-west Australia. Coral Reefs 29, 923–927. doi: 10.1007/s00338-010-0650-1
Thomson, D. P., Bearham, D., Graham, F., and Eagle, J. V. (2011). High latitude, deeper water coral bleaching at Rottnest Island, Western Australia. Coral Reefs 30, 1107. doi: 10.1007/s00338-011-0811-x
Ulstrup, K. E., Berkelmans, R., Ralph, P. J., and Van Oppen, M. J. (2006). Variation in bleaching sensitivity of two coral species across a latitudinal gradient on the Great Barrier Reef: the role of zooxanthellae. Mar. Ecol. Prog. Ser. 314, 135–148. doi: 10.3354/meps314135
Unsworth, R. K., Collier, C. J., Henderson, G. M., and McKenzie, L. J. (2012). Tropical seagrass meadows modify seawater carbon chemistry: implications for coral reefs impacted by ocean acidification. Environ. Res. Lett. 7:024026. doi: 10.1088/1748-9326/7/2/024026
van Hooidonk, R., Maynard, J. A., Manzello, D., and Planes, S. (2014). Opposite latitudinal gradients in projected ocean acidification and bleaching impacts on coral reefs. Global Change Biol. 20, 103–112. doi: 10.1111/gcb.12394
van Oppen, M. J., Oliver, J. K., Putnam, H. M., and Gates, R. D. (2015). Building coral reef resilience through assisted evolution. Proc. Nat. Acad. Sci. U.S.A. 112, 2307–2313. doi: 10.1073/pnas.1422301112
van Oppen, M., Bongaerts, P., Underwood, J. N., Peplow, L. M., and Cooper, T. F. (2011). The role of deep reefs in shallow reef recovery: an assessment of vertical connectivity in a brooding coral from west and east Australia. Mol. Ecol. 20, 1647–1660. doi: 10.1111/j.1365-294X.2011.05050.x
van Woesik, R. (1995). Coral communities at high latitude are not pseudopopulations: evidence of spawning at 32°N, Japan. Coral Reefs 14, 119–120 doi: 10.1007/BF00303433
van Woesik, R., and McCaffrey, K. R. (2017). Repeated thermal stress, shading, and directional selection in the Florida Reef Tract. Front. Mar. Sci. 4:182. doi: 10.3389/fmars.2017.00182
van Woesik, R., Houk, P., Isechal, A. L., Idechong, J. W., Victor, S., and Golbuu, Y. (2012). Climate-change refugia in the sheltered bays of Palau: analogs of future reefs. Ecol. Evol. 2, 2474–2484. doi: 10.1002/ece3.363
Venn, A., Tambutte, E., Holcomb, M., Allemand, D., and Tambutte, S. (2011). Live tissue imagining shows reef corals elevate pH under their calcifying tissue relative to seawater. PLoS ONE 6:e20013. doi: 10.1371/journal.pone.0020013
Wall, M., Fietzke, J., Schmidt, G. M., Fink, A., Hofmann, L. C., De Beer, D., et al. (2016). Internal pH regulation facilitates in situ long-term acclimation of massive corals to end-of-century carbon dioxide conditions. Sci. Rep. 6:30688. doi: 10.1038/srep30688
Wicks, L. C., Hill, R., and Davy, S. K. (2010a). The influence of irradiance on tolerance to high and low temperature stress exhibited by Symbiodinium in the coral, Pocillopora damicornis, from the high-latitude reef of Lord Howe Island. Limnol. Oceanogr. 55, 2476–2486. doi: 10.4319/lo.2010.55.6.2476
Wicks, L. C., Sampayo, E., Gardner, J. P. A., and Davy, S. K. (2010b). Local endemicity and high diversity characterise high-latitude coral–Symbiodinium partnerships. Coral Reefs 29, 989–1003. doi: 10.1007/s00338-010-0649-7
Wiedenmann, J., D'Angelo, C., Smith, E. G., Hunt, A. N., Legiret, F. E., Postle, A. D., et al. (2013). Nutrient enrichment can increase the susceptibility of reef corals to bleaching. Nat. Clim. Change 3, 160–164. doi: 10.1038/nclimate1661
Wilson, J. R., and Harrison, P. L. (2003). Spawning patterns of scleractinian corals at the Solitary Islands—a high latitude coral community in eastern Australia. Mar. Ecol. Prog. Ser. 260, 115–123. doi: 10.3354/meps260115
Witman, J. D., and Smith, F. (2003). Rapid community change at a tropical upwelling site in the Galápagos Marine Reserve. Biodivers. Conserv. 12, 25–45. doi: 10.1023/A:1021200831770
Woolsey, E. S., Keith, S. A., Byrne, M., Schmidt-Roach, S., and Baird, A. H. (2015). Latitudinal variation in thermal tolerance thresholds of early life stages of corals. Coral Reefs 34, 471–478. doi: 10.1007/s00338-014-1253-z
Yamamoto, S., Kayanne, H., Terai, M., Watanabe, A., Kato, K., Negishi, A., et al. (2012). Threshold of carbonate saturation state determined by CO2 control experiment. Biogeoscience 9, 1441. doi: 10.5194/bg-9-1441-2012
Yamano, H., Sugihara, K., and Nomura, K. (2011). Rapid poleward range expansion of tropical reef corals in response to rising sea surface temperatures. Geophys. Res. Lett. 38, 1441–1450. doi: 10.1029/2010GL046474
Yates, K. K., Dufore, C., Smiley, N., Jackson, C., and Halley, R. B. (2007). Diurnal variation of oxygen and carbonate system parameters in Tampa Bay and Florida Bay. Mar. Chem. 104, 110–124. doi: 10.1016/j.marchem.2006.12.008
Yates, K. K., Rogers, C. S., Herlan, J. J., Brooks, G. R., Smiley, N. A., and Larson, R. A. (2014). Diverse coral communities in mangrove habitats suggest a novel refuge from climate change. Biogeoscience 11, 4321–4337. doi: 10.5194/bg-11-4321-2014
Yentsch, C. S., Yentsch, C. M., Cullen, J. J., Lapointe, B., Phinney, D. A., and Yentsch, S. W. (2002). Sunlight and water transparency: cornerstones in coral research. J. Exp. Mar. Biol. Ecol. 268, 171–183. doi: 10.1016/S0022-0981(01)00379-3
Ziegler, M., Seneca, F. O., Yum, L. K., Palumbi, S. R., and Voolstra, C. R. (2017). Bacterial community dynamics are linked to patterns of coral heat tolerance. Nat. Commun. 8:14213. doi: 10.1038/ncomms14213
Keywords: extreme, ocean acidification, marginal, refuge, review
Citation: Camp EF, Schoepf V, Mumby PJ, Hardtke LA, Rodolfo-Metalpa R, Smith DJ and Suggett DJ (2018) The Future of Coral Reefs Subject to Rapid Climate Change: Lessons from Natural Extreme Environments. Front. Mar. Sci. 5:4. doi: 10.3389/fmars.2018.00004
Received: 30 August 2017; Accepted: 10 January 2018;
Published: 02 February 2018.
Edited by:
Hajime Kayanne, The University of Tokyo, JapanReviewed by:
Simon K. Davy, Victoria University of Wellington, New ZealandDouglas Fenner, NOAA NMFS Contractor, United States
Copyright © 2018 Camp, Schoepf, Mumby, Hardtke, Rodolfo-Metalpa, Smith and Suggett. This is an open-access article distributed under the terms of the Creative Commons Attribution License (CC BY). The use, distribution or reproduction in other forums is permitted, provided the original author(s) and the copyright owner are credited and that the original publication in this journal is cited, in accordance with accepted academic practice. No use, distribution or reproduction is permitted which does not comply with these terms.
*Correspondence: Emma F. Camp, ZW1tYS5jYW1wQHV0cy5lZHUuYXU=