- 1College of Earth, Ocean, and Atmospheric Sciences (CEOAS), Oregon State University, Corvallis, OR, United States
- 2Department of Earth and Planetary Sciences, Macquarie University, Sydney, NSW, Australia
- 3Bigelow Laboratory for Ocean Sciences, East Boothbay, ME, United States
Neodymium (Nd) isotopes are considered a valuable tracer of modern and past ocean circulation. However, the promise of Nd isotope as a water mass tracer is hindered because there is not an entirely self-consistent model of the marine geochemical cycle of rare earth elements (REEs, of which Nd is one). That is, the prevailing mechanisms to describe the distributions of elemental and isotopic Nd are not completely reconciled. Here, we use published [Nd] and Nd isotope data to examine the prevailing model assumptions, and further compare these data to emergent alternative models that emphasize benthic processes in controlling the cycle of marine REEs and Nd isotopes. Our conclusion is that changing from a “top-down” driven model for REE cycling to one of a “bottom-up” benthic source model can provide consistent interpretations of these data for both elemental and isotopic Nd distributions. We discuss the implications such a benthic flux model carries for interpretation of Nd isotope data as a tracer for understanding modern and past changes in ocean circulation.
Introduction
Neodymium (Nd) isotopes (denoted εNd, which reflects the 143Nd/144Nd ratio normalized to a Chondritic Uniform Reservoir; Jacobsen and Wasserburg, 1980) are widely recognized as a valuable tracer for ocean circulation (e.g., Piepgras and Wasserburg, 1980, 1987; Elderfield, 1988; von Blanckenburg, 1999; Frank, 2002; Goldstein and Hemming, 2003; Piotrowski et al., 2004; van de Flierdt and Frank, 2010). The potential for Nd isotopes to serve as a circulation tracer lies with the observation that εNd distributions appear to mirror the pattern of global deep-water thermohaline circulation. These observations support the assumption that εNd is conservative or “quasi-conservative” in the oceans and will trace water masses in a manner similar to temperature and salinity (Frank, 2002; Goldstein and Hemming, 2003). Furthermore, elemental Nd is assumed to have negligible bioactivity, although recent work suggests that there are important caveats to this assumption (Shiller et al., 2017). However, because of the concerted efforts invested into measuring neodymium in the oceans as well as within sedimentary archives, inconsistencies between the observed distributions and the assumptions inherent in the use of the Nd as a tracer are becoming increasingly apparent. The best known of these inconsistencies is the “Nd paradox” (Jeandel et al., 1995, 1998; Tachikawa et al., 1999a; Lacan and Jeandel, 2001). Described in many ways, this paradox fundamentally describes the contradictory observations that while εNd appears to behave conservatively, the water column profile of dissolved elemental Nd ([Nd]) appears to reflect the behavior of a reactive element, showing a nutrient-like distribution (see discussion in Goldstein and Hemming, 2003). In short, εNd distributions imply a residence time of ≤103 years, while [Nd] distributions imply a residence time of ≥104 years (Bertram and Elderfield, 1993; Jones et al., 1994). This discrepancy appears in paired geochemical mixing models (Goldstein and Hemming, 2003) and models that attempt to reproduce the global distribution of εNd (Tachikawa et al., 2003; Jones et al., 2008; Arsouze et al., 2009; Rempfer et al., 2011). In addition to the “Nd paradox,” inconsistencies have arisen regarding past records of authigenic εNd, which sometimes prove to be difficult to explain when assuming water mass mixing of fixed end-members (Osborne et al., 2014; Stewart et al., 2016), or show exceptional disparities between adjacent sites (Stumpf et al., 2010; Roberts and Piotrowski, 2015; Howe et al., 2016; Hu et al., 2016).
The problem with εNd as a circulation proxy is fundamentally that while εNd appears to trace water masses effectively, it is not particularly potent at discerning geochemical processes that might impact its distribution. For example, it is difficult to use εNd to determine how a water mass acquires its isotope signature. Although the pattern of global εNd mirrors that of thermohaline circulation the cause of that apparent coincidence is not straightforward. For example, that the deep North Pacific is a Nd isotope end-member for mixing is difficult to justify given that no deep water forms within this basin. If isotopic end members can develop unassociated with preformed properties of the water mass, then there is clearly a need to explain and constrain the effects of these processes on εNd, especially for interpretation of εNd as ancient records of circulation.
Relatively recently, several possible mechanisms for non-conservative behavior of εNd have been forwarded: boundary exchange, Submarine Groundwater Discharge (SGD) and a benthic Nd flux (Lacan and Jeandel, 2005b; Johannesson and Burdige, 2007; Abbott et al., 2015a). These three hypotheses are all similar in that bottom water can be altered along the sediment-water boundary. Although these hypotheses may have been articulated as distinctly different, the differences among these hypotheses may be simply semantic or reflect our incomplete understanding of the processes involved. Boundary exchange, as described by Lacan and Jeandel (2005b) is typically thought to reflect an isotope exchange of Nd with net zero concentration change that occurs between the sediments and bottom water predominantly at ocean margins (Jeandel, 2016). While this model is highly cited, the specific mechanism or processes are not well defined. In contrast, both the SGD and benthic flux models propose more distinct mechanisms but lack the robust global data sets needed for verification. All three of these hypotheses invoke a potential source of dissolved [Nd] to the oceans from sedimentary fluids; SGD waters are considered here to be originally fresh waters, while the benthic flux model considers seawater as the source fluid origin. These latter hypotheses will differ in the quantity and location of the Nd fluxes they predict and the εNd that they carry. Clearly, the distinctions among all three models is nuanced, and again, probably simply reflects the need for further investigation or even simple definition. However, all three models challenge the underlying assumptions around the conservative nature of εNd in the water column.
A more comprehensive understanding of the marine geochemical cycle of REEs in conjunction with εNd is needed to build more accurate geochemical models and interpret down core records with respect to ocean circulation. The problems arising from the notion of εNd as a paleoproxy perhaps reflect the superficial, and perhaps data limited synthesis between analyses of εNd and the rare earth elements (REEs) throughout the ocean basins. Fortunately, the comprehensive nature of the GEOTRACES water column sampling will go far to improve our understanding of water column processes that affect the REEs and εNd. Here, we discuss published εNd and Nd data from the Atlantic Ocean, with the intent to clarify our understanding of these tracers where they appear to be most robust. While we do not present any new data, we attempt to illustrate that a holistic view of published REE and εNd distributions in the oceans is consistent with predominant control from a benthic sedimentary source. Our conclusion is that processes occurring within the water column (e.g., reversible scavenging) are likely a secondary control on εNd or [REE] in the oceans, save where particle fluxes are exceptionally high, and that benthic fluxes exert primary control over the distribution of these elements and their isotopes.
Modern Ocean REEs and Nd Isotopes
It was originally held that the REEs are input to the oceans predominantly via rivers, with potential additions via dust where such inputs are relatively high (Byrne and Sholkovitz, 1996; Greaves et al., 1999; Tachikawa et al., 1999b; Goswami et al., 2014; Dunlea et al., 2015; Stichel et al., 2015; also see Bayon et al., 2004 for the alternative view that dust is a sink term). More recently, the emphasis of REE input to the oceans has shifted to greater consideration of a benthic continental margin source for REEs, as we will discuss further later (Spivack and Wasserburg, 1988; Jeandel et al., 1998; Goldstein and Hemming, 2003; Tachikawa et al., 2003; Arsouze et al., 2009; Rickli et al., 2009, 2010, 2014; Carter et al., 2012; Grasse et al., 2012; Singh et al., 2012; Grenier et al., 2013; Wilson et al., 2013; Garcia-Solsona et al., 2014).
In the open ocean, REEs are thought to be transferred from shallow to deep ocean via reversible scavenging on particles sinking through the water column (Elderfield, 1988; Byrne and Kim, 1990; Sholkovitz et al., 1994; Byrne and Sholkovitz, 1996). However, the heavier atomic mass REEs (HREEs, such as Yb, Lu) tend to have vertical profiles that are more “Si-like,” which is thought to reflect the tendency for greater complexation across the REE series (Cantrell and Byrne, 1987; Elderfield, 1988; Byrne and Sholkovitz, 1996). Finally, removal of REEs from the ocean is thought to be predominantly via metal-oxides, although the role of organic matter, phosphates, or even carbonates, is likely to be important (Byrne and Kim, 1990,?; Byrne and Sholkovitz, 1996; Schijf et al., 2015). This model is considered the standard of marine REEs. Nd isotopes should also behave in a manner that is consistent with this model, although as pointed out previously this does not appear to be the case (Goldstein and Hemming, 2003; Jones et al., 2008; Arsouze et al., 2009). Successful efforts to reconcile the inconsistent behavior between elemental Nd and its isotopes, i.e., the Nd paradox, will likely begin with an examination of these first principles of marine REE cycling.
The shift from considering riverine point sources to broad marginal inputs improves model results (Tachikawa et al., 2003; Arsouze et al., 2009) and offers an explanation for some of the irregularities observed when comparing Nd and its isotopes such as the constancy of surface ocean water εNd (−7.8 upstream vs. −7.9/−8.0 downstream; Osborne et al., 2014) around the mouth of the Mississippi river that carries a far less radiogenic signal (at εNd < −11; Goldstein and Jacobsen, 1987, 1988; Bayon et al., 2015. Budgetary estimates from diffuse benthic REE fluxes, such as SGD or from sedimentary pore water (Sholkovitz et al., 1989; Greaves et al., 1999; Haley and Klinkhammer, 2003; Tachikawa et al., 2003; Johannesson and Burdige, 2007; Schacht et al., 2010; Johannesson et al., 2011, 2017; Abbott et al., 2015a; Fröllje et al., 2016), suggest that they may dominate the flux of REEs to the oceans. Importantly, such diffuse, or at least hard to quantify, sources are consistent with deep-water isopycnal mixing of εNd signals off margins that cannot be explained through surface water sources (Grasse et al., 2012; Grenier et al., 2013). However, while a diffuse benthic source of Nd appears to better describe the dominant flux of REEs to the ocean, outstanding questions remain. Arguably the most important of these is: what drives this flux? Any mechanism must be reconciled with the remarkably consistent REE pattern of seawater in light of diverse sediment compositions and sedimentary/diagenetic environments. As such, it seems unlikely that simple, unidirectional particulate dissolution can be the mechanism, because the dissolved REE pattern and εNd would to some extent reflect such an input, following arguments posed by Sholkovitz (1993) and reiterated by Bau et al. (2013).
Another supposition in the current understanding of marine REE cycling is that reversible scavenging controls the vertical distribution of these elements in the water column. In his deeply insightful paper, Elderfield (1988) suggested cause for concern over this conjecture: not for shallow REE adsorption, but in light of a mechanism for desoprtion at depth. For many trivalent cations the pH-edge/Langmuir-front for adsorption onto particle surfaces lies well under a pH of 7 (Morel and Hering, 1993; Ngwenya et al., 2010); thus, over a typical oceanic pH range of 7.6 and 8.2 desorption is unlikely. The thermodynamics of desorption become even less favorable as the increasing dissolved concentration at depth would tend to push the equilibrium toward further adsorption (reiterating arguments posed by Elderfield, 1988). Moreover, given free Nd is <16% of the total dissolved Nd (Millero, 1992; Schijf et al., 2015), and surface adsorption coefficients are typically not exceptionally high (in the range of 3 to 5 onto carboxyl and moncarboxylic groups; Smith and Martell, 1989; Byrne and Kim, 1990; Ngwenya et al., 2010), the potential for REE transfer from the shallow to deep ocean via particle surface scavenging should be limited (Elderfield and Greaves, 1982; Stichel et al., 2015).
In spite of such arguments, lab and field observations attest that the REEs are indeed highly susceptible to adsorption (Sholkovitz, 1989), and that adsorption onto particles in the ocean certainly does happen (e.g., Sholkovitz et al., 1994; Tachikawa et al., 1999a). One positive test for REE adsorption in the oceans may be seen in conservative Arctic Ocean REE profiles where particles are near absent (Yang and Haley, 2016). We do note, however, that a counter-argument can be made in that there does not appear to be an appreciable difference in the [Nd] profiles relating to marginal sites vs. distal sites, where particle loading may be very different (German and Elderfield, 1990; Grasse et al., 2012; Goswami et al., 2014; Haley et al., 2014; Abbott et al., 2015b; Stichel et al., 2015).
Considering all the arguments and data, a preferred model is that REEs are most likely transferred from the ocean surface to depth through incorporation onto complex organics, such as humic acids, polysaccharides, or other complex organic molecules that have binding coefficients higher than simple carboxyl groups (logK > 9; Byrne and Kim, 1990; Stanley and Byrne, 1990; Davranche et al., 2005; Pourret et al., 2007; Schijf et al., 2015). In this way, scavenging is actually achieved via an organic coating carrier phase that is remineralized, thus offering a mechanism for transfer of REEs from the particulate to dissolved phase without subsequent re-adsorption (i.e., not a desorptive process, but a remineralization; Sholkovitz et al., 1994). This model implies that [Nd] should behave much like δ13C in the water column, wherein their distributions reflect a preformed signal modified by top-down uni-directional input additions related to POC remineralization. Available GEOTRACES data allow us to make an initial comparison of water column [Nd] and δ13C (Mawji et al., 2015; Stichel et al., 2015; Figure 1). We consider the comparison inconclusive; these data appear to share many similarities (e.g., at Stations 12, 24, 10, especially in the upper 1,000 m) but not everywhere (e.g., at Stations 1, 3, and 7). Comparison of these [Nd] and δ13C data yields no correlation (r2 < 0.05; Figure S1), although the fit of all the data from the upper 50 to 500 m, ostensibly under highest remineralization, is better (r2 ~0.4; Figure S2), which would support scavenging of Nd on isotopically light-C POM. The form of the [Nd] profiles in Figure 1, especially in the upper 1,000 m, does appear to share many of the characteristics of POM remineralization in the oceans (e.g., Suess, 1980; Martin et al., 1987) and of AOU (discussed by Stichel et al., 2015). Unfortunately, global compilations, such as presented by Tachikawa et al. (2017), produce poor correlations between δ13C and [Nd] (r2 < 0.001; not shown), and the similarity between deep (>1,000 m) [Nd] and P* (van de Flierdt et al., 2016) becomes difficult to justify under the hypothesis of POM scavenging of Nd.
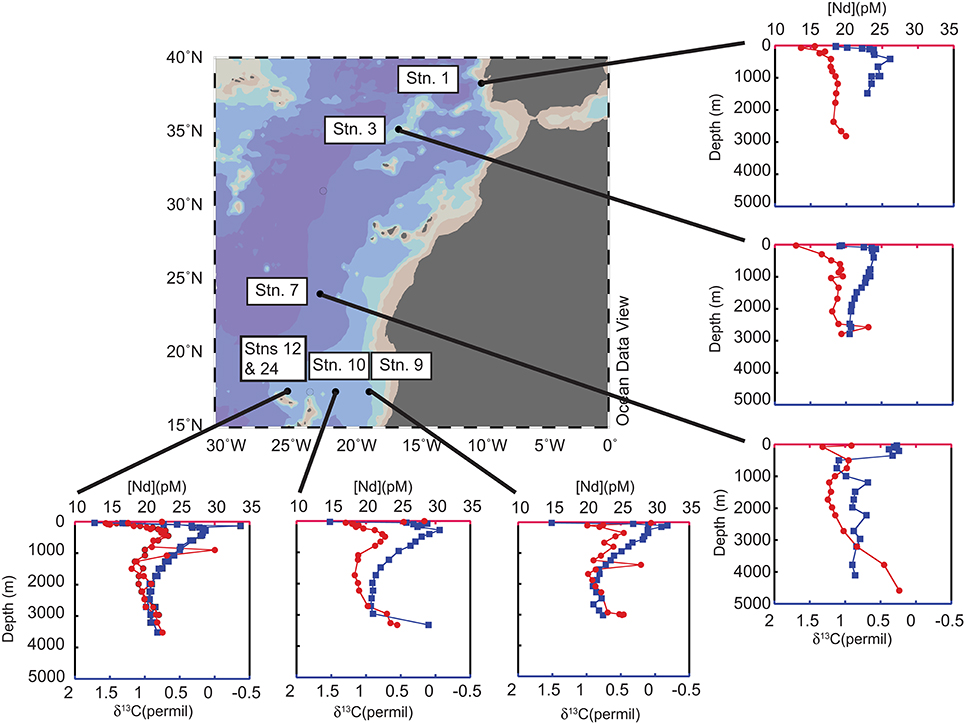
Figure 1. GEOTRACES [Nd] and δ13C data from sites in the eastern North Atlantic. Only data where these parameters were available to the sea floor are shown. Data from Mawji et al. (2015) and Stichel et al. (2015).
A similar uni-directional release of REEs at depth can also potentially come from deterioration of the lithogenic minerals themselves (Rousseau et al., 2015; Abadie et al., 2017). This mechanism would differ markedly in its effect on water column [REE] and εNd in comparison to a model based on an organic surface coating. Unfortunately, the evaluation of [Nd] and εNd in light of a lithogenic source of Nd at depth has been ambiguous in the eastern North Atlantic (Stichel et al., 2015; van de Flierdt et al., 2016).
Evaluation of modes of scavenging may be complicated through multiple substrates for scavenging, each of which can deliver REEs to depth (e.g., complex organic molecules as well as lithogenic and biogenic silicates; Akagi et al., 2011; Akagi, 2014). Adding additional ambiguity to the assessment of reverse scavenging is the issue of preformed REEs at depth that are likely conservative in nature (Haley et al., 2014; Zheng et al., 2016). Such preformed REEs must be an important component of deep water REEs (at least on basinal scales), such that REE profiles cannot be taken at face value: de-convolution of the conservative and non-conservative REEs is needed for accurate interpretation (Bertram and Elderfield, 1993; Haley et al., 2014; Zheng et al., 2016). Such efforts, either using a water mass approach (e.g., Bertram and Elderfield, 1993; Grasse et al., 2012; Stichel et al., 2012, 2015 or a more statistical approach (Singh et al., 2012; Haley et al., 2014; Zheng et al., 2016), are critical in evaluating the importance of scavenging on water column REEs. It goes beyond the scope of this paper to explore solutions for resolving preformed vs. “reactive” REEs. Our goal here is simply to suggest that much of the difficulty in resolving the “Nd paradox” is caused by undue emphasis placed on the function of reverse scavenging, which likely plays only a minor role in controlling the distribution of REEs and εNd in the water column.
Our exemplar here is the outstanding work of Bertram and Elderfield (1993). These authors carefully evaluated the possible influence of scavenging and reverse scavenging, largely under an assumption of “top down” forcing, because of the similarities to Si and a lack of sedimentary data (Bertram and Elderfield, 1993). Their conclusion is that particle-water exchange is necessary to explain deep ocean εNd, and give two possible mechanisms: a “top down” possibility wherein scavenged Nd exchanges over time in the deep ocean and a “…second option [that] simply places the site of exchange close to or within the sediment-porewater system with exchange within the nepheloid zone or transport via diffusion into overlying seawater” (Bertram and Elderfield, 1993). [Interestingly, the authors also note that “the simple (water density) & Si-REE correlations seen in bottom and near-bottom waters may also imply involvement of a benthic source. Unfortunately, no satisfactory data are available on REEs in oxic porewaters.”].
Finally, regardless of the impact on the water column, we emphasize that all of the potential “top down” mechanisms for remobilizing surface REEs at depth would be greatly enhanced within seafloor sediments (Freslon et al., 2014); i.e., manifest as a pore water flux. In summary, while scavenging certainly happens on particles, we argue that the REE mass transfer is likely relatively small and subsequent desorption at depth in the water column is unlikely. On the other hand, any such desorption or remineralization mechanism is likely to be significant in the sediments, where the chemical environment can change significantly.
Benthic Control
Regardless of the discussions presented above, mechanisms driven via a “top-down” flux of REEs return to the disparity between εNd and [Nd] as discussed by Goldstein and Hemming (2003). The question is fundamentally how can such processes occur that account for [Nd] distributions, yet does not obviate the conservative qualities of εNd? That a water mass such as North Atlantic Deep Water (NADW) can maintain its signature on ocean-basin scales is arguably less justifiable in a “top-down” explanation of marine REEs than it is in the “bottom-up” explanation that will be discussed below.
If we consider our prior argument that reversible scavenging does not significantly impact Nd's water column distribution, what then can explain the distribution of [Nd]/εNd at depth? A “bottom up” hypothesis argues that Nd and εNd are truly conservative below the permanent thermocline, except when exposed to a benthic flux. This benthic flux will certainly impact overlying bottom water, but its influence may also be carried laterally along isopycnals, for example off the continental slope (as indicated by Grasse et al., 2012; Singh et al., 2012; Stichel et al., 2015). The interaction with the sediments is then what makes εNd “quasi-conservative,” vs. being truly conservative, as has been suggested previously (Lacan and Jeandel, 2004b; Abbott et al., 2015a). The two important aspects of this suggestion are that (1) in the absence of a positive benthic flux, Nd and εNd will behave conservatively and (2) the ocean biogeochemistry of Nd and εNd is thus defined by benthic diagenetic processes. That is, this benthic control model suggests that the REEs and εNd are controlled by the nature of the benthic flux, which can be a positive, negative, or neutral term.
The idea of a benthic source for REEs, as described previously (Abbott et al., 2015a), would certainly help resolve model and observational inconsistencies of REE in the Pacific Ocean (Jones et al., 2008; Arsouze et al., 2009), but is such a source necessary anywhere else-in the Atlantic, Indian, Arctic or Southern Oceans? The North Atlantic, for example, has been well described in “top-down” models (Jones et al., 2008), or even through simple conservative mixing (van de Flierdt et al., 2016). Although we note here that the Denmark Straits is the ‘birthplace’ of the very non-conservative boundary exchange hypothesis (Lacan and Jeandel, 2004a,b, 2005a) and that other models have required benthic source fluxes in the North Atlantic (Tachikawa et al., 2003).
Why then invoke a benthic model? First, if correct, the benthic model would provide a mechanism to reconcile the apparent conflicts in our interpretation of Nd and εNd; and second, the benthic model makes different predictions about how changes in εNd and REEs records can be interpreted, when compared to a reversible scavenging model, as will be discussed later.
The concept of benthic control of [REE]/εNd on Pacific waters is still a nascent idea (Abbott et al., 2015a, 2016; Du et al., 2016). Here, we expand on this idea in a general sense. First, benthic control of [REE]/εNd will directly influence only the bottom water, such that a water mass detached from the bottom will maintain its εNd so long as it maintains its temperature and salinity (i.e., all its conservative properties). Second, benthic control represents a diffuse boundary flux, which implies that deep water [REE]/εNd distributions may be more disposed to being controlled by eddy diffusivity. Because eddy diffusion coefficients in the vertical are 10−5-10−4 m2/s (or greater; Ku and Luo, 1994), over hundreds to thousands of meters per year (horizontal coefficients are even greater), the impact of a benthic flux may potentially integrate over significant depths within the deep ocean. Furthermore, eddy diffusivity is also an order of magnitude higher on the seafloor relative to the water column because of the turbulence created by topography (Waterhouse et al., 2014); therefore, a benthic flux will be dissipated with great efficiency into the water column. This dissipation is the rationale for the observation that there is an apparent jump in REE concentrations between pore water and overlying water (Abbott et al., 2015b, 2016).
Implications of a Benthic Flux in the Atlantic
There is little direct observational data regarding the benthic REE flux to the oceans (Sholkovitz et al., 1989; Haley et al., 2004; Abbott et al., 2015b), and even less that tracks the εNd of this flux. However, comparison with radium offers some support for the hypothesis. Again, the holistic nature of GEOTRACES sampling has provided rare, directly comparable Ra-REE-εNd data (Charette et al., 2015; Stichel et al., 2015; USGT10/GA03 section from the Gulf of Cadiz to the Mauritanian coast). All isotopes of Ra positively correlate with [Nd] in the bottom water, although we plot only 228Ra, as this isotope is most directly associated with a benthic source influence (Figure 2A). The correlation of 228Ra with [Nd] in bottom water is strong (r2 = 0.7 including all the data, and by omitting Site 7, r2 = 0.9). Such a positive correlation is consistent with both 228Ra and [Nd] sharing a common benthic source. In contrast, benthic 228Th does not covary with [Nd], which might otherwise be expected if the source of deep Nd was delivered via top-down particle-based mechanisms.
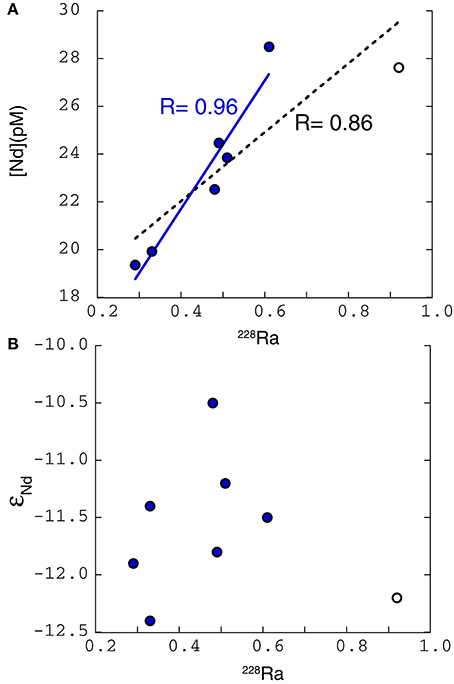
Figure 2. Comparison of bottom water radium and neodymium (data from Charette et al., 2015; Stichel et al., 2015). (A) shows the positive correlation of the benthic-derived Ra with [Nd], consistent with a benthic source for both. (B) shows little correlation of εNd with Ra, although a correlation may not be expected between these variables. The unfilled datum is arbitrarily isolated: there is no reason to consider this datum contaminated.
Bottom water εNd does not covary with Ra isotopes (Figure 2B). While such a correlation is not obligatory under the benthic control model, work with Pacific sediments suggests that certain components of the sediments may be more reactive during diagenesis, and such reactivity is also likely element specific (Abbott et al., 2016; Du et al., 2016). Thus, we might expect that reaction of these sedimentary elements imposes a co-variation between the magnitude and isotopic composition of the flux.
We reiterate that there is undeniable evidence in support of the idea that seawater εNd traces basin scale modern water mass circulation, and the benthic control hypothesis suggested here does not detract or contradict these observations. On the contrary, we suggest that records of εNd may potentially be used to actually quantify current velocities, given that the “exposure time” of a water mass to the bottom (Abbott et al., 2015a) implies a temporal sensitivity necessary to establish velocity. As an example, the bottom water data of Lambelet et al. (2016) for the North Atlantic, can be plotted vs. latitude (Figure 3). A benthic control based interpretation of these data may be that there are three diagenetic regimes in the North Atlantic: north of 55°N, between 45 and 55°N and south of 40°N. We will first focus on the increasing [Nd] and decreasing εNd of the middle regime (~43°-55°N). In this region, there is a total change in εNd of ~2 units, of which conservative mixing with LSW can account for ~1 unit (Figure 4; using water properties defined in van de Flierdt et al. (2016). Applying the simple exposure time model of Abbott et al., 2015a; assuming flux of 20 pmol/cm2/yr with εNd of −15, which is reasonable but arbitrarily chosen, and a deep water [Nd] of 15 pM, which is measured), we might estimate that the residual 1-unit corresponds to ~35 years of benthic flux exposure on a 1 km thick bottom water layer, occurring over ~12° latitude. This combination results in a bottom water current velocity of 0.2 cm/s. This estimate of effective transport rate, despite its obvious simplifications and assumptions, is not incomparable to the 1.8–2 cm/s estimates derived from CFC and tritum/He3 (Smethie et al., 2000; Steinfeldt and Rhein, 2004). This simple model scenario also predicts [Nd] of the water mass increases to 23 pM, similar to that observed (Figure 3). Other scenarios are obviously possible; for example, a 5 pmol/cm2/yr flux with εNd of −20 offers a similar 40 year exposure estimate, but predicts a final [Nd] of only 17 pM. The only existing pore waters from the Atlantic (Buzzard's Bay; Sholkovitz et al., 1989) show pore water [Nd] of >700 pM at ~13 cm depth in the sediment, which compares to fluxes as large as any seen in the Pacific (up to 30 pmol/cm2/yr; Haley and Klinkhammer, 2003; Abbott et al., 2015b). Such a flux of 30 pmol/cm2/yr with an εNd of −25, as implied might be the case in this region (Lambelet et al., 2016), predicts only 4 years of exposure for ~1 εNd unit change, which equates to 1.1 cm/s velocity, but an increase of [Nd] to just 16 pM. Again, these calculations are obviously crude, and poorly constrained; we use them simply to illustrate sensitivity of deep water to benthic fluxes, and the feasibility of using the concept for quantitative reconstruction of circulation.
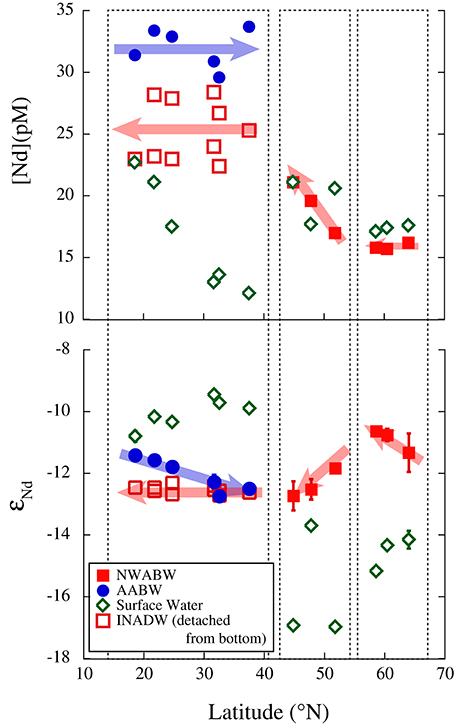
Figure 3. Bottom water [Nd] and εNd from Lambelet et al. (2016). Arrows show interpretation of flow paths of bottom water.
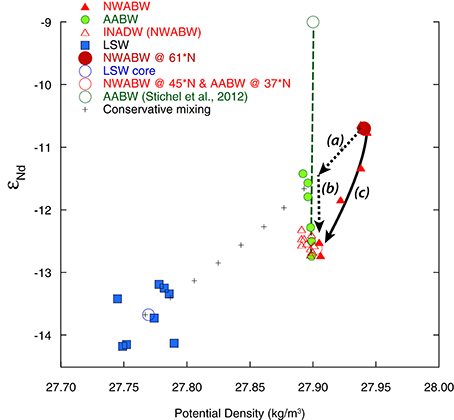
Figure 4. Mixing properties of density and εNd. Conservative mixing of εNd between NWABW and LSW can explain 1-unit of εNd [dashed arrow (a)], leaving 1-unit unexplained [dashed arrow (b)]. Compare to non-conservative alteration via benthic fluxes [solid arrow (c); data from Lambelet et al., 2016]. Note the detached INADW maintains its εNd signature as it does potential density. The highly non-conservative nature of εNd for AABW is illustrated (green dashed arrow; see text for details).
The Lambelet et al. (2016) data show constancy of εNd in the Northwest Atlantic Bottom Water (NWABW) after it detaches (or is displaced) from contact with the bottom south of ~40°N. At these lower latitudes, Antarctic Bottom Water (AABW) is in contact with the sediments, and it is this southern-sourced water mass that appears to be modified during its transit northwards. While such a pattern can be explained with a benthic control model for [REE]/εNd, a “top-down” process that clearly alters bottom water but not the deep water overlying bottom water seems difficult to reconcile. Moreover, the potential density of AABW is remarkably stable along its flow path: σ = 27.9 kg/m3 at ~60°S (Stichel et al., 2012) vs. σ = 27.903 kg/m3 at ~37°N (from Lambelet et al., 2016 data; Figure 4). This constancy indicates that little water mass mixing occurs during this bottom water transit, yet εNd changes significantly: at least one unit in just the northern hemisphere data of Lambelet et al. (2016). Again, if we argue that conservation of NADW εNd precludes a top down driver for AABW εNd change, the logical conclusion is that benthic alteration impacts bottom water (i.e., AABW) εNd. Through the same approximations done above (assuming an arbitrarily chosen 5 pmol/cm2/yr flux with εNd of −15 and measured 30 pM [Nd] of bottom water), we might estimate that the 1-unit ΔεNd of AABW observed corresponds to ~150 years of exposure. Over 25° of latitude, this corresponds to a current velocity of 0.06 cm/s, implying that AABW has about one third the velocity as NWABW. (Note that because this calculation is based on a concentration gradient, the exact positions of where the slope is calculated can differ, making such estimations amenable to paleo-reconstructions where end-members may not be well constrained.) This effective transport rate estimate is, as was the case for our NADW estimate, lower than the CFC estimate of ~1.2 cm/s for AABW (Haine et al., 1998). We can predict that the benthic fluxes affecting AABW must be more complex, because linear extrapolation of the rate of change in εNd over latitude as shown in Figures 3, 4 would imply that AABW has an εNd of −6 at 60°S, which it does not (Stichel et al., 2012). The likely rationale for this difference is that the diagenetic fluxes along the greater AABW flow path are more nuanced in both their magnitude and composition than can be expressed by our simplified approximations.
There are three further points relevant to this discussion. First, the benthic control hypothesis does not invoke water mass mixing to explain patterns in εNd such as those shown in Figures 3, 4) That is, there is no obligate change in conservative properties, such as temperature or salinity, as the bottom water εNd is modified. Again, mid-depth water masses detached from the sediment will likely maintain their εNd signatures, or mix them as they would other conservative tracer properties along isopycnals, for example.
Second, the North Atlantic appears to have a propensity to mask the impacts of a benthic flux because ventilation rates are high, and thus exposure times are low. Instances where benthic influences may be directly observed in the North Atlantic (e.g., Lacan and Jeandel, 2004c) would thus reflect rather extreme cases of high flux or divergent isotopic composition. In contrast, AABW in the South Atlantic (and PDW in the Pacific) circulate rather more slowly and thus would be prone to longer benthic exposure, resulting in more obvious impacts of a benthic source flux.
Third, the benthic flux mechanism seems to be intimately connected to authigenic metal oxides in the sediments (Abbott et al., 2016), which appear to modulate the REE flux and its εNd signature. Authigenic metal oxides appear to act as a “capacitor” of REE: i.e., both a source and sink of pore water and bottom water REE. Analogous to electrical capacitance, more abundant sedimentary metal oxides will produce greater Nd fluxes with less temporally variable εNd signatures (Abbott et al., 2016). While this mechanism is clearly far from resolved, it offers an explanation for the apparent lack of influence from the non-radiogenic lithogenic sediments of the central North Pacific (Jones et al., 1994). Specifically, we predict that there is no positive benthic flux from these sediments, or that reactive diagenetic materials are not present in these sediments (Abbott et al., 2016; Du et al., 2016), or both. We also note that the non-radiogenic Nd data presented by Jones et al. (1994) reflect the post-leach lithogenic fraction of the sediments, which may not play a role at all in defining the benthic flux as defined by our proposed mechanism.
Paleoproxy Nd
Arguably the most important implication of benthic control on marine [REE]/εNd is how we interpret paleoceanographic records of water mass change. For these studies, the difference between a “top-down” or “bottom-up” control on bottom water and on the marine budget of REEs becomes paramount for two reasons:
Firstly, most interpretations of paleo-data treat εNd as fundamentally conservative, discounting the possibility of changes in REE supply that is not the result of water mass mixing or circulation. In a reversible scavenging model, this may be a valid assumption if deep-water sensitivity to surface ocean change is small. However, this supposition then begs the question of why reversible scavenging controls the modern deep [REE]/εNd? On the other hand, in a benthic control scenario the εNd of bottom water may evolve through non-mixing processes: such as a change in the benthic flux or a change in the “exposure time” of the bottom water to this flux (Abbott et al., 2015b). As such, an εNd record interpreted through a benthic control model may reflect a change in benthic flux or exposure time, the latter of which may well be the result of changing circulation patterns or velocities. Despite the ostensible complications, we argue that interpreting εNd records purely as a conservative tracer discounts the “pseudo-conservative” nature of the tracer. Our benthic control model offers an internal cycling constraint on [Nd] and εNd compared to a reverse scavenging model. Both models require the ability to reconstruct fluxes through time to accurately use εNd as a tracer: the reversible scavenging model requires estimation of vertical particle fluxes and particulate type over time, whereas the benthic control model requires estimation of the benthic flux over time. Determining either of these fluxes will be non-trivial tasks, but may be resolvable.
Secondly, similarity between surface sediment archival phases (dispersed oxides, coatings, fish teeth, forams) and bottom water is often cited as evidence for record fidelity under the top down model for εNd. In the benthic control model, such correspondence will result from bottom water that is either dominated by a strong positive benthic flux, wherein the preformed bottom water εNd signature is overwhelmed by a pore water signature supported by the authigenic phases, or from a negative or net-zero pore water flux, such that pore water and authigenic phases passively record bottom water signatures. There is also a range of intermediate conditions where εNd of the archival phase may differ from bottom water, and where we would predict there is a significant, but not overwhelming, positive benthic flux into bottom water (e.g., Du et al., 2016 in the Pacific, or Gutjahr et al., 2008; Huck et al., 2016 in the Atlantic). The relationship between authigenic phases (used as archival records) and contemporaneous bottom water is far more complex in the benthic control model, because the authigenic phases associated with diagenesis are intimately associated with the nature of the benthic flux that impact the bottom water signal (Abbott et al., 2016). Moreover, the concentrations of sedimentary reactive particulate phases, pore water, and bottom water differ by orders of magnitude, so we stress the importance of looking at both εNd and the [REEs].
Our benthic model may give an initially grim outlook for paleoproxy work, but the situation is probably not at all intractable: in fact, these challenges are likely to be fully resolvable with further observations and models. Most pressing is the need to better determine the mechanisms and sources that define the Nd sink terms within the sediment column: these processes are ultimately what will define the proxy records. For example, Abbott et al. (2015b) did not discuss the deep (~10 cm) sink term seen in pore water [Nd] profiles; if this sink records contemporaneous pore water εNd, an apparent temporal offset could be generated in records of εNd from diagenetic Fe-Mn oxides and other isotope and trace element proxies archived in the calcite lattice of foraminifera (Piotrowski et al., 2005).
Theory and observations all indicate that some aspect of bottom water εNd is maintained in authigenic phases, and is likely identifiable in all sediment (Gutjahr et al., 2007; Wilson et al., 2013; Blaser et al., 2016; Du et al., 2016). Furthermore, the additional Nd generated through diagenesis should be far less variable and readily constrained as “baseline” variations in sedimentary records, vs. higher-order changes that are derived from seawater; analogous to Sr isotope records. A compilation of global surface sediment authigenic-phase εNd vs. bottom water εNd (Figure 5) illustrates that authigenic (archival) phases do trend with bottom water globally, but there is a remarkably uniform 1- εNd unit offset toward authigenic phases being more radiogenic: an offset equivalent in the Atlantic and the Pacific. Furthermore, we note that the Pacific data actually have a more robust correlation compared to the Atlantic data, consistent with the preceding discussion that benthic fluxes in the Atlantic are more difficult to identify from bottom water data. Bearing in mind that the concentration of Nd in the authigenic phases (at ppm Nd) is nominally six orders of magnitude greater than the bottom water (at pM Nd), it is difficult to explain these data without invoking a Nd source from within the sediments that is globally persistent. Of course, there are many outstanding questions to answer before better understanding the benthic flux of REEs and εNd: for example, can a flux be more non-radiogenic than the sediment host? How do these fluxes change over time?
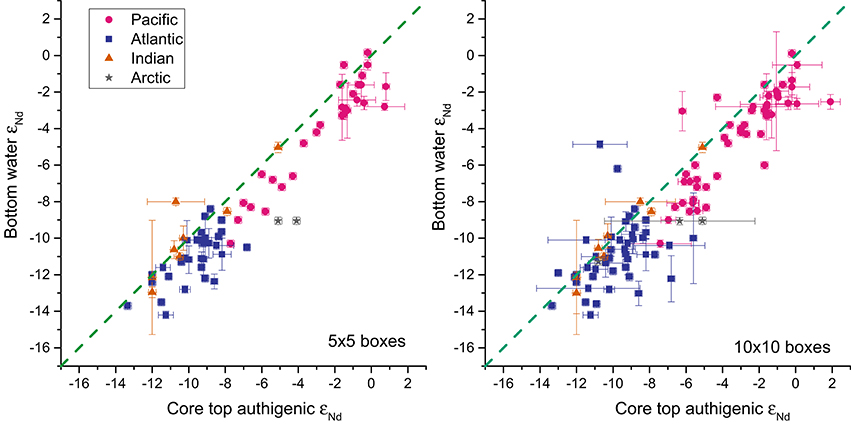
Figure 5. Compiled εNd from core top authigenic phases and Bottom water. These data are compiled as averages from all published data within 5° and 10° boxes (ranges within these boxes are shown as error bars). This data compilation is available upon request, or find a similar compilation from Tachikawa et al. (2017).
In summary, while complex, diagenesis does follow predictable rules that make it amenable to modeling, which, in turn, can provide a robust estimation of past changes in the benthic control on bottom water Nd. In turn, these modeled boundary conditions can be used to calculate current direction and velocities as suggested in the modern examples above. Moreover, such studies may provide further evidence for changes in pore waters that can relate to changes in ocean redox or acid-base chemistry. The benthic model adds complexity, but also potential for the application of [REE]/εNd.
Beyond the REEs and εNd
The REEs and εNd are often touted as being excellent tracers of processes that impact marine geochemical cycles in a much broader sense. As such, the hypothesis of benthic control on deep ocean REE cycling may support suggestions for the importance of these diagenetic processes in marine geochemical cycles in general. For example, the same authigenic Fe/Mn oxides apparently involved in the marine cycling of [REE]/εNd are also rich in other trace metals (e.g., Cu, Zn, Ni, Co). These authigenic metal oxides may be acting in an expansive way as a capacitor for geochemical fluxes; i.e., as both a source and sink of these trace metals.
Author Contributions
All authors listed have made a substantial, direct and intellectual contribution to the work, and approved it for publication.
Conflict of Interest Statement
The authors declare that the research was conducted in the absence of any commercial or financial relationships that could be construed as a potential conflict of interest.
Acknowledgments
This work was supported by NSF grants OCE-1147407 to JM and BH, OCE-1357529 to BH, and OCE-1715106 to JM. We thank Drs. V. Goswami and T. van de Flierdt for their comments, and Catherine Jeandel for editorial handling of this manuscript.
Supplementary Material
The Supplementary Material for this article can be found online at: https://www.frontiersin.org/articles/10.3389/fmars.2017.00426/full#supplementary-material
References
Abadie, C., Lacan, F., Radic, A., Pradoux, C., and Poitrasson, F. (2017). Iron isotopes reveal distinct dissolved iron sources and pathways in the intermediate versus deep Southern Ocean. Proc. Natl. Acad. Sci. U.S.A. 114, 858–863. doi: 10.1073/pnas.1603107114
Abbott, A. N., Haley, B. A., and McManus, J. (2015a). Bottoms up: sedimentary control of the deep North Pacific Ocean's εNd signature. Geology 43, 1035–1035. doi: 10.1130/G37114.1
Abbott, A. N., Haley, B. A., and McManus, J. (2016). The impact of sedimentary coatings on the diagenetic Nd flux. Earth Planet. Sci. Lett. 449, 217–227. doi: 10.1016/j.epsl.2016.06.001
Abbott, A. N., Haley, B. A., McManus, J., and Reimers, C. E. (2015b). The sedimentary flux of dissolved rare earth elements to the ocean. Geochim. Cosmochim. Acta 154, 186–200. doi: 10.1016/j.gca.2015.01.010
Akagi, T. (2014). Diatoms spread a high εNd-signature in the North Pacific Ocean. Geochem. J. 48, 121–131. doi: 10.2343/geochemj.2.0292
Akagi, T., Fu, F., Hongo, Y., and Takahashi, K. (2011). Composition of rare earth elements in settling particles collected in the highly productive North Pacific Ocean and Bering Sea: implications for siliceous-matter dissolution kinetics and formation of two REE-enriched phases. Geochim. Cosmochim. Acta 75, 4857–4876. doi: 10.1016/j.gca.2011.06.001
Arsouze, T., Dutay, J.-C., Lacan, F., and Jeandel, C. (2009). Reconstructing the Nd oceanic cycle using a coupled dynamical – biogeochemical model. Biogeosciences 6, 2829–2846. doi: 10.5194/bg-6-2829-2009
Bau, M., Tepe, N., and Mohwinkel, D. (2013). Siderophore-promoted transfer of rare earth elements and iron from volcanic ash into glacial meltwater, river and ocean water. Earth Planet. Sci. Lett. 364, 30–36. doi: 10.1016/j.epsl.2013.01.002
Bayon, G., German, C. R., Burton, K. W., Nesbitt, R. W., and Rogers, N. (2004). Sedimentary Fe–Mn oxyhydroxides as paleoceanographic archives and the role of aeolian flux in regulating oceanic dissolved REE. Earth Planet. Sci. Lett. 224, 477–492. doi: 10.1016/j.epsl.2004.05.033
Bayon, G., Toucanne, S., Skonieczny, C., André, L., Bermell, S., Cheron, S., et al. (2015). Rare earth elements and neodymium isotopes in world river sediments revisited. Geochim. Cosmochim. Acta 170, 17–38. doi: 10.1016/j.gca.2015.08.001
Bertram, C., and Elderfield, H. (1993). The geochemical balance of the rare earth elements and neodymium isotopes in the oceans. Geochim. Cosmochim. Acta 57, 1957–1986.
von Blanckenburg, F. (1999). Tracing past ocean circulation? Science 286, 1862–1863. doi: 10.1126/science.286.5446.1862b
Blaser, P., Lippold, J., Gutjahr, M., Frank, N., Link, J. M., and Frank, M. (2016). Extracting foraminiferal seawater Nd isotope signatures from bulk deep sea sediment by chemical leaching. Chem. Geol. 439, 189–204. doi: 10.1016/j.chemgeo.2016.06.024
Byrne, R. H., and Kim, K.-H. (1990). Rare earth element scavenging in seawater. Geochim. Cosmochim. Acta 54, 2645–2656. doi: 10.1016/0016-7037(90)90002-3
Byrne, R. H., and Sholkovitz, E. R. (1996). Chapter 158 Marine chemistry and geochemistry of the lanthanides. Handb. Phys. Chem. Rare Earths 22, 497–593. doi: 10.1016/S0168-1273(96)23009-0
Cantrell, K. J., and Byrne, R. H. (1987). Rare earth element complexation by carbonate and oxalate ions. Geochim. Cosmochim. Acta 51, 597–605. doi: 10.1016/0016-7037(87)90072-X
Carter, P., Vance, D., Hillenbrand, C. D., Smith, J. A., and Shoosmith, D. R. (2012). The neodymium isotopic composition of waters masses in the eastern Pacific sector of the Southern Ocean. Geochim. Cosmochim. Acta 79, 41–59. doi: 10.1016/j.gca.2011.11.034
Charette, M. A., Morris, P. J., Henderson, P. B., and Moore, W. S. (2015). Radium isotope distributions during the US GEOTRACES North Atlantic cruises. Mar. Chem. 177, 184–195. doi: 10.1016/j.marchem.2015.01.001
Davranche, M., Pourret, O., Gruau, G., Dia, A., and Le Coz-Bouhnik, M. (2005). Adsorption of REE(III)-humate complexes onto MnO2: experimental evidence for cerium anomaly and lanthanide tetrad effect suppression. Geochim. Cosmochim. Acta 69, 4825–4835. doi: 10.1016/j.gca.2005.06.005
Du, J., Haley, B., and Mix, A. (2016). Neodymium Isotopes in authigenic phases, bottom waters, and detrital sediments in the Gulf of Alaska and their implications for paleo-circulation reconstruction. Geochim. Cosmochim. Acta 193, 14–35. doi: 10.1016/j.gca.2016.08.005
Dunlea, A. G., Murray, R. W., Sauvage, J., Spivack, A. J., Harris, R. N., and D'Hondt, S. (2015). Dust, volcanic ash, and the evolution of the South Pacific Gyre through the Cenozoic. Paleoceanography 30, 1078–1099. doi: 10.1002/2015PA002829
Elderfield, H. (1988). The oceanic chemistry of the rare-earth elements [and discussion]. Philos. Trans. R. Soc. Lond. Math. Phys. Eng. Sci. 325, 105–126. doi: 10.1098/rsta.1988.0046
Elderfield, H., and Greaves, M. J. (1982). The rare earth elements in seawater. Nature 296, 214–219. doi: 10.1038/296214a0
Frank, M. (2002). Radiogenic isotopes: tracers of past ocean circulation and erosional input. Rev. Geophys. 40, 1-1–1-38. doi: 10.1029/2000RG000094
Freslon, N., Bayon, G., Toucanne, S., Bermell, S., Bollinger, C., Chéron, S., et al. (2014). Rare earth elements and neodymium isotopes in sedimentary organic matter. Geochim. Cosmochim. Acta 140, 177–198. doi: 10.1016/j.gca.2014.05.016
Fröllje, H., Pahnke, K., Schnetger, B., Brumsack, H.-J., Dulai, H., and Fitzsimmons, J. N. (2016). Haawiian imprint on dissolved Nd and Ra isotopes and rare earth elements in the central North Pacific: local survey and seasonal variability. Geochim. Cosmochim. Acta 189, 110–131. doi: 10.1016/j.gca.2016.06.001.
Garcia-Solsona, E., Jeandel, C., Labatut, M., Lacan, F., Vance, D., Chavagnac, V., et al. (2014). Rare earth elements and Nd isotopes tracing water mass mixing and particle-seawater interactions in the SE Atlantic. Geochim. Cosmochim. Acta 125, 351–372. doi: 10.1016/j.gca.2013.10.009
German, C. R., and Elderfield, H. (1990). Rare earth elements in the NW Indian Ocean. Geochim.Cosmochim. Acta 54, 1929–1940.
Goldstein, S. J., and Jacobsen, S. B. (1987). The Nd and Sr isotopic systematics of river-water dissolved material: Implications for the sources of Nd and Sr in seawater. Chem. Geol. Isot. Geosci. Sect. 66, 245–272. doi: 10.1016/0168-9622(87)90045-5
Goldstein, S. J., and Jacobsen, S. B. (1988). Nd and Sr isotopic systematics of river water suspended material: implications for crustal evolution. Earth Planet. Sci. Lett. 87, 249–265. doi: 10.1016/0012-821X(88)90013-1
Goldstein, S. L., and Hemming, S. R. (2003). “Long-lived isotopic tracers in oceanography, paleoceanography, and ice-sheet dynamics,” in Treatise on Geochemistry, eds H. D. Holland, and K. K. Turekian (Pergamon; Oxford), 453–489.
Goswami, V., Singh, S. K., and Bhushan, R. (2014). Impact of water mass mixing and dust deposition on Nd concentration and eNd of the Arabian Sea water column. Geochim. Cosmochim. Acta 145, 30–49. doi: 10.1016/j.gca.2014.09.006
Grasse, P., Stichel, T., Stumpf, R., Stramma, L., and Frank, M. (2012). The distribution of neodymium isotopes and concentrations in the Eastern Equatorial Pacific: water mass advection versus particle exchange. Earth Planet. Sci. Lett. 353–354, 198–207. doi: 10.1016/j.epsl.2012.07.044
Greaves, M. J., Elderfield, H., and Sholkovitz, E. R. (1999). Aeolian sources of rare earth elements to the Western Pacific Ocean. Mar. Chem. 68, 31–38.
Grenier, M., Jeandel, C., Lacan, F., Vance, D., Venchiarutti, C., Cros, A., et al. (2013). From the subtropics to the central equatorial Pacific Ocean: neodymium isotopic composition and rare earth element concentration variations. J. Geophys. Res. Oceans 118, 592–618. doi: 10.1029/2012JC008239
Gutjahr, M., Frank, M., Stirling, C. H., Keigwin, L. D., and Halliday, A. N. (2008). Tracing the Nd isotope evolution of North Atlantic Deep and Intermediate Waters in the western North Atlantic since the Last Glacial Maximum from Blake Ridge sediments. Earth Planet. Sci. Lett. 266, 61–77. doi: 10.1016/j.epsl.2007.10.037
Gutjahr, M., Frank, M., Stirling, C. H., Klemm, V., van de Flierdt, T., and Halliday, A. N. (2007). Reliable extraction of a deepwater trace metal isotope signal from Fe–Mn oxyhydroxide coatings of marine sediments. Chem. Geol. 242, 351–370. doi: 10.1016/j.chemgeo.2007.03.021
Haine, T. W. N., Watson, A. J., Liddicoat, M. I., and Dickson, R. R. (1998). The flow of Antarctic bottom water to the southwest Indian Ocean estimated using CFCs. J. Geophys. Res. Oceans 103, 27637–27653. doi: 10.1029/98JC02476
Haley, B. A., Frank, M., Hathorne, E., and Pisias, N. (2014). Biogeochemical implications from dissolved rare earth element and Nd isotope distributions in the Gulf of Alaska. Geochim. Cosmochim. Acta 126, 455–474. doi: 10.1016/j.gca.2013.11.012
Haley, B. A., and Klinkhammer, G. P. (2003). Complete separation of rare earth elements from small volume seawater samples by automated ion chromatography: method development and application to benthic flux. Mar. Chem. 82, 197–220. doi: 10.1016/S0304-4203(03)00070-7
Haley, B. A., Klinkhammer, G. P., and McManus, J. (2004). Rare earth elements in pore waters of marine sediments. Geochim. Cosmochim. Acta 68, 1265–1279. doi: 10.1016/j.gca.2003.09.012
Howe, J. N. W., Piotrowski, A. M., Oppo, D. W., Huang, K.-F., Mulitza, S., Chiessi, C. M., et al. (2016). Antarctic intermediate water circulation in the South Altantic over the past 25,000 years. Paleoc 31, 1302–1314. doi: 10.1002/2016PA002975
Hu, R., Piotrowski, A. M., Bostock, H. C., Crowhurst, S., and Rennie, V. (2016). Variability of neodymium isotopes associated with planktonic foraminifera in the Pacific Ocean during the Holocene and last glacial maximum. Earth Planet. Sci. Lett. 447, 130–138. doi: 10.1016/j.epsl.2016.05.011
Huck, C. E., van de Flierdt, T., Jiménez-Espejo, F. J., Bohaty, S. M., Röhl, U., and Hammond, S. J. (2016). Robustness of fossil fish teeth for seawater neodymium isotope reconstructions under variable redox conditions in an ancient shallow marine setting: robustness of fossil fish tooth nd. Geochem. Geophys. Geosyst. 17, 679–698. doi: 10.1002/2015GC006218
Jacobsen, S. B., and Wasserburg, G. J. (1980). Sm-Nd isotopic evolution of chondrites. Earth Planet. Sci. Lett. 50, 139–155. doi: 10.1016/0012-821X(80)90125-9
Jeandel, C. (2016). Overview of the mechanisms that could explain the ‘Boundary Exchange’ at the land–ocean contact. Philos. Trans. R. Soc. Math. Phys. Eng. Sci. 374:20150287. doi: 10.1098/rsta.2015.0287
Jeandel, C., Bishop, J. K., and Zindler, A. (1995). Exchange of neodymium and its isotopes between seawater and small and large particles in the Sargasso Sea. Geochim. Cosmochim. Acta 59, 535–547. doi: 10.1016/0016-7037(94)00367-U
Jeandel, C., Thouron, D., and Fieux, M. (1998). Concentrations and isotopic compositions of neodymium in the eastern Indian Ocean and Indonesian straits. Geochim. Cosmochim. Acta 62, 2597–2607. doi: 10.1016/S0016-7037(98)00169-0
Johannesson, K. H., and Burdige, D. J. (2007). Balancing the global oceanic neodymium budget: Evaluating the role of groundwater. Earth Planet. Sci. Lett. 253, 129–142. doi: 10.1016/j.epsl.2006.10.021
Johannesson, K. H., Chevis, D. A., Burdige, D. J., Cable, J. E., Martin, J. B., and Roy, M. (2011). Submarine groundwater discharge is an important net source of light and middle REEs to coastal waters of the Indian River Lagoon, Florida, USA. Geochim. Cosmochim. Acta 75, 825–843. doi: 10.1016/j.gca.2010.11.005
Johannesson, K. H., Palmore, C. D., Fackrell, J., Prouty, N. G., Swarzenski, P. W., Chevis, D. A., et al. (2017). Rare earth element behavior during groundwater–seawater mixing along the Kona Coast of Hawaii. Geochim. Cosmochim. Acta 198, 229–258. doi: 10.1016/j.gca.2016.11.009
Jones, C. E., Halliday, A. N., Rea, D. K., and Owen, R. M. (1994). Neodymium isotopic variations in North Pacific modern silicate sediment and the insignificance of detrital REE contributions to seawater. Earth Planet. Sci. Lett. 127, 55–66. doi: 10.1016/0012-821X(94)90197-X
Jones, K. M., Khatiwala, S. P., Goldstein, S. L., Hemming, S. R., and van de Flierdt, T. (2008). Modeling the distribution of Nd isotopes in the oceans using an ocean general circulation model. Earth Planet. Sci. Lett. 272, 610–619. doi: 10.1016/j.epsl.2008.05.027
Ku, T.-L., and Luo, S. (1994). New appraisal of radium 226 as a large-scale oceanic mixing tracer. J. Geophys. Res. Oceans 99, 10255–10273. doi: 10.1029/94JC00089
Lacan, F., and Jeandel, C. (2001). Tracing Papua New Guinea imprint on the central equatorial Pacific Ocean using neodymium isotopic compositions and rare earth element patterns. Earth Planet. Sci. Lett. 186, 497–512. doi: 10.1016/S0012-821X(01)00263-1
Lacan, F., and Jeandel, C. (2004a). Subpolar Mode Water formation traced by neodymium isotopic composition. Geophys. Res. Lett. 31:L14306. doi: 10.1029/2004GL019747
Lacan, F., and Jeandel, C. (2004b). Neodymium isotopic composition and rare earth element concentrations in the deep and intermediate Nordic Seas: constraints on the Iceland Scotland overflow water signature. Geochem. Geophys. Geosystems 5:Q11006. doi: 10.1029/2004GC000742
Lacan, F., and Jeandel, C. (2004c). Denmark Strait water circulation traced by heterogeneity in neodymium isotopic compositions. Deep Sea Res. I Oceanogr. Res. Pap. 51, 71–82. doi: 10.1016/j.dsr.2003.09.006
Lacan, F., and Jeandel, C. (2005a). Acquisition of the neodymium isotopic composition of the North Atlantic Deep Water. Geochem. Geophys. Geosyst. 6:Q12008. doi: 10.1029/2005GC000956
Lacan, F., and Jeandel, C. (2005b). Neodymium isotopes as a new tool for quantifying exchange fluxes at the continent–ocean interface. Earth Planet. Sci. Lett. 232, 245–257. doi: 10.1016/j.epsl.2005.01.004
Lambelet, M., van de Flierdt, T., Crocket, K., Rehkämper, M., Kreissig, K., Coles, B., et al. (2016). Neodymium isotopic composition and concentration in the western North Atlantic Ocean: results from the GEOTRACES GA02 section. Geochim. Cosmochim. Acta 177, 1–29. doi: 10.1016/j.gca.2015.12.019
Martin, J. H., Knauer, G. A., Karl, D. M., and Broenkow, W. W. (1987). VERTEX: carbon cycling in the northeast Pacific. Deep Sea Res. Oceanogr. Res. Pap. 34, 267–285.
Mawji, E., Schlitzer, R., Dodas, E. M., Abadie, C., Abouchami, W., Anderson, R. F., et al. (2015). The GEOTRACES Intermediate Data Product 2014. Mar. Chem. 177, 1–8. doi: 10.1016/j.marchem.2015.04.005
Millero, F. J. (1992). Stability constants for the formation of rare earth-inorganic complexes as a function of ionic strength. Geochim. Cosmochim. Acta 56, 3123–3132. doi: 10.1016/0016-7037(92)90293-R
Morel, F. M. M., and Hering, J. G. (1993). Principles and Applications of Aquatic Chemistry, 1st Edn. New York, NY: Wiley-Interscience.
Ngwenya, B. T., Magennis, M., Olive, V., Mosselmans, J. F. W., and Ellam, R. M. (2010). Discrete Site surface complexation constants for lanthanide adsorption to bacteria as determined by experiments and linear free energy relationships. Environ. Sci. Technol. 44, 650–656. doi: 10.1021/es9014234
Osborne, A. H., Haley, B. A., Hathorne, E. C., Flögel, S., and Frank, M. (2014). Neodymium isotopes and concentrations in Caribbean seawater: Tracing water mass mixing and continental input in a semi-enclosed ocean basin. Earth Planet. Sci. Lett. 406, 174–186. doi: 10.1016/j.epsl.2014.09.011
Piepgras, D. J., and Wasserburg, G. J. (1980). Neodymium isotopic variations in seawater. Earth Planet. Sci. Lett. 50, 128–138. doi: 10.1016/0012-821X(80)90124-7
Piepgras, D. J., and Wasserburg, G. J. (1987). Rare earth element transport in the western North Atlantic inferred from Nd isotopic observations. Geochim. Cosmochim. Acta 51, 1257–1271. doi: 10.1016/0016-7037(87)90217-1
Piotrowski, A. M., Goldstein, S. L., Hemming, S. R., and Fairbanks, R. G. (2004). Intensification and variability of ocean thermohaline circulation through the last deglaciation. Earth Planet. Sci. Lett. 225, 205–220. doi: 10.1016/j.epsl.2004.06.002
Piotrowski, A. M., Goldstein, S. L., Hemming, S. R., and Fairbanks, R. G. (2005). Temporal relationships of carbon cycling and ocean circulation at glacial boundaries. Science 307, 1933–1938. doi: 10.1126/science.1104883
Pourret, O., Davranche, M., Gruau, G., and Dia, A. (2007). Organic complexation of rare earth elements in natural waters: Evaluating model calculations from ultrafiltration data. Geochim. Cosmochim. Acta 71, 2718–2735. doi: 10.1016/j.gca.2007.04.001
Rempfer, J., Stocker, T. F., Joos, F., Dutay, J.-C., and Siddall, M. (2011). Modelling Nd-isotopes with a coarse resolution ocean circulation model: Sensitivities to model parameters and source/sink distributions. Geochim. Cosmochim. Acta 75, 5927–5950. doi: 10.1016/j.gca.2011.07.044
Rickli, J., Frank, M., Baker, A. R., Aciego, S., de Souza, G., Georg, R. B., et al. (2010). Hafnium and neodymium isotopes in surface waters of the eastern Atlantic Ocean: Implications for sources and inputs of trace metals to the ocean. Geochim. Cosmochim. Acta 74, 540–557. doi: 10.1016/j.gca.2009.10.006
Rickli, J., Frank, M., and Halliday, A. N. (2009). The hafnium–neodymium isotopic composition of Atlantic seawater. Earth Planet. Sci. Lett. 280, 118–127. doi: 10.1016/j.epsl.2009.01.026
Rickli, J., Gutjahr, M., Vance, D., Fischer-Gödde, M., Hillenbrand, C.-D., and Kuhn, G. (2014). Neodymium and hafnium boundary contributions to seawater along the West Antarctic continental margin. Earth Planet. Sci. Lett. 394, 99–110. doi: 10.1016/j.epsl.2014.03.008
Roberts, N. L., and Piotrowski, A. M. (2015). Radiogenic Nd isotope labeling of the northern NE Atlantic during MIS 2. Earth Planet. Sci. Lett. 423, 125–133. doi: 10.1016/j.epsl.2015.05.011
Rousseau, T. C. C., Sonke, J. E., Chmeleff, J., van Beek, P., Souhaut, M., Boaventura, G., et al. (2015). Rapid neodymium release to marine waters from lithogenic sediments in the Amazon estuary. Nat. Commun. 6:7592. doi: 10.1038/ncomms8592
Schacht, U., Wallmann, K., and Kutterolf, S. (2010). The influence of volcanic ash alteration on the REE composition of marine pore waters. J. Geochem. Explor. 106, 176–187. doi: 10.1016/j.gexplo.2010.02.006
Schijf, J., Christenson, E. A., and Byrne, R. H. (2015). YREE scavenging in seawater: a new look at an old model. Mar. Chem. 177, Part 3, 460–471. doi: 10.1016/j.marchem.2015.06.010
Shiller, A. M., Chan, E. W., Joung, D. J., and Redmond, M. C. Kessler, J. D. (2017). Light rare earth element depletion during Deepwater Horizon blowout methanotrophy. Sci. Rep. 7:10389. doi: 10.1038/s41598-017-11060-z
Sholkovitz, E. R. (1989). Artifacts associated with the chemical leaching of sediments for rare-earth elements. Chem. Geol. 77, 47–51.
Sholkovitz, E. R. (1993). The geochemistry of rare earth elements in the Amazon River estuary. Geochim. Cosmochim. Acta 57, 2181–2190. doi: 10.1016/0016-7037(93)90559-F
Sholkovitz, E. R., Landing, W. M., and Lewis, B. L. (1994). Ocean particle chemistry: the fractionation of rare earth elements between suspended particles and seawater. Geochim. Cosmochim. Acta 58, 1567–1579. doi: 10.1016/0016-7037(94)90559-2
Sholkovitz, E. R., Piepgras, D. J., and Jacobsen, S. B. (1989). The pore water chemistry of rare earth elements in Buzzards Bay sediments. Geochim. Cosmochim. Acta 53, 2847–2856. doi: 10.1016/0016-7037(89)90162-2
Singh, S. P., Singh, S. K., Goswami, V., Bhushan, R., and Rai, V. K. (2012). Spatial distribution of dissolved neodymium and eNd in the Bay of Bengal: role of particulate matter and mixing of water masses. Geochim. Cosmochim. Acta, 94, 38–96, doi: 10.1016/j.gca.2012.07.017.
Smethie, W. M., Fine, R. A., Putzka, A., and Jones, E. P. (2000). Tracing the flow of North Atlantic Deep Water using chlorofluorocarbons. J. Geophys. Res. Oceans 105, 14297–14323. doi: 10.1029/1999JC900274
Spivack, A. J., and Wasserburg, G. J. (1988). Neodymium isotopic composition of the Mediterranean outflow and the eastern North Atlantic. Geochim. Cosmochim. Acta 52, 2767–2773.
Stanley, J. K., and Byrne, R. H. (1990). The influence of solution chemistry on REE uptake by Ulva lactuca L. in seawater. Geochim. Cosmochim. Acta 54, 1587–1595. doi: 10.1016/0016-7037(90)90393-Y
Steinfeldt, R., and Rhein, M. (2004). Spreading velocities and dilution of North Atlantic Deep Water in the tropical Atlantic based on CFC time series. J. Geophys. Res. Oceans 109:C03046. doi: 10.1029/2003JC002050
Stewart, J. A., Gutjahr, M., James, R. H., Anand, P., and Wilson, P. A. (2016). Influence of the Amazon River on the Nd isotope composition of deep water in the western equatorial Atlantic during the Oligocene–Miocene transition. Earth Planet. Sci. Lett. 454, 132–141. doi: 10.1016/j.epsl.2016.08.037
Stichel, T., Frank, M., Rickli, J., and Haley, B. A. (2012). The hafnium and neodymium isotope composition of seawater in the Atlantic sector of the Southern Ocean. Earth Planet. Sci. Lett. 317–318, 282–294. doi: 10.1016/j.epsl.2011.11.025
Stichel, T., Hartman, A. E., Duggan, B., Goldstein, S. L., Scher, H., and Pahnke, K. (2015). Separating biogeochemical cycling of neodymium from water mass mixing in the Eastern North Atlantic. Earth Planet. Sci. Lett. 412, 245–260. doi: 10.1016/j.epsl.2014.12.008
Stumpf, R., Frank, M., Schoenfeld, J., and Haley, B. A. (2010). Late Quaternary variability of Mediterranean Outflow Water from radiogenic Nd and Pb isotopes. Quat. Sci. Rev. 29, 2462–2472, doi: 10.1016/j.quatscirev.2010.06.021
Suess, E. (1980). Particulate organic carbon flux in the oceans - surface productivity and oxygen utilization. Nature 288, 260–263.
Tachikawa, K., Arsouze, T., Bayon, G., Bory, A., Colin, C., Dutay, J.-C., et al. (2017). The large-scale evolution of neodymium isotopic composition in the global modern and Holocene ocean revealed from seawater and archive data. Chem. Geol. 457, 131–148. doi: 10.1016/j.chemgeo.2017.03.018
Tachikawa, K., Athias, V., and Jeandel, C. (2003). Neodymium budget in the modern ocean and paleo-oceanographic implications. J. Geophys. Res. Oceans 108:3254. doi: 10.1029/1999JC000285
Tachikawa, K., Jeandel, C., and Roy-Barman, M. (1999b). A new approach to the Nd residence time in the ocean: the role of atmospheric inputs. Earth Planet. Sci. Lett. 170, 433–446.
Tachikawa, K., Jeandel, C., Vangriesheim, A., and Dupré, B. (1999a). Distribution of rare earth elements and neodymium isotopes in suspended particles of the tropical Atlantic Ocean (EUMELI site). Deep Sea Res. Oceanogr. Res. Pap. 46, 733–755.
van de Flierdt, T., and Frank, M. (2010). Neodymium isotopes in paleoceanography. Quat. Sci. Rev. 29, 2439–2441. doi: 10.1016/j.quascirev.2010.07.001
van de Flierdt, T., Griffiths, A. M., Lambelet, M., Little, S. H., Stichel, T., and Wilson, D. J. (2016). Neodymium in the oceans: a global database, a regional comparison and implications for palaeoceanographic research. Phil. Trans. R. Soc. A 374:20150293. doi: 10.1098/rsta.2015.0293
Waterhouse, A. F., MacKinnon, J. A., Nash, J. D., Alford, M. H., Kunze, E., Simmons, H. L., et al. (2014). Global patterns of diapycnal mixing from measurements of the turbulent dissipation rate. J. Phys. Oceanogr. 44, 1854–1872. doi: 10.1175/JPO-D-13-0104.1
Wilson, D. J., Piotrowski, A. M., Galy, A., and Clegg, J. A. (2013). Reactivity of neodymium carriers in deep sea sediments: Implications for boundary exchange and paleoceanography. Geochim. Cosmochim. Acta 109, 197–221. doi: 10.1016/j.gca.2013.01.042
Yang, J., and Haley, B. (2016). The profile of the rare earth elements in the Canada Basin, Arctic Ocean. Geochem. Geophys. Geosystems 17, 3241–3253. doi: 10.1002/2016GC006412
Keywords: rare earth elements, neodymium isotopes, marine geochemistry, benthic flux
Citation: Haley BA, Du J, Abbott AN and McManus J (2017) The Impact of Benthic Processes on Rare Earth Element and Neodymium Isotope Distributions in the Oceans. Front. Mar. Sci. 4:426. doi: 10.3389/fmars.2017.00426
Received: 02 October 2017; Accepted: 12 December 2017;
Published: 21 December 2017.
Edited by:
Catherine Jeandel, UMR5566 Laboratoire d'études en Géophysique et Océanographie Spatiales (LEGOS), FranceReviewed by:
Tina Van De Flierdt, Imperial College London, United KingdomVineet Goswami, Colorado State University, United States
Copyright © 2017 Haley, Du, Abbott and McManus. This is an open-access article distributed under the terms of the Creative Commons Attribution License (CC BY). The use, distribution or reproduction in other forums is permitted, provided the original author(s) or licensor are credited and that the original publication in this journal is cited, in accordance with accepted academic practice. No use, distribution or reproduction is permitted which does not comply with these terms.
*Correspondence: Brian A. Haley, YmhhbGV5QGNvYXMub3JlZ29uc3RhdGUuZWR1