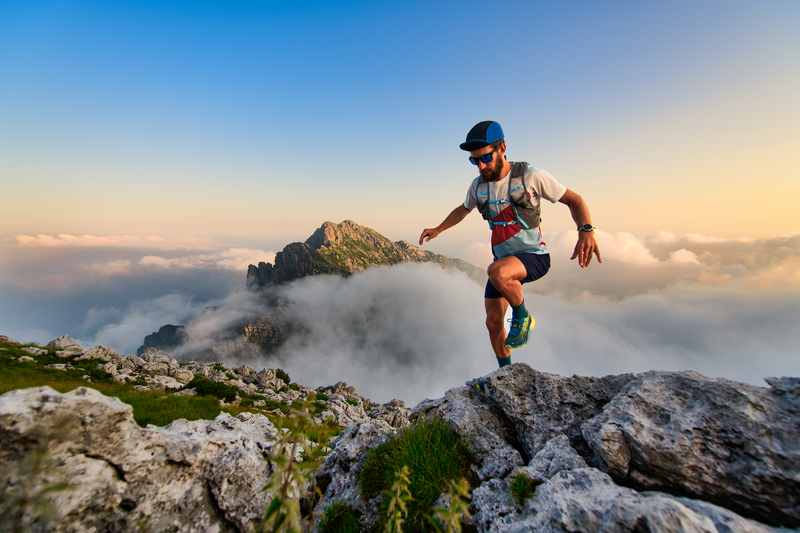
95% of researchers rate our articles as excellent or good
Learn more about the work of our research integrity team to safeguard the quality of each article we publish.
Find out more
ORIGINAL RESEARCH article
Front. Mar. Sci. , 30 June 2017
Sec. Coral Reef Research
Volume 4 - 2017 | https://doi.org/10.3389/fmars.2017.00212
This article is part of the Research Topic The Pathway to Solutions: New Frontiers in Climate Change Adaptation & Mitigation View all 13 articles
Coralline algae provide important ecosystem services. In situ observations of how they respond to different environmental conditions can help us to understand (i) their ability to adapt to their local environment and (ii) their capacity to acclimatize to a novel thermal regime. Here, individuals of the tropical coralline algae, Lithophyllum kotschyanum, were translocated on a coral reef from thermally stable areas to areas characterized by natural temperature variability. Changes in their photosynthetic efficiency were determined using pulse amplitude modulation (PAM) chlorophyll fluorescence. Despite an initial stress response, algae exposed to increases in thermal variation recovered within 24 hours, indicating a rapid, short-term acclimatization capacity. Algae naturally inhabiting thermally variable areas of the reef showed no change in photosynthetic efficiency throughout the study suggesting longer-term adaptation to living in a variable environment also occurs. However, coralline algae living in thermally stable reef areas were abundant and marginally larger, suggesting physiological trade-offs are used to survive in variable environments. Thus, our results suggest that while coralline algae can survive in environmentally variable conditions, there may be structural and ecosystem costs.
Red coralline algae (Rhodophyta: Corallinales) are bio-constructors which have a world-wide distribution and play important structural and ecosystem roles (Foster, 2002). On coral reefs, encrusting morphotypes stabilize the reef structure by forming the algal ridge, enabling reefs to withstand damage from wave action (Tierney and Johnson, 2011). In addition, they provide important settlement cues for juvenile corals (Morse, 1996). The complex structure of free-living coralline algal beds increases habitat heterogeneity, allowing them to have high associated biodiversity (BIOMAERL Team, 1999; Steller et al., 2003) and significant roles in ecosystem service provision (Kamenos et al., 2004; McCoy and Kamenos, 2015).
Despite their large distribution range throughout temperate and tropical oceans, coralline algae are sensitive to changes in irradiance, pCO2, sedimentation, desiccation, salinity and temperature (Wilson et al., 2004; Johnson et al., 2014; Reynier et al., 2015). Recent evidence suggests that tropical coralline algae are locally adapted to their thermal regime, with populations from two reefs differing in their optimal temperature and ability to cope with thermal stress (Siboni et al., 2015). It is well known that through physiological and genetic adaptation, species are able to maintain optimal physiological fitness within their environment (Hoegh-Guldberg and Bruno, 2010; Somero, 2010).
Physiological responses, often referred to as plasticity or acclimatization, can take place over short time scales (hours to days) allowing species to survive (Kingsolver et al., 2001; Stockwell et al., 2003; Berteaux et al., 2004) and in the long term, can be adaptive (Edmunds and Gates, 2008; Hofmann and Todgham, 2010). However, species with long generation times and clonal formation, such as coral reef species, may show significantly slower response rates (Lasker and Coffroth, 1999; Berteaux et al., 2004). Beyond the range of habitable environmental conditions, reduced fitness, increased mortality and greater disease susceptibility can result in local population declines and extinctions. This leads to fundamental changes in assemblage composition which are difficult to project, and in many cases are irreversible (Hochachka and Somero, 2002; Hoegh-Guldberg and Bruno, 2010).
Observations of how coralline algae respond to environmental conditions have helped us to understand and their ability to adapt to their local environment. The majority of these observations have explored the consequences of anthropogenic climate change on algae's eco-physiology, with often negative projections (e.g., Burdett et al., 2013; Johnson et al., 2014; Guy-Haim et al., 2016). For example, pCO2 fluctuations were found to decrease calcification rate of coralline algae, although these may be context-dependent (Johnson et al., 2014). Similarly, changes in temperature, pH, and light intensities have been found to cause decreased net calcification rates (Martin and Gattuso, 2009; Johnson and Carpenter, 2012; Comeau et al., 2014), increased bleaching of surface tissue (Anthony et al., 2008) and decreased abundance (Kuffner et al., 2007; Fabricius et al., 2014).
Whilst laboratory experiments are informative and commonly implemented, in situ experimental studies are largely lacking. The advantage of in situ observations is the ability to understand both the capacity and speed of an organism to acclimatize to natural variation in an ecosystem context, and thus is more likely to reflect realistic and complex nature of the environment (Martone et al., 2010; Martin et al., 2013). Fabricius et al. (2015) exploited natural variation in CO2 to study the effects of decreased pH on coralline algae settlement and abundance whilst also taking into account light intensity and grazing pressure. After 5 months, the authors observed a steep transition from coralline algae presence to absence with decreasing pH. Fringing coral reefs also have naturally varying habitats that can be exploited in a similar way to investigate the acclimatization potential of coralline algae. To estimate the physiological response of coralline algae to an environment, photosynthetic efficiency (photosynthetic yield obtained from fluorescence measurements) can be used as a proxy for physiological stress, indicating sensitivity to environmental variation (Blake and Maggs, 2003; Burdett et al., 2012, 2014) and therefore as an indicator of acclimatization to environmental change.
We investigated if free-living coralline algae from thermally stable areas of the reef (reef crest) can photosynthetically acclimatize to thermally variable (reef flat) environments using translocation. This has significance in understanding the ability of coralline algae to acclimatize and adapt to local, within-reef environmental conditions.
Environmental conditions vary spatially between the reef crest and the reef flat on fringing coral reefs. The reef crest is connected to the open ocean and has a relatively stable environment, characterized by limited changes in temperature, dissolved inorganic carbon (DIC) and pH (Sheppard et al., 2009; Burdett et al., 2012, 2013, 2014). However, the reef flat has a reduced connection to the open ocean at low tide and is characterized by larger variations in temperature, DIC and pH (Sheppard et al., 2009; Burdett et al., 2017). It is possible that coralline algae on the reef flat represent a “sink” population, as is often the case with populations over a heterogeneous habitats (Pulliam, 1988), whereby populations in environmentally less benign conditions are maintained by recruitment from a nearby “source” population (i.e., on the crest). Thus, for the population found on the reef crest, the reef flat represents a novel thermal regime and a natural system to investigate the response of marine organisms to increases in marine environmental variability.
Photosynthetic yield acclimatization experiments were conducted on the free-living coralline alga Lithophyllum kotschyanum in-situ during November 2012 on subtidal areas (~1 m deep) of Suleman Reef, Sinai Peninsula, Egypt (28°28.79'N, 34°30.83'E). The reef is characterized by a reef flat, crest and slope, with an average tidal range of ~1 m. The reef flat is 100 m in width with a depth of 0.8 m at high tide and is dominated by macroalgae including Padina sp., L. kotschyanum, as well as filamentous turf algae. At the edge of the reef flat, the reef crest is predominantly encrusted with Porolithon sp. (0.5 m deep at high tide) and is 20 m in extent. The reef slope is dominated by massive (Porites sp.) and branching (Acropora sp.) corals to a depth of 8 m. The topography of Suleman reef is such that at low tide, the reef crest remains exposed to seawater but the reef flat no longer receives input, analogous to a rock pool situated on the upper littoral zone being more exposed to variation than one closer to the sublittoral zone. The reef flat at this location is characterized by higher variability in environmental conditions than the adjacent reef crest. The reef flat has a greater temperature range (3.15°C) than the reef crest (1.5°C) and dissolved oxygen concentrations have a greater range at the reef flat (60–160%) than at the reef crest (70–110%) (Burdett et al., 2013) in the summer months. Annual temperatures in the region vary by approximately 1.33°C in winter (average 25.51°C) and summer (average 26.84°C) (Eladawy et al., 2015). The shallow depth and macroalgal cover of the reef flat also mean that biological processes (photosynthesis and respiration) impact carbon chemistry to a greater extent than in the reef crest, resulting in variable dissolved inorganic carbon (Burdett et al., 2013).
Water temperature was recorded every 4 h over a 4-day period using temperature data loggers (Gemini Data Loggers Ltd., UK) on both the reef crest and flat. All research was approved by the Dahab Marine Research Center and the Marine Environmental Center. Suez Canal University provided the permit to conduct the research.
Chlorophyll-a fluorescence Pulse Amplitude Modulation (PAM) techniques use pulses of actinic saturated light of predefined frequencies and intensities, providing measurements of the algae's response to light limitation and saturation (Burdett et al., 2012). Environmental stresses such as solar radiation can result in a decrease in photochemistry and changes in heat dissipation (increased heat and fluorescence emitted) (Burdett et al., 2014). PAM can detect such changes in florescence by measuring the ratio of variable (Fv) to maximum (Fm) florescence. This ratio can be used as an indication of photosynthetic efficiency and, therefore, photophysiological stress (Blake and Maggs, 2003; Burdett et al., 2012, 2014). In this study, chlorophyll-a fluorescence yield (Fv/Fm) was determined in-situ using a Walz diving-PAM fluorometer and Win-Control software (Walz GmbH, Effeltrich, Germany) following the methodology in Burdett et al. (2012). Measurements were taken on the topside of the thallus and the position of the fibre optic cable on each thallus was consistent between measurements at a depth of 0.5 m. Environmental conditions (wave action, wind, cloud cover) were noted but did not differ across sampling days and were therefore excluded. Throughout the study period data were collected within a 1-h time frame to ensure changes in Photosynthetically Active Radiation (PAR) would not confound the results.
Four algal treatments were used (Figure 1) (1) Crest control individuals that were randomly selected (n = 5) from the reef crest and (2) Flat control individuals that were randomly selected (n = 5) from the reef flat. In order to show that translocation had no effect on photosynthetic efficiency, (3) Handling effect individuals were randomly selected (n = 5) from the reef crest and were moved to a different location within the reef crest. (4) Acclimatization individuals were randomly selected (n = 5) from the reef crest and were relocated to the reef flat. All measurements and PAM analyses were conducted in-situ using snorkeling. In order to decrease any handling effect, translocated individuals were kept immersed constantly (rather than lifted) and transported within ~10 min to the adjacent reef flat (distance ~50 m). Because we measured photosynthetic efficiency at constant PAR (i.e., within 1 h window), we were not able to reciprocally transplant algae from the reef flat to the crest due to time constraints.
Figure 1. A schematic diagram of the experimental set up, showing the position of individual coralline algae on Suleman Reef (n = 5 per group). Arrows indicate the direction and place of translocation for both the handling and acclimatization groups.
For all treatment groups, chlorophyll-a fluorescence measurements were taken over a 4-day period with translocations taking place after day 1 measurements. In some instances only four replicate measurements could be obtained due to logistical constraints.
Each day, measurements were taken in the morning between 0700 and 0800 and again between 1000 and 1100. The sampling times were chosen as, at this location, photosynthetic efficiency was greatest in the early morning and lowest between 1000 and 1400, correlating with low and maximum PAR respectively (Burdett et al., 2014).
The abundance of free-living L. kotschyanum was determined using five 40 m2 (20 × 2 m) belt transects on the reef crest and flat (on both macroalgae and sand dominated areas of the flat). The longest axis of randomly selected L. kotschyanum (n = 30 in each location) was measured to determine mean longest axis size.
An F-Test of equal variance and a Student's two tailed t-test were performed to analyze differences in temperature between sites. To explore differences in photosynthetic efficiency between treatment groups, analyses of variance (ANOVA) and repeated measures ANOVAs (with individual nested in treatment) were used. The following analyses were carried out; (1) between all treatment groups at day 1 to assess differences prior to translocation, (2) between crest control and handling effect groups to ensure that handling alone had no effects, (3) within the acclimatization group across the 4 day experimental period and (4) within the flat control group across the 4 day experimental period. In all cases, underlying assumptions of ANOVA were met. Pairwise t-tests were used to compare size and abundance of individuals between the reef crest and flat areas. All statistical analyses were conducted using R v3.1.2 (R Development Core Team, 2014).
There was higher variation in temperature on the reef flat (mean: 25.75, ± 1.692 SD) than the reef crest (mean: 25.34, ± 0.359 SD, F-test, P < 0.001) and absolute temperature also differed between sites, with a mean higher temperature at the reef flat (T = 5.77, P ≤ 0.001) (Figure 2). All treatment groups showed diurnal variation in photosynthetic efficiency, with decreased photosynthetic yield at 1100 relative to 0700 (Figure 3). Moreover, those measurements taken at 1100 show significant variation between and within treatment groups caused by high PAR.
Figure 2. Temperature (°C) measurements taken from the reef flat (black line) and reef crest (gray line, dashed) located on Suleman Reef, South Sinai, Egypt. Temperature was taken every 4 h over a 4 day period (Nov) at ~1 m depth.
Figure 3. Mean (±SD) photosynthetic efficiency (FV/FM) for each of the four treatment groups over the 4 day period for (A) 0700, Low PAR and (B) 1100, high PAR. Flat and crest control groups: individuals measured without moving from respective locations on reef; handling group: individuals moved from reef crest to another location within reef crest; acclimatization group: individuals moved from reef crest to reef flat. Dashed vertical line represents time of translocation for handling and acclimatization groups.
There were no significant differences in Fv/Fm yield between crest (mean: 0.48, ± 0.004 SD) and flat control (mean: 0.52, ± 0.005 SD) groups at 0700 [F(1, 32) = 2.05, P = 0.162, difference of means: 0.040] or over the study period [F(3, 32) = 0.34, P = 0.798]. Furthermore, Fv/Fm did not differ significantly between any of the treatment groups prior to translocation on day 1 [F(3, 16) = 1.09, P = 0.383] and there were no significant differences in Fv/Fm between the crest control (mean: 0.48, ± 0.004 SD) and handling effect (mean: 0.49, ± 0.004 SD) group [F(1, 24) = 3.37, P = 0.076, mean difference in Fv/Fm between groups = 0.04], over the duration of the experiment [F(1, 24) = 0.51, P = 0.678] or the interaction between treatment and experimental duration [F(1, 24) = 0.913, P = 0.446].
Whilst no significant differences in the yield of the flat control group were observed [F(3, 16) = 0.552, P = 0.654], thalli translocated from the crest to the flat (acclimatization group) showed significant differences in yield across days [F(3, 15) = 3.47, P = 0.043]. A Tukey post hoc test indicated that Fv/Fm was significantly lower after translocation only (i.e., day 1 vs. day 2: P = 0.030, mean day 1: 0.504 ± 0.06 SD; mean day 2: 0.356, ± 0.03 SD) (Figure 3). However, after the initial decrease in yield, L. kotschyanum appeared to acclimatize to the more variable environment as Fv/Fm returned to pre-translocation levels on days 3 and 4 (day 3 vs. day 4: P = 0.924, mean day 3: 0.435 ± 0.09 SD; mean day 4: 0.462, ± 0.08 SD).
Fv/Fm did not differ significantly between any of the treatment groups [F(3, 11) = 0.77, P = 0.534] prior to translocation and there were no significant differences in Fv/Fm between the crest control and handling effect group at any point over the 4 day experiment [F(1, 25) = 3.27, p = 0.083]; i.e. initial photosynthetic state nor handling affected Fv/Fm.
The abundance of coralline algae was significantly higher at the reef crest (mean: 15.2 per 40 m2, ± 6.06 SD) compared to the macroalgae dominated reef flat (mean: 3.4 per 40 m2, ± 1.51 SD, T = 4.23, P = 0.013) and sand dominated reef flat (sand dominated flat: mean 3.8 per 40 m2, ± 3.34 SD, T = 3.68, P = 0.006) (Figure 4A). Although not statistically significantly different in size (T = 1.948, P = 0.056), individuals on the thermally stable crest appear marginally larger (mean: 7.18 cm, ± 3.57 SD) than those to the thermally variable reef flat (mean: 5.71 cm, ± 2.15 SD) (Figure 4B).
Figure 4. (A) Mean abundance of Lithophyllum kotschyanum on the reef crest, macroalgae dominated reef flat, sand dominated reef flat (n = 5 ± StDev). Each transect covered 40 m2. *Indicates significant difference at p < 0.05. (B) Mean size of Lithophyllum kotschyanum on the reef crest and reef flat measured along the longest axis (n = 30 ± StDev).
Few studies have investigated acclimation of coralline algae to increased radiation or environments experiencing high variability (Burdett et al., 2017). Here, we demonstrate the photosynthetic response of coralline algae to increased thermal variability in a natural reef environment demonstrating the ability of coralline algae to acclimate to variable environments albeit with temporal differences. The rapid changes in ocean conditions associated with climate change will have a direct impact on coralline algae, in particular as they are sensitive to changes in irradiance, pCO2, sedimentation, desiccation, salinity and temperature (Wilson et al., 2004; Johnson et al., 2014). For example, fluctuation in pCO2 associated with ocean acidification affects the carbon chemistry of coralline algae and is likely to result in a decrease of calcification rates, although these were context dependent (Johnson et al., 2014).
The initial decrease in photosynthetic yield of L. kotschyanum after translocation was indicative of physiological stress and initial sensitivity to a change in environmental conditions. However, rapid photosynthetic acclimatization to increased thermal variability after 2 days suggests L. kotschyanum may have the capacity to cope in more thermally variable locations within a coral reef, and by proxy, with more variable conditions in the future. Indeed, as no differences in the yield of the flat control group were observed, reef flat-based environmental variation per se had little or no impact on photosynthetic efficiency for algae once acclimatized, or even adapted, to that variability at the reef flat. For coralline algae, acclimatization to environmental variation may be achieved by having intraspecific differences in physiology which can be related to the degree of shade or sun stress experienced during their life history and depending on locality (Payri et al., 2001; Wilson et al., 2004; Burdett et al., 2014). These physiological differences can include changes in pigment, protein or antioxidant production, which change at sub-diel time scales (Burdett et al., 2014). For example, gene expression analyses have provided evidence of rapid acclimatization in species of tropical coral following translocation to new environmental conditions, with changes in some genes being detected after as little as 5 days (Bay et al., 2013).
While this ability ultimately allows individuals to survive long-term in the environmentally variable reef flat and thus in generally more variable locations, more abundant and marginally larger individuals on the crest would suggest that living on the reef flat may cause energetic trade-offs where resources are re-allocated away from growth, to the physiological processes required for survival in a variable environment (Burdett et al., 2013). Other research suggests that species of coralline algae occurring in fluctuating environments exhibit lower calcification rates, indicating a trade-off to living in more variable conditions (Noisette et al., 2013), and that carbon turnover decreases with increasing temperature (Guy-Haim et al., 2016). If this is the case, then increased environmental variability and warming could result in phenological shifts, calcification and reproduction declines, population decreases, ultimately causing in local extinctions of populations of coralline algae. Alternatively, coralline algae have existed for longer (geologically) on the reef crest, hence their increase abundance and slightly larger size.
It is important to note that while we consider thermal differences between the reef crest and flat, other factors are also known to be more variable on the reef flat and may have impacted photosynthetic efficiency (e.g., Burdett et al., 2013). These include variability in pH, pCO2 and differences in light intensities, all of which can result in lower net calcification rates (Martin and Gattuso, 2009; Johnson and Carpenter, 2012; Comeau et al., 2014), increased bleaching of surface tissue (Anthony et al., 2008) and decreased abundance (Kuffner et al., 2007; Fabricius et al., 2014). Moreover, water movement restricts the distribution of coralline algae as sufficient wave action prevents sedimentation, decreasing the likelihood of burial (Wilson et al., 2004). Consequently, lower wave action on the reef flat may increase sedimentation, decreasing habitat suitability. Increased (King and Schramm, 1982) and decreased (Burdett et al., 2015) salinity negatively affect calcification rates and can initially depress photosynthetic efficiency in coralline algae. Thus, a combination of environmental factors such as biochemistry, sedimentation, salinity, and temperature may reduce photosynthetic efficiency as well as habitat suitability and explain both the short term changes in photosynthetic yield as well as the disparity in the size and abundance of free-living coralline algae between these two reef zones. While measuring a wider range of abiotic variables over the course of the experiment would have been insightful, this was not possible due to logistic restrictions (ability to measure within the sampling window). It is worth noting that on day 2 of the study, when the significant decrease in photosynthetic efficiency was recorded for the translocated algae, coincided with a high temperature difference between flat and crest (i.e., 3.5°C higher temperature measured in the reef flat). In contrast, on the following day, still with a 1.8°C difference in temperature, photosynthetic yield was higher for the translocated algae, suggesting either compensation/acclimatization to the novel regime, or alternatively some cut-off point whereby above a 1.8°C difference, algae have difficulty in maintaining high photosynthetic yield. Further investigation is needed to disentangle these effects and variables and to understand the long-term effects of temperature variability on coralline algae growth, abundance and photosynthesis efficiency. Still, temperature is known to be dominant factor in determining algae physiology and photosynthesis (Davidson, 1991), it can correlate and interact with variables such as pCO2, desiccation and irradiance (Martin and Gattuso, 2009; Martone et al., 2010; Martin et al., 2013) and it is the most frequent and easily measured of the factors at play.
The lack of significant differences between control groups both before and during the study increases our confidence that changes to photosynthetic efficiency we detected in the acclimatization group can be attributed to being exposed to greater thermal variability or higher variability of the environmental factors as discussed above. Likewise, the inclusion of a handling control group decreases the likelihood that results are due to a stress response caused by handling the algae.
Coralline algae often are low light adapted (Wilson et al., 2004), yet large diurnal fluctuations in PAR have also resulted in strategies to minimize light stress (Burdett et al., 2012; Williamson et al., 2014). By sampling twice per day we detected diurnal variation in photosynthetic efficiency, which can be attributed to the diurnal variation in PAR (Burdett et al., 2014). At times of high PAR algae experience dynamic photoinhibition (Burdett et al., 2014) resulting in lower photosynthetic yields, supporting our hypothesis that other factors, such as PAR, impact photosynthesis yield and could have impacted our results. This masks any translocation-induced stress and highlights the importance of a stratified sampling design. At low PAR levels characteristic of 0700, the algae are not expending energy on dynamic photoinhibition and the photosynthetic apparatus is operating more efficiently possibly enabling them to buffer against thermal variability, even though photosynthesis is closely linked to temperature (Davidson, 1991).
We used natural variation between reef zones to test the ability of algae to acclimatize over a short time period when translocated into a different location characterized by significant differences in environmental conditions across a coral reef. While we cannot comment on the long-term effects of increased environmental variability on coralline algae, our findings relating to differences in size and abundance, and rapid in situ acclimatization of algae to increased environmental variability, provide interesting avenues for study in the future. That is, tropical free-living coralline algae exposed to variable environments initially exhibited stress and reduced photosynthetic efficiency, but following rapid acclimatization the algae. While this stress might be due to other factors that varied between locations, including variability in sedimentation rate, dissolved oxygen, carbon or pH, we believe that the main drivers were increased thermal variability across these two locations (Burdett et al., 2013). While this indicates that coralline algae likely have the capacity to survive in more variable locations on the same reef projected for the future, their lower abundance and size suggests that fitness trade-offs may occur.
All authors designed the experiment and collected the data. LB and JF analyzed the data. LB, JF, and NK wrote the paper.
NK was in receipt of funding from the Royal Society of Edinburgh (RES 48704/1) and NERC (NE/D008727/1).
The authors declare that the research was conducted in the absence of any commercial or financial relationships that could be construed as a potential conflict of interest.
We thank iDive Dahab and Callum Farmer, Andrew Nuttall, Nally Tan and Francesca Tee for sampling support and M. El. Alwany for help with the sampling permit. We are grateful for the insightful comments provided by A. Cróquer, S. Bennet and two anonymous referees.
Anthony, K. R. N., Kline, D. I., Diaz-Pulido, G., Dove, S., and Hoegh-Guldberg, O. (2008). Ocean acidification causes bleaching and productivity loss in coral reef builders. Proc. Natl. Acad. Sci. U.S.A. 105, 17442–17446. doi: 10.1073/pnas.0804478105
Bay, L. K., Guerecheau, A., Andreakis, N., Ulstrup, K. E., and Matz, M. V. (2013). Gene expression signatures of energetic acclimatisation in the reef building coral Acropora millepora. PLoS ONE 8:e61736. doi: 10.1371/journal.pone.0061736
Berteaux, D., Réale, D., McAdam, A. G., and Boutin, S. (2004). Keeping pace with fast climate change: can arctic life count on evolution? Integr. Comp. Biol. 44, 140–151. doi: 10.1093/icb/44.2.140
BIOMAERL Team (1999). Final Report, BIOMAERL project (Coordinator: P.G. Moore, University Marine Biological Station Millport, Scotland), EC Contract No. MAS3– CT95–0020, (in 2 vols), 1:1–541, 2:542–973pp + Appendix.
Blake, C., and Maggs, C. A. (2003). Comparative growth rates and internal banding periodicity of maerl species (Corallinales, Rhodophyta) from northern Europe. Phycologia 42, 606–612. doi: 10.2216/i0031-8884-42-6-606.1
Burdett, H. L., Donohue, P. J., Hatton, A. D., Alwany, M. A., and Kamenos, N. A. (2013). Spatiotemporal variability of dimethylsulphoniopropionate on a fringing coral reef: the role of reefal carbonate chemistry and environmental variability. PLoS ONE 8:e64651. doi: 10.1371/journal.pone.0064651
Burdett, H. L., Hatton, A. D., and Kamenos, N. A. (2015). Effects of reduced salinity on the photosynthetic characteristics and intracellular DMSP concentrations of the red coralline alga, Lithothamnion glaciale. Mar. Biol. 62, 1077–1085. doi: 10.1007/s00227-015-2650-8
Burdett, H. L., Hennige, S. J., Francis, F. T. Y., and Kamenos, N. A. (2012). The photosynthetic characteristics of red coralline algae, determined using pulse amplitude modulation (PAM) fluorometry. Bot. Mar. 55, 499–509. doi: 10.1515/bot-2012-0135
Burdett, H. L., Keddie, V., Macarthur, N., McDowall, L., McLeish, J., Spielvogel, E., et al. (2014). Dynamic photoinhibition exhibited by red coralline algae in the Red Sea. BMC Plant Biol. 14:139. doi: 10.1186/1471-2229-14-139
Burdett, H., Keddie, V., Mcdowell, L., and Kamenos, N. (2017). Diurnal Photosynthetic Responses of Tropical Red Coralline Algae in the Red Sea. Coral Reefs.
Comeau, S., Carpenter, R. C., and Edmunds, P. J. (2014). Effects of irradiance on the response of the coral Acropora pulchra and the calcifying alga Hydrolithon reinboldii to temperature elevation and ocean acidification. J. Exp. Mar. Biol. Ecol. 453, 28–35. doi: 10.1016/j.jembe.2013.12.013
Davidson, I. R. (1991). Environmental effects on algal photosynthesis: temperature. J. Phycol. 27, 2–8. doi: 10.1111/j.0022-3646.1991.00002.x
Development Core Team (2014). R: A Language and Environment for Statistical Computing. Vienna: R Foundation for Statistical Computing.
Edmunds, P. J., and Gates, R. D. (2008). Acclimatization in tropical reef corals. Mar. Ecol. Prog. Ser. 361, 307–310. doi: 10.3354/meps07556
Eladawy, A., Nadaoka, K., Negm, A., Saavedra, O. C., and Hanafy, M. (2015). Assessment of long term thermal stress on egyptian coral reefs based on remotely sensed sea surface temperature data. Intern. J. Environ. Sci. Dev. 6:938. doi: 10.7763/IJESD.2015.V6.726
Fabricius, K. E., De'ath, G., Noonan, S., and Uthicke, S. (2014). Ecological effects of ocean acidification and habitat complexity on reef-associated macroinvertebrate communities. Proc. R. Soc. B 281, 2013–2479. doi: 10.1098/rspb.2013.2479
Fabricius, K. E., Kluibenschedl, A., Harrington, L., Noonan, S., and De'ath, G. (2015). In situ changes of tropical crustose coralline algae along carbon dioxide gradients. Sci. Rep. 5:9537 doi: 10.1038/srep09537
Foster, M. S. (2002). Rhodoliths: between rocks and soft places. J. Phycol. 37, 659–667. doi: 10.1046/j.1529-8817.2001.00195.x
Guy-Haim, T., Silverman, J., Raddatz, S., Wahl, M., Israel, A., and Rilov, G. (2016). The carbon turnover response to thermal stress of a dominant coralline alga on the fast warming Levant coast. Limnol. Oceanogr. 61, 1120–1133. doi: 10.1002/lno.10279
Hochachka, P., and Somero, G. (2002). Biochemical Adaptation: Mechanism and Process in Physiological Evolution. New York, NY, Oxford University Press.
Hoegh-Guldberg, O., and Bruno, J. F. (2010). The impact of climate change on the world's marine ecosystems. Science 328, 1523–1528. doi: 10.1126/science.1189930
Hofmann, G. E., and Todgham, A. E. (2010). Living in the now: physiological mechanisms to tolerate a rapidly changing environment. Annu. Rev. Physiol. 72, 127–145. doi: 10.1146/annurev-physiol-021909-135900
Johnson, M. D., and Carpenter, R. C. (2012). Ocean acidification and warming decrease calcification in the crustose coralline alga Hydrolithon onkodes and increase susceptibility to grazing. J. Exp. Mar. Biol. Ecol. 434, 94–101. doi: 10.1016/j.jembe.2012.08.005
Johnson, M. D., Moriarty, V. W., and Carpenter, R. C. (2014). Acclimatization of the crustose coralline alga porolithon onkodes to variable pCO2. PLoS ONE 9:e87678. doi: 10.1371/journal.pone.0087678
Kamenos, N., Moore, P., and Hall-Spencer, J. (2004). Nursery-area function of maerl grounds for juvenile queen scallops Aequipecten opercularis and other invertebrates. Mar. Ecol. Prog. Ser. 274, 183–189. doi: 10.3354/meps274183
King, R., and Schramm, W. (1982). Calcification in the maerl coralline alga Phymatolithon calcareum: effects of salinity and temperature. Mar. Biol. 70, 197–204. doi: 10.1007/BF00397685
Kingsolver, J. G., Hoekstra, H. E., Hoekstra, J. M., Berrigan, D., Vignieri, S. N., Hill, C. E., et al. (2001). The strength of phenotypic selection in natural populations. Am. Nat. 157, 245–261. doi: 10.1086/319193
Kuffner, I. B., Andersson, A. J., Jokiel, P. L., Rodgers, K. S., and Mackenzie, F. T. (2007). Decreased abundance of crustose coralline algae due to ocean acidification. Nat. Geosci. 1, 114–117. doi: 10.1038/ngeo100
Lasker, H. R., and Coffroth, M. A. (1999). Responses of clonal reef taxa to environmental change. Am. Zool. 39, 92–103. doi: 10.1093/icb/39.1.92
Martin, S., Cohu, S., Vignot, C., Zimmerman, G., and Gattuso, J. P. (2013). One-year experiment on the physiological response of the Mediterranean crustose coralline alga, Lithophyllum cabiochae, to elevated pCO2 and temperature. Ecol. Evol. 3, 676–693. doi: 10.1002/ece3.475
Martin, S., and Gattuso, J. P. (2009). Response of Mediterranean coralline algae to ocean acidification and elevated temperature, Glob. Chang. Biol. 15, 2089–2100. doi: 10.1111/j.1365-2486.2009.01874.x
Martone, P. T., Alyono, M., and Stites, S. (2010). Bleaching of an intertidal coralline alga: untangling the effects of light, temperature, and desiccation. Mar. Ecol. Prog. Ser. 416, 57–67. doi: 10.3354/meps08782
McCoy, S. J., and Kamenos, N. A. (2015). Coralline algae (Rhodophyta) in a changing world: integrating ecological, physiological, and geochemical responses to global change. J. Phycol. 51, 6–25. doi: 10.1111/jpy.12262
Morse, D. E. (1996). Flypapers for coral and other planktonic larvae. Bioscience 46, 254–262. doi: 10.2307/1312832
Noisette, F., Egilsdottir, H., Davoult, D., and Martin, S. (2013). Physiological responses of three temperate coralline algae from contrasting habitats to near-future ocean acidification. J. Exp. Mar. Biol. Ecol. 448, 179–187. doi: 10.1016/j.jembe.2013.07.006
Payri, C. E., Maritorena, S., Bizeau, C., and Rodière, M. (2001). Photoacclimation in the tropical coralline alga Hydrolithon onkodes (Rhodophyta, Corallinaceae) from a French Polynesian reef. J. Phycol. 37, 223–234. doi: 10.1046/j.1529-8817.2001.037002223.x
Pulliam, H. R. (1988). Sources, sinks, and population regulation. Am. Nat. 132, 652–661. doi: 10.1086/284880
Reynier, M. V., Tamega, F. T. S., Daflon, S. D. A., Santos, M. A. B., Coutinho, R., and Figueiredo, M. A. O. (2015). Long- and short-term effects of smothering and burial by drill cuttings on calcareous algae in a static-renewal test. Environ. Toxicol. Chem. 34, 1572–1577. doi: 10.1002/etc.2938
Sheppard, C. R. C., Davy, S. K., and Pilling, G. M. (2009). The Biology of Coral Reefs. Oxford: Oxford University Press.
Siboni, N., Abrego, D., Evenhuis, C., Logan, M., and Motti, C. A. (2015). Adaptation to local thermal regimes by crustose coralline algae does not affect rates of recruitment in coral larvae. Coral Reefs 34, 1243–1253. doi: 10.1007/s00338-015-1346-3
Somero, G. (2010). The physiology of climate change: how potentials for acclimatization and genetic adaptation will determine “winners” and “losers”. J. Exp. Biol. 213, 912–920. doi: 10.1242/jeb.037473
Steller, D. L., Riosmena-Rodríguez, R., Foster, M., and Roberts, C. (2003). Rhodolith bed diversity in the gulf of California: the importance of rhodolith structure and consequences of disturbance. Aquat. Conserv. 13, S5–S20. doi: 10.1002/aqc.564
Stockwell, C. A., Hendry, A. P., and Kinnison, M. T. (2003). Contemporary evolution meets conservation biology. Trends Ecol. Evol. 18, 94–101. doi: 10.1016/S0169-5347(02)00044-7
Tierney, P. W., and Johnson, M. E. (2011). Stabilization role of crustose coralline algae during Late Pleistocene reef development on Isla Cerralvo, Baja California Sur (Mexico). J. Coast. Res. 28, 244–254. doi: 10.2112/JCOASTRES-D-11T-00009.1
Williamson, C. J., Najorka, J., Perkins, R., Yallop, M. L., and Brodie, J. (2014). Skeletal mineralogy of geniculate corallines: providing context for climate change and ocean acidification research. Mar. Ecol. Prog. Ser. 513, 71–84. doi: 10.3354/meps10929
Keywords: climate change, adaptation, marine calcifiers, environmental variability, acclimatization, coralline algae
Citation: Bach LL, Freer JJ and Kamenos NA (2017) In situ Response of Tropical Coralline Algae to a Novel Thermal Regime. Front. Mar. Sci. 4:212. doi: 10.3389/fmars.2017.00212
Received: 25 February 2016; Accepted: 16 June 2017;
Published: 30 June 2017.
Edited by:
Carlos M. Duarte, King Abdullah University of Science and Technology, Saudi ArabiaReviewed by:
Aldo Cróquer, Simón Bolívar University, VenezuelaCopyright © 2017 Bach, Freer and Kamenos. This is an open-access article distributed under the terms of the Creative Commons Attribution License (CC BY). The use, distribution or reproduction in other forums is permitted, provided the original author(s) or licensor are credited and that the original publication in this journal is cited, in accordance with accepted academic practice. No use, distribution or reproduction is permitted which does not comply with these terms.
*Correspondence: Nicholas A. Kamenos, bmljay5rYW1lbm9zQGdsYXNnb3cuYWMudWs=
Disclaimer: All claims expressed in this article are solely those of the authors and do not necessarily represent those of their affiliated organizations, or those of the publisher, the editors and the reviewers. Any product that may be evaluated in this article or claim that may be made by its manufacturer is not guaranteed or endorsed by the publisher.
Research integrity at Frontiers
Learn more about the work of our research integrity team to safeguard the quality of each article we publish.