- Research Division 2: Marine Biogeochemistry, GEOMAR Helmholtz Centre for Ocean Research Kiel, Kiel, Germany
Cold-water corals are important bioengineers that provide structural habitat for a diverse species community. About 70% of the presently known scleractinian cold-water corals are expected to be exposed to corrosive waters by the end of this century due to ocean acidification. At the same time, the corals will experience a steady warming of their environment. Studies on the sensitivity of cold-water corals to climate change mainly concentrated on single stressors in short-term incubation approaches, thus not accounting for possible long-term acclimatisation and the interactive effects of multiple stressors. Besides, preceding studies did not test for possible compensatory effects of a change in food availability. In this study a multifactorial long-term experiment (6 months) was conducted with end-of-the-century scenarios of elevated pCO2 and temperature levels in order to examine the acclimatisation potential of the cosmopolitan cold-water coral Lophelia pertusa to future climate change related threats. For the first time multiple ocean change impacts including the role of the nutritional status were tested on L. pertusa with regard to growth, “fitness,” and survival. Our results show that while L. pertusa is capable of calcifying under elevated CO2 and temperature, its condition (fitness) is more strongly influenced by food availability rather than changes in seawater chemistry. Whereas growth rates increased at elevated temperature (+4°C), they decreased under elevated CO2 concentrations (~800 μatm). No difference in net growth was detected when corals were exposed to the combination of increased CO2 and temperature compared to ambient conditions. A 10-fold higher food supply stimulated growth under elevated temperature, which was not observed in the combined treatment. This indicates that increased food supply does not compensate for adverse effects of ocean acidification and underlines the importance of considering the nutritional status in studies investigating organism responses under environmental changes.
Introduction
The cold-water coral Lophelia pertusa is one of six widespread azooxanthellate scleractinian coral species that are known to be capable of building large three-dimensional reef frameworks (Roberts et al., 2009). This ecologically important species is found throughout the world's oceans and forms structural habitat for a variety of fishes and other invertebrates. Thus, L. pertusa bioherms are important biodiversity hotspots, which host more than 2,700 species worldwide that live in association with these reef structures (Roberts and Cairns, 2014).
Up until now about 90% of all scleractinian bioherm-forming cold-water corals live in supersaturated waters with respect to aragonite (Davies and Guinotte, 2011), but ~70% of the presently known cold-water corals are expected to be exposed to calcium carbonate undersaturated waters by the end of the century due to ocean acidification (Guinotte et al., 2006). Ocean acidification—also called “the evil twin of global warming”—is induced by anthropogenic carbon dioxide (CO2) emissions that leads to lowered seawater pH and decreasing carbonate ion concentrations, which in turn results in a diminished saturation state of the oceans with respect to calcium carbonate (CaCO3) (e.g., Caldeira and Wickett, 2003; Feely et al., 2004). As a consequence, calcifying organisms that build their calcareous skeletons and protective shells of calcite or aragonite (the two most common naturally occurring CaCO3 polymorphs) such as scleractinian corals might be particularly affected (i.e., Gattuso et al., 1998; Roberts et al., 2006; Maier et al., 2009; Form and Riebesell, 2012). The oceans—as the largest reservoir of reactive carbon dioxide on Earth—take up approximately one quarter to one third of the current anthropogenic CO2 emissions (e.g., Feely et al., 2004; Sabine et al., 2004). This has already caused a decrease in seawater pH by 0.1 units compared to the pre-industrial level (i.e., Haugan and Drange, 1996; Feely et al., 2004), which corresponds to a 30% increase in ocean acidity (Feely et al., 2004; Riebesell et al., 2010). Since cold-water corals commonly occur at high latitudes and/or deep waters, they are thought to be among the first being exposed to low-pH conditions because of the better solubility of CO2 in colder waters (Orr et al., 2005). Concomitant with ocean acidification, rising temperature even in the deeper water layers plays a role in upcoming environmental pressures (Barnett et al., 2005). Climate change has already caused an increase of global mean sea surface temperature of 0.56–0.92°C in the last 100 years and a further increase of ~3–4°C is projected until the end of this century (IPCC, 2014).
Experimental climate change related studies on cold-water corals conducted so far involved mainly unifactorial manipulations and short-term incubation approaches (i.e., Dodds et al., 2007; Maier et al., 2009, 2013a,b; Hennige et al., 2014; Lunden et al., 2014). Form and Riebesell (2012) highlighted the importance of long-term perturbations of corals in ocean change research as acclimatisation of these slow-growing animals is expected to be relatively slow. While short-term studies can be biased by “shock” reactions, long-term incubation studies of several weeks to months have shown that L. pertusa is able to acclimatise to ocean acidification in single stressor approaches (Form and Riebesell, 2012; Maier et al., 2013a,b; Movilla et al., 2014a). As ocean acidification occurs in parallel with increasing temperature, and because those are the predominant environmental changes marine ecosystems will be subjected to in the coming decades, studies examining the combined effects of these two stressors are urgently needed.
So far, relatively few studies have investigated interactive effects of ocean acidification and warming on marine invertebrates. Responses range from synergistic effects, i.e., one driver amplifying the effect of the other (e.g., Wood et al., 2010; Rodolfo-Metalpa et al., 2011), to antagonistic effects, i.e., one driver dampening the effect of the other (Melatunan et al., 2011), for different physiological processes. Warm-water coral reefs are highly dynamic ecosystems and as such have a relatively high potential of recovery, though cumulative anthropogenic pressures such as climate change lead to chronic threats and increasing coral cover degradation that is often not being reversed due to too frequent and/or multiple perturbations (e.g., Hughes et al., 2003; Spalding and Brown, 2015). Increasing seawater temperature is known to cause coral “bleaching,” meaning the loss of symbiotic algae (zooxanthellae) that most of the warm-water corals exhibit. Mass bleaching events have already led to phase shifts towards macro-algal dominance in some areas (i.e., Graham et al., 2015). Ocean acidification comprises another chronic threat that has led to reduced growth rates and dissolution in coral reefs (i.e., Pandolfi et al., 2011). With regard to cold-water corals, Hennige et al. (2015) reported calcification and respiration rates of L. pertusa from the Mingulay Reef Complex west of Scotland after long-term exposure to combined end-of-the-century temperature and pCO2 scenarios. Their results showed that even under the combined impact corals were able to calcify with only a slightly decreasing trend in calcification rates at undersaturated conditions. Moreover, no significant changes in respiration rates were observed. At the same time, structural disorganisation of the aragonite crystals of the skeleton was observed in newly developed corallites at undersaturated conditions (Hennige et al., 2015).
To date, it is unknown what other metabolic costs might emerge from acclimatisation to ocean change. However, it has already been suspected that certain processes will need additional energy requirements to maintain levels of physiological performance under ocean acidification. McCulloch et al. (2012b), for instance, suggested that the pH homeostasis at the site of calcification might be more costly in the future with an additional 10% energy demand for pH up-regulation to sustain calcification rates under elevated CO2 concentrations equivalent to a decrease in seawater pH by 0.1 units. It is not clear yet whether this additional energy demand can be covered by increased food uptake in L. pertusa, as was observed in tropical coral species, where increased food consumption compensated for negative effects of ocean acidification (i.e., Holocomb et al., 2010; Allemand et al., 2011; Edmunds, 2011). Moreover, Naumann et al. (2014) demonstrated that respiration and calcification rates were significantly reduced in the solitary cold-water coral species Desmophyllum dianthus after exclusion of zooplankton supply for up to 3 weeks, indicating that regular feeding is needed for providing the energy to sustain respiratory metabolism and growth rates.
In the present study, therefore, two important physiological functions of Lophelia pertusa were examined with regards to ocean acidification and warming—growth, and “fitness” in terms of the general condition of the animals—at two different feeding regimes in a 6 months long laboratory experiment in order to expand our knowledge of how this important cold-water coral species might cope with an environment expected by 2100. We aimed at identifying how differently L. pertusa reacts towards multiple climate change stressors when fed with a higher amount of zooplankton food compared to 10-fold lower food availability. It was suggested that calcification rates might be maintained under an acidified environment via mobilising lipid reserves from the coral's tissue (Maier et al., 2013b; Hennige et al., 2014), but this source of stored energy is bound to decline on longer time scales. Furthermore, elevated temperatures might lead to increased metabolic rates (Dodds et al., 2007) that require additional energetic input.
Together with a potential reduction in plankton production in the surface (i.e., Steinacher et al., 2010) accompanied by a reduced export flux of particulate organic carbon to the deep sea (Jones et al., 2014), the additional food items that are needed to sustain the nutritional requirements under ocean warming might be insufficient. Moreover, cold-water corals are likely benefiting from the vertical migration of mesopelagic zooplankton (Roberts et al., 2009). With potential longer stratification periods of the water column due to increasing temperatures, this may affect the diurnal vertical plankton migrations and may, thus, possibly lead to occasionally lower prey availability. In addition, freshwater input to the higher latitudes of the North Atlantic is expected to increase as a result of increasing temperatures, which may lead to a slowdown in water circulation and reduced upwelling (Curry et al., 2003). Since deep sea corals are sessile organisms, they depend on currents that transport food particles to them. Altogether, assuming that higher stratification restricts food particles to reach the cold-water corals and potentially less currents, cold-water corals might be greatly impacted not only by direct, but also by indirect effects resulting from climate change. In view of the ecological importance of cold-water coral reefs as bioengineers and as habitat builders (e.g., Freiwald et al., 2004), long-term effects of ocean acidification and warming on growth rates (net calcification rates), fitness (RNA/DNA ratios) and survival (mortality rates) were assessed in order to address the following research questions:
(1) Can L. pertusa maintain calcification rates on longer time scales when exposed to ocean acidification and elevated temperature and what are the interacting effects of these two drivers?
(2) Will the fitness (activity or general condition) and the survival of L. pertusa be affected after long-term exposure to end-of-the-century ocean change conditions?
(3) Does elevated food availability have an influence on the physiological responses of L. pertusa towards elevated pCO2 and temperature levels and does an increased food input counteract potential negative effects of climate change?
Materials and Methods
Sampling of the Cold-Water Coral Lophelia pertusa
Specimens of L. pertusa used for this study were collected during a research cruise with the RV Poseidon in September 2011 from an in-shore reef in the outer Trondheim-Fjord near the Norwegian island Nord-Leksa (63°36.4′N, 09°22.7′E). Healthy colonies were carefully collected from spatially distinct locations of the reef by means of the manned submersible JAGO at depths between 145 and 220 m. With its minimal invasive manipulator arm, JAGO can selectively collect living coral samples while avoiding damage to the reefs. Corals were transported to the laboratory and cultivated in a closed recirculating system of 1,700 litres in a temperature-controlled room for 1 year before the start of the experiment. This ensured total recovery of the corals from disruption caused by the collection and full acclimatisation to laboratory conditions, allowing the coral's metabolism to adjust to a similar nutritional level in all specimens. Temperature and salinity were kept constant at 7.7°C and 36.1, respectively, to meet the ambient conditions the corals experienced at the sampling site. Corals were fed twice a week with freshly hatched Artemia franciscana nauplii (Premium, Sanders). The water in the system was regularly partially exchanged with natural North Sea water. Two months prior to the start of the experiment, colonies were carefully fragmented into smaller branches of about 100 polyps. The branch size was chosen to make sure that all polyp generations were included in the branches, though a large proportion of the upper parts of the colonies were used containing a high number of relatively young polyps. The corals were buoyant weighed after fragmentation and only coral branches within a weight range of 50 ± 30 g and which showed a comparable growth rate of 0.01 ± 0.007% d−1 were chosen for the experiment. The fragmented coral branches were randomly assigned to the experimental aquaria and were kept for 2 months in the new environment before the treatment conditions were adjusted.
Experimental Setup
Two months after the final fragmentation and distribution to the experimental aquaria the different treatment conditions were adjusted. The experimental design is a three-factor design with fixed end of the century scenarios of CO2 and temperature, as well as food availability in two defined feeding regimes retained over the whole course of the experiment. Two different levels of CO2 concentration and temperature were adjusted in four steps over a 3 week time period to the desired levels described in the following. Each of the two factors CO2 and temperature was examined as single stressor as well as in combination, resulting in eight different treatments with four replicates each. Two pCO2 levels, one ambient (~400 μatm) and one elevated (~800 μatm) reflecting the projected CO2 concentration in 2100 (IPCC, 2014 after the RCP6.0 CO2 emission scenario), and two temperature levels, one at ambient reef temperature of 8°C and one elevated (12°C), were adjusted in four combinations (8°C × 400 μatm, 8°C × 800 μatm, 12°C × 400 μatm, and 12°C × 800 μatm). The four combinations were each subdivided into a “low” fed group of four replicates fed with 5 mL of live A. franciscana nauplii in a homogenous seawater solution twice a week and a “high” fed group fed with 50 mL. The two volumes of the dense Artemia solutions of 5 and 50 mL corresponded to ~0.305 nauplii mL−1 in the low food and 3.05 nauplii mL−1 in the high food treatments, respectively.
In total, 32 independent 55 L aquaria were equipped with a streaming pump (Hydor® KORALIA Nano, flow rate 900 L/h) for water circulation, a biological air filter (Tropical® Delfin SINGLE), an internal protein skimmer (Aqua Medic® miniflotor 200), a heater (Jäger, 75 W) regulated via a temperature regulator (Hobby® Biotherm Pro), and a pH probe connected to an aquaristic computer (IKS® aquastar) for permanent control of pH. A pre-mixed CO2-air-mixture provided through the gas mixing system KICO2 (Kiel CO2 manipulation experimental facility) or just pressured air—depending on the treatment—were bubbled into the aquaria through air stones of the skimmer and through the filter sponge. The corals were kept in complete darkness throughout the experiment simulating the natural conditions. Routine handling and regular measurements were conducted with head lamps. The aquaria systems were checked twice a week for pH, temperature, and salinity by means of a portable pH meter (WTW® pH 3310) equipped with a Sentix-81 pH electrode and a conductivity handheld (WTW® Cond 3210) with a TetraCon® 325 probe, respectively.
Determination of Growth Rates
One month after adjustment of the different treatment conditions, corals were weighed under water the first time and afterwards at monthly intervals for 6 months. Growth rates were determined applying the buoyant weighing technique for skeletal weight of single coral branches developed by Davies (1989) on the basis of descriptions by Jokiel et al. (1978). The measurements took place in a separate aquarium with a high precision analytical balance (Sartorius CPA225D, readability: 0.1 mg) placed on top of it. Corals were transferred separately to the weighing aquarium and weighed under water by being hooked to a below-balance weighing facility. Corals were permanently equipped with a Nylon thread to minimise handling effects through touches of the polyps. The water conditions in the weighing aquarium were adjusted to the specific treatment conditions beforehand. Each coral was weighed at least twice to a precision of ±1 mg buoyant weight. The growth rates were normalised to weight gain per day as a percentage of the initial weight of the coral (G % d−1) using the following equation:
where G = growth rates in % per day, xt2 = mass (mg dry weight) at the end of a measurement interval, xt1 = mass (mg dry weight) at the beginning of a measurement interval, t2 − t1 = number of days between measurements.
Determination of RNA/DNA Ratios (Fitness)
The RNA/DNA ratio is an index of protein synthetic capacity, as the amount of RNA that is actively being transcribed in a cell is measured in relation to the constant amount of DNA. Therefore, it reflects an organism's metabolic activity and can thus be used as an indicator of “health” or “fitness.” For fitness analyses via RNA/DNA ratios single polyps of L. pertusa were preserved by being fixed in the RNA stabilisation reagent RNAlater® (Qiagen). Three polyps from each of the 32 coral branches were preserved in RNAlater® and frozen at—40°C after 1 day of being stored at incubation temperature to allow the reagent to diffuse into the tissue. RNA/DNA ratios were analysed via fluorometric RNA/DNA measurements according to the methods described for nucleic acid extraction of fish larvae by Clemmesen (1993). The preserved polyps were thawed temporarily slowly on ice for the isolation of the tissue of the single polyps prior to the measurements. In order to separate the coral's tissue from the carbonate skeleton, polyps were rinsed with an airbrush gun filled with cold natural seawater into 1.5 mL safe-lock reaction vials (SafeSeal, SARSTEDT). The often very tight inner epithelium was removed from the calyx by means of tweezers. Care had to be taken that no calcium carbonate particles were added to the vials as this would lead to high auto fluorescence signals during the measurement. During the entire sample preparation procedure, all samples were kept on ice at all times in order to keep the temperature sensitive RNA stable. Immediately after airbrushing, samples were refrozen at −80°C for at least half an hour and freeze dried (Alpha 1–4, Christ) for 12 h at −51°C and a consistent vacuum pressure of 0.1 mbar. After freeze drying the samples were weighed again and homogenized with glass beads of different sizes (Ø 0.2–2.0 mm) in 500 μL of 0.01% Tris EDTA-SDS buffer (short: TE-SDS) with a shaking mill (MM2, Retsch) to solubilise the tissues and extract the nucleic acids. Homogenates were then centrifuged in a cooling centrifuge (3–18 K, Sigma) for 8 min at 1°C and 6,000 rpm (corresponding to 3,830 g). The supernatant was analysed fluorometrically with regard to nucleic acids in a microliter fluorescence reader using ethidium bromide as the fluorescent dye. As the coral tissue exhibits relatively high auto fluorescence signals, the supernatant of all samples was pre-diluted 1:10 with TE-SDS buffer prior to analysis and diluted again with the appropriate dilution factor after an initial test measurement.
Determination of Mortality
To check for mortality, pictures were taken from all replicates at the end of the experiment and all living and dead polyps were counted from the top view shot. Living polyps were marked with a green dot in an image editing programme (GNU Image Manipulation Program 2.8.8) and dead polyps or empty calyces with a red dot. With ImageJ (1.47v) all dots in the pictures were counted and the percentage of dead polyps was computed.
Carbonate Chemistry
The carbonate system was recorded monthly and water samples for total alkalinity (TA) measurements and dissolved inorganic carbon (DIC) analyses were taken in the same week as the buoyancy weighing took place. TA and DIC samples were taken as duplicates from the middle of the aquarium, poisoned with mercuric chloride, and stored as recommended by Dickson et al. (2007). TA was analysed by means of a potentiometric open-cell titration procedure according to Dickson et al. (2003) with an automatic titrator (Titrino 862 Compact Titrosampler, Metrohm). DIC was analysed via infrared detection of CO2 using an automated infra-red inorganic carbon analyser (AIRICA with LI-COR 7000, Marianda). The device repeats the measurement of a single sample four times and calculates the mean and coefficient of variation (CV ≤ 1.8 μmol ). TA and DIC were calculated accounting for the salinity of the samples and corrected against Certified Reference Materials from A. G. Dickson (Scripps Institution of Oceanography). For calculations of pH (total scale), pCO2, bicarbonate ions (), carbonate ions (), and aragonite saturation (ΩAr), the carbonate chemistry calculation software CO2SYS (v2.1) by Pierrot et al. (2006) based on the code developed by Lewis and Wallace (1998) was applied using the thermodynamic constants of Mehrbach et al. (1973), refitted by Dickson and Millero (1987) on the total scale.
Statistical Analysis
Statistical analyses were performed using the statistic graph and data analysis programme SigmaPlot© Version 12.0 (Systat Software Inc.). Data are given as mean ± standard deviation (SD). A Three-Way Analysis of Variance (ANOVA) was performed for significance testing between the four different treatment groups for growth and mortality rates. To test for differences of RNA/DNA ratios between treatment groups, a Three-Way ANOVA with Holm-Sidak method for normality testing and pairwise multiple comparison procedures was applied.
Results
Temperature and Carbonate Chemistry
The adjusted treatment conditions in terms of temperature und pCO2 were kept constant over the course of the experiment with an average of 7.9 ± 0.07°C for the ambient temperature treatments and 11.9 ± 0.05°C for the elevated temperature treatments as well as 426 ± 55 μatm (equivalent to a pH of ~7.926 ± 0.046) for the ambient pCO2treatments and 842 ± 138 μatm (pH ≈ 7.712 ± 0.064) for the elevated pCO2 treatments (Table 1).
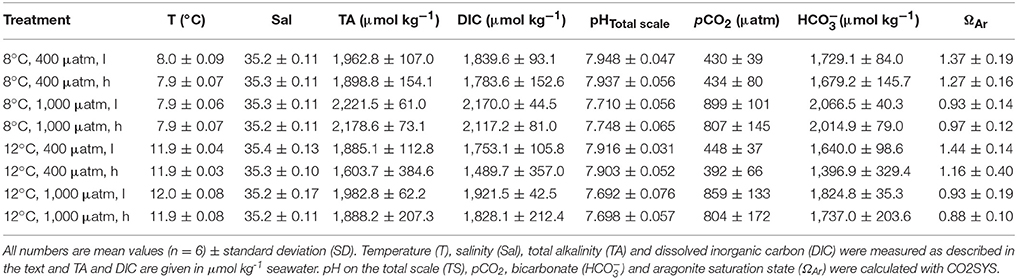
Table 1. Carbonate system parameters integrated and averaged over all measurement months for each treatment (l and h indicating “low” and “high” food treatments).
Growth Rates
Growth rates averaged over all treatments and measurement intervals ranged from −0.003 to 0.029% d−1 (Table 2). Low net calcification was attained in both “high CO2” treatments (800 μatm) at ambient temperature of 8°C (see Figure 1A). In the “high food” treatment at 800 μatm, growth rates averaged 0.001 ± 0.003% d−1, whereas the mean growth in the low fed treatment was 0.000 ± 0.003% d−1. The highest net calcification was achieved under high temperature and ambient carbon dioxide conditions (12°C, 400 μatm) (Figure 1B). The “high food” treatment at elevated temperature and ambient pCO2 had the highest growth rates altogether with a mean of 0.017 ± 0.006% d−1. Hence, under elevated temperature higher food supply led to 70% increased growth rates compared to the low fed group under the same environmental conditions (0.010 ± 0.004% d−1). At 8°C growth rates were not affected by a higher food quantity (Table 2, Figure 1A). The combination of “high temperature” and “high CO2” yielded an antagonistic effect on growth rates, with net calcification rates similar to ambient conditions. Mean rates of the “low” fed combined treatment were 0.007 ± 0.006% d−1 compared to 0.006 ± 0.004% d−1 in the “low” fed ambient treatment, whereas in the “high food” combined treatment calcification rates were slightly increased with 0.009 ± 0.003% d−1 compared to the “low” fed treatment under the same conditions and to 0.006 ± 0.005% d−1 in the “high” fed ambient treatment. The mean value of the “low” fed combined treatment is based on only three replicates instead of four, as one replicate was lost within the first 2 months of the experiment due to a technical failure in the corresponding aquarium.
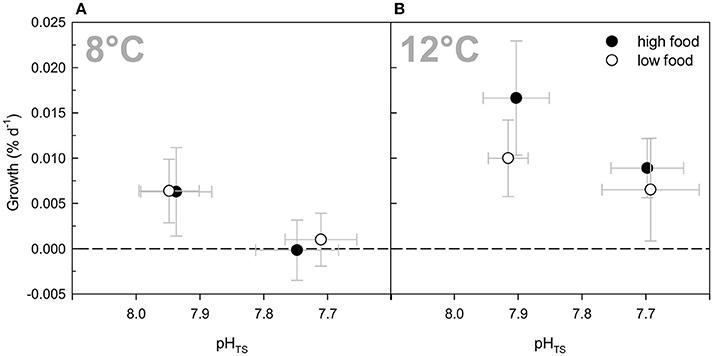
Figure 1. Growth rates (in % per day) of Lophelia pertusa integrated over all measurement intervals of the 6 months long incubation experiment. (A) Growth rates under ambient temperature (8°C) and ambient vs. elevated pCO2 levels as a function of pH on a total scale (pHTS). (B) Growth rates under elevated temperature (12°C) and ambient vs. elevated pCO2 conditions. Black symbols denote the high fed groups, white symbols the low fed groups of each combination of pCO2 and temperature. Data represent mean values ± SD.
Considering the observed low rates, standard deviations were relatively high (Figure 1; Table 2). Therefore, no statistically significant differences were obtained between the single treatments compared to the control (ambient conditions of 8°C and 400 μatm), nor between the different feeding regimes or an interaction between any of the factors. However, an overall statistically significant difference between the mean values among the two temperature levels (P = 0.002) and for the two CO2 levels (P = 0.008) was found taking the effects of the respective other two factors into account (Three-Way ANOVA and Holm-Sidak method for pairwise multiple comparisons between all treatment groups).
Comparing single measurement intervals from month to month revealed even under ambient conditions quite variable growth rates. Thus, no constant growth pattern was seen over time and a general decline of growth rates towards the end of the experiment was observed in all treatments, especially in the “high CO2” treatments, which is probably caused by cultivation effects due to a decrease in alkalinity. In the “high temperature” treatment, a strong increase in growth was observed during the first months of the experiment, which is also indicated for the combined treatment.
Towards the end of the experiment, structural deformations were observed in all manipulated treatments, particularly in the combined elevated CO2 and temperature treatments. Extensions of the calyces grew in the direction of the centre of the calyx (Figure 2A, yellow arrows) and started to grow “upwards” again in the usual growth direction of a polyp as a thinner and more fragile corallite on top of an already grown polyp (Figures 2A,B, red arrows). Polyps growing their calyces inward towards the calyx centre were observed in the elevated pCO2 treatments at “low food” and “high food” conditions in three of four replicates in both cases. Under elevated temperature and “low food,” one replicate showed already newly built “on top” calyces, whereas one replicate in the “low food” treatment at elevated temperature as well as three replicates of the “high food” group in this treatment revealed growth towards the centre of the calyx, but no “on top” calyces. Under combined elevated pCO2 and temperature, all replicates showed structural deformations except for one replicate in the “high food” group. Hence, in this treatment the degree of morphological alterations was most pronounced and the highest number of newly formed and thinner “on top” calyces was observed.
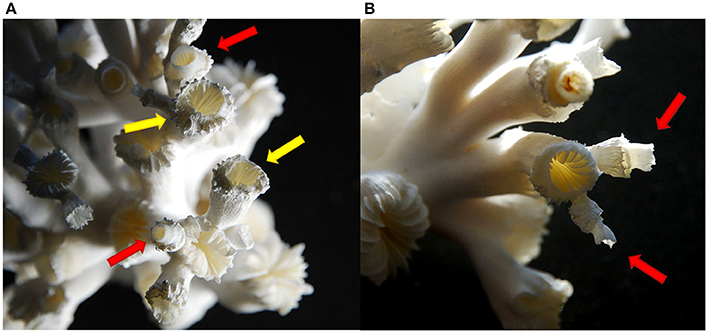
Figure 2. Structural deformations of the corallites of a coral from the combined elevated pCO2 and temperature treatment. Arrows indicate polyps of different stages in structural changes from growing towards the centre of the calyx (yellow arrows) (A) to thin newly grown calices on top of a polyp (red arrows) (A,B).
Fitness (RNA/DNA Ratios)
The RNA/DNA ratios in the present experiment ranged from 0.76 to 1.84 on average over all treatments (Table 2). In all temperature and CO2 combinations except for the combined treatments of both factors, the RNA/DNA ratio was higher in the “high food” treatments (Figure 3). This was statistically significant for the elevated pCO2 treatment that had a 40% higher ratio than the “low food” treatment (P = 0.015) as well as for the “high temperature” treatment with the “high food” treatment ratio being more than twice as high as the “low food” group RNA/DNA ratio (P = 0.009). No consistent pCO2 effect or temperature effect as a single factor observation over all treatments was found, whereas a statistically significant interaction between all three factors (temperature, CO2, and food) was identified (P = 0.004, Three-Way ANOVA). A pairwise multiple comparison test following the Holm-Sidak post-hoc procedure revealed a significant interaction of temperature and CO2 within the high food treatments: At an ambient pCO2 level a statistically significant difference in the mean values between the two temperature levels among the “high food” groups was found (P ≤ 0.001). Moreover, the difference in mean values between the different CO2 levels in the high temperature treatments and the “high food” groups is greater than would be expected by chance (P ≤ 0.001). Comparing RNA/DNA ratios with growth rates revealed a statistically significant correlation between RNA/DNA ratios and growth rates (Pearson Product Moment Correlation, P = 0.0196). The correlation did not show a strong significant relationship, which is explainable by the fact that the correlation is not based on the individual polyp growth rate of the measured polyps for “fitness” analyses, but on the whole branch growth rate averaged over the entire duration of the experiment.
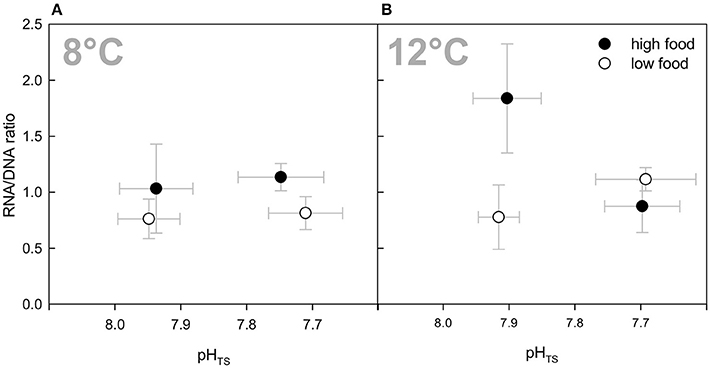
Figure 3. RNA/DNA ratios as an indication of the corals' overall condition (“fitness”) at the end of the 6-months incubation. Data are plotted over the integrated carbonate system over the entire experiment as in Figure 1 for comparability reasons. Black symbols denote the high fed groups, white symbols the low fed groups of each combination of pCO2 and temperature. Data represent mean values ± SD.
Mortality
In general, mortality was relatively low with less than 8% for all branches on average over the entire duration of the experiment (Table 2). No statistically significant treatment effects were detected for the three factors (Three-Way ANOVA) or compared to the ambient treatment groups. Nevertheless, there were tendencies towards slightly higher mortality rates in the “high food” treatments (about 5–7%), except for the elevated temperature treatment (Figure 4). The highest mortality occurred in both high food groups of the elevated pCO2 treatments with an average percentage of died polyps of about 13%. However, in case of the combined treatment, the increased mortality was caused by one replicate of that treatment that lost 36.1% of its polyps (to a not known reason). Excluding this replicate from the average percentage would result in ~4.6 ± 1.8% mortality, which is less than the mortality of the “low food” group of the combined treatment.
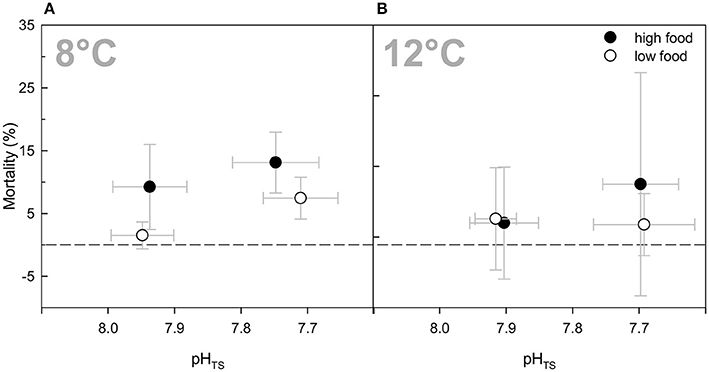
Figure 4. Mortality rates (%) of Lophelia pertusa at the end of the experiment as percentage of dead polyps to live polyps of a branch. Data are plotted over the integrated carbonate system over the entire experiment as in Figure 1 for comparability reasons. Black symbols denote the high fed groups, white symbols the low fed groups of each combination of pCO2 and temperature. Data represent mean values ± SD.
Discussion
Growth Rates
The results of this study revealed treatment-specific responses of Lophelia pertusa exposed to elevated temperature and CO2 as single stressors or in combination and dependent on different food supply. Under elevated temperature of 12°C, growth rates were increased compared to ambient temperature of 8°C and were in the lower range of rates estimated by Orejas et al. (2011) with the same technique for Mediterranean Lophelia pertusa grown for 3 months at the same temperature, which is typical for Mediterranean cold-water coral sites. This shows that, although adapted to lower temperatures, L. pertusa from the North Atlantic gets along with elevated temperatures as high as 12°C that are within the range of temperatures reflected in the wide global distribution of this species. The reason for the yet lower rates might be due to a different temperature optimum of the NE Atlantic corals exposed to constant low temperatures compared to Mediterranean corals adapted to higher temperatures.
Growth rates in the elevated temperature treatment were further increased when more food was available, indicating that the metabolism was stimulated and more energy was allocated to processes such as calcification. Several studies on tropical scleractinian corals demonstrated that nutrient enrichment lowers the sensitivity in calcification and net production processes towards changes in concentration and aragonite saturation (i.e., Langdon and Atkinson, 2005; Atkinson and Cuet, 2008; Edmunds, 2011). As nutrient enrichment serves to stimulate photosynthetic production of the zooxanthellae symbionts, increased food supply or nutrient addition helps to counteract the negative effects of ocean acidification (Langdon and Atkinson, 2005; Atkinson and Cuet, 2008). But also the improvement of the nutritional status by increased heterotrophic feeding was detected to modulate the responses of warm-water corals to elevated pCO2 levels (Holocomb et al., 2010; Edmunds, 2011).
However, under ambient temperature in our study, a higher food supply did not lead to increased growth rates, which indicates that the corals were not able to utilise the additional food at this temperature, neither under control conditions, nor at an elevated pCO2 level.
Under the end-of-the-century ocean acidification scenario (~800 μatm) growth rates were decreased compared to the control. Although the difference was not statistically significant, net calcification was close to zero in both low pH treatments at ambient temperature. A tendency towards decreased calcification at undersaturated conditions was also observed by Hennige et al. (2015). In contrast, Form and Riebesell (2012) detected a slight increase in calcification rates in response to long-term exposure (6 months) to undersaturated conditions.
These opposing responses to ocean acidification may be due to differences in cultivation conditions or may reflect the different origins of the coral specimens and the specific adaptation to the variability in carbonate chemistry experienced in their natural habitat.
But in fact, several studies emphasised that on longer time scales (weeks to months) scleractinian cold-water corals are capable of calcifying under low pH conditions (i.e., Form and Riebesell, 2012; Maier et al., 2013a; Movilla et al., 2014b; Hennige et al., 2015; Georgian et al., 2016; Gori et al., 2016), sometimes revealing slightly increased and sometimes reduced calcification rates or no change at all.
Moreover, lowered net growth rates are not necessarily a result of reduced calcification alone. They may also originate from dissolution, which might be more pronounced when the coral fragments have more “free” skeleton (without protective coenosarc), i.e., due to frequent experimental handling. Net growth rates just above zero might therefore indicate that growth and dissolution balance each other out. If calcification is masked by dissolution, the impact of elevated CO2 concentration might not be a concern of acclimatisation of the living polyps, but rather a problem of increased dissolution rates of the coral's skeleton, since it was shown here that the corals are able to grow at undersaturated conditions, especially in combination with elevated temperature (compare also Venn et al., 2013). One reason for calcification even in unfavourable conditions is probably the internal pH up-regulation at the site of calcification (McCulloch et al., 2012b; Wall et al., 2015). The pH of the calcifying fluid (pHcf) at the site of calcification was found to be closely related to seawater saturation state ΩAr and therewith to Ωcf, which is thought to be the parameter that primarily controls calcification in azooxanthellate cold-water corals (McCulloch et al., 2012b). Slower growth rates under ocean acidification are therefore presumably attributed to additional energetic costs that require ~10% per 0.1 units decrease in seawater pH in order to maintain elevated pH values at the site of calcification (McCulloch et al., 2012b). pH homeostasis during calcification was also observed in several tropical shallow-water corals (Hönisch et al., 2004; Krief et al., 2010; Trotter et al., 2011; McCulloch et al., 2012a; Holocomb et al., 2014; Georgiou et al., 2015) but was found to be not as strong as in cold-water corals (ΔpH between seawater pHT and pHcf) (McCulloch et al., 2012b), demonstrating the adaptation of these corals to live in environments with naturally lower aragonite saturation states.
The skeleton of the living corals is, furthermore, protected by the coenosarc, an organic layer, which helps to prevent the skeletal material from dissolution, whereas the Lophelia pertusa reef ecosystems consist to large parts of dead, bioeroded framework that is not protected by organic material and, therefore, more vulnerable and more easily to dissolve.
Moreover, Wisshak et al. (2012, 2014) demonstrated that reef bioerosion through boring sponges and probably many other bioeroders is accelerated by ocean acidification, which might lead to a weaker reef framework in the future and comprises an additional factor especially in the “dead zone” of the reefs.
No difference in growth rates was detected between the control groups and the combined elevated temperature and pCO2 treatment, the climate scenario that will most likely occur in the future. When compared to the single factor treatments, this appears to be an antagonistic response where elevated growth at increased temperature compensates for decreased calcification at undersaturated conditions. Thus, without information of the responses to single stressors one may conclude that there is no impact of climate change on coral growth.
Considering that the underlying processes are altered and energetic pathways are modified, it cannot be excluded that acclimatisation comes at a cost. Essential processes may be sustained to the benefit of growth but other physiological processes might be disadvantaged by re-allocation of energy (see also Hennige et al., 2015). Under elevated temperature the costs of acclimatisation could probably be met by higher food supply as was observed by seemingly higher fitness and calcification rates in this study. However, our results also revealed that an elevated energetic input in the combined treatment did not induce elevated calcification compared to the low fed group, although it could be shown for Lophelia that the food uptake increases with food availability (Purser et al., 2010). According to food capture rate calculations based on a flume experiment by Purser and colleagues, the maximum feeding rates of L. pertusa in one feeding occasion were about 73 Artemia nauplii polyp−1 h−1, equating to 66 μg C polyp−1 h−1 (Purser et al., 2010). However, Tsounis et al. (2010) reported higher capture rates of ~284 ± 130 individuals polyp−1 h−1 by L. pertusa, but experiments in this study were done at 12°C and, therewith, four degrees higher temperature than in the compared study. At higher temperatures metabolic rates can be increased (Dodds et al., 2007) and, thus, require greater energetic demands. Lower flow velocities applied by Tsounis et al. (2010) additionally enhance the food capture efficiency (Purser et al., 2010). A recently published feeding study by Orejas et al. (2016) using different food types confirmed that lower flow velocities enhance food capturing by L. pertusa. In their experiment, copepods were used as zooplankton food source and were captured best at the lowest tested flow velocity of 2 cm s−1, while phytoplankton was consumed preferentially at 5 cm s−1 (Orejas et al., 2016). At 10 cm s−1 the least food particles were captured.
In Tsounis et al. (2010) initial food densities were far higher with 10–20 Artemia nauplii mL−1 compared to 0.345–1.035 nauplii mL−1 in the study by Purser et al. (2010) and 0.1 copepods mL−1 in the study by Orejas et al. (2016). Compared to these studies, our food concentration in the experimental tanks was well in the range of the study by Purser et al. (2010) with ~0.3 Artemia nauplii mL−1 in the low food treatments and ~3 nauplii mL−1 in the high food aquaria. Purser and colleagues demonstrated in their study that at the lower initial food density average net capture rates were less than half as much as under a doubled food concentration (0.69 individuals mL−1), while an even higher food density of about 1 individual mL−1 did not result in much further increased average net uptake rates. Assuming similar capture rates as in Purser et al. (2010), we achieved two different feeding regimes and capture efficiency statuses with the 10-fold difference in initial food densities, corresponding to at least a doubling in C capture by the “high food” groups.
Thus, despite the strong correlation between food density and food capture efficiency, additional food could not compensate for the presumable detrimental effects of CO2 under combined elevated temperature and CO2 impact, but seems to solely stimulate the metabolism when only temperature is elevated. While some species are able to increase their feeding rates on zooplankton to meet potentially increased energetic demands for CaCO3 production caused by ocean acidification (Holocomb et al., 2010; Allemand et al., 2011), Houlbrèque et al. (2015) postulated that others might suffer from lowered capture rates of prey items as well as reduced organic nutrient acquisition due to pH-dependent carrier transports and enzyme functions. Reduced feeding rates as demonstrated for the scleractinian reef-building coral Stylophora pistillata (Houlbrèque et al., 2015) would therefore counteract the positive mechanisms of increased heterotrophic feeding of tropical corals to sustain their growth rates as shown by Edmunds (2011). Possible reasons for decreased capture rates could be a higher frequency in polyp retraction or reduced mobility. In this context it would be interesting to investigate polyp behaviour under ocean acidification.
The monthly repeated buoyancy weight measurements at the same conditions over 6 months of incubation showed high variability in growth rates. Not only between replicates, but also single replicate comparisons over time revealed varying calcification rates. Thus, over longer time periods growth rates in L. pertusa colonies are not constant, which is probably one of the reasons for the high variability within as well as high standard deviation between replicates. Mortensen (2001) described episodic fast growth occasions and allometric growth patterns of L. pertusa, which underlines that growth in L. pertusa is not consistent and might be different for different branches and polyps at different time points. The used method of buoyancy weighing represents growth increments in weight gain (or loss) of the whole branch over time and does not distinguish allometric growth of different polyps of a branch. Basal parts of the branches might grow in thickness, while the younger, distal polyps rather extend thinner corallites in length. Although it was taken care that all coral fragments were similarly distributed with regard to polyp counts and sizes, different polyp generations between the branches might lead to continuously higher growth rates in some branches than others. A different method to measure growth rates in corals while keeping them alive is the analysis of linear extension rates. However, this technique requires marking of the corals with dye or isotopic labels. While staining of the corals might lead to impairment to health, isotopic labelling for calcification analyses has been successfully applied on tropical corals without affecting physiological processes (Brahmi et al., 2012). Besides, linear extension rates are rather applicable for younger, more distal polyp generations and do not account for thickening growth of the entire branch. Thus, buoyancy weighing comprises the most suitable method to keep the organisms alive with the least possible handling and gives the measure on the entire fragment examined, which is especially valuable with regard to detecting differences in growth dynamics of the different treatment conditions and potential dissolution effects.
High variability in Lophelia responses was also referred to in previous studies (e.g., Form and Riebesell, 2012; Lunden et al., 2014; Hennige et al., 2015) and might also result from genetically distinct populations used in the different experimental treatments (Morrison et al., 2011; Lunden et al., 2014). In the present study it was taken care that fragments from the same colonies were not transferred to aquaria of the same treatment in order to represent a broader range of genotypes in each treatment.
The general trend of the different responses towards the various treatments developed relatively early during the experimental period. That is to say the direction of response to the different treatment conditions emerged already during the first month after modification of pCO2 and temperature. This indicates that there was a quick adjustment of the metabolism of the corals within about 4–6 weeks and relatively little change in response orientation during the following months, despite the general decline of growth rates in all treatments towards the end of the experiment. Hence, acclimatisation in terms of metabolic adjustments appears to occur relatively quickly, but it is questionable if positive net growth rates can be maintained over even longer time periods beyond half a year and if this comes at a cost, which according to our results cannot be met by additional food input alone. Thus, it appears likely that energy from other processes might be drawn to sustain growth. It would therefore be worthwhile to measure the metabolic rate of the corals under long-term influence of ocean acidification and warming in order to see if the increased costs of mineralisation are reflecting in enhanced respiration for instance. In two mid- and long-term studies, respiration rates did not significantly change with elevated pCO2 levels compared to ambient levels (Maier et al., 2013b; Hennige et al., 2015), while a significant decline was found after short-term exposure to acidified conditions (3 weeks at 750 μatm) (Hennige et al., 2014). Elevated temperature by 2°C led to a 50% increase in respiration rates of L. pertusa (Dodds et al., 2007), but combined long-term exposure of elevated temperature and CO2 concentration did not result in significant changes (Hennige et al., 2015).
Morphological Alterations
Towards the end of the experiment, structural deformations of the polyps were observed in most of the manipulated replicates, at elevated temperature, lower pH conditions and the combined treatments. Recently, Hennige et al. (2015) observed altered crystal organisation of the aragonite skeleton of newly grown corallites after long-term exposure to elevated pCO2 levels, which became even more pronounced at undersaturated conditions (ΩAr = 0.76) at 1,000 μatm. However, no changes in skeletal microstructure were observed under elevated temperature or the combined impact of increased CO2 concentration and temperature. In contrast to observations by Hennige et al. (2015), pure morphological deformations in coral calyx structure observed in this study (Figure 2) were most prominent in the combined treatment. The combined treatment (750 μatm and 12°C) in the study of Hennige et al. (2015) was still supersaturated with respect to aragonite (ΩAr = 1.19), whereas in the present study undersaturation was also prominent in the combined treatment of elevated pCO2 and temperature (Table 1).
Although the deformations observed in this study must not necessarily underlie changes in the crystal organisation, morphological alterations with regard to the shape of the calyces might be interrelated with structural disorganisation or changes in density of the skeleton at undersaturated conditions, as observed under similar conditions by Hennige et al. (2015).
In contrast, Wall et al. (2015) did not see distinctive differences in skeletal morphology in L. pertusa specimens analysed with Raman mapping between corals kept under ambient and low pH conditions (corresponding to a pCO2 of up to 980 μatm). The authors speculated that no detrimental effects on the skeletal morphology appears as long as individuals have sufficient energy (i.e., through food intake) to sustain pH-upregulation at the site of calcification even at undersaturated conditions (Wall et al., 2015). However, observations were only made on adult polyps, whereas structural alterations in Hennige et al. (2015) were observed in newly grown polyps and also in the present study deformations were most prominent in newly developed corallites.
The development of longer and thinner corallites was suggested as a strategy to enhance prey capture opportunities for the polyps (Hennige et al., 2015). While this might be true for the natural habitat, no difference in the degree of deformation was observed in the laboratory between highly and lightly fed corals within one temperature and/or pCO2 treatment in the present study, which again might be due to reduced food capture rates in case of increased CO2 concentrations (compare Houlbrèque et al., 2015).
A change in morphology during incubation at elevated pCO2 conditions was also observed for the tropical scleractinian coral species S. pistillata (Tambutte et al., 2015). Tambutte and colleagues found that these morphological alterations were accompanied by an increased incorporation of organic matrix proteins into the skeleton, which was suggested to occur to facilitate calcification at lower aragonite saturation by catalysing the precipitation of new aragonite crystals (Mass et al., 2013).
In the present study, deformations were also observed in the elevated temperature treatment, which demonstrates that it is not only pCO2 that leads to morphological alterations. Here, the deformations of polyps were probably a result of unfavourable conditions for “normal” calcification, rather than an attempt to increase chances to reach more food particles.
Fitness
To the author's best knowledge, this is the first attempt of measuring RNA/DNA ratios in cold-water corals to assess the metabolic activity under different conditions with regard to ocean change. RNA/DNA ratios were investigated in numerous studies as an indicator of the condition or health status of larval fish (i.e., Bulow, 1987; Clemmesen, 1987; Buckley et al., 1999) and marine invertebrates (i.e., Wright and Hetzel, 1985; Frantzis et al., 1992) with a few studies on cnidarians (Meesters et al., 2002; Buckley and Szmant, 2004). In fish larvae as well as in reef-building warm-water corals it has been shown that the RNA/DNA ratio is a very sensitive parameter for determining the nutritional status with significantly higher ratios in fed compared to starved fish larvae (Clemmesen, 1987). In corals, indirect nutritional effects are assumed to be reflected by the correlation of RNA/DNA ratios and light intensity with elevated RNA/DNA ratios at lower depth and/or less turbid areas, respectively, suggesting that metabolic effects of the close relationship of coral host and endosymbiotic zooxanthellae are related to their dependence on light (Meesters et al., 2002; Buckley and Szmant, 2004).
Our RNA and DNA measurements on the azooxanthellate cold-water coral L. pertusa show diverging responses to the different treatment conditions and support the overall pattern obtained for growth rates. There was a statistically significant interaction between all three factors (pCO2, temperature, food), which indicates that the effect of one factor differs when applied in combination with any of the two other factors (Three-Way ANOVA). Except for the combined treatments, fitness was higher in all “high food” than in the “low food” treatments with the highest RNA/DNA ratio being present at 12°C and ambient pCO2 level under elevated food availability, which is in accordance with additionally increased growth rates in this treatment.
In the combined treatment, elevated food availability did not result in higher RNA/DNA ratios. Thus, the fitness data for the combined treatment supports the trends observed for growth rates with no difference between “high” fed and “low” fed corals of this treatment. This emphasises the above mentioned hypothesis that the additional food provided was not taken up by L. pertusa or could not be used to stimulate metabolic activity or calcification under the combined impact of elevated CO2 and temperature.
In contrast, under ambient temperature, fitness was slightly but significantly higher in the “high” fed group of the elevated pCO2 treatment. Thus, if the elevated fitness is related to higher food availability at 8°C, additional food must have been captured compared to the “low food” group, which was suggested not to take place under elevated pCO2 levels (compare Houlbrèque et al., 2015). One explanation for the additional food uptake under elevated CO2 concentration at ambient temperature, while this is supposed to be suppressed in the combined elevated pCO2 treatment at 12°C, might be temperature dependent enzymes and/or carrier transports involved in the food consumption. Enzyme functions and carrier transports are usually temperature dependent and have a pH optimum. Since the optimal pH of some enzymes is temperature dependent (Hazel et al., 1978) and might be different when adapted to lower temperatures, the enzyme activity of enzymes involved in food consumption might be different under different temperatures. This might explain that the tropical species S. pistillata in the study by Houlbrèque et al. (2015) was not able to additionally take up food particles at its ambient temperature (27°), while L. pertusa was able to incorporate additional food at an elevated pCO2 level at a far lower ambient temperature, but not at additionally elevated temperature. However, a significantly higher fitness under “high food” conditions at ambient temperature and elevated pCO2 at the end of the experiment did not result in elevated growth rates, demonstrating the yet negative effects of lowered pH, either due to a strongly increased dissolution effect or a re-allocation of energy to other processes.
Mortality
The mortality was generally relatively low over the course of the experiment. On average 8% of all polyps died during the 6 months of incubation, which was not attributable to any of the manipulations. In the 8°C treatments, mortality was slightly higher under elevated food availability despite higher fitness values. This increased mortality might be explainable by elevated nutrient concentrations due to food particles that were not consumed. However, nutrient concentrations were similarly elevated for all “high food” treatments (3- to 4-fold higher compared to “low food” treatments) and in the same range of values. Under elevated temperature, increased nitrogen waste products (nitrate, nitrite, and ammonium) due to food addition did not cause higher mortality rates, which might be due to the fact that corals were better able to take up the additional food and had presumably higher metabolic rates than at ambient temperature. Conversely, highest mortality rates were found in the combined “low food” treatment, although growth rates and fitness in this treatment remained in a range comparable to the other treatments. The high mortality in this treatment was attributable to one replicate that showed 36% mortality at the end of the experiment with no explainable reason and which, thus, may be seen as an outlier.
Conclusions
The different responses with respect to ocean acidification and warming show how highly variable and plastic this species is in terms of coping with environmental changes. Several studies have shown that cold-water corals are able to sustain positive growth under ocean acidification and/or warming, but net calcification rates of L. pertusa exposed to ΩAr-undersaturated conditions often decreased close to zero or even became negative (i.e., Lunden et al., 2014; Hennige et al., 2015) as was likewise observed in the elevated pCO2 treatment of the present study. If dissolution leads to decreased net growth even in living parts of coral reefs, the reef stability of cold-water coral ecosystems might be considerably endangered in the future, particularly since large parts of the reefs consist of eroded skeleton material that is not protected by organic tissues and is more susceptible to dissolution and bioerosion, which might predominantly lead to degradation in cold-water corals under future climate change. But yet, even in the living parts ocean change might lead to changes in physiological performance, entailing probably higher energetic demands to sustain calcification rates, as well as reduced fitness and viability in case food availability is not sufficient.
However, laboratory studies are conducted at narrowly controlled environmental parameters, thus, do not simulate the dynamic and changing environment on the reef sites. Therefore, more realistic predictions of how cold-water corals will behave in the field in a future “high CO2 world” should be conducted taking into account environmental fluctuations and seasonality of the natural habitat as well as natural nutrition and interactions with other species. Thus, in future cold-water coral research, the impacts of climate change should be addressed considering the dynamics in cold-water coral ecosystems to understand the physiological performance under changing environmental conditions.
Author Contributions
JB and AF designed the study and performed the experimental measurements. JB wrote the manuscript. All authors contributed substantially to the discussion of the results and revision of the manuscript.
Conflict of Interest Statement
The authors declare that the research was conducted in the absence of any commercial or financial relationships that could be construed as a potential conflict of interest.
Acknowledgments
This study was carried out as part of the German coordinated project Biological Impacts of Ocean Acidification (BIOACID II, Grant number: FKZ 03F0655A) funded by the Federal Ministry of Education and Research (BMBF). The coral sampling at the outer Norwegian Trondheim fjord was conducted with kind permission of the Norwegian Directorate of Fisheries (Fiskeridirektoratet). The captain and crew of RV POSEDION are greatly thanked for support during the research cruise POS420. Export and import permits for the specimens, following the Convention on International Trade in Endangered Species of Wild Fauna and Flora (CITES), were admitted by the Norwegian Environment Agency (Miljø Direktoratet) and the Federal Agency for Nature Conservation (BfN). We would like to thank Susann Diercks and Marie Küter for technical assistance throughout the experiment, and Kerstin Nachtigall, Andrea Ludwig, as well as Dr. Catriona Clemmesen and Burkhard von Dewitz for assistance with chemical analyses. Moreover, Dr. Max Wisshak and Dr. Sascha Flögel are thanked for their constructive feedback on the manuscript.
References
Allemand, D., Tambutté, É., Zoccola, D., and Tambutté, S. (2011). “Coral calcification, cells to reefs,” in Coral Reefs: An Ecosystem in Transition, eds Z. Dubinsky and N Stambler (Dordrecht; Heidelberg; London; New York, NY: Springer), 119–150. doi: 10.1007/978-94-007-0114-4_9
Atkinson, M. J., and Cuet, P. (2008). Possible effects of ocean acidification on coral reef biogeochemistry: topics for research. Mar. Ecol. Prog. Ser. 379, 249–256. doi: 10.3354/meps07867
Barnett, T. P., Pierce, D. W., AchutaRao, K. M., Gleckler, P. J., Santer, B. D., Gregory, J. M., et al. (2005). Penetration of human-induced warming into the world's oceans. Science 309, 284–287. doi: 10.1126/science.1112418
Brahmi, C., Kopp, C., Domart-Coulon, I., Stolarski, J., and Meibom, A. (2012). Skeletal growth dynamics linked to trace-element composition in the scleractinian coral Pocillopora damicornis. Geochim. Cosmochim. Acta 99, 146–158. doi: 10.1016/j.gca.2012.09.031
Buckley, B. A., and Szmant, A. M. (2004). RNA/DNA ratios as indicators of metabolic activity in four species of Caribbean reef-building corals. Mar. Ecol. Prog. Ser. 282, 143–149. doi: 10.3354/meps282143
Buckley, L., Caldarone, E., and Ong, T. L. (1999). RNA-DNA ratio and other nucleic acid-based indicators for growth and condition of marine fishes. Hydrobiologia 401, 265–277. doi: 10.1023/A:1003798613241
Bulow, F. J. (1987). “RNA–DNA ratios as indicators of growth in fish: a review,” in The Age and Growth of Fish, eds R. C. Summerfelt and G. C. Hall (Ames, IA: Iowa State University Press), 45–65.
Caldeira, K., and Wickett, M. E. (2003). Anthropogenic carbon and ocean pH. Nature 425:365. doi: 10.1038/425365a
Clemmesen, C. M. (1987). Laboratory studies on RNA/DNA ratios of starved and fed herring (Clupea harengus) and turbot (Scophthalmus maximus) larvae. J. Conseil 43, 122–128. doi: 10.1093/icesjms/43.2.122
Clemmesen, C. M. (1993). Improvements in the fluorometric-determination of the RNA and DNA content of individual marine fish larvae. Mar. Ecol. Prog. Ser. 100, 177–183. doi: 10.3354/meps100177
Curry, R., Dickson, B., and Yashayaev, I. (2003). A change in the freshwater balance of the Atlantic Ocean over the past four decades. Nature 426, 826–829. doi: 10.1038/nature02206
Davies, A. J., and Guinotte, J. M. (2011). Global habitat suitability for framework-forming cold-water corals. PLoS ONE 6:e18483. doi: 10.1371/journal.pone.0018483
Davies, P. S. (1989). Short-term growth measurements of corals using an accurate buoyant weighing technique. Mar. Biol. 101, 389–395. doi: 10.1007/BF00428135
Dickson, A. G., Afghan, J. D., and Anderson, G. C. (2003). Reference materials for oceanic CO2 analysis: a method for the certification of total alkalinity. Mar. Chem. 80, 185–197. doi: 10.1016/S0304-4203(02)00133-0
Dickson, A. G., and Millero, F. J. (1987). A comparison of the equilibrium constants for the dissociation of carbonic acid in seawater media. Deep Sea Res. 34, 1733–1743. doi: 10.1016/0198-0149(87)90021-5
Dickson, A. G., Sabine, C. L., and Christian, J. R. (eds.). (2007). Guide to Best Practices for Ocean CO2 Measurements. Sidney, BC: PICES Special Publication 3.
Dodds, L. A., Roberts, J. M., Taylor, A. C., and Marubini, F. (2007). Metabolic tolerance of the cold-water coral Lophelia pertusa (Scleractinia), to temperature and dissolved oxygen change. J. Exp. Mar. Biol. Ecol. 349, 205–214. doi: 10.1016/j.jembe.2007.05.013
Edmunds, P. J. (2011). Zooplanktivory ameliorates the effects of ocean acidification on the reef coral. Limnol. Oceanogr. 56, 1–11. doi: 10.4319/lo.2011.56.6.2402
Feely, R. A., Sabine, C. L., Lee, K., Berelson, W., Kleypas, J., Fabry, V. J., et al. (2004). Impact of anthropogenic CO2 on the CaCO3 system in the oceans. Science 305, 362–366. doi: 10.1126/science.1097329
Form, A. U., and Riebesell, U. (2012). Acclimation to ocean acidification during long-term CO2 exposure in the cold-water coral Lophelia pertusa. Glob. Chang. Biol. 18, 843–853. doi: 10.1111/j.1365-2486.2011.02583.x
Frantzis, A., Gremare, A., and Vetion, G. (1992). Growth rates and RNA/DNA ratios in Paracentrotus lividus (Echinodermata: Echinoidea) fed on benthic macrophytes. J. Exp. Mar. Biol. Ecol. 156, 125–138. doi: 10.1016/0022-0981(92)90020-B
Freiwald, A., Fosså, J. H., Grehan, A., Koslow, T., and Roberts, J. M. (2004). “Cold-water coral reefs: out of sight - no longer out of mind,” in UNEP-WCMC Biodiversity Series 22, eds S. Hain and E. Corcoran (Cambridge, UK: UNEP World Conservation Monitoring Centre), 1–86.
Gattuso, J.-P., Frankignoulle, M., Bourge, I., Romaine, S., and Buddemeier, R. W. (1998). Effect of calcium carbonate saturation of seawater on coral calcification. Glob. Planet Change 18, 37–46. doi: 10.1016/S0921-8181(98)00035-6
Georgian, S. E., Dupont, S., Kurman, M., Butler, A., Strömberg, S. M., Larsson, A. I., et al. (2016). Biogeographic variability in the physiological response of the cold-water coral Lophelia pertusa to ocean acidification. Mar. Ecol. 31, 1345–1359. doi: 10.1111/maec.12373
Georgiou, L., Falter, J., Trotter, J., Kline, D. I., Holcomb, M., Dove, S. G., et al. (2015). pH homeostasis during coral calcification in a free ocean CO2 enrichment (FOCE) experiment, Heron Island reef flat, Great Barrier Reef. Proc. Natl. Acad. Sci. U.S.A. 112, 13219–13224. doi: 10.1073/pnas.1505586112
Gori, A., Ferrier-Pagès, C., Hennige, S. J., Murray, F., Rottier, C., Wicks, L. C., et al. (2016). Physiological response of the cold-water coral Desmophyllum dianthus to thermal stress and ocean acidification. PeerJ 4:e1606. doi: 10.7717/peerj.1606
Graham, N. A. J., Jennings, S., MacNeil, M. A., Mouillot, D., and Wilson, S. K. (2015). Predicting climate-driven regime shifts versus rebound potential in coral reefs. Nature 518, 94–97. doi: 10.1038/nature14140
Guinotte, J. M., Orr, J. C., Cairns, S. D., Freiwald, A., Morgan, L., and George, R. (2006). Will human-induced changes in seawater chemistry alter the distribution of deep-sea scleractinian corals? Front. Ecol. Environ. 4, 141–146. doi: 10.1890/1540-9295(2006)004[0141:WHCISC]2.0.CO;2
Haugan, P. M., and Drange, H. (1996). Effects of CO2 on the ocean environment. Proc. Int. Ener. Agency Greenhouse Gases 37, 1019–1022. doi: 10.1016/0196-8904(95)00292-8
Hazel, J. R., Garlick, W. S., and Sellner, P. A. (1978). The effects of assay temperature upon the pH optima of enzymes from poikilotherms: a test of the imidazole alphastat hypothesis. J. Comp. Physiol. 123, 97–104. doi: 10.1007/BF00687837
Hennige, S. J., Wicks, L. C., Kamenos, N. A., Bakker, D. C. E., Findlay, H. S., Dumousseaud, C., et al. (2014). Short-term metabolic and growth responses of the cold-water coral Lophelia pertusa to ocean acidification. Deep-Sea Res. II 99, 27–35. doi: 10.1016/j.dsr2.2013.07.005
Hennige, S. J., Wicks, L. C., Kamenos, N. A., Perna, G., Findlay, H. S., and Roberts, J. M. (2015). Hidden impacts of ocean acidification to live and dead coral framework. Proc. R. Soc. B 282, 1–10. doi: 10.1098/rspb.2015.0990
Holocomb, M., McCorkle, D. C., and Cohen, A. L. (2010). Long-term effects of nutrient and CO2 enrichment on the temperate coral Astrangia poculata (Ellis and Solander, 1786). J. Exp. Mar. Biol. Ecol. 386, 27–33. doi: 10.1016/j.jembe.2010.02.007
Holocomb, M., Venn, A. A., Tambutte, E., Tambutte, S., Allemand, D., Trotter, J., et al. (2014). Coral calcifying fluid pH dictates response to ocean acidification. Sci. Rep. 4, 1–4. doi: 10.1038/srep05207
Hönisch, B., Hemming, N. G., Grottoli, A. G., Amat, A., Hanson, G. N., and Bijma, J. (2004). Assessing scleractinian corals as recorders for paleo-pH: empirical calibration and vital effects. Geochim. Cosmochim. Acta 68, 3675–3685. doi: 10.1016/j.gca.2004.03.002
Houlbrèque, F., Reynaud, S., Godinot, C., Oberhänsli, F., Rodolfo-Metalpa, R., and Ferrier-Pagès, C. (2015). Ocean acidification reduces feeding rates in the scleractinian coral Stylophora pistillata. Limnol. Oceanogr. 60, 89–99. doi: 10.1002/lno.10003
Hughes, T. P., Baird, A. H., Bellwood, D. R., Card, M., Connolly, S. R., Folke, C., et al. (2003). Climate change, human impacts, and the resilience of coral reefs. Science 301, 929–933. doi: 10.1126/science.1085046
IPCC (2014). Climate Change 2014: Synthesis Report. Contribution of Working Groups, I., II and III to the Fifth Assessment Report of the Intergovernmental Panel on Climate Change, eds Core Writing Team, R. K. Pachauri, and L. A. Meyer. Geneva: IPCC.
Jokiel, P. L., Maragos, J. E., and Franzisket, L. (1978). “Coral growth: buoyant weight technique,” in Coral Reefs: Research Methods, eds D. R. Stoddart, R. E. Johannes (Paris: UNESCO Monographs on Oceanographic Methodology), 529–542.
Jones, D. O. B., Yool, A., Wei, C.-L., Henson, S. A., Ruhl, H. A., Watson, R. A., et al. (2014). Global reductions in seafloor biomass in response to climate change. Glob. Chang. Biol. 20, 1861–1872. doi: 10.1111/gcb.12480
Krief, S., Hendy, E. J., Fine, M., Yam, R., Meibom, A., Foster, G. L., et al. (2010). Physiological and isotopic responses of scleractinian corals to ocean acidification. Geochim. Cosmochim. Acta 74, 4988–5001. doi: 10.1016/j.gca.2010.05.023
Langdon, C., and Atkinson, M. J. (2005). Effect of elevated pCO2 on photosynthesis and calcification of corals and interactions with seasonal change in temperature/irradiance and nutrient enrichment. J. Geophys. Res. 110, 1–16. doi: 10.1029/2004JC002576
Lewis, E., and Wallace, D. W. R. (1998). Program Developed for CO2 System Calculations. Oak Ridge, TN: Carbon Dioxide Information and Analysis centre, Oak Ridge National Laboratory, U.S. Department of Energy.
Lunden, J. J., McNicholl, C. G., Sears, C. R., Morrison, C. L., and Cordes, E. E. (2014). Acute survivorship of the deep-sea coral Lophelia pertusa from the Gulf of Mexico under acidification, warming, and deoxygenation. Front. Mar. Sci. 1:78. doi: 10.3389/fmars.2014.00078
Maier, C., Bils, F., Weinbauer, M. G., Watremez, P., Peck, M. A., and Gattuso, J.-P. (2013b). Respiration of Mediterranean cold-water corals is not affected by ocean acidification as projected for the end of the century. Biogeosciences 10, 5671–5680. doi: 10.5194/bg-10-5671-2013
Maier, C., Hegeman, J., Weinbauer, M. G., and Gattuso, J.-P. (2009). Calcification of the cold-water coral Lophelia pertusa under ambient and reduced pH. Biogeosciences 6, 1671–1680. doi: 10.5194/bg-6-1671-2009
Maier, C., Schubert, A., Sanchez, M. M. B., Weinbauer, M. G., Watremez, P., and Gattuso, J.-P. (2013a). End of the century pCO2 levels do not impact calcification in Mediterranean cold-water corals. PLoS ONE 8:e62655. doi: 10.1371/journal.pone.0062655
Mass, T., Drake, J. L., Haramaty, L., Kim, J. D., Zelzion, E., Bhattacharya, D., et al. (2013). Cloning and characterization of four novel coral acid-rich proteins that precipitate carbonates in vitro. Curr. Biol. 23, 1126–1131. doi: 10.1016/j.cub.2013.05.007
McCulloch, M., Falter, J., Trotter, J., and Montagana, P. (2012a). Coral resilience to ocean acidification and global warming through pH up-regulation. Nat. Clim. Chang. 2, 623–627. doi: 10.1038/nclimate1473
McCulloch, M., Trotter, J., Montagana, P., Faltera, J., Dunbarf, R., Freiwald, A., et al. (2012b). Resilience of cold-water scleractinian corals to ocean acidification: boron isotopic systematics of pH and saturation state up-regulation. Geochim. Cosmochim. Acta 87, 21–34. doi: 10.1016/j.gca.2012.03.027
Meesters, E. H., Nieuwland, G., Duineveld, G. C. A., Kok, A., and Bak, R. P. M. (2002). RNA/DNA ratios of scleractinian corals suggest acclimatisation/adaptation in relation to light gradients and turbidity regimes. Mar. Ecol. Prog. Ser. 227, 233–239. doi: 10.3354/meps227233
Mehrbach, C., Culberso, C. H., Hawley, J. E., and Pytkowic, R. M. (1973). Measurement of apparent dissociation constants of carbonic acid in seawater at atmospheric pressure. Limnol. Oceanogr. 18, 897–907. doi: 10.4319/lo.1973.18.6.0897
Melatunan, S., Calosi, P., Rundle, S. D., Moody, A. J., and Widdicombe, S. (2011). Exposure to elevated temperature and pCO2 reduces respiration rate and energy status in the periwinkle Littorina littorea. Physiol. Biochem. Zool. 84, 583–594. doi: 10.1086/662680
Morrison, C. L., Ross, S. W., Nizinski, M. S., Brooke, S., Järnegren, J., Waller, R. G., et al. (2011). Genetic discontinuity among regional populations of Lophelia pertusa in the North Atlantic Ocean. Conserv. Genet. 12, 713–729. doi: 10.1007/s10592-010-0178-5
Mortensen, P. B. (2001). Aquarium observations on the deep-water coral Lophelia pertusa (L., 1758) (Scleractinia) and selected associated invertebrates. Ophelia 54, 83–104. doi: 10.1080/00785236.2001.10409457
Movilla, J., Gori, A., Calvo, E., Orejas, C., López-Sanz, À., Domínguez-Carrió, C., et al. (2014a). Resistance of two Mediterranean cold-water coral species to low-pH conditions. Water 6, 59–67. doi: 10.3390/w6010059
Movilla, J., Orejas, C., Calvo, E., Gori, A., López-Sanz, À., Grinyó, J., et al. (2014b). Differential response of two Mediterranean cold-water coral species to ocean acidification. Coral Reefs 33, 675–686. doi: 10.1007/s00338-014-1159-9
Naumann, M. S., Orejas, C., Wild, C., and Ferrier-Pagès, C. (2014). First evidence for zooplankton feeding sustaining key physiological processes in a scleractinian cold-water coral. J. Exp. Biol. 214, 3570–3576. doi: 10.1242/jeb.061390
Orejas, C., Ferrier-Pagès, C., Reynaud, S., Gori, A., Beraud, E., Tsounis, G., et al. (2011). Long-term growth rates of four Mediterranean cold-water coral species maintained in aquaria. Mar. Ecol. Prog. Ser. 429, 57–65. doi: 10.3354/meps09104
Orejas, C., Gori, A., Rad-Menéndez, C., Last, K. S., Davies, A. J., Beveridge, C. M., et al. (2016). The effect of flow speed and food size on the capture efficiency and feeding behaviour of the cold-water coral Lophelia pertusa. J. Exp. Mar. Biol. Ecol. 481, 34–40. doi: 10.1016/j.jembe.2016.04.002
Orr, J. C., Fabry, V. J., Aumont, O., Bopp, L., Doney, S. C., Feely, R. A., et al. (2005). Anthropogenic ocean acidification over the twentyfirst century and its impact on calcifying organisms. Nature 437, 681–686. doi: 10.1038/nature04095
Pandolfi, J. M., Connolly, S. R., Marshall, D. J., and Cohen, A. L. (2011). Projecting coral reef futures under global warming and ocean acidification. Science 333, 418–422. doi: 10.1126/science.1204794
Pierrot, D., Lewis, E., and Wallace, D. W. R. (2006). MS Excel Program Developed for CO2 System Calculations. ORNL/CDIAC-105a. Oak Ridge, TN: Carbon Dioxide Information Analysis Centre, Oak Ridge National Laboratory, U.S. Department of Energy.
Purser, A., Larsson, A. I., Thomsen, L., and van Oevelen, D. (2010). The influence of flow velocity and food concentration on Lophelia pertusa (Scleractinia) zooplankton capture rates. J. Exp. Mar. Biol. Ecol. 395, 55–62. doi: 10.1016/j.jembe.2010.08.013
Riebesell, U., Fabry, V. J., Hansson, L., and Gattuso, J.-P. (eds.). (2010). Guide to Best Practices for Ocean Acidification Research and Data Reporting. Luxembourg: Publications Office of the European Union.
Roberts, J. M., and Cairns, S. D. (2014). Cold-water corals in a changing ocean. Curr. Opin. Environ. Sustain. 7, 118–126. doi: 10.1016/j.cosust.2014.01.004
Roberts, J. M., Wheeler, A. J., and Freiwald, A. (2006). Reefs of the deep: the biology and geology of cold-water coral ecosystems. Science 312, 543–547. doi: 10.1126/science.1119861
Roberts, J. M., Wheeler, A. J., Freiwald, A., and Cairns, S. (2009). Cold-Water Corals: The Biology and Geology of Deep-Sea Coral Habitats. Cambridge: University Press.
Rodolfo-Metalpa, R., Houlbrèque, F., Tambutte, É., Boisson, F., Baggini, C., Patti, F. P., et al. (2011). Coral and mollusc resistance to ocean acidification adversely affected by warming. Nat. Clim. Chang. 1, 308–312. doi: 10.1038/nclimate1200
Sabine, C. L., Feely, R. A., Gruber, N., Key, R. M., Lee, K., Bullister, J. L., et al. (2004). The oceanic sink for anthropogenic CO2. Science 305, 367–371. doi: 10.1126/science.1097403
Spalding, M. D., and Brown, B. E. (2015). Warm-water coral reefs and climate change. Science 350, 769–771. doi: 10.1126/science.aad0349
Steinacher, M., Joos, F., Frölicher, T. L., Bopp, L., Cadule, P., Cocco, V., et al. (2010). Projected 21st century decrease in marine productivity: a multi-model analysis. Biogeosciences 7, 979–1005. doi: 10.5194/bg-7-979-2010
Tambutte, E., Venn, A. A., Holcomb, M., Segonds, N., Techer, N., Zoccola, D., et al. (2015). Morphological plasticity of the coral skeleton under CO2-driven seawater acidification. Nat. Commun. 6, 1–9. doi: 10.1038/ncomms8368
Trotter, J. A., Montagna, P., McCulloch, M. T., Silenzi, S., Reynaud, S., Mortimer, G., et al. (2011). Quantifying the pH ‘vital effect’ in the temperate zooxanthellate coral Cladocora caespitosa: validation of the boron seawater pH proxy. Earth Planet. Sci. Lett. 303, 163–173. doi: 10.1016/j.epsl.2011.01.030
Tsounis, G., Orejas, C., Reynaud, S., Gili, J.-M., Allemand, D., and Ferrier-Pagès, C. (2010). Prey-capture rates in four Mediterranean cold water corals. Mar. Ecol. Prog. Ser. 398, 140–155. doi: 10.3354/meps08312
Venn, A. A., Tambutte, E., Holcomb, M., Laurent, J., Allemand, D., and Tambutte, S. (2013). Impact of seawater acidification on pH at the tissue-skeleton interface and calcification in reef corals. Proc. Natl. Acad. Sci. U.S.A. 110, 1634–1639. doi: 10.1073/pnas.1216153110
Wall, M., Ragazzola, F., Foster, L. C., Form, A., and Schmidt, D. N. (2015). pH up-regulation as a potential mechanism for the cold-water coral Lophelia pertusa to sustain growth in aragonite undersaturated conditions. Biogeosciences 12, 6869–6880. doi: 10.5194/bg-12-6869-2015
Wisshak, M., Schoenberg, C. H. L., Form, A., and Freiwald, A. (2012). Ocean acidification accelerates reef bioerosion. PLoS ONE 7:e45124. doi: 10.1371/journal.pone.0045124
Wisshak, M., Schoenberg, C. H. L., Form, A., and Freiwald, A. (2014). Sponge bioerosion accelerated by ocean acidification across species and latitudes? Helgol. Mar. Res. 68, 253–262. doi: 10.1007/s10152-014-0385-4
Wood, H. L., Spicer, J. I., Lowe, D. M., and Widdicombe, S. (2010). Interaction of ocean acidification and temperature; the high cost of survival in the brittlestar Ophiura ophiura. Mar. Biol. 157, 2001–2013. doi: 10.1007/s00227-010-1469-6
Keywords: climate change, ocean acidification, global warming, food availability, Lophelia pertusa, cold-water corals
Citation: Büscher JV, Form AU and Riebesell U (2017) Interactive Effects of Ocean Acidification and Warming on Growth, Fitness and Survival of the Cold-Water Coral Lophelia pertusa under Different Food Availabilities. Front. Mar. Sci. 4:101. doi: 10.3389/fmars.2017.00101
Received: 19 December 2016; Accepted: 27 March 2017;
Published: 26 April 2017.
Edited by:
Hajime Kayanne, University of Tokyo, JapanReviewed by:
Aldo Cróquer, Simón Bolívar University, VenezuelaJarosław Stolarski, Polish Academy of Sciences (PAN), Poland
Copyright © 2017 Büscher, Form and Riebesell. This is an open-access article distributed under the terms of the Creative Commons Attribution License (CC BY). The use, distribution or reproduction in other forums is permitted, provided the original author(s) or licensor are credited and that the original publication in this journal is cited, in accordance with accepted academic practice. No use, distribution or reproduction is permitted which does not comply with these terms.
*Correspondence: Janina V. Büscher, amJ1ZXNjaGVyQGdlb21hci5kZQ==