- 1Department of Oceanography, Texas A&M University, College Station, TX, USA
- 2Department of Marine Sciences, Texas A&M University at Galveston, Galveston, TX, USA
- 3EcoLab, Université de Toulouse, CNRS, Institute National Polytechnique de Toulouse, Université Paul Sabatier Toulouse, France
A number of recent studies have documented that pyrogenic carbon (PyC) is an integral and significant proportion of DOM in worldwide rivers. This material originates from all fractions of the PyC continuum, from highly condensed PyC to more functionalized components that retain some structural identity of fuel molecules. Understanding the transfer of PyC to river systems is paramount for Arctic regions, given the projected increase in frequency and intensity of forest fires within these ecosystems. However, the environmental distribution and concentration of soluble and particulate PyC, parameters that govern the overall fate of PyC in aquatic systems, has so far been unstudied. Here, we analyze the concentration and phase distribution of the anhydrosugar biomarker levoglucosan, as a proxy for low-temperature PyC, in two high-latitude river systems: a small sub-Arctic Canadian river, the Great Whale River in northern Québec, and the largest Arctic River, the Yenisei River in north-central Siberia. Low-temperature PyC, as estimated by levoglucosan concentrations, is exported predominantly in the dissolved phase. Peak export of low-temperature PyC occurs during the spring freshet period in both rivers. Seasonal variability of dissolved and particulate PyC export in each river elucidated that the export of PyC in the particulate and dissolved phases were temporally decoupled throughout the peak discharge events. While the present work confirms that levoglucosan is exported in particulate phase at a high enough level to enter sedimentary deposits and record historical wildfire signatures, as the phase distribution varies between rivers and during different flow regimes, spatial and temporal differences may affect the usage of levoglucosan as a PyC proxy in depositional settings.
Introduction
Vegetation fires affect carbon cycling in all major earth systems. These fires release 1.6–2.8 petagrams of carbon (Pg C) to the atmosphere worldwide (Santín et al., 2016), mostly as gaseous CO2. However, inefficient combustion conditions in the environment regularly leave a proportion of the fire-affected carbon pool as carbonaceous residues, called pyrogenic carbon (PyC), which varies in molecular structure and environmental reactivity. The fate of pyrogenic carbon (PyC) in the environment, and the subsequent impact PyC has on carbon cycling, are a result of both its transport and degradation potential. As PyC is comprised of a continuum of materials, of which their formation and resulting chemical structures are temperature dependent (Masiello, 2004), the nature of PyC varies dramatically along the PyC continuum. The PyC continuum is marked by sequential changes in the proportion of chemical markers of combustion by-products, and increased aromatic condensation level (decreasing the ratio of Hydrogen to Carbon; H/C ratios) along temperature gradients (0–1,000°C; Kuo et al., 2008a,b, 2011a; Keiluweit et al., 2010; Figure 1). At low-temperatures (150–300°C), macropolymer dehydration and fragmentation leads to the release of free monomers and oligomers rich in −OH and −COOH functionalities (Kuo et al., 2008a,b, 2011a; Keiluweit et al., 2010; Harvey et al., 2012; Norwood et al., 2013). At these temperatures, the formation of anhydrosugars (levoglucosan and its isomers, mannosan, and galactosan) is prevalent (Kuo et al., 2008a; Figure 1). At intermediate temperatures (350–500°C), the rapid disappearance of recognizable plant lignocellulosic macromolecules, and the rapid drop in levoglucosan yields around 300–400°C (Kuo et al., 2008a, 2011a), occur synchronously with a steep increase in the proportion of amorphous aromatic structures (Keiluweit et al., 2010; Schneider et al., 2011; Harvey et al., 2012) as noted by the rapid decrease in H/C ratios and the rise in benzene polycarboxylic acids (BPCA), selective molecular markers of aromatic clusters (Ziolkowski and Druffel, 2010; Schneider et al., 2011; Figure 1). At the highest range of the temperature continuum (>500°C), PyC is characterized by increasing graphene-like structures in highly condensed turbostratic aromatics, marked by the increase in condensation levels of molecular markers such as BPCAs (Keiluweit et al., 2010; Schneider et al., 2011). This PyC-derived turbostratic carbon is relatively unordered (Nguyen et al., 2010) and is therefore structurally different than graphite found in natural environments, which is derived from high temperature and pressure conditions (such as within metamorphic rock) and is likely more ordered and highly crystalline (Brandes et al., 2008). High-temperature PyC can also be formed from the condensation of gas-phase intermediates (i.e., soot carbon; Schmidt and Noack, 2000; Hammes et al., 2007). As the lability of organic matter decreases along the PyC continuum (Ascough et al., 2011), the reactivity potential of PyC in the environment decreases with higher combustion temperatures (Masiello, 2004).
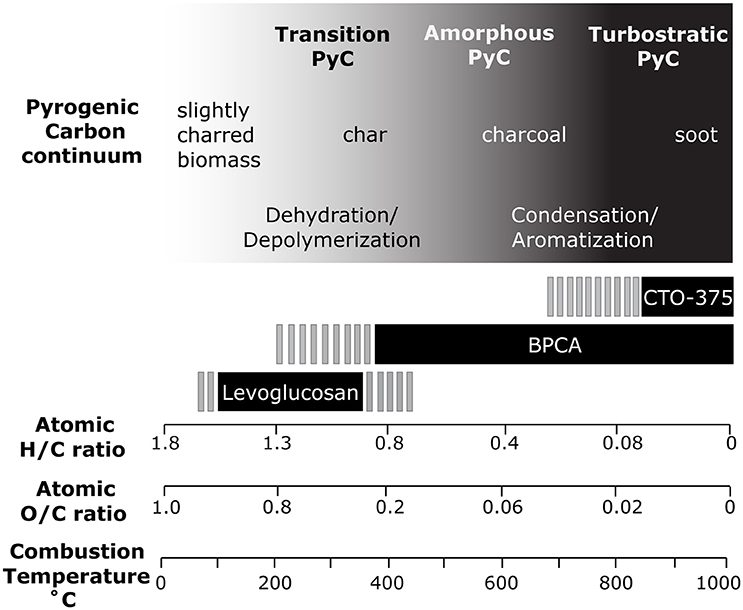
Figure 1. Updated pyrogenic carbon continuum inspired by Masiello (2004), including modifications inspired by Kuo et al. (2008a) and Hammes et al. (2007). This figure includes changes in the proportion of chemical markers of combustion by-products and the increased aromatic condensation level observed in biomass chars along a heat treatment temperature (HTT) gradient (0–1,000°C; Keiluweit et al., 2010; Harvey et al., 2012). Decreasing H/C ratios and O/C ratios along the HTT are synthesized from Kuo et al. (2008a), Keiluweit et al. (2010), and Schneider et al. (2011). Note that these vary dependent on feedstock and the scales on this figure are non-linear. Biomarker ranges derived from a variety of sources: benzene polycarboxylic acids (BPCA) from Schneider et al. (2010) and Schneider et al. (2011), levoglucosan range from Kuo et al. (2008a) and upper limit uncertainty from Lakshmanan et al. (1970), Chemo-thermal oxidation (CTO-375) range from Kuo et al. (2008a).
Wildfires are an important source of PyC to Arctic environments (Preston and Schmidt, 2006; Hansen et al., 2013; Santín et al., 2015). However, both the overall export and mechanisms for export of PyC within these systems is still an area of active research. Soil regimes in these systems vary widely; for example, the Yenisei River watershed is underlain by every type of permafrost regime, causing the soil active layer depth to vary dramatically within high latitude watersheds (Kawahigashi et al., 2004). It has been suggested that subsoil transport is a primary mechanism for PyC export from terrestrial to aquatic systems (Güereña et al., 2015), which may be regulated by soil-PyC associations. Czimczik and Masiello (2007) suggest that the ability of soil minerals to sorb PyC may be a main controller of PyC storage within soils, similar to bulk soil organic matter (SOM). When PyC is incorporated into soils, it may be associated with soil minerals (Hockaday et al., 2007; Knicker, 2011). PyC-mineral interactions observed on the surface of charcoals may also influence associations of PyC with minerals in the dissolved phase within soils (Hockaday et al., 2007), as soil mineral-DOM interactions affect the quantity of DOM exported from soil layers (Kaiser and Guggenberger, 2000; Kawahigashi et al., 2006). The distribution of PyC in Arctic soil profiles indicates vertical movement of PyC between soil layers (Guggenberger et al., 2008), pointing toward transport of Py-DOC within soil profiles. In many high-latitude systems, the highest PyC content within soils is associated with soil mineral layers (Rodionov et al., 2006; Guggenberger et al., 2008). In permafrost regions, DOM export is controlled by active layer thickness and extent of seasonally thawed mineral layers above permafrost regimes (Kawahigashi et al., 2004). These relationships within soils may affect both the phase and timing of PyC export to aquatic systems. Additionally, these processes may govern the turnover time of soil PyC, which is generally <100 years in boreal ecosystems (Hammes et al., 2008). This is much less than the millennial time-scales traditionally thought for PyC turnover in soils, and accounts for all loss processes (decomposition, leaching, erosion). Therefore, the translocation of PyC from soils into riverine systems is likely an important process for PyC cycling.
Export of high-temperature dissolved PyC (dissolved PyC will be henceforth referred to as Py-DOC; high temperature Py-DOC is also known as dissolved black carbon, or DBC) to aquatic systems does not appear to be controlled by fire history (Ding et al., 2013; Wagner et al., 2015), implying that high-temperature PyC needs to be functionalized before export, i.e., the solubility of high-temperature PyC is likely a function of charcoal age and exposure to “weathering” processes (Abiven et al., 2011). This idea is supported by the continual mobilization and export of high-temperature Py-DOC in deforested ecosystems long after slash-and-burn agriculture has stopped (Dittmar et al., 2012). However, as low-temperature Py-DOC also appears to be non-correlated to recent fire history (Myers-Pigg et al., 2015), the processes controlling the export of low- and high-temperature Py-DOC may be coupled. The relationship between Py-DOC from throughout the continuum and bulk DOC in Arctic river systems (Jaffe et al., 2013; Myers-Pigg et al., 2015; Stubbins et al., 2015) indicates that the mechanisms governing their export are similar. Therefore, it is likely that PyC export is controlled by similar processes as bulk organic matter. However, the temporal variability in PyC mobilization, as well as the variability of PyC export from the entire PyC continuum needs to be further explored before final conclusions on overall PyC export characteristics can be drawn. The export from terrestrial to aquatic systems is important to quantify in order to understand the impacts of PyC on carbon cycling (Masiello and Louchouarn, 2013).
Recent studies have documented the transfer of PyC to river systems in the dissolved and particulate phases, originating from all portions of the PyC continuum, from highly resistant PyC to more soluble, labile components (Dittmar et al., 2012; Jaffe et al., 2013; Myers-Pigg et al., 2015; Wagner et al., 2015; Wang et al., 2016). Understanding the transfer of PyC to aquatic systems is especially pertinent for Arctic regions, given the projected increase in frequency and intensity of boreal forest fires (Kasischke et al., 1995; Running, 2006; Soja et al., 2007) and a larger proportion of PyC created in these environments (Santín et al., 2015). The phase in which these materials are mobilized throughout the environment has implications on its availability for in-situ degradation/transformation, and ability for PyC to be stored in long-term carbon pools (Czimczik and Masiello, 2007; Santín et al., 2016). For example, PyC in the dissolved phase (Py-DOC) can be quickly remineralized (Stubbins et al., 2012; Ward et al., 2014; Myers-Pigg et al., 2015), while PyC in the particulate phase (Py-POC) may be buried in intermediate carbon pools along transport paths (such as river sediments) or on continental margins (Hunsinger et al., 2008; Cotrufo et al., 2016). Given the high potential for biological degradation of levoglucosan and other low-temperature PyC on environmentally relevant time scales (Norwood et al., 2013), one might expect that low-temperature PyC produced in the environment is quickly degraded in-situ during transport from terrestrial systems. However, a few studies have shown that biomarkers of low-temperature biomass combustion by-products (levoglucosan and isomers) can be found in sedimentary and soil records 100's–1,000's of years old and help trace fire events induced by climate cyclicity and/or anthropogenic activities (Elias et al., 2001; Hunsinger et al., 2008; Kuo et al., 2011b; Kirchgeorg et al., 2014; Gao et al., 2016). Chemical functionality of low-temperature PyC should favor mobilization and transport in the aqueous phase and therefore, preservation of low-temperature PyC and deposition into sedimentary records may be a function of source and/or associations of low-temperature PyC with minerals or high-temperature PyC components.
Here, we present the first study to our knowledge that analyzes the phase distribution of low-temperature PyC, using levoglucosan as a biomarker proxy, in two high-latitude systems: a small sub-Arctic Canadian River, the Great Whale River, in northern Québec, and the largest Arctic River, the Yenisei River, in north-central Siberia throughout various flow regimes, to discover the evolution and relative importance of phase distribution on the export of low-temperature PyC in these high-latitude systems.
Materials and Methods
For each river, we collected particulate and dissolved organic matter across the spring freshet period. Approximately 100 L of river water was pre-filtered for gross particulates (64 μm) in the field and transported to a field laboratory station in either in the village of Whapmagoostui-Kuujjuarapik for the Great Whale River (2012), or Igarka for the Yenisei River (2014), and immediately filtered using tangential flow filtration (TFF, 0.45 μm filter size) to separate the particulate and dissolved/colloidal phases. The particulate portion, 64–0.45 μm, was concentrated to around 1 litter and immediately frozen. The dissolved portion, <0.45 μm, was isolated using a portable reverse osmosis system (Serkiz and Perdue, 1990), and the concentrate was also frozen. Samples were then transported to the Laboratoire d'Écologie Fonctionnelle et Environnement (EcoLab) laboratory in Toulouse, France, for further treatment. The samples were all freeze-dried at EcoLab, France, and chemical analyses were performed at Texas A&M University at Galveston, USA.
Percent OC (% OC) was determined on vapor-phase acidified (to remove carbonates) freeze-dried subsamples on a Costech Elemental Analyzer. Freeze-dried subsamples were then analyzed for fire-derived biomarkers, monomeric lignin phenols (syringyl, cinnamyl, and vanillyl phenols) and anhydrosugars (levoglucosan, mannosan, galactosan), following methods outlined in Louchouarn et al. (2009), Shakya et al. (2011), and Myers-Pigg et al. (2015). Briefly, biomarkers were extracted from freeze-dried materials, pre-spiked with deuterated (d-7) levoglucosan (NIST SRM 2267), using 9:1 dichloromethane:methanol on an accelerated solvent extractor (ASE 200; Dionex) at 1500 PSI and 100°C. Sample were then concentrated in a RapidVap (LabConco) under Argon gas at 50°C, dried to completion in a CentriVap centrifugal concentrator (LabConco) at 50°C, and re-suspended in pyridine. Extracts were then derivatized for 15 min using O-bis (trimethylsilyl) trifluoroacetamide containing 1% trimethylchlorosilane (9:1 BSTFA:TMCS) at 75°C, and analyzed on a Varian triple quadrupole 480-300 GC-MS system using a fused silica column (J&W DB-5MS, 30 m × 0.25 mm i.d., 0.25 μm film thickness; Agilent Technologies). Each sample was injected splitless using helium as a carrier gas. Monomeric lignin phenol and anhydrosugar analyses were performed independently under single ion monitoring (SIM) mode, and using specific ions for compound determination. Sample detection limits, recoveries, and precision were determined through concurrently running blanks, standard reference materials (SRM 1649b), and replicates. All quality control parameters were within the ranges presented in Louchouarn et al. (2009).
Ratios of monomeric lignin phenols (syringyl/vanillyl: S/V) and anhydrosugars (levoglucosan to its isomer mannosan: L/M) were calculated to determine sources of PyC in the dissolved and particulate phases (Table 1; Kuo et al., 2008a; Myers-Pigg et al., 2015).
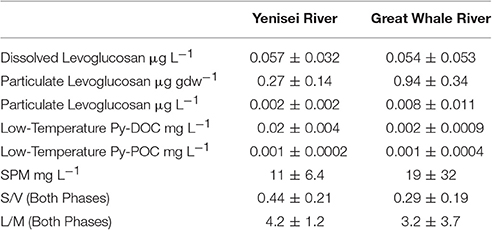
Table 1. Average levoglucosan concentrations, low-temperature PyC concentrations, suspended particulate matter (SPM), and source signature ratios from free lignin phenols and anhydrosugars (syringyl/vanillyl: S/V and levoglucosan/mannosan: L/M) for the Yenisei River and Great Whale River.
Low-temperature Py-DOC concentrations (Table 1) were calculated according to the methodology outlined in Myers-Pigg et al. (2015), using measured levoglucosan concentrations and a conversion factor based on the ratio of levoglucosan to low-temperature Py-DOC potentially present at the sampling site. Briefly, transit times were calculated between sample locations and average fire locations in each watershed to account for degradation during transit. The experimentally-derived ratio of levoglucosan to Py-DOC (Norwood et al., 2013), corresponding to each calculated transit time, was used to convert measured levoglucosan concentrations into an estimate of low-temperature Py-DOC in the rivers (Myers-Pigg et al., 2015). Fire locations were assumed to be concentrated at ~52–53°N for the Yenisei River (Myers-Pigg et al., 2015; Ponomarev et al., 2016); the range of fire locations from 1980 to 2014 using fire data from the Canadian Forest Service (2016) were used for the Great Whale River (Figure S1). Error of fire location for each sampling point was propagated for the Py-DOC and Py-POC calculations (Figure 3).
To estimate the concentrations of low-temperature Py-POC in each river, mass-weighted distribution coefficients (Kds; Table 2) were calculated by the following equation:
where the concentrations of levoglucosan (l) is measured in the isolated particulate and dissolved matter from each river (Figure 2; Table 2). Low-temperature Py-POC concentrations were then calculated using Kd values for levoglucosan calculated in Equation 1, substituting Py-DOC concentrations for l in DOM in Equation 1 and solving for the concentration of Py-POC in mg kg−1. These concentrations were then scaled to mg L−1 using total suspended solids (TSS; suspended particulate material, SPM) measured in mg L−1 (Table 1).
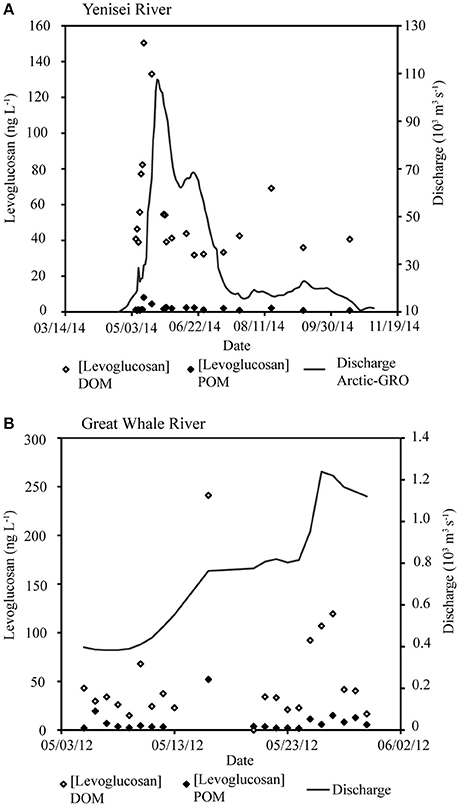
Figure 2. (A) Levoglucosan concentrations throughout the freshet hydrograph in the Yenisei River in 2014, (B) the Great Whale River in 2012.
Fluxes of Py-DOC and Py-POC were calculated by scaling Py-DOC and Py-POC concentrations to discharge in each river (Figure 3). The percentage of flux in Py-DOC vs. in Py-POC was calculated by dividing the Py-POC flux calculated at a specific time point by the sum of Py-DOC and Py-POC fluxes at the same time point, multiplied by 100%(Table 3).
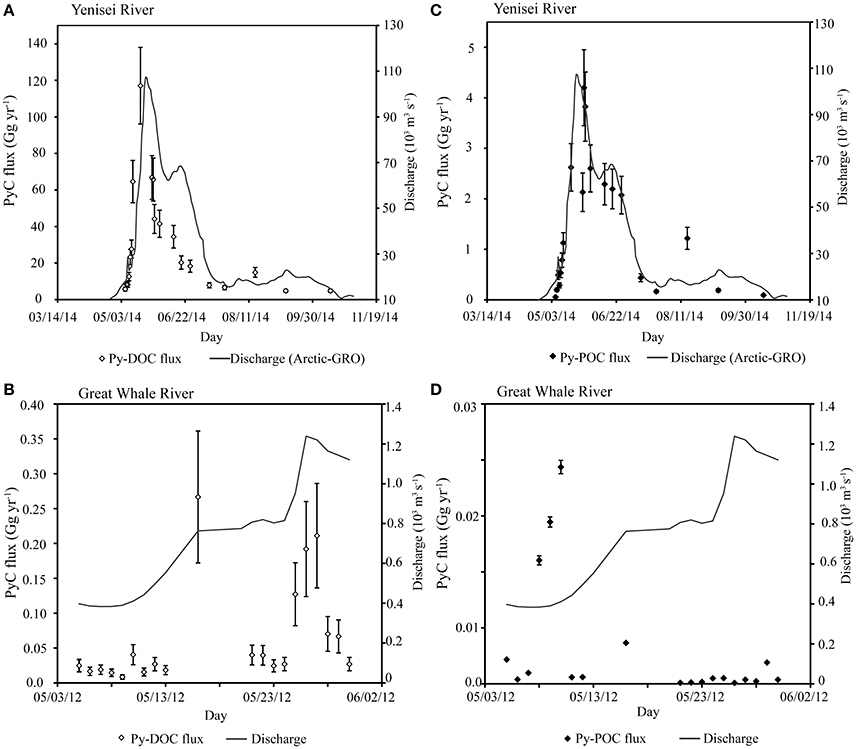
Figure 3. PyC fluxes with discharge. (A) Py-DOC fluxes with discharge Yenisei River in 2014 (B) Py-DOC fluxes with discharge Great Whale River in 2012 (C) Py-POC fluxes with discharge Yenisei River in 2014 (D) Py-POC fluxes with discharge Great Whale River in 2012.
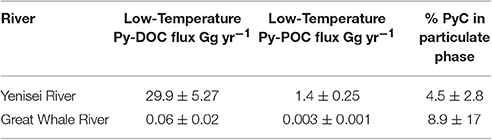
Table 3. Average yearly flux estimates for Py-DOC and Py-POC, and average % PyC in the particulate phase for the Great Whale River and the Yenisei River.
Low-temperature PyC phase distribution in these systems were also compared to high-temperature PyC phase distribution in other systems (Table 2), using concentrations of low- and high-temperature PyC biomarkers to calculate distribution coefficients as in Equation 1. These distribution coefficients are a reflection of the environmental distribution of PyC and, therefore, reflect all factors influencing this phase distribution, including source, association with minerals, and particle physical properties (such as surface area, porosity, number of reactive sites, etc.).
Results
Arctic River
Levoglucosan concentrations in the dissolved and particulate phase increase with increasing discharge (Figure 2). Particulate organic carbon (POC) in the Yenisei River was 0.08 ± 0.02 kg OC kg−1 throughout the sampling period. Monomeric lignin phenol ratios (syringyl/vanillyl: S/V) are 0.44 ± 0.21 in the Yenisei River (Table 1), and the ratios of levoglucosan to its isomer mannosan (L/M) are 4.2 ± 1.2 (Table 1).
The timing of peak export of low-temperature Py-DOC and Py-POC were decoupled in the Yenisei River throughout the freshet period (Figure 3). Low-temperature Py-DOC peaks during the rising limb of the hydrograph, while low-temperature Py-POC peaks during the recession limb for the Yenisei River (Figure 3). LogKd values, the measure of environmental phase distribution, for levoglucosan were nearly one order of magnitude lower during peak flow in the Yenisei River (Figure S2) than during base flow, indicating a higher proportion of total PyC in the dissolved phase during high flow regimes. LogKd values of levoglucosan are within the same range as estimated logKd values for higher temperature PyC markers (BPCA) in rivers (Table 2 and references therein).
Sub-Arctic River
POC in the Great Whale River was 0.17 ± 0.08 kg OC kg−1 throughout the sampling period. Monomeric lignin phenol ratios S/V are 0.29 ± 0.19 and L/M ratios are 3.2 ± 3.7 (Table 1). In the Great Whale River, the flux of levoglucosan and low-temperature Py-DOC increased with increasing discharge, while the flux of low-temperature Py-POC decreased with increasing discharge (Figures 2, 3). LogKd values decreased with increasing discharge (Figure S2).
Discussion
Characterization of Organic Matter and Pyrogenic Carbon
Free monomeric lignin phenol ratios in both phases (syringyl/vanillyl: S/V), coupled with levoglucosan and its isomer, mannosan (L/M), are within the range of values reported for gymnosperm source inputs in charcoals, aerosols, and soils, suggesting that low-temperature PyC in the Yenisei River and the Great Whale River in both phases are influenced predominantly by gymnosperm fire fuels (Table 1, source ratios from Kuo et al., 2011a). These ratios are consistent with previously reported source reconstructions for Py-DOC in Arctic Rivers (Myers-Pigg et al., 2015).
Absolute concentrations of levoglucosan in the dissolved phase are on the lower end of the range previously reported in river DOM. For the Yenisei River, concentrations in this study are 0.06 ± 0.04 μg L−1 vs. 0.8 ± 0.5 μg L−1 from 2004 to 2006 (Myers-Pigg et al., 2015). Differences in the observed concentrations may be dependent on interannual variability in the mechanisms that govern export of PyC, such as hydrological flow regimes. However, further research must be done to ascertain the varying importance of the mechanisms that control PyC export to and through aquatic systems. Concentrations of levoglucosan in the particulate phase of the studied rivers are also low (Figure 2; Table 2, 0.73 ± 1.1 μg gdw−1) compared to concentrations of levoglucosan in surface sediments from a river plume (1.3–6.9 μg gdw−1) of a small, mountainous stream (Hunsinger et al., 2008). It should be noted that erosion, resuspension, and deposition of PyC along transport is particularly high in mountainous systems (Cotrufo et al., 2016). This may affect the total amount of PyC transported in the particulate phase to estuarine systems compared to the relatively larger river systems in this study, which also have relatively smaller proportions of total carbon exported in the particulate phase.
Differences in Phase Distribution throughout the Hydrograph
In watersheds that contain permafrost coverage (such as the watersheds in this study), the permafrost can act as a boundary layer, causing rapid translocation of water to river systems during spring thaw events, which influences SOM transport to rivers. The depth of the soil active layer, in which organic matter has the ability to exchange with the surface terrestrial ecosystem, though generally quite shallow in these regions, tends to vary based on location within a basin. In a subwatershed of the Yenisei River, storage of PyC is largest in areas with permafrost and shallow active layers, whereas mineral soils with thick active layer or lacking permafrost stored less PyC, suggesting increased mobility of PyC from the latter (Guggenberger et al., 2008). The same study showed that most of the export of PyC occurs during the period of snowmelt with dominance of surface flow pointing to a strong relationship between PyC concentrations and hydrological regime. This may help to explain the observed lack of relationship between fire frequency and riverine export of dissolved black carbon (DBC as determined by BPCAs; Ding et al., 2013, 2014), and the strong reported relationships between DBC and hydrological cycling (Jaffe et al., 2013), particularly in Arctic systems (Stubbins et al., 2015).
Here, low-temperature Py-DOC peaks during the rising limb of the hydrograph in the Yenisei River (Figure 3A). This early peak of low-temperature Py-DOC is not mirrored in bulk DOC concentrations, which increase linearly with discharge (R2 = 0.82, p < 0.001; graph not shown). This apparently “early” release of Py-DOC has not been previously observed, as Py-DOC from both high and low-temperature portions of the continuum have been previously correlated to discharge and DOC in these and other systems (Jaffe et al., 2013; Myers-Pigg et al., 2015; Stubbins et al., 2015). However, here we present the first dataset with targeted high resolution sampling during spring peak flow. Hence, the decoupling of Py-DOC and DOC during the rising limb may be a phenomenon not previously captured. During the rest of the hydrograph (low flow, peak flow, and the falling limb) low-temperature Py-DOC is correlated to bulk DOC (R2 = 0.63, p < 0.001), as has been previously observed. Therefore, further high-resolution sampling is needed to understand Py-DOC dynamics during the early part of the spring freshet period in other years to determine if the periodic decoupling of Py-DOC and DOC is systematic or only an isolated case. This decoupling of low-temperature Py-DOC and DOC in the rising limb of the hydrograph may be linked to the mobilization of a different source of Py-DOC during this period than during other flow regimes.
Although very little research has been done on the transport of particulate PyC from river systems, it has been suggested that transport in this phase may be of importance in recently fire-affected ecosystems (Wagner et al., 2015), where increased surface run off (Moody et al., 2013), soil erosion (Shakesby and Doerr, 2006), and increases in TSS (Ryan et al., 2011) have been observed. In the study regions, particulate carbon export is often a small proportion of the total carbon export; POC export accounts for ~15% of the total OC flux from the pan-Arctic per year (Holmes et al., 2012; McClelland et al., 2016). Around 23% of the total POC export occurs during the spring freshet period in both the Great Whale River and Yenisei River (Hudon et al., 1996; McClelland et al., 2016), making high-resolution sampling important to capture temporal variability in the Py-POC flux. As previous work estimates that Py-POC export in Arctic Rivers is between 3 and 9% of total POC fluxes (Elmquist et al., 2008), transport of Py-POC may be an important portion of the total POC in Arctic Rivers. Here, we find that low-temperature Py-POC comprises 4.5 ± 2.8% of the low-temperature PyC flux from the Yenisei River (Figure 3; Table 3). These relatively lower levels of export of low-temperature Py-POC in our systems has implications for the usage of levoglucosan to track fire activity in depositional records, as low-temperature PyC determined from levoglucosan is exported predominantly in the dissolved phase. In the Yenisei River, low-temperature Py-POC export peaks during the falling limb of the freshet hydrograph (Figure 3C), which may reflect varying sources of PyC between phases or variations in export mechanisms between the two phases. For example, the composition and bioavailability of OM exported from soils in high-latitude systems is a function of active layer depth and mineral soil association (Kawahigashi et al., 2006). Terrestrial organic carbon (as determined by n-alkanoic acids) in the particulate phase has been found to associate with two distinct pools of soil carbon released during the spring freshet period; a young pool dominated by humics and an old pool with a mineral soil component (Vonk et al., 2010). This observed difference in mobilization of terrestrial OC may also influence the mobilization of PyC in high-latitude systems, which in turn, affects availability for transformations during transport, as a large portion of labile materials, if associated with a mineral layer, may be protected from biodegradation during transport (Hedges et al., 1997; Kaiser and Guggenberger, 2000; Vonk et al., 2010). During the freshet period, low-temperature PyC is proportionally exported more in the dissolved phase than during low-flow (Figure 3; Figure S2). This may be due to hydrophilic DOM, such as low-temperature PyC (chemical functionality in Figure 1), bypassing association with soils within the active layer, and directly entering the aquatic system (Kawahigashi et al., 2006) during high-flow regimes. Because high-flow regimes release the largest proportion of yearly DOM and POM during a relatively short time period (Amon et al., 2012; Holmes et al., 2012; McClelland et al., 2016), these flow regimes are similarly expected to contribute substantially to the overall flux of PyC from high-latitude rivers. Additionally, since PyC is predominantly released in the dissolved phase, this may allow highly functionalized and hydrophilic portions of PyC to be more accessible to in-situ microbial degradation and lead to increased riverine respiration rates during this period (Masiello and Louchouarn, 2013).
In the Great Whale River, physical protection of PyC may regulate its release from soils. Only around 10% of the total DOC is released in this river system during the ice break-up period (Hudon et al., 1996), as opposed to almost half of the DOC released from the major Arctic Rivers during the spring freshet period (Holmes et al., 2012). This observation suggests that the timing and release of organic matter from small southern boreal watersheds are driven by different controls than those operating in the major Arctic Rivers. The flux of organic matter may be normalized in these latter systems by the size of the watersheds, whereas soil heterogeneities in smaller southern boreal watersheds may show a strong influence of soil processes. For example, in southern boreal forests, accumulation of large, long-lived stocks of PyC have been observed in mineral horizons with little to no soil profile development (Miesel et al., 2015). The opposite has been reported in boreal forests containing mineral soils underlying thick active layers (Guggenberger et al., 2008). The Great Whale River watershed is itself highly heterogeneous and contains a variety of soil types including discontinuous permafrost (Bhiry et al., 2011). However, and in conjunction with high fire return intervals, the export of PyC from this watershed may be more regulated by soil processes than hydrological flow regimes, as highlighted by the lack of a clear relationship of PyC export with hydrography (Table 3; Figure 3).
Implications for the Entire Pyrogenic Carbon Continuum
The similarity between phase distribution calculated for high-temperature PyC components (BPCA and PAHs) and low-temperature PyC (levoglucosan) suggest that low- and high-temperature PyC may be exported similarly from river systems (Table 2). However, as physical weathering has been shown to be an important loss process for both high- and low-temperature charcoals, although on very different time scales (Naisse et al., 2015), understanding the ages of these exported carbon pools is necessary before final conclusions on their similarities can be determined. Additionally, given the fact that bulk DOC and POC in Arctic River systems have vastly different ages (e.g., Goñi et al., 2005; Amon et al., 2012), we must understand the sources and ages of dissolved and particulate PyC in order to fully understand how export of Py-DOC and Py-POC might be linked both temporally and throughout the PyC continuum. For example, high-temperature PyC (as determined by the CTO-375 method; PyC range covered by the method in Figure 1) has been found to contain two distinct sources between the particulate and dissolved phases in river systems (Wang et al., 2016), and much of this high-temperature PyC can be derived from fossil fuels (up to 33% of the DBC and around 50% of the PBC). In contrast, the sources of low-temperature PyC (derived from biomass burning) using biomarker source ratios appear to be similar in our studied rivers (S/V and L/M; suggesting gymnosperm sources in both phases). Therefore, it may become important to characterize potential variability of PyC generation during biomass burning and its effects on PyC mobilization. Isotopic approaches may help identify some of this variability (e.g., isotopic fractionation of levoglucosan occurs during atmospheric processes; Sang et al., 2016).
The apparent order of magnitude increase in the ratio of Py-DOC to Py-POC for high-temperature PyC from rivers to oceans (Table 2; calculated from Ziolkowski and Druffel, 2010; Coppola et al., 2014; Wagner et al., 2015) suggests that Py-POC plays an increasingly important role in PyC cycling through transport from riverine to oceanic systems. This may be due to the potential degradation of dissolved PyC during transit (Stubbins et al., 2012; Masiello and Louchouarn, 2013; Ward et al., 2014; Myers-Pigg et al., 2015) and/or the importance sorption of Py-DOC to Py-POC in marine systems (Coppola et al., 2014). Sorption processes and transfer to sediments are considered as one of the major loss processes for Py-DOC in the world's ocean (Coppola et al., 2014). Py-DOC exported from Arctic Rivers could sorb to sinking particulates once it enters the Arctic Ocean. This has implications on how much Py-DOC may survive transport and end up in regions of North Atlantic Deep Water formation (Stubbins et al., 2015), vs. what is sorbed to sinking particles and buried on the vast Arctic continental shelves. Measures of Py-POC in Arctic Ocean sediments and sinking particles (using CTO-375 method) suggest that Py-POC quickly sinks and is stored within coastal margins (Fang et al., 2016). To understand loss terms and PyC cycling, the relative proportion of all component of the PyC continuum in the dissolved and particulate phases during transfer to and within ocean systems must be further explored.
Flux Estimates from Rivers for Arctic Pyrogenic Carbon
The percentage of the total PyC flux from these rivers in the particulate phase varies dramatically throughout the hydrograph. During low-flow stages (<2 × 104 m3 s −1), low-temperature Py-POC was 3.1 ± 2.3% of the total PyC flux in the Yenisei River, while during high flow low-temperature Py-POC was 5.1 ± 2.8% of this flux. This is much less than a calculated distribution of Py-POC in worldwide flux estimates (~16% of total PyC flux from rivers; Santín et al., 2016). This is also lower than previous estimates of Py-POC fluxes from the pan-Arctic (~8% of total PyC flux) using pan-Arctic Py-POC flux estimates calculated using BC/POC ratios from Elmquist et al. (2008) and pan-Arctic POC fluxes from McClelland et al. (2016) and pan-Arctic Py-DOC fluxes from Stubbins et al. (2015). However, as our estimates measure in-situ phase distribution based on biomarker composition within rivers, they can represent a conservative estimate of the amount of Py-POC exported from the studied Arctic Rivers. Additionally, previous Py-POC flux estimates from Arctic Rivers rely on measurements of the most refractory PyC (using the CTO-375 method; see Figure 1 for range of detection within the PyC continuum) in estuarine sediments to estimate in-situ Py-POC fluxes (see Elmquist et al. (2008) for further explanation of previous Py-POC flux calculations). As refractory PyC analytical methods (such as CTO-375 method) may overestimate PyC contribution in environmental samples (Hammes et al., 2007), higher temperature PyC can be derived from both fossil fuel combustion and biomass burning, and the most refractory portion of the PyC continuum measured by these techniques may be more particle associated than other forms of PyC, the previously reported flux estimates may overestimate the actual distribution of PyC transported from Arctic Rivers. In environments where POC export is a proportionally important part of the TOC flux, the relative influence of Py-POC on the total export of PyC from the region may be of higher significance. For example, in the Great Whale River, total POC export accounts 18.9% of the total OC export (Hudon et al., 1996), slightly higher than the average Arctic River system (~15%; Holmes et al., 2012; McClelland et al., 2016). In this river system, Py-POC was 8.9 ± 17.5% of the total PyC flux (Figure 3; Table 3). The export of low-temperature PyC varied with the hydrograph (Figure 3), implying that the export of PyC in the particulate phase varies throughout the year. This is important to consider when looking at PyC in deposited sedimentary records and estimating the impact of fire on past climate, as the records of these signatures may vary with hydrographic regimes and particulate matter loadings.
Considering the changing fire regimes in the Arctic (Kelly et al., 2015), the intrinsic tie of Py-DOC with discharge in the region (Myers-Pigg et al., 2015; Stubbins et al., 2015), and changing hydrological regimes (Wrona et al., 2016), the transport of PyC from Arctic terrestrial to aquatic systems is likely to increase. As the Arctic Ocean continental margins have recently been suggested as an efficient location for PyC burial (Fang et al., 2016), understanding the phase distribution of PyC during transport through rivers and estuaries is relevant for understanding overall PyC fluxes, the potential degradation of PyC during transit, and losses of PyC from the active carbon cycle through burial into sediments.
Author Contributions
All authors contributed to experimental design for this study. RT and AM collected and processed the samples. AM and PL interpreted the data and prepared the manuscript. All authors participated in revisions and approved the final manuscript.
Funding
This project was funded under the TOMCAR-Permafrost Marie Curie International Reintegration Grant FP7-PEOPLE-2010-RG (project reference: 277059) within the Seventh European Community Framework Programme awarded to RT (http://www.tomcar.fr). Yenisei River daily discharge data was provided by the Arctic Great Rivers Observatory (NSF-1107774). Travel and living expenses were also funded thanks to GDRI Car-Wet-Sib II and INP-Toulouse SMI program.
Conflict of Interest Statement
The authors declare that the research was conducted in the absence of any commercial or financial relationships that could be construed as a potential conflict of interest.
Acknowledgments
We thank the Chateaubriand Fellowship Program led by the Office for Science and Technology of the Embassy of France in the United States, which provided funding for AM to spend 6 months at EcoLab in France. We thank Nikita Tananaev for his assistance while in Igarka, including sampling, coordination and use of field facilities. The Melnikov Permafrost Institute is thanked for their hospitality and support of this research. Sample collection and transport could not have been possible without the hard work of countless amazing people, including those in Toulouse at EcoLab, the team at ULISSE, Elena Fedorova, the entire Geocryology Laboratory team in Igarka, and particularly Anatolii Pimov. We thank R. Amon, K. Kaiser, and M. Norwood for helpful comments on earlier versions of this manuscript. Figure S1 was created with assistance from M. Norwood. We also thank two reviewers for their constructive and helpful comments on this manuscript. Open access publication fees were supported in part by the Gordon and Betty Moore Foundation.
Supplementary Material
The Supplementary Material for this article can be found online at: http://journal.frontiersin.org/article/10.3389/fmars.2017.00038/full#supplementary-material
References
Abiven, S., Hengartner, P., Schneider, M. P. W., Singh, N., and Schmidt, M. W. I. (2011). Pyrogenic carbon soluble fraction is larger and more aromatic in aged charcoal than in fresh charcoal. Soil Biol. Biochem. 43, 1615–1617. doi: 10.1016/j.soilbio.2011.03.027
Amon, R. M. W., Rinehart, A. J., Duan, S., Louchouarn, P., Prokushkin, A., Guggenberger, G., et al. (2012). Dissolved organic matter sources in large Arctic Rivers. Geochim. Cosmochim. Acta 94, 217–237. doi: 10.1016/j.gca.2012.07.015
Ascough, P., Bird, M., Francis, S., Thornton, B., Midwood, A., Scott, A., et al. (2011). Variability in oxidative degradation of charcoal: influence of production conditions and environmental exposure. Geochim. Cosmochim. Acta 75, 2361–2378. doi: 10.1016/j.gca.2011.02.002
Bhiry, N., Delwaide, A., Allard, M., Bégin, Y., Filion, L., Lavoie, M., et al. (2011). Environmental change in the great whale river region, Hudson Bay: five decades of multidisciplinary research by Centre d'études Nordiques (CEN). Ecoscience 18, 182–203. doi: 10.2980/18-3-3469
Brandes, J. A., Cody, G. D., Rumble, D., Haberstroh, P., Wirick, S., and Gelinas, Y. (2008). Carbon K-edge XANES spectromicroscopy of natural graphite. Carbon 46, 1424–1434. doi: 10.1016/j.carbon.2008.06.020
Canadian Forest Service (2016). National Fire Database — Agency Fire Data. Natural Resources Canada, Canadian Forest Service, Northern Forestry Centre, Edmonton, AB. Available online at: http://cwfis.cfs.nrcan.gc.ca/ha/nfdb
Coppola, A. I, Ziolkowski, L. A., Masiello, C. A., and Druffel, E. R. M. (2014). Aged black carbon in marine sediments and sinking particles. Geophys. Res. Lett. 41, 2427–2433. doi: 10.1002/2013GL059068
Cotrufo, M. F., Boot, C. M., Kampf, S., Nelson, P. A., Brogan, D. J., Covino, T., et al. (2016). Redistribution of pyrogenic carbon from hillslopes to stream corridors following a large montane wildfire. Global Biogeochem. Cycles 30, 1348–1355. doi: 10.1002/2016GB005467
Czimczik, C. I, and Masiello, C. A. (2007). Controls on black carbon storage in soils. Global Biogeochem. Cycles 21:GB3005. doi: 10.1029/2006GB002798
Ding, Y., Watanabe, A., and Jaffé, R. (2014). Dissolved black nitrogen (DBN) in freshwater environments. Org. Geochem. 68, 1–4. doi: 10.1016/j.orggeochem.2013.12.009
Ding, Y., Yamashita, Y., Dodds, W. K., and Jaffé, R. (2013). Dissolved black carbon in grassland streams: is there an effect of recent fire history? Chemosphere 90, 2557–2562. doi: 10.1016/j.chemosphere.2012.10.098
Dittmar, T., de Rezende, C. E., Manecki, M., Niggemann, J., Ovalle, A. R. C., Stubbins, A., et al. (2012). Continuous flux of dissolved black carbon from a vanished tropical forest biome. Nat. Geosci. 5, 618–622. doi: 10.1038/ngeo1541
Elias, V. O., Simoneit, B. R. T., Cordeiro, R. C., and Turcq, B. (2001). Evaluating levoglucosan as an indicator of biomass burning in Carajas, Amazonia: a comparison to the charcoal record. Geochim. Cosmochim. Acta 65, 267–272. doi: 10.1016/S0016-7037(00)00522-6
Elmquist, M., Semiletov, I., Guo, L., and Gustafsson, Ö. (2008). Pan-Arctic patterns in black carbon sources and fluvial discharges deduced from radiocarbon and PAH source apportionment markers in estuarine surface sediments. Global Biogeochem. Cycles 22:GB2018. doi: 10.1029/2007GB002994
Fang, Z., Yang, W., Chen, M., Zheng, M., and Hu, W. (2016). Abundance and sinking of particulate black carbon in the western Arctic and Subarctic Oceans. Sci. Rep. 6:29959. doi: 10.1038/srep29959
Gao, X., Norwood, M., Frederick, C., McKee, A., Masiello, C. A., and Louchouarn, P. (2016). Organic geochemical approaches to identifying formation processes for middens and charcoal-rich features. Org. Geochem. 94, 1–11. doi: 10.1016/j.orggeochem.2016.01.007
Goñi, M. A., Yunker, M. B., Macdonald, R. W., and Eglinton, T. I (2005). The supply and preservation of ancient and modern components of organic carbon in the Canadian Beaufort Shelf of the Arctic Ocean. Mar. Chem. 93, 53–73. doi: 10.1016/j.marchem.2004.08.001
Güereña, D. T., Lehmann, J., Walter, T., Enders, A., Neufeldt, H., Odiwour, H., et al. (2015). Terrestrial pyrogenic carbon export to fluvial ecosystems: lessons learned from the white Nile watershed of East Africa. Global Biogeochem. Cycles 29, 1911–1928. doi: 10.1002/2015GB005095
Guggenberger, G., Rodionov, A., Shibistova, O., Grabe, M., Kasansky, O. A., Fuchs, H., et al. (2008). Storage and mobility of black carbon in permafrost soils of the forest tundra ecotone in Northern Siberia. Glob. Chang. Biol. 14, 1367–1381. doi: 10.1111/j.1365-2486.2008.01568.x
Hammes, K., Schmidt, M. W. I, Smernik, R. J., Currie, L. A., Ball, W. P., Nguyen, T. H., et al. (2007). Comparison of quantification methods to measure fire-derived (black/elemental) carbon in soils and sediments using reference materials from soil, water, sediment and the atmosphere. Global Biogeochem. Cycles 21:GB3016. doi: 10.1029/2006GB002914
Hammes, K., Torn, M. S., Lapenas, A. G., and Schmidt, M. W. I (2008). Centennial black carbon turnover observed in a Russian steppe soil. Biogeosciences 5, 1339–1350. doi: 10.5194/bg-5-1339-2008
Hansen, M. C., Potapov, P. V, Moore, R., Hancher, M., Turubanova, S. A., Tyukavina, A., et al. (2013). High-resolution global maps of 21st-century forest cover change. Science 342, 850–853. doi: 10.1126/science.1244693
Harvey, O. R., Kuo, L. J., Zimmerman, A. R., Louchouarn, P., Amonette, J. E., and Herbert, B. E. (2012). An index-based approach to assessing recalcitrance and soil carbon sequestration potential of engineered black carbons (biochars). Environ. Sci. Technol. 46, 1415–1421. doi: 10.1021/es2040398
Hedges, J. I, Keil, R. G., and Benner, R. (1997). What happens to terrestrial organic matter in the ocean? Org. Geochem. 25, 195–212. doi: 10.1016/S0146-6380(97)00066-1
Hockaday, W. C., Grannas, A. M., Kim, S., and Hatcher, P. G. (2007). The transformation and mobility of charcoal in a fire-impacted watershed. Geochim. Cosmochim. Acta 71, 3432–3445. doi: 10.1016/j.gca.2007.02.023
Holmes, R. M., McClelland, J. W., Peterson, B. J., Tank, S. E., Bulygina, E., Eglinton, T. I, et al. (2012). Seasonal and annual fluxes of nutrients and organic matter from large rivers to the Arctic ocean and surrounding seas. Estuaries Coasts 35, 369–382. doi: 10.1007/s12237-011-9386-6
Hudon, C., Morin, R., Bunch, J., and Harland, R. (1996). Carbon and nutrient output from the great Whale river (Hudson Bay) and a comparison with other rivers around Quebec. Can. J. Fish. Aquat. Sci. 53, 1513–1525. doi: 10.1139/f96-080
Hunsinger, G. B., Mitra, S., Warrick, J. A., and Alexander, C. R. (2008). Oceanic loading of wildfire-derived organic compounds from a small mountainous river. J. Geophys. Res. Biogeosci. 113:G02007. doi: 10.1029/2007JG000476
Jaffe, R., Ding, Y., Niggemann, J., Vahatalo, A. V, Stubbins, A., Spencer, R. G., et al. (2013). Global charcoal mobilization from soils via dissolution and riverine transport to the oceans. Science 340, 345–347. doi: 10.1126/science.1231476
Kaiser, K., and Guggenberger, G. (2000). The role of DOM sorption to mineral surfaces in the preservation of organic matter in soils. Org. Geochem. 31, 711–725. doi: 10.1016/S0146-6380(00)00046-2
Kasischke, E. S., Christensen, N. L., and Stocks, B. J. (1995). Fire, global warming and the carbon balance of boreal forests. Ecol. Appl. 5, 437–451. doi: 10.2307/1942034
Kawahigashi, M., Kaiser, K., Kalbitz, K., Rodionov, A., and Guggenberger, G. (2004). Dissolved organic matter in small streams along a gradient from discontinuous to continuous permafrost. Glob. Chang. Biol. 10, 1576–1586. doi: 10.1111/j.1365-2486.2004.00827.x
Kawahigashi, M., Kaiser, K., Rodionov, A., and Guggenberger, G. (2006). Sorption of dissolved organic matter by mineral soils of the Siberian forest tundra. Glob. Chang. Biol. 12, 1868–1877. doi: 10.1111/j.1365-2486.2006.01203.x
Keiluweit, M., Nico, P. S., Johnson, M. G., and Kleber, M. (2010). Dynamic molecular structure of plant biomass-derived black carbon (biochar). Environ. Sci. Technol. 44, 1247–1253. doi: 10.1021/es9031419
Kelly, R., Genet, H., McGuire, A. D., and Hu, F. S. (2015). Palaeodata-informed modelling of large carbon losses from recent burning of boreal forests. Nat. Clim. Chang. 6, 79–82. doi: 10.1038/nclimate2832
Kirchgeorg, T., Schupbach, S., Kehrwald, N., McWethy, D. B., and Barbante, C. (2014). Method for the determination of specific molecular markers of biomass burning in lake sediments. Org. Geochem. 71, 1–6. doi: 10.1016/j.orggeochem.2014.02.014
Knicker, H. (2011). Pyrogenic organic matter in soil: its origin and occurrence, its chemistry and survival in soil environments. Quaternary Int. 243, 251–263. doi: 10.1016/j.quaint.2011.02.037
Kuo, L.-J., Herbert, B. E., and Louchouarn, P. (2008a). Can levoglucosan be used to characterize and quantify char/charcoal black carbon in environmental media? Org. Geochem. 39, 1466–1478. doi: 10.1016/j.orggeochem.2008.04.026
Kuo, L.-J., Louchouarn, P., and Herbert, B. E. (2008b). Fate of CuO-derived lignin oxidation products during plant combustion: application to the evaluation of char input to soil organic matter. Org. Geochem. 39, 1522–1536. doi: 10.1016/j.orggeochem.2008.07.011
Kuo, L.-J., Louchouarn, P., and Herbert, B. E. (2011a). Influence of combustion conditions on yields of solvent-extractable anhydrosugars and lignin phenols in chars: implications for characterizations of biomass combustion residues. Chemosphere 85, 797–805. doi: 10.1016/j.chemosphere.2011.06.074
Kuo, L.-J., Louchouarn, P., Herbert, B. E., Brandenberger, J. M., Wade, T. L., and Crecelius, E. (2011b). Combustion-derived substances in deep basins of Puget Sound: historical inputs from fossil fuel and biomass combustion. Environ. Pollut. 159, 983–990. doi: 10.1016/j.envpol.2010.12.012
Lakshmanan, C. M., Gal-Or, B., and Hoelsche, H. E. (1970). Production of levoglucosan by pyrolysis of carbohydrates. Die Stärke 22, 221–227. doi: 10.1002/star.19700220703
Louchouarn, P., Kuo, L.-J., Wade, T. L., and Schantz, M. (2009). Determination of levoglucosan and its isomers in size fractions of aerosol standard reference materials. Atmos. Environ. 43, 5630–5636. doi: 10.1016/j.atmosenv.2009.07.040
Masiello, C. A. (2004). New directions in black carbon organic geochemistry. Mar. Chem. 92, 201–213. doi: 10.1016/j.marchem.2004.06.043
Masiello, C. A., and Louchouarn, P. (2013). Ecology. fire in the ocean. Science 340, 287–288. doi: 10.1126/science.1237688
McClelland, J. W., Holmes, R. M., Peterson, B. J., Raymond, P. A., Striegl, R. G., Zhulidov, A. V., et al. (2016). Particulate organic carbon and nitrogen export from major Arctic Rivers. Global Biogeochem. Cycles 30, 629–643. doi: 10.1002/2015GB005351
Miesel, J. R., Hockaday, W. C., Kolka, R. K., and Townsend, P. A. (2015). Soil organic matter composition and quality across fire severity gradients in coniferous and deciduous forests of the southern boreal region. J. Geophys. Res. Biogeosci. 120, 1124–1141. doi: 10.1002/2015JG002959
Moody, J. A., Shakesby, R. A., Robichaud, P. R., Cannon, S. H., and Martin, D. A. (2013). Current research issues related to post-wildfire runoff and erosion processes. Earth Sci. Rev. 122, 10–37. doi: 10.1016/j.earscirev.2013.03.004
Myers-Pigg, A. N., Louchouarn, P., Amon, R. M. W., Prokushkin, A., Pierce, K., and Rubtsov, A. (2015). Labile pyrogenic dissolved organic carbon in major Siberian Arctic Rivers: implications for wildfire-stream metabolic linkages. Geophys. Res. Lett. 42, 377–385. doi: 10.1002/2014GL062762
Naisse, C., Girardin, C., Lefevre, R., Pozzi, A., Maas, R., Stark, A., et al. (2015). Effect of physical weathering on the carbon sequestration potential of biochars and hydrochars in soil. GCB Bioenergy 7, 488–496. doi: 10.1111/gcbb.12158
Nguyen, B. T., Lehmann, J., Hockaday, W. C., Joseph, S., and Masiello, C. A. (2010). Temperature sensitivity of black carbon decomposition and oxidation. Environ. Sci. Technol. 44, 3324–3331. doi: 10.1021/es903016y
Norwood, M. J., Louchouarn, P., Kuo, L. J., and Harvey, O. R. (2013). Characterization and biodegradation of water-soluble biomakers and organic carbon extracted from low temperature chars. Org. Geochem. 56, 111–119. doi: 10.1016/j.orggeochem.2012.12.008
Ponomarev, E., Kharuk, V., and Ranson, K. (2016). Wildfires dynamics in Siberian Larch forests. Forests 7:125. doi: 10.3390/f7060125
Preston, C. M., and Schmidt, H. W. H. (2006). Black (pyrogenic) carbon: a synthesis of current knowledge and uncertainties with special considerations of boreal regions. Biogeosciences 3, 397–420. doi: 10.5194/bg-3-397-2006
Rodionov, A., Amelung, W., Haumaier, L., Urusevskaja, I., and Zech, W. (2006). Black carbon in the zonal steppe soils of Russia. J. Plant Nutr. Soil Sci. 169, 363–369. doi: 10.1002/jpln.200521813
Running, S. W. (2006). Is global warming causing more, larger wildfires. Science 313, 927–928. doi: 10.1126/science.1130370
Ryan, S. E., Dwire, K. A., and Dixon, M. K. (2011). Impacts of wildfire on runoff and sediment loads at little Granite Creek, western Wyoming. Geomorphology 129, 113–130. doi: 10.1016/j.geomorph.2011.01.017
Sang, X. F., Gensch, I., Kammer, B., Khan, A., Kleist, E., Laumer, W., et al. (2016). Chemical stability of levoglucosan: an isotopic perspective. Geophys. Res. Lett. 43, 5419–5424. doi: 10.1002/2016GL069179
Santín, C., Doerr, S. H., Kane, E. S., Masiello, C. A., Ohlson, M., de la Rosa, J. M., et al. (2016). Towards a global assessment of pyrogenic carbon from vegetation fires. Glob. Chang. Biol. 22, 76–91. doi: 10.1111/gcb.12985
Santín, C., Doerr, S. H., Preston, C. M., and Gonzalez-Rodriguez, G. (2015). Pyrogenic organic matter production from wildfires: a missing sink in the global carbon cycle. Glob. Chang. Biol. 21, 1621–1633. doi: 10.1111/gcb.12800
Schmidt, M. W. I., and Noack, A. G. (2000). Black carbon in soils and sediments: analysis, distribution, implications, and current challenges. Global Biogeochem. Cycles 14, 777–793. doi: 10.1029/1999GB001208
Schneider, M. P. W., Hilf, M., Vogt, U. F., and Schmidt, M. W. I. (2010). The benzene polycarboxylic acid (BPCA) pattern of wood pyrolyzed between 200°C and 1000°C. Org. Geochem. 41, 1082–1088. doi: 10.1016/j.orggeochem.2010.07.001
Schneider, M. P. W., Smittenberg, R. H., Dittmar, T., and Schmidt, M. W. I (2011). Comparison of gas with liquid chromatography for the determination of benzenepolycarboxylic acids as molecular tracers of black carbon. Org. Geochem. 42, 275–282. doi: 10.1016/j.orggeochem.2011.01.003
Serkiz, S. M., and Perdue, E. M. (1990). Isolation of dissolved organic matter from the Suwannee River using reverse osmosis. Water Res. 24, 911–916. doi: 10.1016/0043-1354(90)90142-S
Shakesby, R. A., and Doerr, S. H. (2006). Wildfire as a hydrological and geomorphological agent. Earth Sci. Rev. 74, 269–307. doi: 10.1016/j.earscirev.2005.10.006
Shakya, K. M., Louchouarn, P., and Griffin, R. J. (2011). Lignin-derived phenols in Houston Aerosols: implications for natural background sources. Environ. Sci. Technol. 45, 8268–8275. doi: 10.1021/es201668y
Soja, A. J., Tchebakova, N. M., French, N. H. F., Flannigan, M. D., Shugart, H. H., Stocks, B. J., et al. (2007). Climate-induced boreal forest change: predictions versus current observations. Glob. Planet. Change 56, 274–296. doi: 10.1016/j.gloplacha.2006.07.028
Stubbins, A., Niggemann, J., and Dittmar, T. (2012). Photo-lability of deep ocean dissolved black carbon. Biogeosciences 9, 1661–1670. doi: 10.5194/bg-9-1661-2012
Stubbins, A., Spencer, R. G. M., Mann, P. J., Holmes, R. M., McClelland, J. W., Niggemann, J., et al. (2015). Utilizing colored dissolved organic matter to derive dissolved black carbon export by Arctic Rivers. Front. Earth Sci. 3:63. doi: 10.3389/feart.2015.00063
Vonk, J. E., van Dongen, B. E., and Gustafsson, Ö. (2010). Selective preservation of old organic carbon fluvially released from sub-Arctic soils. Geophys. Res. Lett. 37:L11605. doi: 10.1029/2010GL042909
Wagner, S., Cawley, K. M., Rosario-Ortiz, F. L., and Jaffé, R. (2015). In-stream sources and links between particulate and dissolved black carbon following a wildfire. Biogeochemistry 124, 145–161. doi: 10.1007/s10533-015-0088-1
Wang, X., Xu, C., Druffel, E. M., Xue, Y., and Qi, Y. (2016). Two black carbon pools transported by the Changjiang and Huanghe Rivers in China. Global Biogeochem. Cycles 30, 1778–1790. doi: 10.1002/2016GB005509
Ward, C. P., Sleighter, R. L., Hatcher, P. G., and Cory, R. M. (2014). Insights into the complete and partial photooxidation of black carbon in surface waters. Environ. Sci. Process. Impacts 16, 721–731. doi: 10.1039/C3EM00597F
Wrona, F. J., Johansson, M., Culp, J. M., Jenkins, A., Mård, J., Myers-Smith, I. H., et al. (2016). Transitions in Arctic ecosystems: ecological implications of a changing hydrological regime. J. Geophys. Res. Biogeosci. 121, 650–674. doi: 10.1002/2015jg003133
Yunker, M. B., Backus, S. M., Graf Pannatier, E., Jeffries, D. S., and Macdonald, R. W. (2002). Sources and significance of Alkane and PAH hydrocarbons in Canadian Arctic Rivers. Estuar. Coast. Shelf Sci. 55, 1–31. doi: 10.1006/ecss.2001.0880
Yunker, M., Macdonald, R. W., and Whitehouse, B. G. (1994). Phase associations and lipid distributions in the seasonally ice-covered Arctic estuary of the Mackenzie Shelf. Org. Geochem. 22, 651–669. doi: 10.1016/0146-6380(94)90131-7
Keywords: pyrogenic carbon, levoglucosan, freshet, Arctic Rivers, dissolved pyrogenic carbon, particulate pyrogenic carbon
Citation: Myers-Pigg AN, Louchouarn P and Teisserenc R (2017) Flux of Dissolved and Particulate Low-Temperature Pyrogenic Carbon from Two High-Latitude Rivers across the Spring Freshet Hydrograph. Front. Mar. Sci. 4:38. doi: 10.3389/fmars.2017.00038
Received: 07 October 2016; Accepted: 31 January 2017;
Published: 15 February 2017.
Edited by:
Nicholas David Ward, University of Florida, USAReviewed by:
Rudolf Jaffe, Florida International University, USAAlysha Coppola, University of Zurich, Switzerland
Copyright © 2017 Myers-Pigg, Louchouarn and Teisserenc. This is an open-access article distributed under the terms of the Creative Commons Attribution License (CC BY). The use, distribution or reproduction in other forums is permitted, provided the original author(s) or licensor are credited and that the original publication in this journal is cited, in accordance with accepted academic practice. No use, distribution or reproduction is permitted which does not comply with these terms.
*Correspondence: Allison Myers-Pigg, YW5tcEB0YW11LmVkdQ==
†Present Address: Allison N. Myers-Pigg, Memorial University of Newfoundland, Department of Earth Sciences, St. John's, NL, Canada