- 1Red Sea Research Center, King Abdullah University of Science and Technology, Thuwal, Saudi Arabia
- 2Arctic Research Centre, Bioscience, Aarhus University, Århus C, Denmark
- 3Department of Bioscience, Aarhus University, Silkeborg, Denmark
Seagrasses export a substantial portion of their primary production, both in particulate and dissolved organic form, but the fate of this export production remains unaccounted for in terms of seagrass carbon sequestration. Here we review available evidence on the fate of seagrass carbon export to conclude that this represents a significant contribution to carbon sequestration, both in sediments outside seagrass meadows and in the deep sea. The evidence presented implies that the contribution of seagrass meadows to carbon sequestration has been underestimated by only including carbon burial within seagrass sediments.
Introduction
Seagrass meadows have been identified as intense carbon sinks, accumulating large carbon stocks in their sediments (Duarte et al., 2005, 2013; Mcleod et al., 2011; Fourqurean et al., 2012). This has led to the formulation of seagrass conservation and restoration plans to contribute to blue carbon strategies to mitigate climate change (Nellemann et al., 2009; Mcleod et al., 2011; Duarte et al., 2013). However, seagrass meadows also have a role as sources of carbon to adjacent ecosystems (Suchanek et al., 1985; Duarte and Cebrián, 1996; Heck et al., 2008), with seagrass meadows exporting, on average, 24.3% of their net primary production (NPP, Duarte and Cebrián, 1996). The fate of this carbon is diverse, as some is used by fauna or remineralized in adjacent ecosystems, while a part is buried or exported to the deep sea.
Hence, a fraction of the exported 24.3% NPP of seagrass meadows contributes to carbon sequestration beyond the meadows, so that the present focus on storage of organic carbon in sediments within seagrass meadows alone underestimates the true magnitude of the carbon sequestration that seagrasses support. We recently reported that export of materials from macroalgal stands support a large carbon sequestration, comprised by macroalgal carbon buried in sediments or sequestered below 1000 m in the deep ocean (Krause-Jensen and Duarte, 2016). Likewise, a significant fraction of seagrass net primary production may be sequestered elsewhere in the ocean, as the transport and sequestration mechanisms in operation are similar to those reported for macroalgal-derived carbon (Krause-Jensen and Duarte, 2016). Indeed, reports of important contributions of seagrass carbon to sediment stocks outside seagrass meadows were already produced a century ago, as Boysen-Jensen (1914) concluded that the organic matter of sediments in Danish fjords derive almost entirely from Zostera marina. Direct observations of the deep sea floor in the 1960's and 1970's also revealed the presence of abundant seagrass detritus, found to provide an important subsidy to deep-sea fauna (Moore, 1963; Wolff, 1976). Recognizing the spill-over effect of seagrass meadows in supporting carbon sequestration beyond the meadows will add to the rationale to conserve and restore meadows as an efficient action to mitigate against climate change. Moreover, this contribution may be significant, as the fraction of seagrass NPP exported is almost twice that buried in seagrass sediments (Duarte and Cebrián, 1996).
Here we review the existing evidence for exported seagrass carbon to contribute to carbon sequestration. Exported seagrass carbon would be sequestered whether it is deposited, for significant time scales, in sediments outside seagrass meadows or it enters the deep sea, below 1000 m depth, where the carbon is prevented from exchanging with the atmosphere for significant time scales. We did so by searching the published literature for reports of seagrass carbon sequestered in sediments beyond seagrass meadows and seagrass export to the deep sea.
Sequestration of Seagrass Carbon in Coastal Sediments Beyond Seagrass Meadows
We found a total of 65 literature reports of seagrass contribution to sedimentary organic carbon (Corg) beyond seagrass meadows, spanning from shallow coastal waters to the shelf break (Figure 1, cf. Supplementary Table 1). These reports were based on direct and remote observations as well as inferences derived from identification of seagrass detritus in samples collected by bottom sampling devices, such as seafloor trawling, corers and dredges, and direct observations by divers, as well as analyses of sediments for markers of seagrass carbon, such as pentosan, fatty acids, and stable carbon isotopes (Figure 2). However, existing reports are dominated by observation in Europe, North America and the Caribbean Sea, with a single report in the southern hemisphere and none in Asia or Oceania (Figure 1).
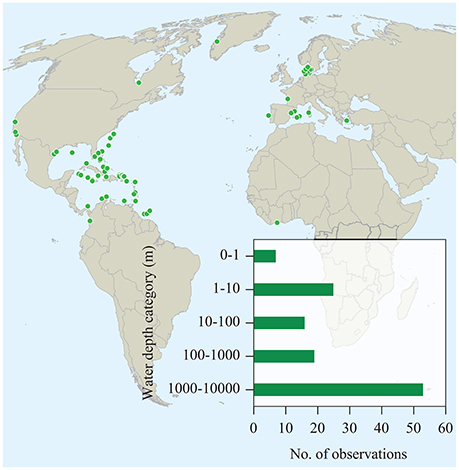
Figure 1. Locations where seagrass carbon has been detected in sediments outside seagrass meadows or in the deep sea. The data set, including sources of data and details on the findings, is available in Supplementary Table 1.
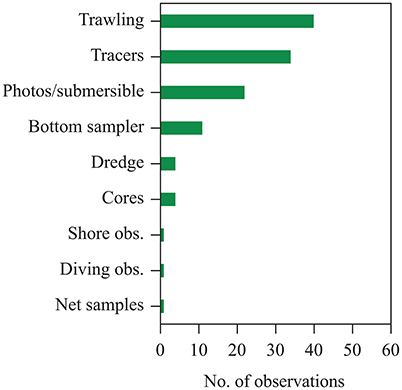
Figure 2. Methods used to identify seagrass material in published reports collected in the data set compiled here of the contribution of exported seagrass detritus to continental shelf sediments and the deep sea.
A comparison between organic carbon (Corg) isotope signatures in seagrass sediments with that in bare sediments adjacent to the meadows concluded that the relative contribution of seagrass to the Corg stock was similar between seagrass and adjacent bare sediments (Kennedy et al., 2010). A more detailed inspection of the data, reveals a close relationship, conforming to a 1:1 line, between the stable isotope composition of Corg in seagrass sediments and that in adjacent sediments (Figure 3). Hence, not only isotopic compositions are, on average, similar, but seagrass sediments and adjacent bare sediments show similar patterns of variability indicating that the percent contribution of seagrass vs. allochthonous material to carbon stocks in seagrass meadows is conserved at some distance beyond the meadow. This confirms that the footprint of seagrass meadows on sediment Corg stocks extends beyond the boundaries of the meadows. Whereas the sedimentary Corg stocks declined away from seagrass meadows, the relative (i.e., as percent of the total) contribution of seagrass to the stock is just as strong in adjacent unvegetated sediments as it is within the meadows. The export range can exceed hundreds to thousands of kilometers, as evidenced by observations of seagrass material in deep-sea sediment far away from the nearest possible sources (Figure 1, Supplementary Table 1), particularly where submarine canyons or strong current systems focalized the export. However, in most situations we would expect an exponential decline in the export flux with distance from the source meadows, as expected from a diffusive process (Okubo, 1971).
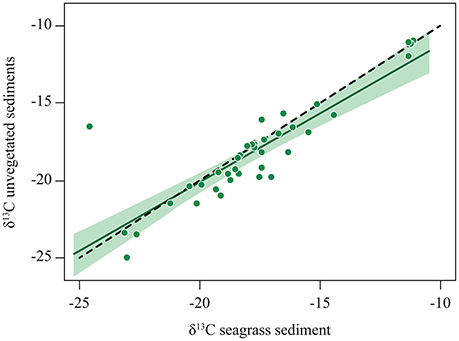
Figure 3. The relationship between the stable isotope signature of organic carbon in bare sediments adjacent (<10 away) to seagrass meadows and that in the sediments within the seagrass meadow. The solid line shows the fitted regression equation: δ13C unvegetated sediments = −2.39 + 0.89 * δ13C seagrass sediments (R2 = 0.75, p < 0.0001), with the shaded area representing the 95% confidence limits of the regression line. The dotted line shows the 1:1 relationship. Data from Kennedy et al. (2010).
A historic assessment, using pentosan as biomarker of the contribution of eelgrass (Z. marina) to sediment organic matter in Danish fjords and coastal sediments in the Kattegat reported the prevalent presence of eelgrass carbon in all sediments examined, and concluded that eelgrass contribution to carbon stocks ranged from 31 to 78% in fjord sediments and 15% in coastal sediments in the Kattegat (Boysen-Jensen, 1914). Likewise, use of α-, β-, and ω-hydroxy fatty acids as well as small amounts of α, β-dihydroxy fatty acids as biomarker for eelgrass (Z. marina), revealed a significant presence of eelgrass-derived carbon in Dutch Wadden Sea sediments (van Dongen et al., 2000). Unfortunately, no systematic surveys for the presence of seagrass Corg in coastal sediments has been attempted to-date, as indicated by the lack of observations in the Southern Hemisphere as well as in Asia and Oceania. However, anecdotal information portends that such surveys are likely to report the presence of seagrass in those sediments. For instance, surveys of sediments in depositional areas in deep Greenland fjords and bays, have reported the presence of macroscopic eelgrass remains in these sediments (Seidenkrantz et al., 2013, Ann Lennert pers com.), which was unexpected as seagrass meadows are relatively sparse along the Greenland coast (Olesen et al., 2015).
Widespread loss of eelgrass following the wasting disease in the 1930's and eutrophication in the 1970's and 1980's (Boström et al., 2014) may have reduced the contribution of seagrass to sediment carbon stocks in Danish waters relative to the large contribution reported by Boysen-Jensen (1914). Likewise, global losses of seagrass meadows (Orth et al., 2006; Waycott et al., 2009) may have impacted on their contribution to carbon sequestration in bare sediments elsewhere. The consequences of seagrass loss for the capacity of seagrass meadows to sequester and store organic carbon have been assessed on the basis of the impacts on sediment organic carbon stocks and fluxes in the meadows (e.g., Fourqurean et al., 2012; McGlathery et al., 2012; Duarte et al., 2013; Marba et al., 2015). However, the finding that seagrasses act as significant sources of organic carbon to sediments beyond the meadows, extending to the hadal zone of the deep sea, indicates that the consequences of continuing seagrass decline extend far beyond the areas where seagrasses grow (Heck et al., 2008). Hence, accounting for the benefits of seagrass conservation, in terms of climate change mitigation, need consider their role in supporting carbon sequestration beyond the meadows.
Remedial actions to reduce nutrient inputs in Denmark and elsewhere have been shown to lead to eelgrass recovery (Riemann et al., 2016) and seagrass planting programs are likely to contribute to rebuild these carbon stocks (e.g., McGlathery et al., 2012; Marba et al., 2015). However, assessment of carbon budgets in seagrass sediments have shown that older meadows export more material than younger ones (Cebrián et al., 2000), so that compensatory planting programs would not compensate for previous losses until the meadows reach a mature state.
The capacity to apportion sedimentary organic carbon between seagrass and other possible sources is, however, subject to uncertainty. Mixing models based on carbon (and nitrogen and sulfur) stable isotopes (e.g., Kennedy et al., 2010) are affected by limitations when resolving multiple sources of organic carbon and by the overlapping isotopic signatures between different primary producers (e.g., seagrass and macroalgae). Organic markers, such as pentosan and fatty acids also involve uncertainties in their limitation to discriminate seagrass-derived carbon from that of land angiosperms. Environmental DNA, the DNA material released by organisms in the environment, has a potential to allow a precise and unambiguous identification of organic carbon sources to marine sediments (Lucas et al., 2012; Nguyen et al., 2015), but its capacity to track seagrass-derived carbon in sediments has not yet been tested.
Evidence of Sequestration of Seagrass Carbon in the Deep Sea
Seagrass shoots and seeds have been reported to raft for significant time (Thiel and Gutow, 2005), allowing for mid- to large-distance transport into the ocean. This allows seagrass material to drift far away from the meadows and, thus, reach the deep sea as also reported for macroalgae (Krause-Jensen and Duarte, 2016). Evidence for sequestration of seagrass carbon in the deep sea derives from reports of seagrass shoots on deep-sea sediments (Figure 1). There are more than 50 records of seagrass shoots laying on the deep-sea floor (>1000 m), derived from trawling, photos and samples collected with box corers or dredges (Supplementary Table 1). These records extend to hadal sediments, with a record of Thalassia testudinum shoots in sediments at 8330 m depth in the Puerto Rico Trench (Wolff, 1979). Additional evidence derives from the finding of use of T. testudinum by large protozoans, actinians, gastropods, a tanaid crustacean, a polychaete, an echiurid worm, and isopods at depths ranging from 5000 to 8330 m in the Caribbean (Wolff, 1976).
Whereas a quantitative assessment of the flux and mass of seagrass to the deep sea cannot be derived from those observations, they do not represent, in any way, exceptional or unusual records, but rather suggest that seagrass detritus is a prevalent feature of deep-sea landscapes (Wolff, 1976). For instance, Roper and Brundage (1972) identified seagrass detritus in each one of the thousands of photos from the Virgin Islands Basin they explored, with densities up to 10 fragments per square meter. Indeed, evidence of seagrass detritus in the deep sea derived overwhelmingly from identification of seagrass leaves or shoots in videos and images collected by deep-sea submersible. In addition evidence was also derived using a range of techniques used to sample sediments on the continental shelf (Figure 2).
Sequestration of seagrass carbon in the deep sea does not require that seagrass reaches the sediments, but it suffices that seagrass carbon reaches depths >1000 m. Even if remineralized to CO2, carbon reaching below 1000 m in the marine water column is removed from atmospheric exchange for time scales sufficient long (>100 years) as to be significant for climate regulation, thereby qualifying as sequestration in this context (Caldeira et al., 2002). For instance, Maher and Eyre (2010) first identified seagrass meadows as significant sources of dissolved organic carbon (DOC), and suggested that they may contribute to the pool of refractory DOC in the ocean, one of the largest sink reservoirs of carbon in the biosphere. Indeed, Barrón and Duarte (2015) estimated that the large export of DOC from the coastal to the open ocean contributes to carbon sequestration as DOC stored in the deep sea.
The delivery of seagrass-derived carbon to the deep sea (i.e., water column or sediments >1000 m depth) is constrained by the decomposition time scales of seagrass carbon. Estimates of decomposition rates of seagrass organic matter indicate first order decay coefficients of about 0.006 day−1, indicating characteristic half-lives of seagrass detritus of about 110 days (Enríquez et al., 1993). The half-lives of seagrass detritus are about 10 times longer than that of phytoplankton detritus (14 days, Enríquez et al., 1993), suggesting longer transport and sinking length scales than those for phytoplankton-derived detritus. Whereas the time scales of seagrass carbon delivery to the deep sea is poorly constrained, frequent observations of intact seagrass shoots in the deep sea indicates that transport may be, at times, much faster than detritus half-lives. For instance, rafts of drifting Thalassia ca. 50 m in diameter were noted in the Florida current after a hurricane (Menzies et al., 1967). Subsequent dredging operations on the deep-sea floor off North Carolina revealed substantial drift detrital Thalassia 550–1100 km from any known source region (Menzies and Rowe, 1969). The delivery of seagrass carbon to the deep-sea is, therefore, likely to be episodic, following major storms lifting and transporting seagrass detritus offshore, through transport mechanisms reported in detail for macroalgae (Krause-Jensen and Duarte, 2016).
Contribution of Exported Seagrass Carbon to Carbon Sequestration. Implications for Blue Carbon
The export of carbon by seagrass, which includes a substantial fraction of their net primary production, had not been considered as contributing to their role as carbon sinks. The observations summarized here (Figure 1) conclusively demonstrate that the role of seagrass in carbon sequestration extends beyond the area occupied by the meadows themselves to contribute to carbon sequestration in estuarine, shelf and deep-sea sediments. Together, carbon burial in bare sediments in estuarine, shelf and deep-sea environments has been estimated at 132 Tg C year−1 (Duarte et al., 2005), which is comparable to the estimated global export of seagrass carbon of 120 Tg C year−1 (Duarte and Cebrián, 1996). Whereas not all exported carbon is buried in sediments, as some is decomposed or used by the food webs, the comparison of the magnitudes above indicate that there is scope for seagrasses to have a large contribution to carbon burial in bare sediments across the entire range of ocean depth.
The observations on seagrass contributions to organic carbon sequestration beyond the boundaries of seagrass meadows summarized here were originated as largely serendipitous outcomes of research motivated by goals other than assessing seagrass contributions to carbon sequestration (Figure 4). These were largely derived from research focused on the ecology of deep-sea fauna (e.g., Wolff, 1979) and carbon sources to marine food webs (Baeta et al., 2009), and also included research motivated by the study of organic matter fluxes to the deep sea (e.g., Wiebe et al., 1976), surveys of marine debris (Wei et al., 2012), assessments of kelp contributions to deep-sea sediments (e.g., Harrold et al., 1998), and assessments of the sources of sediment organic matter (e.g., Boysen-Jensen, 1914; Boschker et al., 2000), among other research topics. Taken in concert, however, these reports produce strong and compelling evidence that seagrass meadows contribute to sedimentary carbon stocks in bare sediments extending from shallow littoral areas to the hadal regions of the ocean.
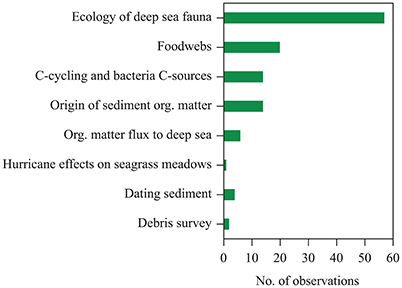
Figure 4. Motivation for studies included in the data set compiled providing evidence of the contribution of exported seagrass detritus to continental shelf sediments and the deep sea.
Export represents, on average, 24.3% of net seagrass primary production, estimated at 490 Tg C year−1, resulting in a total export of 120 Tg C year−1 (Duarte and Cebrián, 1996, Figure 5). Barron et al. (2014) estimated that global seagrass DOC export represents 33 Tg C year−1, thereby implying an export of 87 Tg C year−1 of seagrass detritus as POC (Figure 5). Krause-Jensen and Duarte (2016) estimated that 2/3's of macroalgal DOC is remineralized within the photic layer, while 1/3 is exported below the photic layer, and potentially sequestered in the deep sea. Assuming similar partitioning for seagrass-derived DOC implies an upper limit to seagrass-derived DOC sequestration of 11 Tg C year−1 (Figure 5), or about 14% of the carbon buried in seagrass sediments. However, the observational basis on the fate of seagrass-derived POC does not suffice to calculate how much of this is buried in continental shelf sediments beyond the meadows or in the deep sea (Figure 5). The seagrass carbon mineralized by microbes or entering the food web in the deep sea represents a contribution to seagrass carbon sequestration, as the seagrass carbon remains trapped in deep-sea waters for time spans long enough to qualify as sequestration even if it is fully respired to CO2. Krause-Jensen and Duarte (2016) provided a very coarse estimate that about 15% of macroalgal POC export is eventually sequestered in continental shelf sediments or the deep sea. Assuming a similar fate for seagrass-derived detritus, POC sequestration beyond seagrass sediments would represent 13 Tg C year−1, resulting in a possible sequestration of seagrass organic carbon (DOC + POC) beyond seagrass meadows of 24 Tg C year−1 (Figure 5). Total organic carbon burial in shelf and deep-sea sediments is estimated at 132.2 Tg C year−1 (Duarte et al., 2005), with an additional 50 Tg C sequestered annually as (anthropogenic) CO2 in ocean waters below 1000 m (Honjo et al., 2014). Hence, the total organic carbon sequestration in shelf sediments, deep-sea sediments and the deep-sea water column is >6 times greater than the 24 Tg C of seagrass-derived carbon possibly sequestered beyond seagrass meadows. Hence, our rough estimate of possible seagrass sequestration can be reconciled with estimates of carbon sinks beyond seagrass meadows. However, the indicative estimates provided are subject to important uncertainties derived from those affecting the magnitude of the global export of seagrass carbon (i.e., the global area covered by seagrass and the primary production exported), and the fraction of the exported carbon that may reach sink reservoirs, for which there are no published estimates and the estimates available for macroalgae can only be considered as indicative.
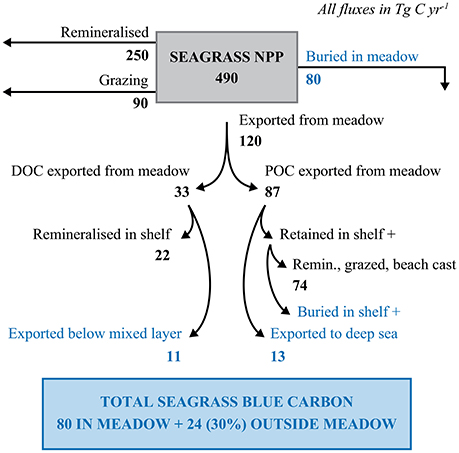
Figure 5. Pathways to seagrass carbon sequestration. The fate of seagrass NPP in terms of remineralization, grazing, burial in seagrass bed and export from seagrass beds are derived from (Duarte and Cebrián, 1996). DOC export is derived from Barron et al. (2014). The fraction of DOC export that is exported below the photic layer as well as the potential sequestration of exported POC is from Krause-Jensen and Duarte (2016), assuming a similar fate to that of exported macroalgal DOC and POC.
Recently, we reported that burial of exported macroalgal carbon represents a major contribution to biological carbon sequestration in the ocean (Krause-Jensen and Duarte, 2016). Likewise, we show here that the potential sequestration of seagrass carbon exported beyond the meadows may approach 30% of the carbon sequestered within sediments in seagrass meadows. Even considering current uncertainties as to the magnitudes of seagrass export sequestered beyond the meadows, there is abundant evidence that seagrass carbon can be found in shelf and deep-sea sediments. Carbon stocks and exports rates are likely to vary greatly among seagrass meadows, with export controlled by local hydrodynamics and turbulence. In addition, features such as submarine canyons that facilitate the delivery of organic matter to the deep sea will enhance export and subsequent sequestration of carbon in the deep sea. Evaluating the contribution of seagrasses to carbon sequestration in sediments beyond seagrass meadows and the deep sea is important to improve current estimates of the role of seagrass in marine carbon sequestration capacity.
Author Contributions
CD and DK jointly conceived and executed this research, gathered, and analyzed the data and wrote the manuscript. Both contributed equally to this research
Conflict of Interest Statement
The authors declare that the research was conducted in the absence of any commercial or financial relationships that could be construed as a potential conflict of interest.
Acknowledgments
The study was funded by the King Abdullah University of Science and Technology through the baseline funding to CD. DK received support from the COCOA project under the BONUS program funded by the EU 7th framework program and the Danish Research Council and from the NOVAGRASS (0603-00003DSF) project funded by the Danish Council for Strategic Research. We thank Tinna Christensen and Ane Kjeldgård for help with the figures.
Supplementary Material
The Supplementary Material for this article can be found online at: http://journal.frontiersin.org/article/10.3389/fmars.2017.00013/full#supplementary-material
References
Baeta, A., Valiela, I., Rossi, F., Pinto, R., Richard, P., Niquil, N., et al. (2009). Eutrophication and trophic structure in response to the presence of the eelgrass Zostera noltii. Mar. Biol. 156, 2107–2120. doi: 10.1007/s00227-009-1241-y
Barron, C., Apostolaki, E. T., and Duarte, C. M. (2014). Dissolved organic carbon fluxes by seagrass meadows and macroalgal beds. Front. Mar. Sci. 1:42. doi: 10.3389/fmars.2014.00042
Barrón, C., and Duarte, C. M. (2015). Dissolved organic carbon pools and export from the coastal ocean. Global Biogeochem. Cycles 29, 1725–1738. doi: 10.1002/2014GB005056
Boschker, H. T. S., Wielemaker, A., Schaub, B., and Holmer, M. (2000). Limited coupling of macrophyte production and bacterial carbon cycling in the sediments of Zostera spp. meadows. Mar. Ecol. Prog. Ser. 203, 181–189. doi: 10.3354/meps203181
Boström, C., Baden, S., Bockelmann, A. C., Dromph, K., Fredriksen, S., Gustafsson, C., et al. (2014). Distribution, structure and function of Nordic seagrass ecosystems: implications for coastal management and conservation. Aquat. Conserv. 24, 410–434. doi: 10.1002/aqc.2424
Boysen-Jensen, P. (1914). Studies concerning the organic matter of the sea bottom. Rep. Dan. Biol. Stat. 22, 1–39.
Caldeira, K., Wickett, M. E., and Duffy, P. B. (2002). Depth, radiocarbon, and the effectiveness of direct CO2 injection as an ocean carbon sequestration strategy. Geophys. Res. Lett. 29. doi: 10.1029/2001GL014234
Cebrián, J., Pedersen, M. F., Kroeger, K. D., and Valiela, I. (2000). Fate of production of the seagrass Cymodocea nodosa in different stages of meadow formation. Mar. Ecol. Prog. Ser. 204, 119–130. doi: 10.3354/meps204119
Duarte, C. M., and Cebrián, J. (1996). The fate of marine autotrophic production. Limnol. Oceanogr. 41, 1758–1766. doi: 10.4319/lo.1996.41.8.1758
Duarte, C. M., Losada, I. J., Hendriks, I. E., Mazarrasa, I., and Marbà, N. (2013). The role of coastal plant communities for climate change mitigation and adaptation. Nat. Clim. Change 3, 961–968. doi: 10.1038/nclimate1970
Duarte, C. M., Middelburg, J. J., and Caraco, N. (2005). Major role of marine vegetation on the oceanic carbon cycle. Biogeosciences 2, 1–8. doi: 10.5194/bg-2-1-2005
Enríquez, S., Duarte, C. M., and Sand-Jensen, K. (1993). Patterns in decomposition rates among photosynthetic organisms: the importance of detritus C:N:P content. Oecologia 94, 457–471. doi: 10.1007/BF00566960
Fourqurean, J. W., Duarte, C. M., Kennedy, H., Marbà, N., Holmer, M., Mateo, M. A., et al. (2012). Seagrass ecosystems as a globally significant carbon stock. Nat. Geosci. 5, 505–509. doi: 10.1038/ngeo1477
Harrold, C., Light, K., and Lisin, S. (1998). Organic enrichment of submarine canyon and continental shelf benthic communities by macroalgal drift imported from nearshore kelp forests. Limnol. Oceanogr. 43, 669–678. doi: 10.4319/lo.1998.43.4.0669
Heck, K. L. Jr., Carruthers, T. J. B., Duarte, C. M., Hughes, A. R., Kendrick, G., Orth, R., et al. (2008). Trophic transfers from seagrass meadows subsidize diverse marine and terrestrial consumers. Ecosystems 11, 1198–1210. doi: 10.1007/s10021-008-9155-y
Honjo, S., Eglinton, T. I., Taylor, C. D., Ulmer, K. M., Sievert, S. M., and Bracher, A. (2014). Understanding the role of the Biological Pump in the Global Carbon Cycle: an imperative for Ocean Science. Oceanography 27, 10–16. doi: 10.5670/oceanog.2014.78
Kennedy, H., Beggins, J., Duarte, C. M., Fourqurean, J. W., Holmer, M., Marbà, N., et al. (2010). Seagrass sediments as a global carbon sink: isotopic constraints. Global Biogeochem. Cycles 24, GB4026. doi: 10.1029/2010GB003848
Krause-Jensen, D., and Duarte, C. M. (2016). Substantial role of macroalgae in marine carbon sequestration. Nat. Geosci. 9, 737–742. doi: 10.1038/ngeo2790
Lucas, C., Thangaradjou, T., and Papenbrock, J. (2012). Development of a DNA barcoding system for seagrasses: successful but not simple. PLoS ONE 7:e29987. doi: 10.1371/journal.pone.0029987
Maher, D. T., and Eyre, B. D. (2010). Benthic fluxes of dissolved organic carbon in three temperate Australian estuaries: implications for global estimates of benthic DOC fluxes. J. Geophys. Res. 115:G04039. doi: 10.1029/2010JG001433
Marba, N., Arias-Ortiz, A., Masque, P., Kendrick, G. A., Mazarrasa, I., Bastyan, G. R., et al. (2015). Impact of seagrass loss and subsequent revegetation on carbon sequestration and stocks. J. Ecol. 103, 296–302. doi: 10.1111/1365-2745.12370
McGlathery, K., Reynolds, L., Cole, L., Orth, R., Marion, S. R., and Schwarzschild, A. (2012). Recovery trajectories during state change from bare sediment to eelgrass dominance. Mar. Ecol. Prog. Ser. 448, 209–221. doi: 10.3354/meps09574
Mcleod, E., Chmura, G. L., Bouillon, S., Salm, R., Björk, M., Duarte, C. M., et al. (2011). A Blueprint for Blue Carbon: towards an improved understanding of the role of vegetated coastal habitats in sequestering CO2. Front. Ecol. Env. 9, 552–560. doi: 10.1890/110004
Menzies, R. J., and Rowe, C. T. (1969). The distribution and significance of detrital turtle grass, Thalassia testudinum, on the deep-sea floor off North Carolina. Intern. Rev. Ges. Hydrobiol. 54, 217–222. doi: 10.1002/iroh.19690540206
Menzies, R. J., Zaneveld, J. S., and Pratt, R. M. (1967). Transported turtle grass as a source of organic enrichment of abyssal sediments off North Carolina. Deep Sea Res. Oceanogr. Abstr. 14, 111–112. doi: 10.1016/0011-7471(67)90032-0
Nellemann, C., Corcoran, E., Duarte, C. M., Valdes, L., DeYoung, C., Fonseca, L., et al. (eds.). (2009). Blue Carbon. A Rapid Response Assessment, Vol, 80. United Nations Environment Programme, GRID-Arendal.
Nguyen, X.-V., Höfler, S., Glasenapp, Y., Thangaradjou, T., Lucas, C., and Papenbrock, J. (2015). New insights into DNA barcoding of seagrasses. Sys. Biodiver. 13, 496–508. doi: 10.1080/14772000.2015.1046408
Okubo, A. (1971). Oceanic diffusion diagrams. Deep Sea Res. 18, 789–802. doi: 10.1016/0011-7471(71)90046-5
Olesen, B., Krause-Jensen, D., Marbà, N., and Christensen, P. B. (2015). Eelgrass (Zostera marina L.) in subarctic Greenland: dense meadows with slow biomass turnover in cold waters. Mar. Ecol. Prog. Ser. 518, 107–121. doi: 10.3354/meps11087
Orth, R. J., Carruthers, T. J. B., Dennison, W. C., and Duarte, C. M. (2006). A global crisis for seagrass ecosystems. BioScience 56, 987–996.
Riemann, B., Carstensen, J., Dahl, K., Fossing, H., Hansen, J. W., Jakobsen, H. H., et al. (2016). Recovery of Danish coastal ecosystems after reductions in nutrient loading: a holistic ecosystem approach. Estuar. Coast 39, 82–97. doi: 10.1007/s12237-015-9980-0
Roper, C. F., and Brundage, W. (1972). Cirrate octopods with associated deep-sea organisms: new biological data based on deep benthic photographs (Cephalopoda). Smithson. Contrib. Zool. 121, 1–46. doi: 10.5479/si.00810282.121
Seidenkrantz, M. S., Ebbesen, H., Aagaard-Sørensen, S., Moros, M., Lloyd, J. M., Olsen, J., et al. (2013). Early Holocene large-scale meltwater discharge from Greenland documented by foraminifera and sediment parameters. Palaeogeogr. Palaeocl. 391, 71–81. doi: 10.1016/j.palaeo.2012.04.006
Suchanek, T. H., Williams, S. L., Ogden, J. C., Hubbard, D. K., and Gill, L. P. (1985). Utilization of shallow-water seagrass detritus by Caribbean deep-sea macrofauna: δ13C evidence. Deep Sea Res. 32, 201–214. doi: 10.1016/0198-0149(85)90028-7
Thiel, M., and Gutow, L. (2005). The ecology of rafting in the marine environment. I. The floating substrata. Oceanogr. Mar. Biol. 42, 181–264. doi: 10.1201/9780203507810.ch6
van Dongen, B. E., Rijpstra, W. I. C., Philippart, C. J., de Leeuw, J. W., and Damsté, J. S. S. (2000). Biomarkers in upper Holocene Eastern North Sea and Wadden Sea sediments. Org. Geochem. 31, 1533–1543. doi: 10.1016/S0146-6380(00)00125-X
Waycott, M., Duarte, C. M., Carruthers, T. J., Orth, R. J., Dennison, W. C., Olyarnik, S., et al. (2009). Accelerating loss of seagrasses across the globe threatens coastal ecosystems. Proc. Natl. Acad. of Sci. U.S.A. 106, 12377–12381. doi: 10.1073/pnas.0905620106
Wei, C. L., Rowe, G. T., Nunnally, C. C., and Wicksten, M. K. (2012). Anthropogenic “Litter” and macrophyte detritus in the deep Northern Gulf of Mexico. Mar. Pol. Bull. 64, 966–973. doi: 10.1016/j.marpolbul.2012.02.015
Wiebe, P. H., Boyd, S. H., and Winget, C. L. (1976). Particulate Matter Sinking to the Deep-Sea Floor at 2000 m in the Tongue of the Ocean, Bahamas, with a description of a New Sedimentation Trap. Technical Report, Woods Hole Oceanographic Institution.
Wolff, T. (1976). Utilization of seagrass in the deep sea. Aquat. Bot. 2, 161–174. doi: 10.1016/0304-3770(76)90017-6
Keywords: seagrass, carbon, export, blue carbon, deep sea
Citation: Duarte CM and Krause-Jensen D (2017) Export from Seagrass Meadows Contributes to Marine Carbon Sequestration. Front. Mar. Sci. 4:13. doi: 10.3389/fmars.2017.00013
Received: 29 June 2016; Accepted: 09 January 2017;
Published: 17 January 2017.
Edited by:
Stelios Katsanevakis, University of the Aegean, GreeceReviewed by:
Damien Troy Maher, Southern Cross University, AustraliaCosimo Solidoro, National Institute of Oceanography and Experimental Geophysics, Italy
Copyright © 2017 Duarte and Krause-Jensen. This is an open-access article distributed under the terms of the Creative Commons Attribution License (CC BY). The use, distribution or reproduction in other forums is permitted, provided the original author(s) or licensor are credited and that the original publication in this journal is cited, in accordance with accepted academic practice. No use, distribution or reproduction is permitted which does not comply with these terms.
*Correspondence: Carlos M. Duarte, Y2FybG9zLmR1YXJ0ZUBrYXVzdC5lZHUuc2E=