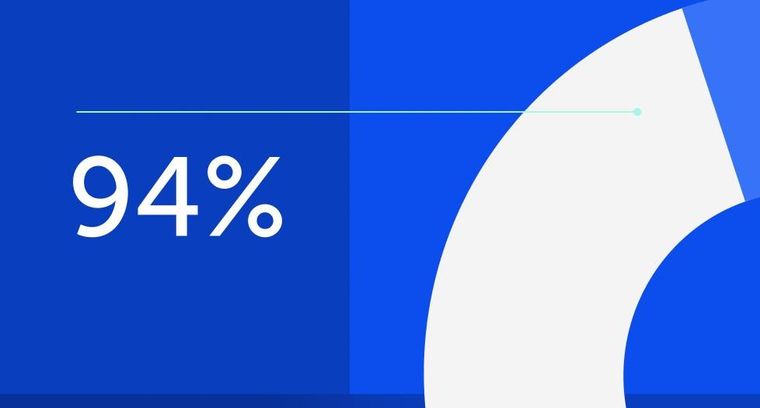
94% of researchers rate our articles as excellent or good
Learn more about the work of our research integrity team to safeguard the quality of each article we publish.
Find out more
ORIGINAL RESEARCH article
Front. Mar. Sci., 10 January 2017
Sec. Marine Biogeochemistry
Volume 3 - 2016 | https://doi.org/10.3389/fmars.2016.00289
This article is part of the Research TopicOrganic ligands - A key control on trace metal biogeochemistry in the oceanView all 22 articles
Planktonic grazers such as salps may have a dominant role in iron (Fe) cycling in surface waters of the Southern Ocean (SO). Salps have high ingestion rates and egest large, fast sinking fecal pellets (FPs) that potentially contribute to the vertical flux of carbon. In this study, we determined the impact of FPs from Salpa thompsoni, the most abundant salp in the SO, on Fe biogeochemistry. During the Polarstern expedition ANT-XXVII/3, salps were sampled from a large diatom bloom area in the Atlantic sector of the SO. Extensive work on carbon export and salp FPs export at the sampling location had shown that salps were a minor component of zooplankton and were responsible for only a 0.2% consumption of the daily primary production. Furthermore, at 100 m, export efficiency of salp FPs was ~2–3 fold higher than that of the bulk of sinking particulate organic carbon (POC). After collection, salps were maintained in 200 μm screened seawater and their FPs were collected for further experiments. To investigate whether the FPs release Fe and/or Fe-binding ligands into the filtered seawater (FSW) under different experimental conditions, they were either incubated in the dark or under full sunlight at in situ temperatures for 24 h, or placed into the dark after a freeze/thaw treatment. We observed that none of the treatments caused release of dissolved Fe (dFe) or strong Fe ligands from the salp FPs. However, humic-substance like (HS-like) compounds, weak Fe ligands, were released at a rate of 8.2 ± 4.7 μg HS-like FP−1 d−1. Although the Fe content per salp FP was high at 0.33 ± 0.02 nmol dFe FP−1, the small contribution of salps to the zooplankton pool resulted in an estimated dFe export flux of 11.3 nmol Fe m−2 d−1 at 300 m. Since salp FPs showed an export efficiency at 100 m well above that shown by the bulk of sinking POC, our results suggest that in those areas of the SO where salps play a major role in the grazing of primary production, they could be actively contributing to the depletion of the dFe pool in surface water.
In the Southern Ocean (SO), known to be the largest high nutrient low chlorophyll (HNLC) oceanic region, iron (Fe) availability is paramount in controlling primary productivity with subsequent implications for atmospheric carbon dioxide concentrations (Martin et al., 1990; Blain et al., 2007; Hassler et al., 2012). Knowledge and study of the different potential Fe sources are thus proving to be of major importance. Depending on the region, Fe can enter the SO mixed layer via different “external” inputs such as seasonal sea-ice retreat, dust deposition, resuspension of coastal and shallow sediments (Duce and Tindale, 1991; Lannuzel et al., 2007; Moore and Braucher, 2008) and upwelling phenomena (de Jong et al., 2012). Nevertheless, it has been demonstrated that in the HNLC regions from the SO, recycled Fe can account up to 90% of the total Fe biological supply (Boyd et al., 2005, 2010; Tagliabue et al., 2014), emphasizing that Fe recycling is an essential process sustaining primary productivity.
Studies have shown that zooplankton grazing affects the recycling of trace metals (Hutchins and Bruland, 1994; Strzepek et al., 2005; Tovar-Sanchez et al., 2007), but the relative contribution of the different key SO grazers krill, salps and copepods with respect to Fe recycling vs. export is still virtually undefined (Sarthou et al., 2008). In the western subarctic Pacific, copepod grazing has been shown to be a major route for the release of regenerated Fe and organic Fe-binding ligands, key compounds for its reactivity and bioavailability (Sato et al., 2007). Krill have also been shown to have an important role in Fe recycling which might be essential to maintain primary production in the SO (Tovar-Sanchez et al., 2007). Nevertheless, despite an observed decline in krill stocks coupled with a coincident increase in salps (Atkinson et al., 2004; Alcaraz et al., 2014), the impact of salps on Fe recycling has yet to be assessed.
Compared to krill or copepods (Pakhomov et al., 2006; Ducklow et al., 2012), it has been suggested that salps strongly contribute to the vertical flux of biogenic carbon due to their production of large, fast sinking fecal pellets (FPs; Pakhomov et al., 2002). Sinking velocity is an important parameter since it will determine the residence time during which the FPs will be subjected in the mixed layer to physicochemical recycling processes such as photo-degradation, before being exported and undergoing further microbial decomposition and coprophagy (Turner, 2002; Rontani, 2008; Pisani et al., 2011; Giering et al., 2014). For instance, FPs sinking velocity produced by small salp aggregates (10–20 mm length) was estimated at around 200–400 m d−1 and from larger aggregates (20–30 mm length) at around 700 m d−1 (Phillips et al., 2009). However, those values can be highly variable depending on size, density, form, and nutritional content of the FPs. Extensive work during our cruise, spotlighted a high retention of the salp FPs in the upper 300 m at ~80% suggesting extensive recycling processes and thus, their relatively weak contribution to the carbon export (Iversen et al., 2016). This assumption is in contrast with the one from Pakhomov et al. (2002) and highlights that salp FPs are not always conduits for rapid export.
This study explores the interaction between salp FPs and Fe biogeochemistry. In order to quantify the impact of salp FPs to both Fe recycling and export, the release of dissolved Fe (dFe) and Fe-binding ligands from Salpa thompsoni FPs was studied during laboratory and on-board incubation experiments. These studies simulated the physicochemical conditions that freshly released FPs encounter during their residence in surface waters. FPs used in this study were produced by salps collected in a large diatom bloom within the SO, suggesting that FPs were packed with diatom cells and therefore, rich in nutrients, Fe, and ligands. In order to assess the impact of light on Fe chemistry, we exposed FPs to visible and UV light. Furthermore, we tested the impact of freezing in order to determine a potential release of Fe and/or Fe-binding ligands due to physical break-up of FPs. Additionally, we made direct measurements of Fe content within salp FPs as well as their Fe:C ratio. The latter measurements allowed to estimate the role of salp FPs for vertical Fe fluxes.
During the Antarctic expedition ANTXXVIII/3 on-board the R.V. Polarstern (January to March 2012; Wolf-Gladrow 2013), salps were sampled from the center of a stable diatom bloom at 51°12.38 S, 12°39.80 W. The chlorophyll-a concentrations within the diatom bloom reached up to 2 μg chlorophyll a L−1 (Hoppe et al., 2015).
For experiments, salps were collected during the night at a sampling depth of 25 m using a bongo net with cod ends lined with plastic to minimize metal contamination. Seawater that was previously collected at a sampling depth of 25 m using a Teflon double diaphragm pump (Wilden A100 with Teflon fittings; Kelair Pumps Australia Pty Ltd) and polyethylene tubing was used to maintain the salps and rinse salps and FPs. The seawater, collected in using trace metal clean techniques, was then directly pumped into a shipboard laminar flow hood inside of a trace metal clean room container. The sampled seawater was either screened through a 200-μm plankton net (screened seawater, SSW) or 0.2-μm filter (filtered seawater, FSW) (Acropak 1000, PALL). The collected salps were gently rinsed three times with this FSW, placed in a 50 L tank filled with SSW and kept in the dark at the in situ seawater temperature (3°C). Every 6–8 h, approximately one third of the SSW was carefully replaced with fresh SSW. From the incubation tanks, FPs were collected with a clean plastic pipette followed by FSW rinse. The FPs were either placed in a 100 mL trace metal clean low density polyethylene (LDPE) bottle filled with FSW for the on-board experiments or stored at −80°C for laboratory experiments. The salp FPs were compact looking pellets packed with particles (described as “type 1” in Iversen et al., 2016).
These experiments aimed to measure short-term effects of freezing and light exposure on dFe and Fe-binding ligands release from salp FPs. Indeed, particulate organic matter may undergo photochemical transformations in the upper surface water that may lead to a production of dissolved organic matter (Pisani et al., 2011) likely to interact with Fe chemistry. In the same manner, freezing causes break-up of the particles within the FPs and will potentially result in release of dissolved organic compounds to the surrounding waters. Considering that salp FPs take about 4–24 h to travel the first 100 upper meters, all the incubations were performed over a period of 24 h (Iversen et al., 2016). The on-board experiments were done in FSW with and without FPs. Two 20 L carboys were filled with FSW and 40 FPs were added to one of them. For consistency, both carboys were rolled and shaken for 20 min in order to homogenize the FPs. The two homogenized seawater batches were then transferred into 4 L polycarbonate bottles that were exposed to three different experimental conditions. Two incubation bottles were (i) frozen at −20°C for 10 h followed by thawing in the dark at sea surface temperature (SST) for 24 h (“freezer”), (ii) incubated for 24 h in the dark (“dark”), or (iii) exposed for 24 h to natural visible sunlight at SST in an on-deck incubator with a “flow-through” seawater system (“VIS”). Finally, 2 L were sampled from each 20 L carboy into 2 L polycarbonate bottles for the analysis of initial parameters (“control”). Treatments “freezer” and “dark” were wrapped in several layers of black plastic to maintain dark conditions whilst treatment “VIS” was contained in clear plastic bags (double wrapped). At the end of the incubation period, unfiltered and filtered (0.2-μm, Nuclepore, Millipore) samples were collected from each incubation bottle for determination of Fe speciation, humic substance-like material (HS-like, weak Fe ligands), dFe and macronutrients. Samples for dFe and macronutrients were analyzed on-board whereas Fe speciation and HS-like samples were stored at −20°C until analysis at the University of Geneva.
We determined the amount Fe leaching from the FPs after a period of 48 h acidification at pH 2.0 (HCl, Ultrapur, Merck). Six FPs were placed in six 30 mL LDPE containers filled with 10 mL of filtered surface seawater (dFe = 0.15 nM) and kept at room temperature before analysis by voltammetry (Laglera et al., 2013) with internal calibration. FP suspensions were not filtered before analysis since voltammetry is not responsive to particulate Fe.
Laboratory experiments were performed in synthetic seawater [AQUIL media based on Price et al. (1989) using major salts only, pH = 8.13] to facilitate Fe binding ligands detection and to extend light exposure to ultraviolet light (“UV”). Four polytetrafluoroethylene-capped quartz sample tubes were filled with 10 mL of synthetic seawater and six FPs were added to two of them. One tube with FPs and one without FPs were placed under a sunlight simulator and exposed to UV and visible light (220–780 nm, 1940 μEinstein, ABET Technologies Sun 2000) for 30 min on ice prior to 24 h incubation in the dark at 4°C on an agitation plate (100 rpm; VWR STD 3500). The two other tubes were placed directly on the agitation plate without exposure to light (“dark”). After incubation, each sample was filtered on to a 0.2 μm polycarbonate filter for analysis of dFe, HS-like and Fe speciation. Samples for Fe speciation and HS-like were stored at −20°C and dFe samples were acidified [0.5% with quartz distilled hydrochloric acid (qHCl), VWR] and left at room temperature until analysis.
The total Fe content in FPs was determined after storing five FPs in a mixture of HCl/HNO3 (pH = 1.52) for 2 months (n = 2) prior being analyzed by Inductively Coupled Plasma Mass Spectrometry (Agilent 7700). Aluminum (Al), nickel (Ni), copper (Cu), zinc (Zn), and molybdenum (Mo) were also quantified. Standard solutions for ICP (Sigma) were used for the calibrations and the blank averages in counts per second were 343 (27Al), 18076 (56Fe), 437 (64Zn), 2848 (65Cu), 120 (60Ni), and 247 (98Mo).
DFe, HS-like and Fe speciation analyses were performed using a μAutolab potentiostat (Ecochemie, Netherlands) with a 663 Metrohm stand as in Norman et al. (2015) and Laglera et al. (2013). Fe speciation was measured by Competitive Ligand Exchange-Adsorptive Cathodic Stripping Voltammetry (CLE-AdCSV) as per Abualhaija and van den Berg (2014) in order to provide the concentration of Fe-binding ligands (LT) and the conditional stability of their complexes with Fe (K'Fe'L). Analysis of SAFe (Sampling and Analysis of Fe) reference sample S (0.101 ± 0.005 nM; n = 3), detection limit (0.05 nM) and side reaction coefficient for salycilaldoxime (SA) at a concentration of 5 μM (αFe'SA = 20.1) were in accordance with the literature (Abualhaija and van den Berg, 2014; Mahmood et al., 2015). Peak heights and the chemical speciation parameters were calculated with ECDSoft and proMCC software, respectively (Omanović et al., 2015). Titrations performed on AQUIL samples in the presence of 5 nM desferrioxamine B (DFB; Figure 1C) allowed us to validate our analytical method. Both LT (4.61 ± 0.13 nM; n = 2) and stability constant (log K'Fe'L of 12.25 ± 0.04) corresponded with reported values (Witter et al., 2000; Abualhaija and van den Berg, 2014). HS-like compounds were determined using the voltammetric method of Laglera et al. (2007) with standard addition on each sample of Suwannee River Fulvic Acid (SRFA, International Humic Substances Society, standard 1) for calibration. The limit of detection was 1.88 μg SRFA.L−1. DFe was measured onboard by catalytic cathodic voltammetry (Laglera et al., 2013) and a summary of data can be found in (Hoppe et al., 2015). Macronutrients (nitrate (), nitrite (), orthophosphate (), and orthosilicate (Si (OH)4) were determined colorimetrically on-board using a Technicon TRAACS 800 autoanalyzer (details in Hoppe et al., 2015).
Figure 1. Iron speciation titrations performed by CLE-AdCSV (Competitive Ligand Exchange-Adsorptive Cathodic Stripping Voltammetry) of 0.2 μm filtered seawater (FSW) (A) and unfiltered seawater (unFSW) (B) from on-board experiments, as well as on artificial seawater (AQUIL) from laboratory experiments (C). Presence or absence of salp fecal pellets (FPs) is indicated by “+” and “−”, respectively. Control curves correspond to the first sampling time, before the start of the incubation processes and “freezer” [freezing for 10 h followed by thawing in the dark at sea surface temperature (SST)], “dark” (24 h incubation in the dark, SST), “vis” (24 h incubation under natural sunlight, SST), and “UV” (30 min under UV and visible light followed by 24 h in the dark at 4°C) are the different treatments applied. Calibration titrations were performed in artificial seawater (AQUIL) in presence of 5 nM of the siderophore desferrioxamine B (DFB).
All plastic-ware was trace metal cleaned as per the GEOTRACES guidelines (Cutter et al., 2010) to avoid contamination. Experimental samples were sealed in triple bags during equilibration/incubation periods and all the manipulations were carried out in an ISO Class 5 laminar flow hood.
Salp FP fluxes were collected with free-drifting sediment traps at 100 and 300 m and a detailed description is provided by Iversen et al. (2016). Salp FPs are very large and fairly easy to identify. They are very compact and much bigger than FPs from crustaceans like copepods, amphipods, and krill. They are further surrounded by mucus membrane which differentiate salp pellets from physically formed aggregates such as marine snow. The sediment traps were surface attached to a buoy that provide GPS positions during the deployment. Additionally, the sediment trap had two surface floats for buoyancy as well as 12 small air-filled balls (used as wave-breakers). The trap deployments lasted ~24 h. The collection cylinders at the two depths contained a viscous gel that preserved the size, shape, and structure of the collected aggregates, including salp FPs (Iversen et al., 2016). We measured the x, y, and z dimensions of each salp FP in order to determine its volume and used a carbon to volume ratio to calculate carbon fluxes from salp FPs at 100 and 300 m. The carbon to volume ratio was determined from pellets produced during the pellet production incubation (Iversen et al., 2016).
Statistical analysis was performed using Sigma Plot (Systat Software Inc.). Whether for the on-board or laboratory experiments, the various treatments (“freezer,” “dark,” and “VIS” for on-board incubations; “UV” and “dark” for laboratory experiments) did not have a significant impact on any parameter (one-way ANOVA, p = 0.167). A t-test was therefore performed to verify whether the FPs affected analytical parameters over all the treatments. Finally, the concentration differences related to the presence of FPs are presented as averages calculated over all the treatments.
Integrated numbers of salp individuals in the upper 250 m of the water column were between 19.8 and 54.4 individuals.m−2 (Iversen et al., 2016). The daily egestion rate per S. thompsoni individual and the carbon content from salp FPs measured at our sampling site were 0.33 FP ind−1 h−1 (resulting in a daily production of 310 ± 126 FPs m−2 for the upper 250 m) and 17.6 ± 27 μg C FP−1, (n = 33), respectively (Iversen et al., 2016; Table 1). Those values are comparable to the average values reported from salp individuals and their respective FPs collected at the Western Antarctic Peninsula (WAP) of 0.25 FP ind−1 h−1 and 3.62 μg C FP−1, respectively (Phillips et al., 2009; Gleiber et al., 2012). According to calculated sinking rates, salp FPs take about 4–24 h to travel the 100 m upper meters (Iversen et al., 2016). Continuous sampling over 2 weeks using sediment traps at the location of the diatom bloom showed that carbon export by salp FPs products accounted in average for 1.6 mg C m−2 d−1 at 100 m and 0.6 mg C m−2 d−1 at 300 m (Table 1), indicating substantial recycling before 300 m depth (Iversen et al., 2016).
Table 1. Salp fecal pellets (FPs) properties from ANTXXVIII/3 expedition at the sampling site from Iversen et al. (2016).
Results from the determination of leachable Fe from salp FPs and total trace-metals content are summarized in (Table 2). Our analysis revealed a total Fe concentration of 0.33 ± 0.02 nmol Fe FP−1 (n = 2). Fe was in a very refractory form since only 10% was released following acidification to pH 2.0 for 48 h (0.033 ± 0.040 nmol Fe.FP−1, n = 6). Taking into account the total carbon content quantified in salp FPs collected during the same sampling period (17.6 ± 27 μg C FP−1; Iversen et al., 2016), we estimated a Fe:C ratio of 225 μmol Fe mol C−1. Finally, ICP-MS analysis of other trace elements showed that aluminum was the most abundant element found in the FPs (1.45 ± 0.13 nmol FP−1) followed by Fe (0.33 ± 0.02 nmol FP−1), zinc (0.177 ± 0.002 nmol FP−1), copper (0.0.25 ± 0.006 nmol FP−1), nickel (0.011 ± 0.001 nmol FP−1), and molybdenum (0.003 ± 0.000 nmol FP−1).
Table 2. Amount of Fe leached after a period of 48 h at pH 2.0 and FPs trace metals content (iron [Fe], aluminum [Al], nickel [Ni], copper [Cu], zinc [Zn], and molybdenum [Mo]) after 2 months at pH 1.5.
All measured parameters from the on-board incubation are summarized in the top part section of (Table 3). None of the experimental treatments (“freezer,” “dark,” and “VIS”) significantly increased or NOx ( + ) concentrations (p = 0.49 and 0.90 respectively, n = 8). In contrast, an increase in Si(OH)4 concentrations (p = 0.001, n = 8) by 0.53 ± 0.11 μmol L−1 was observed. No variation in dFe concentration was detected for any of the experimental treatments (p = 0.26, n = 14) despite a potential release of 0.66 nmol Fe L−1 estimated from the salp FPs total Fe content. Results from Fe-binding ligand titrations indicated that FPs did not significantly affect LT or log K'Fe'L of the ligand class detected in filtered samples (p = 0.14 and 0.65, n = 8; Figure 1A). Although for unfiltered samples, we observed a slight release of LT from the FPs (between 0.07 and 0.22 nmol LT FP−1; Figure 1B), this effect was, however, not statistically significant (p = 0.088 and p = 0.46, n = 6). HS-like concentrations increased significantly in the presence of FPs (p = 0.031, n = 14) by 16.43 ± 9.45 and 30.86 ± 18.57 μg L−1 for filtered and unfiltered samples, respectively, corresponding to a release of 8.22 ± 4.72 and 15.43 ± 9.28 μg HS-like.FP−1 d−1 (since we had a concentration of two FPs per liter).
Table 3. Concentrations of macronutrients (phosphate [], orthosilicate [Si(OH)4], nitrate [NOx = +]), dissolved iron (dFe), humic-substances-like [HS-like], total ligand [LT] as well as stability constant [log K'FeL]) data are given.
All measured parameters from the laboratory incubation are summarized in the bottom section of the (Table 3). For both treatments (“UV” and “dark”), the presence of FPs did not have any impact on the dFe concentrations (p = 0.73, n = 4). Similarly to the on-board experiments, we observed a release of humic substances (p = 0.002, n = 4) of 8.74 ± 0.52 μg L−1 corresponding to a daily release of 0.78 ± 0.05 μg HS-like FP−1 d−1. Based on the Fe organic complexation analyses, no ligands were detected in any of the samples (both treatments, with and without FPs) since the titration curves were virtually linear (Figure 1C).
Salps were collected at the center of a stable diatom bloom covering an area of about 8000 km2 between the Antarctic Polar Front and the Southern Antarctic Circumpolar Current Front (Strass et al., 2016). Compared to previous studies (Pakhomov et al., 2006; Phillips et al., 2009), low to medium abundances of salp were observed in the upper 250 m of the water column (19.8 to 54.4 ind m−2; Iversen et al., 2016). Particulate organic carbon per day produced as salp FPs was 3.3 mg C.m−2, this is equivalent to salp ingesting and releasing daily only ≈ 0.2% of the net primary production (NPP) estimated in the study area (1751 mg C m−2, Hoppe et al., 2015). The total POC flux averaged over the 3 weeks of study at 100 m was 18% of the NPP, 317 ± 175 mg C m−2 d−1. High POC transfer efficiencies were observed between 100 m and 300 m at ~60% (flux of 176 ± 126 mg C m−2 d−1 at 300 m) mainly due to efficient sinking of diatoms (Roca Martí et al., 2016). Large fast sinking salp FPs are remarkably more efficiently exported below 100 m (about ~50% of the salp FP production is exported vs. ~20% POC). In their transit from 100 to 300 m, the transfer efficiencies are similar at ~60% (Figure 2).
Figure 2. Graphic overview of carbon (C) and dissolved iron (dFe) fluxes generated by salp fecal pellets (FPs) as well as total particulate organic carbon (POC) fluxes at different depths through the water column, between the surface (e.g., site of production) and 100 m and between 100 and 300 m. PP is for the average daily primary production in mg C m−2 (Hoppe et al., 2015), FPC_PROD and FPdFe_PROD are the total average daily salp C and dFe production in mg C m−2 and nmol Fe m−2, respectively. FPdFe_PROD were calculated using the Fe:C ratio of 225 μmol Fe mol−1 C−1. Total POC fluxes and C fluxes generated by salp FPs were reported from Roca Martí et al. (2016) and Iversen et al. (2016), respectively.
FPs are composed predominantly of organic compounds directly related to the material ingested (Madin, 1982; Manno et al., 2015). Therefore, the increasing dSi(OH)4 concentrations observed in presence of FPs in our on-board experiments can be linked to the high ingestion rates of the salps at the time of sampling. Accordingly, dSi(OH)4 concentrations were depleted in the collected FSW (2.93 μmol L−1, Table 3). Our incubations showed that salp FPs released 265 nmol dSi(OH)4 FP−1 d−1. Using the daily salp FP production (Table 1) determined during the cruise, the integrated Si(OH)4 release from salp FPs was 82.2 μmol dSi(OH)4 m−2 d−1 in the upper 250 m (Table 4). Despite concentrations below 3 μmol L−1 dSi(OH)4 in the upper 40 m, the Si(OH)4 standing stock in the upper water column was stable with values ranging between ~600 and 700 mmol dSi(OH)4 m−2 (Hoppe et al., 2015). Hence, the daily added dSi(OH)4 concentrations from salp FPs were almost one order of magnitude below the standing stock, suggesting that the release observed of dSi(OH)4 (corresponding to our three physico-chemical experimental treatments: “freezer,” “dark,” and “VIS”) would not have substantially contribute to the maintenance of the observed diatom bloom.
Table 4. Estimated orthosilicate (Si(OH)4), humic substances-like (HS-like), and dissolved iron (dFe) fluxes generated by salp FPs from on-board and laboratory experiments and Fe:C ratio.
Satellite images showed that the diatom bloom maintained high chl a concentrations (> μg L−1) for many weeks (weeks before and after our period of monitoring), implying sufficient Fe supply over all this period. DFe concentrations remained low at 0.1–0.2 in the upper 100 m during our 3 weeks sampling (Laglera et al., 2013; Hoppe et al., 2015). Due to the absence of major “external” Fe inputs at our sampling site, Fe recycling processes should have consequently played a major role in the maintaining of the diatom bloom. From our experimental results, the different treatments that we applied to the FPs did not lead to the release of dFe suggesting that salp FPs could have barely contributed to Fe recycling in the euphotic zone. However, we cannot discount biological processes such as zooplankton grazing on fecal products (coprophagy) and microbial degradation that were not evaluated in this work (Turner, 2002; Dagg et al., 2014; Morata and Seuthe, 2014). Acidification to pH 2.0, a treatment too mild to leach intracellular Fe from intact planktonic cells (Lannuzel et al., 2011), released only 10% of Fe associated with salp FPs (Table 2). In comparison, the same treatment was found to be strong enough to leach ~100% of the Fe in FPs egested by individuals of the SO copepod Calanus simillimus (Laglera et al., unpublished data). This difference might be explained by different ingestion procedures and gut passage efficiencies. Copepods crack and grind what they capture before ingestion, potentially allowing their FPs to be more easily degradable, contrary to the salp that are purely passive filterers (Kiørboe, 2011). In this sense, our results show that the Fe inside salp FPs is in extremely refractory form (possibly due to a low digestion efficiency), supporting the result that physico-chemical processes did not alter our salp FPs content. Accordingly, the zooplankton group dominant in a determined area of the ocean can have an important effect on the percentage of Fe that is recycled in the euphotic zone.
The Fe:C ratio estimated in our salp FPs (225 μmol Fe mol C−1; Table 4) is relatively high in comparison with the commonly observed Fe:C ratios in plankton of HNLC waters, which are in the order of 10–50 μmol Fe mol−1 C−1 (Twining and Baines, 2013; Boyd et al., 2015). In the absence of other studies, it seems from our results that salps have neither significant Fe metabolic requirements nor do they accumulate Fe in their tissues. The enrichment of Fe in salp FPs determined during our experiments seems to demonstrate that most of the Fe ingested by the salps was packed into FPs, contrary to the copepods for whom half of ingested Fe was regenerated to dissolved forms (Schmidt et al., 1999) during the ingestion in a process denoted sloppy feeding. In the same way, using salp FP fluxes we determined an export at 100 and 300 m depth of ~30 nmol Fe m−2 d−1 and ~11.3 nmol Fe m−2 d−1 respectively (Table 4). In comparison to the total dFe stock in the upper 100 m at the sampling location (~10–15 μmol Fe m−2; Laglera et al., 2013; Hoppe et al., 2015), our export flux represents only ~0.1% of this dFe standing stock, which is in agreement with low Fe exports estimated in previous works where salp FPs represent ~0.3% of the estimated total Fe used by phytoplankton across the SO (Boyd et al., 2012). Taking into account previous estimates of new Fe supplies resulting from island wake (vertical flux of 4 nmol Fe m−2 d−1, Blain et al., 2007), atmospheric deposition (3.2–51.2 nmol Fe m−2 d−1, Wagener et al., 2008), and seasonal sea-ice melt (<10–800 nmol Fe m−2 d−1, Lancelot et al., 2009), our Fe sequestration flux (at 300 m) might significantly reduce new Fe supply in remote areas of limited inputs and high salp concentrations.
Regarding the other trace-metals, Fe was the most abundant essential element after Al, followed by Zn, Cu, Ni, and Mo, which is in accordance with the reported trace metal composition of marine phytoplankton (Twining and Baines, 2013). The high Al content determined in our salp FPs could be explained by the fact that the diatoms are able to take up significant amount of Al (both adsorption and incorporation into soft tissues) during bloom events (Moran and Moore, 1988; Ren et al., 2011) despite its recognized toxicity toward numerous organisms (Gillmore et al., 2016).
Fe-binding ligands are key compounds that control both Fe reactivity (Barbeau, 2006; Rijkenberg et al., 2006) and bioavailability to phytoplankton (Hassler et al., 2011a,b, 2015; Norman et al., 2014). In this study, we could not detect, after any treatment (“freezer,” “VIS,” “UV,” and “dark”), a release of ligands by salp FPs. Such an observation was not due to an absence of ligands in the pellets, but possibly to the reduction of the assimilation and digestion efficiency of the ingested food under blooming conditions referred to above (Von Harbou, 2009); i.e., salps could not break the hard frustule of a good percentage of the ingested diatoms.
Zooplankton grazing is known to be an important autochthonous source of colored and fluorescent organic matter, including marine humic substances (Ortega-Retuerta et al., 2009; Nakayama et al., 2011). In fact, Fe-binding HS-like compounds were released in the presence of salp FPs and we could calculate an HS-like potential flux from FPs of 2.5 and 0.24 mg HS-like m−2 d−1 for the field and laboratory work, respectively (Table 4). The difference could be explained by the fact that the field-work was carried out in SSW containing bacteria and viruses which could have enhanced the release of HS-like compounds. We therefore concluded that salp FPs could be a source of weak Fe-binding ligands with low log K'Fe'L likely below our analytical detection limit (αFe'SA = 20.1), explaining why we did not detect them by CLE-AdCSV. In line with this, previous work on humic substances showed that the log K'Fe'L values of their complexes with Fe were around 10.6 (Laglera and van den Berg, 2009), nearly 1 log unit below that of other major ligand groups. Hence, our results show that HS-like compounds contributed to the pool of photo-sensitive HS material likely to be autochtonously produced, such as exopolymeric substances or marine humic substances (Obernosterer and Herndl, 2000; Barbeau et al., 2003; Hassler et al., 2011a; Laglera et al., 2011). Unfortunately, to date, the nature of organic compounds contributing to the pool of HS material detected by CSV remains poorly characterized.
The SO plays a critical role in global ocean circulation (Lumpkin and Speer, 2007) and acts as a significant atmospheric CO2 sink (Bopp et al., 2001; Sabine et al., 2004). As such, the ocean circulation dynamics of the SO globally affects biogeochemical cycles, biodiversity and climate regulation. The hypothesized increase in salp dominance in the SO due to a depletion of sea-ice (Atkinson et al., 2004) could enhance their annual contribution to carbon vertical flux. Compared to krill, salps consume more food more effectively (Perissinotto and Pakhomov, 1998), produce larger and denser FPs with high carbon content (Gleiber et al., 2012) and have higher sinking velocity (Phillips et al., 2009; Turner, 2015). Increasing abundances of salps over krill could imply important changes to the lower and higher trophic levels (Fraser and Hofmann, 2003) with strong implications for the biological pump activity and associated carbon sequestration.
Based on our incubation experimental settings, salps do not easily release strong Fe ligands as it is the case during grazing by other zooplankton groups such as copepods (Sato et al., 2007). However, the rapid release of weak Fe-binding ligands from salp FPs could affect Fe bioavailability by enhancing Fe solubility and accelerating Fe uptake (Hassler et al., 2011a,b). Therefore, Fe speciation in the presence of salps is difficult to predict as it will depend on food quality and egestion rate, both strongly dependent on the phytoplankton community and chlorophyll concentration present (Von Harbou, 2009).
Our study shows that salp FPs store high concentrations of Fe that are not released rapidly enough by physicochemical processes to enter the recycling loop before sinking below the euphotic zone. Differences in the decrease of organic carbon associated with the bulk of POC (which included particles such as single diatom cells, marine snow, and copepod and krill FPs) and with the salp FPs between the surface (e.g., site of production) and 100 m, indeed suggest that the export efficiencies of salp FPs are 2.5-fold higher (Figure 2). Nevertheless, the organic carbon associated with FPs decrease by a factor of two as they settle down to 100 m, suggesting that biological recycling of FPs occur. In our case, relatively low salp numbers, compared to other reports from the Southern Ocean (Pakhomov et al., 2002; Atkinson et al., 2004; Phillips et al., 2009), allowed that Fe export was kept to a minimum, resulting in less Fe export that what would be exported in situation of high salp abundances. Under such conditions, with a carbon export in the form of salp FPs of about 20 mg C m−2 d−1 at 300 m depth (Phillips et al., 2009), a Fe:C ratio of 225 μmol Fe mol C−1 would generate an Fe export of ≈ 375 nmol Fe m−2 d−1 which would deplete dFe stocks in HNLC areas during a period of a few days with consequences for phytoplankton Fe limitation.
More studies on biological remineralization processes associated with salp FPs are needed to estimate whether salp FPs contribute to Fe export. Due to changes in the dominance of zooplankton predicted across the SO and its potential impact for Fe biogeochemistry, comparative studies on the impact of grazing activities and FP degradation rates between salps, krill and copepods are urgently needed.
DC contributed to the experiments, chemical analyses, interpretation of data and redaction of the manuscript. LN contributed to the experiments, and redaction of the manuscript. JS contributed to the analyses. MI contributed to the interpretation of data as well as the manuscript. ST participated to the experiments and to manuscript. LL contributed to the analyses, interpretation of the data and the manuscript. CH contributed to the experimental design, interpretation of data as well to the manuscript.
DC was funded by the Swiss National Science Foundation (PP00P2_138955), LN by the UTS Chancellor Fellowship and CH by the Australian Research Council (Discovery Project DP1092892 and LIEF grand LE0989539) and the Swiss National Science Foundation (PP00P2_138955). LL and JS participation was funded by the MINECO of Spain (Grant CGL2010-11846-E) and the Government of the Balearic Islands (Grant AAEE083/09). ST was funded by the Deutsche Forschungsgemeinschaft (DFG) in the framework of the priority programme “Antarctic Research with comparative investigations in Arctic ice areas,” project TR 899/2 as well as the Helmholtz Impulse Fond (Helmholtz Young Investigators Group EcoTrace). MI was funded by the Helmholtz Association for the Helmholtz Young Investigator Group SeaPump.
The authors declare that the research was conducted in the absence of any commercial or financial relationships that could be construed as a potential conflict of interest.
We would like to thank Captain and crew of the RV. Polarstern, as well as the scientific party, for their assistance and support during the ANT-XXVIII-3 voyage. We especially thank E. Pakhomov and B. Hunt for their assistance with the collection and maintenance of the salps and S. Ossebaar for the on-board macronutrient analysis.
Abualhaija, M. M., and van den Berg, C. M. G. (2014). Chemical speciation of iron in seawater using catalytic cathodic stripping voltammetry with ligand competition against salicylaldoxime. Mar. Chem. 164, 60–74. doi: 10.1016/j.marchem.2014.06.005
Alcaraz, M., Almeda, R., Duarte, C. M., Horstkotte, B., Lasternas, S., and Agustí, S. (2014). Changes in the C, N and P cycles by the predicted salps-krill shift in the Southern Ocean. Front. Mar. Sci. 1:45. doi: 10.3389/fmars.2014.00045
Atkinson, A., Siegel, V., Pakhomov, E., and Rothery, P. (2004). Long-term decline in krill stock and increase in salps within the Southern Ocean. Nature 432, 100–103. doi: 10.1038/nature02996
Barbeau, K. (2006). Photochemistry of organic iron(III) complexing ligands in oceanic systems. Photochem. Photobiol. 82, 1505–1516. doi: 10.1111/j.1751-1097.2006.tb09806.x
Barbeau, K., Rue, E. L., Trick, C. G., Bruland, K. W., and Butler, A. (2003). Photochemical reactivity of siderophores produced by marine heterotrophic bacteria and cyanobacteria based on characteristic Fe(III) binding groups. Limnol. Oceanogr. 3, 1069–1078. doi: 10.4319/lo.2003.48.3.1069
Blain, S., Queguiner, B., Armand, L., Belviso, S., Bombled, B., Bopp, L., et al. (2007). Effect of natural iron fertilization on carbon sequestration in the Southern Ocean. Nature 446, 1070–1074. doi: 10.1038/nature05700
Bopp, L., Monfray, P., Aumont, O., Dufresne, J.-L., LeTreut, H., Madec, G., et al. (2001). Potential impact of climate change on marine export production. Glob. Biogeochem. Cycles 15, 81–99. doi: 10.1029/1999gb001256
Boyd, P., Strzepek, R., Ellwood, M., Hutchins, D., Nodder, S., Twining, B., et al. (2015). Why are biotic iron pools uniform across high-and low-iron pelagic ecosystems? Global Biogeochem. Cycles 29, 1028–1043. doi: 10.1002/2014GB005014
Boyd, P. W., Arrigo, K. R., Strzepek, R., and van Dijken, G. L. (2012). Mapping phytoplankton iron utilization: insights into southern ocean supply mechanisms. J. Geophys. Res. 117:C06009. doi: 10.1029/2011JC007726
Boyd, P. W., Ibisanmi, E., Sander, S. G., Hunter, K. A., and Jackson, G. A. (2010). Remineralization of upper ocean particles: implications for iron biogeochemistry. Limnol. Oceanogr. 55, 1271–1288. doi: 10.4319/lo.2010.55.3.1271
Boyd, P. W., Law, C. S., Hutchins, D. A., Abraham, E. R., Croot, P. L., Ellwood, M., et al. (2005). FeCycle: attempting an iron biogeochemical budget from a mesoscale SF6 tracer experiment in unperturbed low iron waters. Global Biogeochem. Cycles 19:GB4S20. doi: 10.1029/2005GB002494
Cutter, G., Andersson, P., Codispoti, L., Croot, P., François, R., Lohan, M. C., et al (2010). Sampling and Sample-Handling Protocols for GEOTRACES Cruises. Available online at: http://www.geotraces.org/libraries/documents/Intercalibration/Cookbook.pdf; http://epic.awi.de/34484/1/Cookbook.pdf
Dagg, M. J., Jackson, G. A., and Checkley, D. M. (2014). The distribution and vertical flux of fecal pellets from large zooplankton in Monterey bay and coastal California. Deep Sea Res. 94(Pt I), 72–86. doi: 10.1016/j.dsr.2014.09.001
de Jong, J., Schoemann, V., Lannuzel, D., Croot, P., deBaar, H., and Tison, J.-L. (2012). Natural iron fertilization of the Atlantic sector of the Southern Ocean by continental shelf sources of the Antarctic Peninsula. J. Geophys. Res. 117:G01029. doi: 10.1029/2011jg001679
Duce, R. A., and Tindale, N. W. (1991). Atmospheric transport of iron and its deposition in the ocean. Limnol. Oceanogr. 36, 1715–1726. doi: 10.4319/lo.1991.36.8.1715
Ducklow, H., Clarke, A., Dickhut, R., Doney, S. C., Geisz, H., Huang, K., et al. (2012). “The marine system of the western Antarctic Peninsula,” in Antarctic Ecosystems: An Extreme Environment in a Changing World, eds A. D. Rogers, N. M. Johnston, E. J. Murphy, A. Clarke (London: Blackwell), 121–159.
Fraser, W. R., and Hofmann, E. E. (2003). A predator's perspective on causal links between climate change, physical forcing and ecosystem response. Mar. Ecol. Prog. Ser. 265, 1–15. doi: 10.3354/meps265001
Giering, S. L. C., Sanders, R., Lampitt, R. S., Anderson, T. R., Tamburini, C., Boutrif, M., et al. (2014). Reconciliation of the carbon budget in the ocean's twilight zone. Nature 507, 480–483. doi: 10.1038/nature13123
Gillmore, M. L., Golding, L. A., Angel, B. M., Adams, M. S., and Jolley, D. F. (2016). Toxicity of dissolved and precipitated aluminium to marine diatoms. Aquat. Toxicol. 174, 82–91. doi: 10.1016/j.aquatox.2016.02.004
Gleiber, M. R., Steinberg, D. K., and Ducklow, H. W. (2012). Time series of vertical flux of zooplankton fecal pellets on the continental shelf of the western Antarctic Peninsula. Mar. Ecol. Prog. Ser. 471, 23–36. doi: 10.3354/meps10021
Hassler, C. S., Alasonati, E., Mancuso Nichols, C. A., and Slaveykova, V. I. (2011a). Exopolysaccharides produced by bacteria isolated from the pelagic Southern Ocean—Role in Fe binding, chemical reactivity, and bioavailability. Mar. Chem. 123, 88–98. doi: 10.1016/j.marchem.2010.10.003
Hassler, C. S., Norman, L., Mancuso Nichols, C. A., Clementson, L. A., Robinson, C., Schoemann, V., et al. (2015). Iron associated with exopolymeric substances is highly bioavailable to oceanic phytoplankton. Mar. Chem. 173, 136–147. doi: 10.1016/j.marchem.2014.10.002
Hassler, C. S., Schoemann, V., Nichols, C. M., Butler, E. C., and Boyd, P. W. (2012). “Iron bioavailability in the Southern Ocean” in Oceanography and Marine Biology: An Annual Review, Vol. 50, eds R. N. Gibson, R. J. A. Atkinson, J. D. M. Gordon, R. N. Hughes (Boca Raton, FL: CRC Press), 1–64.
Hassler, C. S., Schoemann, V., Mancuso Nichols, C., Butler, E. C. V., and Boyd, P. W. (2011b). Saccharides enhance iron bioavailability to Southern Ocean phytoplankton. Proc. Natl. Acad. Sci. U.S.A. 108, 1076–1081. doi: 10.1073/pnas.1010963108
Hoppe, C. J. M., Klaas, C., Ossebaar, S., Soppa, M. A., Cheah, W., Laglera, L. M., et al. (2015). Controls of primary production in two phytoplankton blooms in the Antarctic Circumpolar Current. Deep Sea Res. Part II Top. Stud. Oceanogr. doi: 10.1016/j.dsr2.2015.10.005. [Epub ahead of print].
Hutchins, D. A., and Bruland, K. W. (1994). Grazer-mediated regeneration and assimilation of Fe, Zn and Mn from planktonic prey. Mar. Ecol. Prog. Ser. 110, 259–269. doi: 10.3354/meps110259
Iversen, M. H., Pakhomov, E. A., Hunt, B. P. V., van der Jagt, H., Wolf-Gladrow, D., and Klaas, C. (2016). Sinkers or floaters? contribution from salp fecal pellets to the export flux during a large bloom event in the Southern Ocean. Deep Sea Res. II. [Epub ahead of print].
Kiørboe, T. (2011). How zooplankton feed: mechanisms, traits and trade-offs. Biol. Rev. Camb. Philos. Soc. 86, 311–339. doi: 10.1111/j.1469-185X.2010.00148.x
Laglera, L. M., Battaglia, G., and van den Berg, C. M. G. (2007). Determination of humic substances in natural waters by cathodic stripping voltammetry of their complexes with iron. Anal. Chim. Acta 599, 58–66. doi: 10.1016/j.aca.2007.07.059
Laglera, L. M., Battaglia, G., and van den Berg, C. M. G. (2011). Effect of humic substances on the iron speciation in natural waters by CLE/CSV. Mar. Chem. 127, 134–143. doi: 10.1016/j.marchem.2011.09.003
Laglera, L. M., Santos-Echeandía, J., Caprara, S., and Monticelli, D. (2013). Quantification of iron in seawater at the low picomolar range based on optimization of bromate/ammonia/dihydroxynaphtalene system by catalytic adsorptive cathodic stripping voltammetry. Anal. Chem. 85, 2486–2492. doi: 10.1021/ac303621q
Laglera, L. M., and van den Berg, C. M. G. (2009). Evidence for geochemical control of iron by humic substances in seawater. Limnol. Oceanogr. 54, 610–619. doi: 10.4319/lo.2009.54.2.0610
Lancelot, C., de Montety, A., Goosse, H., Becquevort, S., Schoemann, V., Pasquer, B., et al. (2009). Spatial distribution of the iron supply to phytoplankton in the Southern Ocean: a model study. Biogeosciences 6, 2861–2878. doi: 10.5194/bg-6-2861-2009
Lannuzel, D., Bowie, A. R., Remenyi, T., Lam, P., Townsend, A., et al. (2011). Distributions of dissolved and particulate iron in the sub-Antarctic and Polar Frontal Southern Ocean (Australian sector). Deep Sea Res. II 58, 2094–2112. doi: 10.1016/j.dsr2.2011.05.027
Lannuzel, D., Schoemann, V., de Jong, J., Tison, J.-L., and Chou, L. (2007). Distribution and biogeochemical behaviour of iron in the East Antarctic sea ice. Mar. Chem. 106, 18–32. doi: 10.1016/j.marchem.2006.06.010
Lumpkin, R., and Speer, K. (2007). Global ocean meridional overturning. J. Phys. Oceanogr. 37, 2550–2562. doi: 10.1175/JPO3130.1
Madin, L. P. (1982). Production, composition and sedimentation of salp fecal pellets in oceanic waters. Mar. Biol. 67, 39–45. doi: 10.1007/bf00397092
Mahmood, A., Abualhaija, M. M., van den Berg, C. M. G., and Sander, S. G. (2015). Organic speciation of dissolved iron in estuarine and coastal waters at multiple analytical windows. Mar. Chem. 177, 706–719. doi: 10.1016/j.marchem.2015.11.001
Manno, C., Stowasser, G., Enderlein, P., Fielding, S., and Tarling, G. A. (2015). The contribution of zooplankton faecal pellets to deep-carbon transport in the Scotia Sea (Southern Ocean). Biogeosciences 12, 1955–1965. doi: 10.5194/bg-12-1955-2015
Martin, J. H., Fitzwater, S. E., and Gordon, R. M. (1990). Iron deficiency limits phytoplankton growth in Antarcticwaters. Global Biogeochem. Cycles 4, 5–12. doi: 10.1029/GB004i001p00005
Moore, J. K., and Braucher, O. (2008). Sedimentary and mineral dust sources of dissolved iron to the world ocean. Biogeosciences 5, 631–656. doi: 10.5194/bg-5-631-2008
Moran, S. B., and Moore, R. M. (1988). Evidence from mesocosm studies for biological removal of dissolved aluminium from sea water. Nature 335, 706–708. doi: 10.1038/335706a0
Morata, N., and Seuthe, L. (2014). Importance of bacteria and protozooplankton for faecal pellet degradation. Oceanologia 56, 565–581. doi: 10.5697/oc.56-3.565
Nakayama, Y., Fujita, S., Kuma, K., and Shimada, K. (2011). Iron and humic-type fluorescent dissolved organic matter in the Chukchi Sea and Canada Basin of the western Arctic Ocean. J. Geophys.l Res. Oceans 116, C07031. doi: 10.1029/2010jc006779
Norman, L., Cabanes, D. J. E., Blanco-Ameijeiras, S., Moisset, S. A. M., and Hassler, C. S. (2014). Iron biogeochemistry in aquatic systems: from source to bioavailability. Chimia 68, 764–771. doi: 10.2533/chimia.2014.764
Norman, L., Worms, I. A. M., Angles, E., Bowie, A. R., Nichols, C. M., Ninh Pham, A., et al. (2015). The role of bacterial and algal exopolymeric substances in iron chemistry. Mar. Chem. 173, 148–161. doi: 10.1016/j.marchem.2015.03.015
Obernosterer, I., and Herndl, G. J. (2000). Differences in the optical and biological reactivity of the humic and non-humic dissolved organic carbon component in two contrasting coastal marine environments. Limnol. Oceanogr. 45, 1120–1129. doi: 10.4319/lo.2000.45.5.1120
Omanović, D., Garnier, C., and Pižeta, I. (2015). ProMCC: an all-in-one tool for trace metal complexation studies. Mar. Chem. 173, 25–39. doi: 10.1016/j.marchem.2014.10.011
Ortega-Retuerta, E., Frazer, T. K., Duarte, C. M., Ruiz-Halpern, S., Tovar_Sanchez, A., Arrieta, J. M., et al. (2009). Biogeneration of chromophoric dissolved organic matter by bacteria and krill in the southern ocean. Limnol. Oceanogr. 54, 1941–1950. doi: 10.4319/lo.2009.54.6.1941
Pakhomov, E. A., Dubischar, C. D., Strass, V., Brichta, M., and Bathmann, U. V. (2006). The tunicate Salpa thompsoni ecology in the Southern Ocean. I. Distribution, biomass, demography and feeding ecophysiology. Mar. Biol. 149, 609–623. doi: 10.1007/s00227-005-0225-9
Pakhomov, E. A., Froneman, P. W., and Perissinotto, R. (2002). Salp/krill interactions in the Southern Ocean: spatial segregation and implications for the carbon flux. Deep Sea Res. Part II Top. Stud. Oceanogr. 49, 1881–1907. doi: 10.1016/S0967-0645(02)00017-6
Perissinotto, R., and Pakhomov, E. A. (1998). The trophic role of the tunicate Salpa thompsoni in the Antarctic marine ecosystem. J. Mar. Syst. 17, 361–374. doi: 10.1016/S0924-7963(98)00049-9
Phillips, B., Kremer, P., and Madin, L. (2009). Defecation by Salpa thompsoni and its contribution to vertical flux in the Southern Ocean. Mar. Biol. 156, 455–467. doi: 10.1007/s00227-008-1099-4
Pisani, O., Yamashita, Y., and Jaffé, R. (2011). Photo-dissolution of flocculent, detrital material in aquatic environments: contributions to the dissolved organic matter pool. Water Res. 45, 3836–3844. doi: 10.1016/j.watres.2011.04.035
Price, N. M., Harrison, G. I., Hering, J. G., Hudson, R. J., Nirel, P. M. V., and Palenik, B. (1989). Preparation and chemistry of the artificial algal culture medium aquil. Biol. Oceanogr. 6, 443–461.
Ren, J.-L., Zhang, G.-L., Zhang, J., Shi, J.-H., Liu, S.-M., Li, F.-M., et al. (2011). Distribution of dissolved aluminium in the Southern Yellow Sea: influences of a dust storm and the spring bloom. Mar. Chem. 125, 69–81. doi: 10.1016/j.marchem.2011.02.004
Rijkenberg, M. J. A., Gerringa, L. J. A., Carolus, V. E., Velzeboer, I., and de Baar, H. J. W. (2006). Enhancement and inhibition of iron photoreduction by individual ligands in open ocean seawater. Geochim. Cosmochim. Acta 70, 2790–2805. doi: 10.1016/j.gca.2006.03.004
Roca Martí, M., Puigcorbé, V., Iversen, M. H., van der Loeff, M. R., Klaas, C., Cheah, W., et al. (2016). High particulate organic carbon export during the decline of a vast diatom bloom in the Atlantic sector of the Southern Ocean. Deep Sea Res. II. doi: 10.1016/j.dsr2.2015.12.007. [Epub ahead of print].
Rontani, J.-F. (2008). “Photooxidative and autoxidative degradation of lipid components during the senescence of phototrophic organisms” in Phytochemistry Research Progress, ed T. Matsumoto (Hauppauge, NY: Nova Science Publishers), 115–144.
Sabine, C. L., Feely, R. A., Gruber, N., Key, R. M., Lee, K., Bullister, J. L., et al. (2004). The oceanic sink for anthropogenic CO2. Science 305, 367–371. doi: 10.1126/science.1097403
Sarthou, G., Vincent, D., Christaki, U., Obernosterer, I., Timmermans, K. R., and Brussaard, C. P. D. (2008). The fate of biogenic iron during a phytoplankton bloom induced by natural fertilization: impact of copepod grazing. Deep Sea Res. II. 55, 734–751. doi: 10.1016/j.dsr2.2007.12.033
Sato, M., Takeda, S., and Furuya, K. (2007). Iron regeneration and organic iron(III)-binding ligand production during in situ zooplankton grazing experiment. Mar. Chem. 106, 471–488. doi: 10.1016/j.marchem.2007.05.001
Schmidt, M. A., Zhang, Y. H., and Hutchins, D. A. (1999). Assimilation of Fe and carbon by marine copepods from Fe-limited and Fe-replete diatom prey. J. Plankton Res. 21, 1753–1764. doi: 10.1093/plankt/21.9.1753
Strass, V. H., Leach, H., Prandke, H., Donnelly, M., Bracher, A. U., and Wolf-Gladrow, D. A. (2016). The physical environmental conditions for biogeochemical differences along the Antarctic Circumpolar Current in the Atlantic Sector during late austral summer 2012. Deep Sea Res. II. doi: 10.1016/j.dsr2.2016.05.018. [Epub ahead of print].
Strzepek, R. F., Maldonado, M. T., Higgins, J. L., Hall, J., Safi, K., Wilhelm, S. W., et al. (2005). Spinning the “Ferrous Wheel”: the importance of the microbial community in an iron budget during the FeCycle experiment. Global Biogeochem. Cycles 19:GB4S26. doi: 10.1029/2005GB002490
Tagliabue, A., Sallée, J.-B., Bowie, A. R., Lévy, M., Swart, S., and Boyd, P. W. (2014). Surface-water iron supplies in the Southern Ocean sustained by deep winter mixing. Nat. Geosci. 7, 314–320. doi: 10.1038/ngeo2101
Tovar-Sanchez, A., Duarte, C. M., Hernandez-Leon, S., and Sanudo-Wilhelmy, S. A. (2007). Krill as a central node for iron cycling in the Southern Ocean. Geophys. Res. Lett. 34, L11601. doi: 10.1029/2006GL029096
Turner, J. T. (2002). Zooplankton fecal pellets, marine snow and sinking phytoplankton blooms. Aquat. Microb. Ecol. 27, 57–102. doi: 10.3354/ame027057
Turner, J. T. (2015). Zooplankton fecal pellets, marine snow, phytodetritus and the ocean's biological pump. Prog. Oceanogr. 130, 205–248. doi: 10.1016/j.pocean.2014.08.005
Twining, B. S., and Baines, S. B. (2013). The Trace Metal Composition of Marine Phytoplankton. Annu. Rev. Mar. Sci. 5, 191–215. doi: 10.1146/annurev-marine-121211-172322
Von Harbou, L. (2009). Trophodynamics of Salps in the Atlantic Southern Ocean. Dissertation's thesis, University of Bremen, Germany.
Wagener, T., Guieu, C., Losno, R., Bonnet, S., and Mahowald, N. (2008). Revisiting atmospheric dust export to the Southern Hemisphere ocean: biogeochemical implications. Global Biogeochem. Cycles 22:GB2006. doi: 10.1029/2007GB002984
Keywords: Salpa thompsoni, fecal pellets, iron speciation, southern ocean, iron cycling, humic substances
Citation: Cabanes DJE, Norman L, Santos-Echeandía J, Iversen MH, Trimborn S, Laglera LM and Hassler CS (2017) First Evaluation of the Role of Salp Fecal Pellets on Iron Biogeochemistry. Front. Mar. Sci. 3:289. doi: 10.3389/fmars.2016.00289
Received: 24 November 2016; Accepted: 21 December 2016;
Published: 10 January 2017.
Edited by:
Douglas Patrick Connelly, National Oceanography Centre, UKReviewed by:
Katlin Louise Bowman, University of California, Santa Cruz, USACopyright © 2017 Cabanes, Norman, Santos-Echeandía, Iversen, Trimborn, Laglera and Hassler. This is an open-access article distributed under the terms of the Creative Commons Attribution License (CC BY). The use, distribution or reproduction in other forums is permitted, provided the original author(s) or licensor are credited and that the original publication in this journal is cited, in accordance with accepted academic practice. No use, distribution or reproduction is permitted which does not comply with these terms.
*Correspondence: Damien J. E. Cabanes, RGFtaWVuLkNhYmFuZXNAdW5pZ2UuY2g=
Disclaimer: All claims expressed in this article are solely those of the authors and do not necessarily represent those of their affiliated organizations, or those of the publisher, the editors and the reviewers. Any product that may be evaluated in this article or claim that may be made by its manufacturer is not guaranteed or endorsed by the publisher.
Research integrity at Frontiers
Learn more about the work of our research integrity team to safeguard the quality of each article we publish.