- 1Department of Zoology, Faculty of Life Sciences, Tel-Aviv University, Tel Aviv, Israel
- 2School of Marine Science, Ruppin Academic Center, Mikhmoret, Israel
High Microbial Abundance (HMA) sponges constitute a guild of suspension-feeding sponges that host vast populations of symbiotic microbes. These symbionts mediate a complex series of biogeochemical transformations that fuel the holobiont's metabolism. Although sponges are aerobic animals, suboxic and anaerobic bacteria are known to reside within their bodies. However, little is known about the chemical characteristics of the sponge environment in which they occur and almost no data are available regarding the dissolved oxygen (DO) dynamics inside the holobiont in its natural habitat. In this study we examined the oxygen dynamics in situ in the HMA sponge Theonella swinhoei. A submersed data-logging system equipped with micro-sensors was used to continuously record DO concentrations inside the sponge body and in its outflowing water for up to 48 h. Actively pumping sponges exhibited high DO removal rates punctuated with short bursts of extreme DO uptake (>90 μmol DO Lpumped−1), never before observed in sponges. Such a high DO removal rate indicates the consumption of a considerable amount of reduced matter, far exceeding the available sources in the surrounding water of the oligotrophic coral-reef ecosystem inhabited by this sponge. The inner body of the sponge remained suboxic throughout the experiments, with short events of further rapid DO concentration decline. Moreover, DO concentrations measured in the body and in the outflowing water were found to be uncorrelated. Our findings support a previous hypothesis of bacterial symbiont farming by the sponge as a potential source for acquiring reduced material. Moreover, this suggests a complex and highly localized control of the holobiont's metabolism, probably associated with the microbial community's metabolism. Our results indicate that temporal micro-environments exist in the sponge at alternating locations, providing suitable conditions for the activity of its anaerobic microbial symbionts.
Introduction
Sponges are an ancient (>800 myr) and successful phylum, inhabiting all marine and some freshwater ecosystems (Taylor et al., 2007). Most sponges are filter-feeders, able to filter up to 50,000 times their body volume per day (Weisz et al., 2008). Part of their evolutionary success is ascribed to their intricate association with a diverse consortium of microorganisms (Thomas et al., 2016), which provide a multitude of services for their host, from chemical defense (Guo et al., 2011) to recycling waste (Mohamed et al., 2010; Maldonado et al., 2012). Sponges, therefore, must necessarily maintain a supportive environment for their symbionts.
Even though sponges have been the subject of research for over a century, very few data exist on the physio-chemical conditions, specifically oxygen dynamics, that occur inside their bodies. Such data are of utmost importance for studying the sponge's microbial counterparts which may comprise up to 40% of their body mass (Magnino et al., 1999). The lack of data in the literature is mainly due to the technical challenges involved in measuring most parameters in vivo. Other than the seminal study by Reiswig (1974) and the work of Leys and co-workers (Leys et al., 2011; Ludeman, 2015), oxygen dynamics have been mostly examined using metabolic chambers (Hadas et al., 2008; Koopmans et al., 2010; Mills et al., 2014). This method induces stress, since it confines a filter-feeder within a space with reduced water circulation (e.g., Hadas et al., 2008). Other studies have measured spatial dissolved oxygen (DO) concentration in the sponge using microsensors (Hoffmann et al., 2005a, 2007, 2008; Schläppy et al., 2007, 2010a), but only one such study was conducted in situ (Schläppy et al., 2010a).
By increasing its pumping rate, the sponge may aerate its body (Hoffmann et al., 2008), supplying it with oxygen and nutrients (Maldonado et al., 2012). A considerable variation in pumping rates can occur between different pumping units of a single sponge as each unit of the sponge acts independently. A study of the sponge Aplysina aerophoba showed that each osculum, representing a pumping unit, could start or stop pumping regardless of the activity of other units within the same sponge (Pfannkuchen et al., 2009).
Sponges with High Microbial Abundance (HMA) tend to have a lower choanocyte chamber density and a slower specific pumping rate compared to Low Microbial Abundance (LMA) sponges (Poppell et al., 2014). Moreover, feeding behavior also differs between the two types. While HMA sponges feed intensively on dissolved organic matter (DOM), LMA sponges rely mostly on particulate organic matter (POM) (Ribes et al., 2005; Weisz et al., 2007; Yahel et al., 2007; de Goeij et al., 2008; Poppell et al., 2014).
Even though sponges are aerobic organisms, local anaerobic conditions occur in their bodies when pumping diminishes for short periods of time (Schläppy et al., 2007, 2010a; Hoffmann et al., 2008). Moreover, oxygen consumption can be greatly influenced by the high abundance of microbial symbionts (Weisz et al., 2008), which in HMA sponges are much more abundant than the actual sponge cells (Hentschel et al., 2006; Taylor et al., 2007; Gloeckner et al., 2014).
The HMA coral reef sponge Theonella swinhoei is common in the Indo-Pacific Ocean and the Red Sea (Ilan et al., 2004). The sponge harbors a dense consortium of photosynthetic and heterotrophic associated bacteria, reaching up to 1010 bacteria mL−1 sponge (Magnino et al., 1999). To date, 12 bacterial phyla (Gemmatimonadetes, Chloriflexi, Cyanobacteria, Nitrospira, Proteobacteria, Spirochaetes, Verrucomicrobia, Actinobacteria, Acidobacteria, Bacteriodetes, Firmicutes, and Lentisphaerae) and four candidate phyla (Poribacteria, Tectomicrobia, OP10, and OS-K) have been detected in the genus Theonella (Schmitt et al., 2012; Lavy et al., 2014; Wilson et al., 2014). In a culturing experiment, 20 out of 59 isolated OTUs from T. swinhoei required suboxic or anaerobic conditions, suggesting that these conditions may occur naturally in the sponge. Only one study to date has addressed oxygen removal in T. swinhoei (Yahel et al., 2003). In that study, several discrete measurements recorded an O2 removal rate of 23.4 ± 13.0 nmol O2 min−1 (ml sponge)−1.
It is our hypothesis that suboxic and even anaerobic conditions prevail temporarily within T. swinhoei, supporting its microaerobic symbionts. Anaerobiosis states may be achieved in the holobiont by: (1) halting pumping activity, thus increasing the residence time of water inside the sponge body and allowing removal of DO (Schläppy et al., 2007, 2010a; Hoffmann et al., 2008); (2) increasing internal metabolism; and/or (3) increasing the oxygen demand of the holobiont by elevating the pumping rate since the energetic cost of pumping rises as the square of the pumping rate (Leys et al., 2011). In order to broaden our understanding of oxygen consumption by the sponge and detect possible anaerobic periods within its body, we deployed underwater microsensors to continuously record DO concentrations inside the sponge (henceforth, sponge tissue) and in its outflowing water for periods of up to 48 h.
Materials and Methods
Running Seawater Aquarium
A preliminary experiment was conducted to investigate the feasibility of using microsensors to measure DO in T. swinhoei. One T. swinhoei individual (T0, N = 1) was carefully collected by SCUBA diving at 15 m depth from the coral reef of Eilat, Red Sea (29°29′57.63″N / 34°54′54.61″E) and transferred within 20 min (in a light-impenetrable plastic bag filled with seawater) to a running seawater aquarium. Care was taken to collect the sponge attached to a portion of the substrate in order to avoid any damage. The sponge was placed in a 42 L aquarium and left to acclimate for 24 h. The aquarium was fitted with running seawater, pumped from 40 m depth, ensuring replacement of the aquarium volume at least 10 times per hour, 3-fold the estimated sponge pumping rate (Yahel et al., 2003). To verify the quality of the water supply (Alexander et al., 2015), chlorophyll concentration in the aquarium and the ambient seawater were measured using the non-acidification method (Yahel et al., 2005b). The concentration of chlorophyll in the aquarium was 0.41 μg L−1, ~80% of the intake value and well within the range of reported value for the coral reef of Eilat (Shaked and Genin, 2014). The aquarium was placed in the shade but under a natural lighting regime.
Sponge pumping activity was evaluated qualitatively as high, low, or not pumping. This was achieved by slowly injecting fluorescein-dyed seawater, filtered by a 0.2 μm disposable syringe filter, near the ostia (the incurrent openings), and observing the dye outflow through the oscula. Pumping was categorized as either “low” or “high,” when the exhaled current jet reached <2 or > 4 cm from the osculum, respectively, before dispersing with the strong ambient current. This procedure was repeated hourly.
DO was continuously measured (5 s intervals) for two consecutive periods. First, for 14 h (from 20:00 to10:00 the following day) in ambient water and in the exhalent current from two oscula, using a four-channel FireSting O2 (PyroScience, Germany) equipped with retractable needle optodes (OXR430) (Figure 1A). Possible temperature fluctuations were accounted for by a temperature compensation probe. Ambient DO and temperature were monitored with an identical probe from the same device. A ProODO optical DO instrument (YSI, USA) was positioned adjacent to the ambient FireSting sensors for cross-comparison of sensor performance. Ambient temperature and DO in the aquarium were constant at 22°C and 98% saturation (211 μmol L−1, measured by three different sensors), and salinity was 40.5 PSU. DO was measured concurrently in the ambient water and in the exhalent current of two oscula. DO removal was calculated as the difference between the ambient DO and that measured in the osculum.
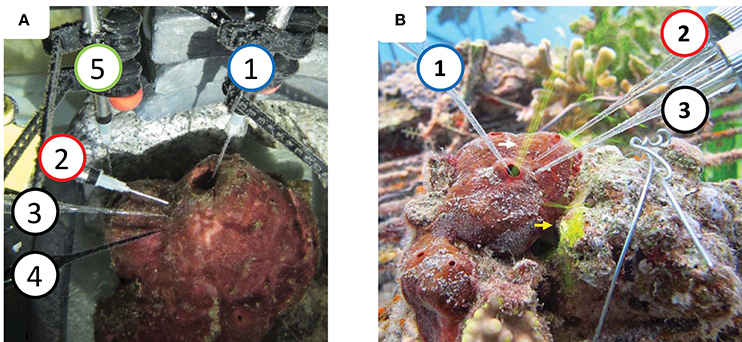
Figure 1. System setup (A) in a running seawater aquaria. (B) In situ. (1) Dissolved oxygen (DO) sensors in the osculum measuring the DO levels in the water exhaled by the sponge [micro-optode in (A), micro-electrode in (B)]. (2) DO sensor inserted ~0.8–10 mm into the sponge body to measure internal DO levels [micro-optode in (A), micro-electrode in (B)]. (3) RedOx and (4) sulfide micro-electrodes inserted ~8–10 mm into the body. DO sensor (5) (micro-optode) inserted to the small osculum. Fluorescein dye (0.2 μm filtered) was released near the dermal surface of the sponge to be pumped in (yellow arrow). Flow through the osculum (white arrow) was used to assess sponge pumping activity. RedOx and sulfur data are not presented in the current manuscript.
During the second measurement period, spanning 18 h (from 18:00 to 12:00 the following day), a fourth OXRD430 retractable needle DO optode, connected to the FireSting, was inserted 15 mm into the sponge tissue in the upper third of its larger tube. The exact type of morphological structure (e.g., choanoctye chamber, mesohyl, water channel etc.) in which the tip of the sensor was located could not be determined. However, the tip was at least 10 mm away from a main water channel and a similar distance from the cyabobacterial layer lining the sponge body. The FireSting optodes measuring DO at the oscula were placed so that one optode measured DO inside the large osculum while the second was positioned near the sponge ostia, close to the small osculum. At 9:44 am, when a drastic change in DO concentration was observed in the large osculum, the second FireSting optode was inserted into the small osculum. Throughout the drastic change in DO, the sponge pumping activity was continuously assessed manually, using flourescein dye. During this set of measurements, a third DO sensor (100 μm tip glass microelectrode, Underwater Meter, Unisense, Denmark) was attached next to the FireSting and ProDO optodes that measured DO concentration in ambient water for validation.
Prior to each session all sensors were cross-calibrated according to the manufacturer's protocols. H2S and Redox potential were measured by micro-electrodes connected to a Unisense UnderWater Meter (Unisense Denmark), inserted near the DO optode (8 mm). However, due to inconsistencies of the signal, these measurements are not reported here.
In situ Experiment
For the in situ experiment, three T. swinhoei individuals (T1–T3) were collected from 17 m depth at the coral reef (29°30′11.35″N / 34°55′7.66″E) while attached to a portion of the substrate, and transferred underwater to a 7.5 m deep coral nursery, located within 300 m of the place of collection (29°30′6.94″N / 34°55′5.25″E). The relocation was performed to allow on-line monitoring by connecting the devices to land based computer and electricity. Hydrographic profiles of water temperature and salinity indicate that during the experiments both sites were located well within the upper mixing layer and experienced the same ambient conditions (Figure S1). Ambient temperature and salinity were stable at 24°C, and 40.5 PSU, respectively (The Israel National Monitoring Program at the Gulf of Aqaba). Chlorophyll concentrations ranged between 0.4 and 0.6 μg L−1 and the calculated DO saturation in the ambient water was 208 μmol L−1. To minimize a possible effect of elevated light intensity due to the relocation, the sponges were placed in the north-west corner of the nursery, where it was shaded by a 1.5 m high mesh sparsely occupied by corals. The nursery in which the experiments were conducted is located within the distribution range of T. swinhoei and several individuals of this species naturally occur around it. Fluorescein dye was used to assess the activity of the individuals (i.e., water pumping), which appeared perfectly healthy throughout the experiment and for many weeks afterwards. The sponges were allowed to acclimate for 24 h before oxygen measurements commenced. DO concentration was measured by a Unisense underwater meter equipped with 100 μm tip glass microelectrodes. Sensors were calibrated according to the manufacturer's protocol in well-aerated water and nitrogen-purged water (100 and 0% DO saturation, respectively). DO readings were validated with a ProODO optical DO instrument (YSI, USA). Sensors were calibrated at three time points: before the experiment commenced, after measurements of the first sponge individual (T1), and after the experiment ended. A second set of measurements carried out at the study site with a robust FireSting O2 optode (PyroScience, Germany), established that ambient water was always close to 100% saturation, with <5% variation throughout the day (Yahel, unpublished data).
In each sponge one DO sensor was placed inside one of its oscula and another pierced 8–10 mm into the sponge body (Figure 1B). Similar to the preliminary experiment, the tips of the sensors were inserted into the sponge body at least 10 mm away from a main water channel or cyanobacterial rich layer of the sponge body. The sensors were positioned using a custom-designed, 3D-printed, manipulator (Printed Tech, Israel). The device allowed accurate and stable insertion of each sensor and measurement of the distance into which each sensor was inserted. It should be noted that the complex internal structure of the sponge does not allow exact positioning of the sensor tip inside a specific morphological structure such as a choanocyte chamber or water canal, since their locations are unknown. However, considering the structure of the sponge aquiferous system with its unidirectional water flow, it is likely that water was drawn over the sensor tip, which was integrating the physiological conditions of an unknown area around and upstream to the tip.
Measurements were conducted continuously every second for 27, 24, and 48 h for the first (T1), second (T2), and third (T3) sponges, respectively. Following the 24 h measurement in T2, the sensor from the osculum was transferred to a second osculum. A DO sensor placed in vivo was relocated to another area within the same sponge and measurements were performed over a 48 h period (T2b). The duration of each experiment as well as the total number of sponge studied were limited by logistics related to the challenging nature of an underwater microsensor experiment. These included dive limits, battery time, the need to recalibrate the sensors, and microelectrode survival rate.
Sponge pumping was evaluated qualitatively and quantitatively. Qualitative assessment was carried out in the same manner as described in the aquarium experiment. This method was used only to assess whether the sponge was active. Quantitative measurement was done by determining the fluorescein dye front speed (DFS) in an open-ended tube located just above the sponge osculum, as describe in Yahel et al. (2005a). Sponge pumping was expressed as exhalent current speed (cm s−1) or pumping rate calculated as the product of the DFS and tube cross-section area (Yahel et al., 2005a). Specific pumping rate [mL min−1 (mL sponge)−1] was calculated using the flow rate measured at the osculum and the volume of the sponge. The volume of sponges T1, T2, and T3 was 21, 50, and 38 ml, respectively, determined by using the in situ, photogrammetry-based, 3D modeling method developed by Lavy et al. (2015). For sponges with more than one osculum, the actual fraction of the sponge that each osculum is “draining” is unknown. To calculate this volume, the surface area of the opening of each osculum was measured, assuming that the volume drained by each osculum is proportional to the surface area of its opening. To estimate the specific pumping rate [mL min−1 (mL sponge)−1] of the osculum at which measurements were taken, the pumping rate was normalized to the relative surface area of the osculum.
Statistical analysis was carried out in R v3.2.3 (R Core Team, 2012) using Rstudio v0.99.489 (R Studio Team, 2015). The DO signal was smoothed using a 30 s central moving average in order to remove momentary reading drifts. Lag-correlation, autocorrelation and Pearson cross-correlation were assessed using PAST v3.12 (Hammer et al., 2001).
Results
Fluorescein dye visualization indicated that all sponges were actively, but not necessarily continuously, pumping water throughout the experiment. For example, sponge T2 (at osculum location T2b) did not pump for a period of 12 h. Pumping activity was detected again 12 h after the experiment had ended. The sponges' pumping rate (Figure 2), measured for a specific osculum for T1, T2, T2b, and T3, ranged from 0 to 11 [cm3 s−1) (ml sponge)−1], well within the reported range (Yahel et al., 2003) (Figure 2, see also black squares in Figures S6–S9 that represent the raw exhaled water current speeds). Pumping rates varied greatly among individuals, with T1 being the most active sponge and T2b the least active. There was little or no apparent correlation between the pumping rates of adjacent oscula, representing a “specific pumping unit” in the same sponge. For example, in sponge T2, one osculum was not pumping while a 3.1 ± 0.1 [cm3 s−1 (ml sponge)−1] pumping rate was measured concomitantly at an adjacent osculum (star and triangle in Figure 2).
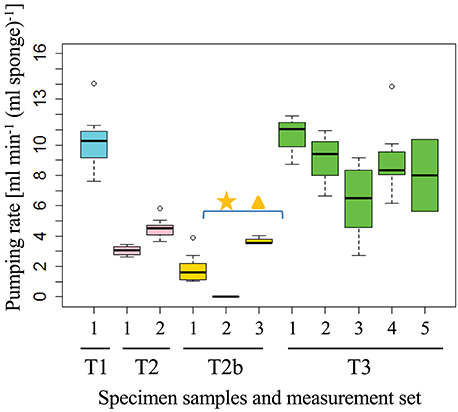
Figure 2. Pumping rates of an individual sponge (osculum, T1–T3) measured by the DFS method at different times (black squares in Figures S6–S9). The measured flow rate is normalized to the volume of the sponge “pumping unit” drained by the measured osculum. Each set of measurements consisted of 5 ± 3 DFS experiments over 21 ± 20 min. The first osculum in which DO was measured in T2b was not pumping (star), the water flow speed was therefore measured in a second osculum (triangle). Individual Dye Front speeds for each sponge are presented in Figures S3–S5, S7.
The DO concentration in the exhaled water provides an integrated measure of oxygen dynamics and metabolic rate of the specific pumping unit (i.e., of the sponge volume drained by the specific osculum). In order to elucidate possible patterns, frequency distribution of DO concentration over time was investigated. Consolidating DO saturation at the oscula from all sponges resulted in a clear bimodal distribution with a dominant peak of 85–100% DO saturation (Figure 3A), suggesting removal of 31–0 μmol DO Lpumped−1 from the inflowing water. However, much lower DO concentrations were also observed in the oscula for considerable periods of time. For about one-third of the time, the sponges removed at least 93 μmol DO Lpumped−1 (55% DO saturation). T3 had a bimodal distribution with two main states of DO saturation ranging from 30 to 55% and 75 to 110% (Figure 3F). Similar to T3, sponge T1 exhibited a distribution with peaks at 40–60% saturation, and 90–100% saturation, as well as a smaller third state of 110–115%, suggesting oxygen production for 5% of the time (80 min) (Figure 3C). Sponge T2b demonstrated three oxygenation states in its osculum (Figure 3E), the most prominent being the lowest (25–50% saturation). In its two other frequent states DO saturation ranged between 55 and 80% and 85–100%. T0 and T2 had a unimodal distribution of DO saturation over time, both with peaks at 85–100% (Figures 3B,D). On one occasion, DO saturation in the osculum of T0 reached nearly 0% for 14 min (Figures S3, S4). DO concentration in the tissue did not correlate with DO in the osculum in T1, T2, T2b, and T3 and only partial lag-correlation was found in T0 (Pearson coefficient of 0.55, 11 min lag).
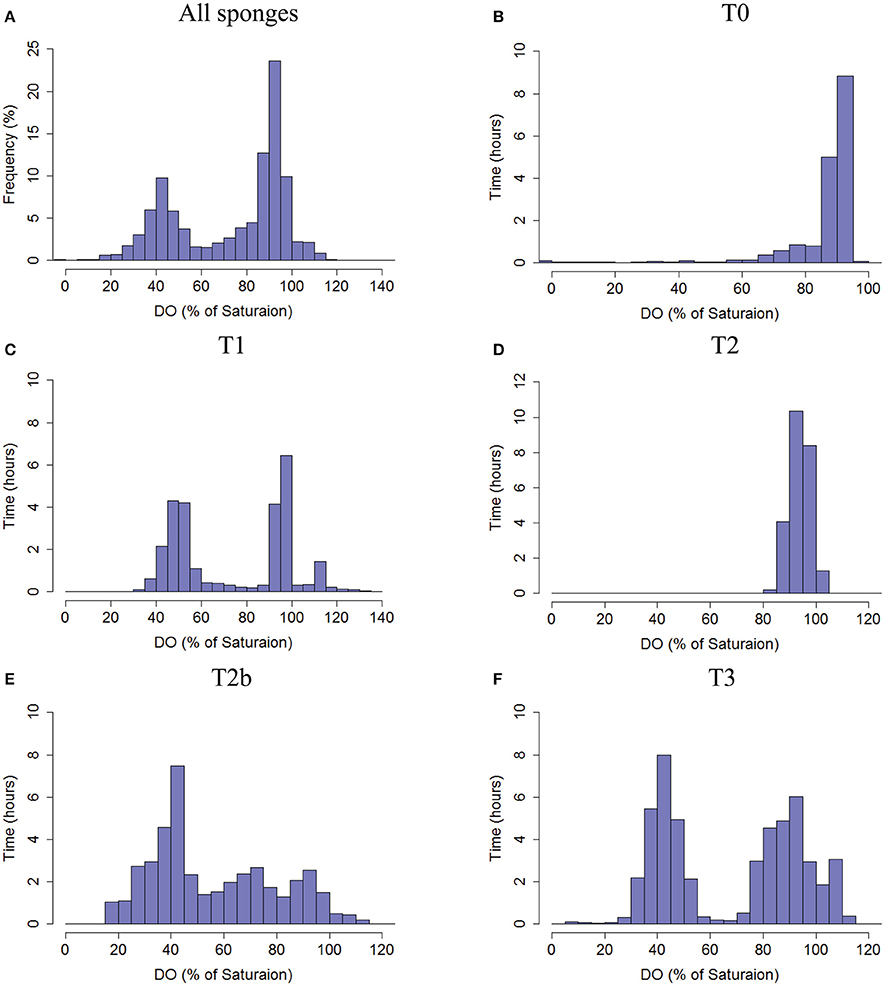
Figure 3. Frequency distribution of the DO saturation (%, 30 s averages) in the water exhaled by the sponges as measured in the sponge oscula. (A) The total frequency of DO % of saturation from all sponges pooled together. (B) T0, (C) T1, (D) T2, (E) T2b, and (F) T3. Co-occurrence of DO saturation in osculum and tissue of each sponge is given in Figure S2.
The body of T. swinhoei was constantly sub-saturated with oxygen throughout the experiments (Figure 4A). Moreover, hypoxic conditions (DO<30% saturation) were detected during 14% of all time points measured and in three out of four individuals at the time of measurement. DO in the tissue dropped to <10% saturation mostly for short periods (4 ± 8% of the measurements). The DO concentration in the tissue of sponge T0 was below 75% at all times and <50% saturated most of the time (Figure 4B). Sponges T2 and T3 displayed a clear unimodal frequency distribution of DO saturation, peaking at 75% saturation (49 and 38% of time, respectively, Figures 4D,F). An intriguing bimodal distribution was apparent in T1 and T2b. The tissue of T1 was mostly either 15–35% or 45–60% saturated, while other states of saturation existed for short periods (Figure 4C). In sponge T2b, the difference between the two states was even greater (Figure 4E). The tissue of T2b was frequently either 0–5% or 60–85% saturated.
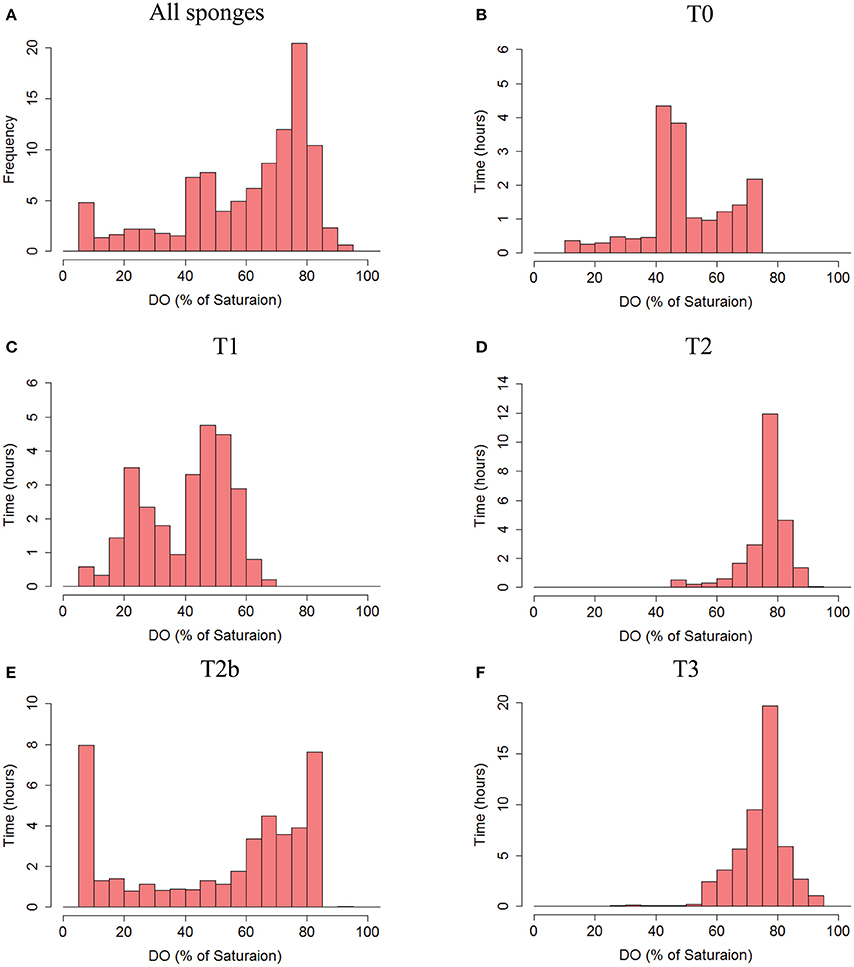
Figure 4. Frequency distribution of the DO saturation (%, 30 s averages) in the tissue of the sponges. (A) The total frequency of DO % of saturation from all sponges pooled together. (B) T0, (C) T1, (D) T2, (E) T2b, and (F) T3. Co-occurrence of DO saturation in osculum and tissue of each sponge is given in Figure S2.
Extreme fluctuations, as great as 80% change, in DO saturation in the osculum (Figure 5A and Figure S3), and up to 70% in the tissue (Figures 5A,B, and Figures S5, S9), occurred over a scale of several minutes. Smaller scale changes (up to 10%) were more frequent (Figure 5 and Figures S3–S5). Drops in DO saturation in both osculum and tissue occurred in all sponges and were neither specific to the time of day nor could any auto-correlation be established, other than in T2b (data not shown).
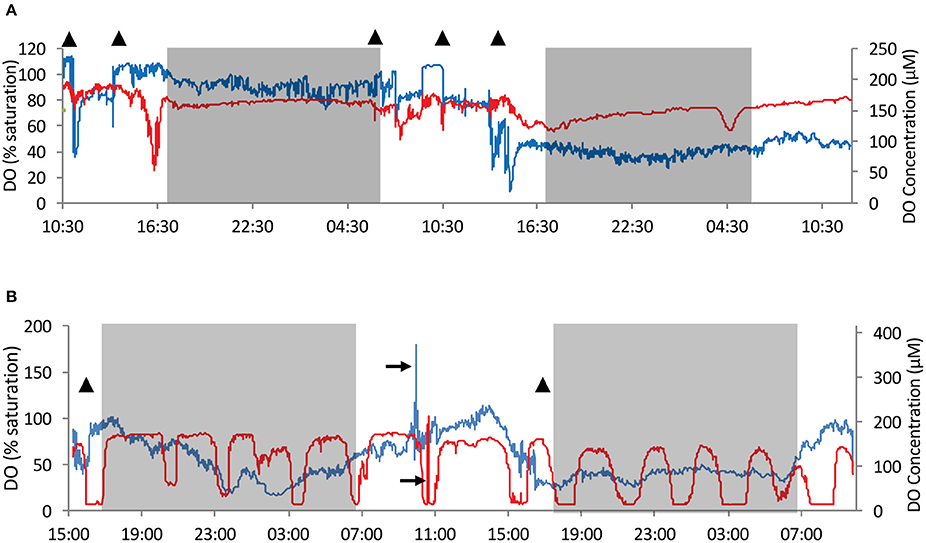
Figure 5. An example of time series of dissolved oxygen (DO) concentration and saturation in the water exhaled from the osculum and in the sponge tissue (red–tissue, blue–osculum). Sponges T3 and T2b were chosen as examples as they present very different behavior patterns. (A) DO measurements in T3 over 48 h. (B) DO measurements in T2b over 42 h. T2b represents a second set of measurements taken in the individual T2 but at a different osculum and locality within the sponge. Time series of T0, T1, and T2 are given in Figures S3–S5. Gray shade– night time. Triangles–times at which qualitative and/or quantitative flow speed was measured (Figure 2 and Figures S6–S9). 100% saturation = 208 μmol L−1. Black arrows mark temporary repositioning of sensors to ambient water for performance check.
The most unique DO dynamics were recorded in T2b, which was measured at a second osculum and location in the tissue of T2. The measurements of T2b were performed immediately after T2 and before T3. DO concentration in the tissue of T2b followed a rhythmic pattern, differing from all other individuals (Figure 5B). The oscillations ranged between 6 and 84% saturation in 14 cycles over 41 h. The oscillation crests were maintained for 70 to 130 min. The troughs lasted 30–90 min each, with 10 of the 14 cycles almost reaching anoxia (5–7% saturation). DO in the tissue was significantly auto-correlated, giving a cycle frequency of 172 min (correlation = 0.378, confidence = 0.031).
Discussion
The occurrence of a temporal anaerobic environment in sponges has been previously studied. With the exception of the seminal study of Reiswig (1974), and the work of Leys and co-workers (Leys et al., 2011; Ludeman, 2015), most of the knowledge regarding oxygen concentration in sponges is based on short-term DO measurements carried out in metabolic chambers (Koopmans et al., 2010; Mills et al., 2014), or in aquaria (Hoffmann et al., 2005a, 2007, 2008; Schläppy et al., 2007, 2010a; Hadas et al., 2008). Further support for the possible occurance of anaerobic periods in sponges stems from the presence of anaerobic sponge microbial symbionts (e.g., Hoffmann et al., 2005b; Lavy et al., 2014). Such bacteria may have important roles in the holobiont, based on their anaerobic metabolism [e.g., anaerobic denitrification, described by Schläppy et al. (2010b)]. To further investigate to what extent internal conditions support anaerobic symbionts, DO dynamics were studied in situ in the HMA sponge T. swinhoei. DO concentrations were measured in the T. swinhoei sponge exhaled current jet and inside the body of sponges. Long-term, continuous, in situ measurements detected a remarkably high oxygen utilization rate by actively pumping T. swinhoei, including long-term periods of suboxic conditions in the exhaled water as well as events of anoxia.
Most of the time the sponges removed at least 10 μmol DO (5%) from each liter of seawater they pumped (Figure 3). DO uptake can be translated into water transport rate in terms of L pumped per mL DO consumed. The average DO uptake measured in one osculum of sponge T1 (pumping unit volume 10.5 mL) was 53 ± 51 μmol DO per liter pumped, when translated into transport rate of 1.2 Lpumped (mL O2)−1. These values fall within the known low range of other HMA sponges' as reported by Reiswig (1971, 1974) and Weisz et al. (2008). Considering an average pumping rate of 105 mL min−1 (N = 8 DFS measurements), this uptake results in a calculated daily pumping of 150 L (10 mL seawater (mL sponge)−1 min−1) and an uptake of 8 mmol DO day−1 or 0.76 mmol DO (mL sponge)−1day−1.
The uptake of such large amounts of DO requires the oxidation of an equivalent amount of reduced material that should ultimately be brought in by the inflowing water. Assuming complete catabolism of typical planktonic organic matter (OM) with a respiratory quotient (RQ) in the range of 0.7–0.8 (Hatcher, 1989; Anderson, 1995), these figures correspond to the complete oxidation of 37 μmol C (L pumped)−1, or 1.3 mg OM (mL sponge)−1day−1. Yahel et al. (2003) reported that the Total Organic Carbon (TOC) concentration in local seawater is 70–100 μmol L−1. However, only 10–40 μmol L−1 are considered labile, readily available for the holobiont's consumption (Hansell and Carlson, 2015).
T. swinhoei exhibited an extremely high DO removal efficiency for prolonged periods. In many cases the sponges utilized >100 μmol DO Lpumped−1 (measured at the exhaled water) and maintained this activity for up to 12 h (Figure 5 and Figure S5). For example, in sponge T1 the average DO removal rate during the first 12 h (16:00–04:00) of measurement was 93 ± 26 μmol DO Lpumped−1. Similar prolonged periods with extremely high DO removal efficiency were also observed in T2b and T3 (Figures 3E,F, respectively). Clearly, there is not sufficient organic matter in the reef water to sustain such a high oxidation rate. T. swinhoei hosts symbiotic cyanobacteria in a thin outer layer, which pass assimilated carbon to their host (Steindler, unpublished data). However, the same process also generates at least one mole of oxygen for each mole of carbon fixed (usually more) and, therefore, the respiration of photosynthetically-assimilated carbon cannot account for the missing amount of reduced material. Internal pools of reduced forms of carbon, nitrogen, and sulfur (e.g., ammonia and sulfide resulting from the holobiont's metabolism) or other unknown reduced chemicals, could serve as intermittent electron donors during the events of high oxygen demand that we observed. Yahel and Ribes (unpublished data) measured a production of 0.9 ± 0.4 μmol nitrate Lpumped−1 by T. swinhoei while ammonia rarely exceeded 200 nM in ambient water. Their data suggest complete oxidation of organic nitrogen but this process can account for the additional reduction of only 5–6 μmol DO per liter pumped. No data are available in the literature with respect to sulfate production in T. swinhoei, but such a process requires a sulfide source unknown on the reef. Another possibility is that the sponge uses its own symbiotic bacteria as a transient and renewable food source (Sara, 1971; Ilan and Abelson, 1995). The predation of symbionts in a sustained manner would necessitate alternating between feeding and no feeding in order to allow the bacterial community to proliferate. The observed changes between high and low DO consumption (e.g., Figure 5 and Figure S5B) can support the hypothesized alternations in feeding. To resolve the sponge metabolic budget and better understand this unique boom and bust mechanism, a long-term follow-up metabolic study is required. Such a study should include a coupled continuous tracking of pumping and respiration rates, as well as of TOC and total organic nitrogen (TON) concentrations in the sponge's inflowing and outflowing water.
The bimodal frequency distribution of DO in the sponge body and lack of correlation with DO concentration in the osculum suggest that the holobiont contains constantly changing microenvironments. Activation and inactivation of choanocytes or the choanosome is considered an efficient mechanism for regulating water pumping through the sponge (Leys et al., 2011). Such a mechanism could potentially account for the formation of these microenvironments. The temporal environments can be visualized as similar to the ever-changing wax blobs in a lava lamp, in which the blobs represent the aerated areas moving within the sponge body due to the “turn-on”/“turn-off” mechanism. Temporal changes in DO concentration within the microenvironments suggest that aerophilic and microaerophilic bacterial communities of T. swinhoei may be co-localized. A lack of spatial separation between bacterial communities in sponges was previously reported for A. aerophoba (Pfannkuchen et al., 2010) and Tethya californiana (Sipkema and Blanch, 2010), but its context in regard to temporal niches was not examined. Co-locality allows both aerobic and anaerobic processes to occur, enabling efficient recycling of metabolic waste of the host, e.g., nitrogen fixation (Mohamed et al., 2008), nitrate denitrification (Mohamed et al., 2010), anaerobic ammonia oxidation (Hoffmann et al., 2009), aerobic nitrification (Schläppy et al., 2010b), sulfate reduction (Hoffmann et al., 2005b), and sulfide oxidation (Tian et al., 2014). All these processes may be acting in a synergistic manner, completing the nutrient cycles and achieving an ultra-efficient utilization of the meager amount of organic matter available in the oligotrophic coral-reef ecosystem in which T. swinhoei resides.
An intriguing DO dynamic was exhibited by specimen T2b, with strong and regular DO oscillations observed in the tissue and elevated DO in the osculum during the day. Surprisingly, we could not find any correlation or lag-correlation between the tissue and outflowing water DO dynamics, nor did we observe any similar regularity in other sponge specimens. Because the distribution of water channels and choanocyte chambers in the sponge are not homogenous, and because each pumping unit acts as a separate system, the measurement of T2b cannot be considered as a repeated measurement of T2. Metabolic oscillations, represented by DO oscillations as well as other parameters, were previously described in the yeast Saccharomyces cerevisiae (Tu et al., 2005); for which the average DO cycle period was 4–5 h and periodicity analysis of gene expression revealed a ~5 h cycle. If a similar process occurs in sponge cells, and a partial volume of mesohyl within the holobiont is temporally isolated or at least less exposed to rapid DO exchange with the environment, this might explain the observed oscillations of DO in the sponge tissue.
Oxygen consumption inside the sponges and at their oscula varied between individuals and even within the same sponge (i.e., sponge T2 and T2b). This variability may be ascribed to the somewhat autonomous nature of each pumping unit within the sponge (Pfannkuchen et al., 2009), as observed in the current experiment (Figure 2). The differential activity of pumping units may generate spatio-temporal differences in DO concentration within the sponge. Another probable cause for variability is the non-homogeneous nature of the sponge body. Structural analysis of another leuconoid sponge revealed a complex and non-uniform inner structure (Hammel et al., 2011). Furthermore, differences in morphology and body volume add to the variability between and within individuals.
Future studies of DO dynamics and other physiological parameters in sponges should be coupled with composition and activity analysis of their microbial community. A temporal analysis at times of anaerobiosis and other oxygenation states should produce interesting insights as to the roles of the symbionts within the changing environment. Metagenomic and metatranscripotmic approaches, both widely used in microbial ecology studies, would probably prove useful when undertaking such an effort.
Many sponge-associated bacteria have been successfully isolated from sponges (Fuerst, 2014). However, most of the sponge symbionts, including many potent producers of bioactive natural compounds, are still unculturable. Our findings of a constant internal suboxic environment and temporal anoxic conditions support previous suggestions regarding the use of suboxic conditions for culturing sponge symbionts (Lavy et al., 2014; Keren et al., 2015, 2016). Fluctuating aeration conditions within the sponge may very well support co-locality of aerobes, microaerophiles, and even aerotolerant anaerobes, each with its distinct metabolic functions in the holobiont. Our results also suggest that using fluctuating oxygenation regimes and co-cultures as a method to simulate the natural environment may assist in cultivating T. swinhoei associated bacteria. To further improve our ability to isolate novel bacteria from the holobiont, other attributes of the mesohyl, such as sulfur, nitrogen, amino acid, and carbohydrate composition, and pH, would also have to be measured in vivo. Fine-tuning of DO concentration in future culturing experiments could be the next step in increasing the diversity of cultured symbionts.
Overall, we have shown that T. swinhoei can generate sub-oxic conditions within its body by means of an as yet unknown mechanism(s). These areas of sub-oxic conditions are confined by time and space and their occurrence may provide the necessary environment for growth of anaerobic bacteria, such as those previously isolated from this sponge. Based on our findings, we suggest that current models of interactions between the sponge and its symbionts are oversimplified. Differences in the DO removal patterns between sponges, and even changes within a single individual sponge, suggest that interactions such as predation and chemical communication between the sponge and its symbionts may shape the behavior of the holobiont. The temporal nature of microenvironments within the sponge and the rhythmic DO uptake pattern could imply the existence of a complex control mechanism that may involve both the host and its symbionts.
Ethics Statement
The study was conducted on marine sponges (invertebrates) and therefore was exempted from this requirement. However, permits from the National parks and nature reserves authority of Israel were obtained.
Author Contributions
AL, RK, MI, and GY designed the study and concluded its results. AL, RK, and GY conducted the field experiments. AL and GY conducted the statistical analysis. AL and RK wrote the manuscript, with significant input and editing from all authors. The study was supervised by MI.
Funding
AL was funded by an Eshkol scholarship from the Israel Ministry of Science, Technology and Space, and the Interuniversity Institute for Marine Sciences in Eilat scholarship for students residing outside Eilat. The Israel Science Foundation (ISF) grants (1280/13 and 957/14) supported GY and MI, respectively.
Conflict of Interest Statement
The authors declare that the research was conducted in the absence of any commercial or financial relationships that could be construed as a potential conflict of interest.
Acknowledgments
The authors thank The Interuniversity Institute for Marine Sciences in Eilat, Israel for supporting and allowing the use of their facilities. The authors would also like to thank Dr. Roi Holtzman for assisting in setting up the in situ experiment and allowing the use of his instruments; and Mr. Marcos Dorfman, who designed and 3D-printed the micromanipulator for this study.
Supplementary Material
The Supplementary Material for this article can be found online at: http://journal.frontiersin.org/article/10.3389/fmars.2016.00263/full#supplementary-material
References
Alexander, B. E., Mueller, B., Vermeij, M. J., van der Geest, H. H., and de Goeij, J. M. (2015). Biofouling of inlet pipes affects water quality in running seawater aquaria and compromises sponge cell proliferation. Peer J. 3:e1430. doi: 10.7717/peerj.1430
Anderson, L. A. (1995). On the hydrogen and oxygen content of marine phytoplankton. Deep Sea Res. Part I 42, 1675–1680. doi: 10.1016/0967-0637(95)00072-E
de Goeij, J. M., Moodley, L., Houtekamer, M., Carballeira, N. M., and van Duyl, F. C. (2008). Tracing C-13-enriched dissolved and particulate organic carbon in the bacteria-containing coral reef sponge Halisarca caerulea: evidence for DOM feeding. Limnol. Oceanogr. 53, 1376–1386. doi: 10.4319/lo.2008.53.4.1376
Fuerst, J. A. (2014). Diversity and biotechnological potential of microorganisms associated with marine sponges. Appl. Microbiol. Biotechnol. 98, 7331–7347. doi: 10.1007/s00253-014-5861-x
Gloeckner, V., Wehrl, M., Moitinho-Silva, L., Gernert, C., Schupp, P., Pawlik, J. R., et al. (2014). The HMA-LMA Dichotomy revisited: an electron microscopical survey of 56 sponge species. Biol. Bull. 227, 78–88. doi: 10.1086/BBLv227n1p78
Guo, X. C., Zheng, L., Zhou, W. H., Cui, Z. S., Han, P., Tian, L., et al. (2011). A case study on chemical defense based on quorum sensing: antibacterial activity of sponge-associated bacterium Pseudoalteromonas sp NJ6-3-1 induced by quorum sensing mechanisms. Ann. Microbiol. 61, 247–255. doi: 10.1007/s13213-010-0129-x
Hadas, E., Ilan, M., and Shpigel, M. (2008). Oxygen consumption by a coral reef sponge. J. Exp. Biol. 211, 2185–2190. doi: 10.1242/jeb.015420
Hammel, J. U., Filatov, M. V., Herzen, J., Beckmann, F., Kaandorp, J. A., and Nickel, M. (2011). The non-hierarchical, non-uniformly branching topology of a leuconoid sponge aquiferous system revealed by 3D reconstruction and morphometrics using corrosion casting and X-ray microtomography. Acta Zool. 93, 160–170. doi: 10.1111/j.1463-6395.2010.00492.x
Hammer, Ø., Harper, D. A. T., and Ryan, P. D. (2001). PAST: Paleontological statistics software package for education and data analysis. Palaeontol. Electron. 4, 9.
Hansell, D. A., and Carlson, C. A. (eds.). (2015). “Chemical Characterization and Cycling of Dissolved Organic Matter,” in Biogeochemistry of Marine Dissolved Organic Matter, 2nd Edn. (London: Elsevier), 22–58.
Hatcher, A. (1989). RQ of benthic marine invertebrates. Mar. Biol. 102, 445–452. doi: 10.1007/BF00438345
Hentschel, U., Usher, K. M., and Taylor, M. W. (2006). Marine sponges as microbial fermenters. FEMS Microbiol. Ecol. 55, 167–177. doi: 10.1111/j.1574-6941.2005.00046.x
Hoffmann, F., Larsen, O., Thiel, V., Rapp, H. T., Pape, T., Michaelis, W., et al. (2005b). An anaerobic world in sponges. Geomicrobiol. J. 22, 1–10. doi: 10.1080/01490450590922505
Hoffmann, F., Larsen, O., Tore Rapp, H., and Osinga, R. (2005a). Oxygen dynamics in choanosomal sponge explants. Mar. Biol. Res. 1, 160–163. doi: 10.1080/17451000510019006
Hoffmann, F., Radax, R., Woebken, D., Holtappels, M., Lavik, G., Rapp, H., et al. (2009). Complex nitrogen cycling in the sponge Geodia barretti. Environ. Microbiol. 11, 2228–2243. doi: 10.1111/j.1462-2920.2009.01944.x
Hoffmann, F., Røy, H., Bayer, K., Hentschel, U., Pfannkuchen, M., Brümmer, F., et al. (2008). Oxygen dynamics and transport in the Mediterranean sponge Aplysina aerophoba. Mar. Biol. 153, 1257–1264. doi: 10.1007/s00227-008-0905-3
Hoffmann, F., Sauter, E., Sachs, O., Roy, H., and Klages, M. (2007). Oxygen Distribution in Tentorium Semisuberites and in its Habitat in the Arctic deep Sea. Porifera Research Biodiversity, Innovation and Sustainability, Rio De Janeiro, MUSEU NACIONAL – Universidade Federal do Rio de Janeiro.
Ilan, M., and Abelson, A. (1995). The life of a sponge in a sandy lagoon. Biol. Bull. 189, 363–369. doi: 10.2307/1542154
Ilan, M., Gugel, J., and Van Soest, R. W. M. (2004). Taxonomy, reproduction and ecology of new and known Red Sea sponges. Sarsia 89, 388–410. doi: 10.1080/00364820410002659
Keren, R., Lavy, A., and Ilan, M. (2016). Increasing the richness of culturable arsenic-tolerant bacteria from Theonella swinhoei by addition of sponge skeleton to the growth medium. Microb. Ecol. 71, 873–886. doi: 10.1007/s00248-015-0726-0
Keren, R., Lavy, A., Mayzel, B., and Ilan, M. (2015). Culturable associated-bacteria of the sponge Theonella swinhoei show tolerance to high arsenic concentrations. Front. Microbiol. 6:154. doi: 10.3389/fmicb.2015.00154
Koopmans, M., Martens, D., and Wijffels, R. (2010). Growth efficiency and carbon balance for the sponge Haliclona oculata. Mar. Biotechnol. 12, 340–349. doi: 10.1007/s10126-009-9228-8
Lavy, A., Eyal, G., Neal, B., Keren, R., Loya, Y., and Ilan, M. (2015). A quick, easy and non-intrusive method for underwater volume and surface area evaluation of benthic organisms by 3D computer modelling. Methods Ecol. Evol. 6, 521–531. doi: 10.1111/2041-210X.12331
Lavy, A., Keren, R., Haber, M., Schwartz, I., and Ilan, M. (2014). Implementing sponge physiological and genomic information to enhance the diversity of its culturable associated bacteria. FEMS Microbiol. Ecol. 87, 16. doi: 10.1111/1574-6941.12240
Leys, S. P., Yahel, G., Reidenbach, M. A., Tunnicliffe, V., Shavit, U., and Reiswig, H. M. (2011). The Sponge Pump: The role of current induced flow in the design of the sponge body plan. PLoS ONE 6:e27787. doi: 10.1371/journal.pone.0027787
Ludeman, D. A. (2015). Sponges as Sensitive Animals: Sensory Systems and Energetics of Filtration in Demosponges. Master of Science, University of Alberta.
Magnino, G., Sara, A., Lancioni, T., and Gaino, E. (1999). Endobionts of the coral reef sponge Theonella swinhoei (Porifera, Demospongiae). Invertebr. Biol. 118, 213–220. doi: 10.2307/3226993
Maldonado, M., Ribes, M., and Van Duyl, F. C. (2012). “Nutrient fluxes through sponges: Biology, budgets, and ecological implications,” in Advances in Sponge Science: Physiology, Chemical and Microbial Diversity, Biotechnology, eds M. A. Becerro, M. J. Uriz, M. Maldonado and X. Turon (San Diego, CA: Elsevier Academic Press Inc.), 62, 113–182.
Mills, D. B., Ward, L. M., Jones, C., Sweeten, B., Forth, M., Treusch, A. H., et al. (2014). Oxygen requirements of the earliest animals. Proc. Natl. Acad. Sci. U.S.A. 111, 4168–4172. doi: 10.1073/pnas.1400547111
Mohamed, N. M., Colman, A. S., Tal, Y., and Hill, R. T. (2008). Diversity and expression of nitrogen fixation genes in bacterial symbionts of marine sponges. Environ. Microbiol. 10, 2910–2921. doi: 10.1111/j.1462-2920.2008.01704.x
Mohamed, N. M., Saito, K., Tal, Y., and Hill, R. T. (2010). Diversity of aerobic and anaerobic ammonia-oxidizing bacteria in marine sponges. ISME J. 4, 38–48. doi: 10.1038/ismej.2009.84
Pfannkuchen, M., Fritz, G. B., Schlesinger, S., Bayer, K., and Brümmer, F. (2009). In situ pumping activity of the sponge Aplysina aerophoba, Nardo 1886. J. Exp. Mar. Biol. Ecol. 369, 65–71. doi: 10.1016/j.jembe.2008.10.027
Pfannkuchen, M., Schlesinger, S., Fels, A., and Brummer, F. (2010). Microscopical techniques reveal the in situ microbial association inside Aplysina aerophoba, Nardo 1886 (Porifera, Demospongiae, Verongida) almost exclusively consists of cyanobacteria. J. Exp. Mar. Biol. Ecol. 390, 169–178. doi: 10.1016/j.jembe.2010.04.038
Poppell, E., Weisz, J., Spicer, L., Massaro, A., Hill, A., and Hill, M. (2014). Sponge heterotrophic capacity and bacterial community structure in high- and low-microbial abundance sponges. Mar. Ecol. 35, 414–424. doi: 10.1111/maec.12098
R Core Team (2012). R: A Language and Environment for Statistical Computing. Vienna: R Foundation for Statistical Computing.
R Studio Team (2015). R Studio: Integrated Development for R. Boston, MA: RStudio, Inc. Available online at: http://www.rstudio.com/
Reiswig, H. M. (1971). Particle feeding in natural populations of three marine demosponges. Biol. Bull. 141, 568–591. doi: 10.2307/1540270
Reiswig, H. M. (1974). Water transport, respiration and energetics of three tropical marine sponges. J. Exp. Mar. Biol. Ecol. 14, 231–249. doi: 10.1016/0022-0981(74)90005-7
Ribes, M., Coma, R., Atkinson, M. J., and Kinzie, R. A. (2005). Sponges and ascidians control removal of particulate organic nitrogen from coral reef water. Limnol. Oceanogr. 50, 1480–1489. doi: 10.4319/lo.2005.50.5.1480
Sara, M. (1971). Ultrastructural aspects of the symbiosis between two species of the genus Aphanocapsa (Cyanophyceae) and Ircinia variabilis (Demospongiae). Mar. Biol. 11, 214–221. doi: 10.1007/BF00401270
Schläppy, M. L., Hoffmann, F., Roy, H., Wijffels, R. H., Mendola, D., Sidri, M., et al. (2007). Oxygen dynamics and flow patterns of Dysidea avara (Porifera: Demospongiae). J. Mar. Biol. Assoc. UK 87, 1677–1682. doi: 10.1017/S0025315407058146
Schläppy, M. L., Weber, M., Mendola, D., Hoffmann, F., and de Beer, D. (2010a). Heterogeneous oxygenation resulting from active and passive flow in two Mediterranean sponges, Dysida avara and Chondrosia reniformis. Limnol. Oceanogr. 55, 1289–1300. doi: 10.4319/lo.2010.55.3.1289
Schläppy, M., Schöttner, S., Lavik, G., Kuypers, M. M. M., de Beer, D., and Hoffmann, F. (2010b). Evidence of nitrification and denitrification in high and low microbial abundance sponges. Mar. Biol. 157, 593–602. doi: 10.1007/s00227-009-1344-5
Schmitt, S., Tsai, P., Bell, J., Fromont, J., Ilan, M., Lindquist, N., et al. (2012). Assessing the complex sponge microbiota: core, variable and species-specific bacterial communities in marine sponges. ISME J. 6, 564–576. doi: 10.1038/ismej.2011.116
Shaked, Y., and Genin, A. (2014). The Israel National Monitoring Program in the Northern Gulf of Aqaba. Eilat: The Interuniversity Institute for Marine Sciences in Eilat.
Sipkema, D., and Blanch, H. W. (2010). Spatial distribution of bacteria associated with the marine sponge Tethya californiana. Mar. Biol. 157, 627–638. doi: 10.1007/s00227-009-1347-2
Taylor, M. W., Radax, R., Steger, D., and Wagner, M. (2007). Sponge-associated microorganisms: evolution, ecology, and biotechnological potential. Microbiol. Mol. Biol. Rev. 71, 295–347. doi: 10.1128/MMBR.00040-06
Thomas, T., Moitinho-Silva, L., Lurgi, M., Björk, J. R., Easson, C., Astudillo-García, C., et al. (2016). Diversity, structure and convergent evolution of the global sponge microbiome. Nat. Commun. 7:11870. doi: 10.1038/ncomms11870
Tian, R. M., Wang, Y., Bougouffa, S., Gao, Z. M., Cai, L., Bajic, V., et al. (2014). Genomic analysis reveals versatile heterotrophic capacity of a potentially symbiotic sulfur-oxidizing bacterium in sponge. Environ. Microbiol. 16, 3548–3561. doi: 10.1111/1462-2920.12586
Tu, B. P., Kudlicki, A., Rowicka, M., and McKnight, S. L. (2005). Logic of the yeast metabolic cycle: temporal compartmentalization of cellular processes. Science 310, 1152–1158. doi: 10.1126/science.1120499
Weisz, J. B., Hentschel, U., Lindquist, N., and Martens, C. S. (2007). Linking abundance and diversity of sponge-associated microbial communities to metabolic differences in host sponges. Mar. Biol. 152, 475–483. doi: 10.1007/s00227-007-0708-y
Weisz, J. B., Lindquist, N., and Martens, C. S. (2008). Do associated microbial abundances impact marine demosponge pumping rates and tissue densities? Oecologia 155, 367–376. doi: 10.1007/s00442-007-0910-0
Wilson, M. C., Mori, T., Ruckert, C., Uria, A. R., Helf, M. J., and Takada, K. (2014). An environmental bacterial taxon with a large and distinct metabolic repertoire. Nature 506, 58–62. doi: 10.1038/nature12959
Yahel, G., Marie, D., and Genin, A. (2005a). InEx - a direct in situ method to measure filtration rates, nutrition, and metabolism of active suspension feeders. Limnol. Oceanogr. Methods 3, 46–58. doi: 10.4319/lom.2005.3.46
Yahel, G., Sharp, J. H., Marie, D., Hase, C., and Genin, A. (2003). In situ feeding and element removal in the symbiont-bearing sponge Theonella swinhoei: Bulk DOC is the major source for carbon. Limnol. Oceanogr. 48, 141–149. doi: 10.4319/lo.2003.48.1.0141
Yahel, G., Whitney, F., Reiswig, H. M., Eerkes-Medrano, D. I., and Leys, S. P. (2007). In situ feeding and metabolism of glass sponges (Hexactinellida, Porifera) studied in a deep temperate fjord with a remotely operated submersible. Limnol. Oceanogr. 52, 428–440. doi: 10.4319/lo.2007.52.1.0428
Keywords: microsensors, porifera, oxygen, anoxia, suboxic, symbionts, bacteria, holobiont
Citation: Lavy A, Keren R, Yahel G and Ilan M (2016) Intermittent Hypoxia and Prolonged Suboxia Measured In situ in a Marine Sponge. Front. Mar. Sci. 3:263. doi: 10.3389/fmars.2016.00263
Received: 25 July 2016; Accepted: 28 November 2016;
Published: 09 December 2016.
Edited by:
Mike Taylor, University of Auckland, New ZealandReviewed by:
Simon K. Davy, Victoria University of Wellington, New ZealandGeorg Steinert, Wageningen University, Netherlands
Copyright © 2016 Lavy, Keren, Yahel and Ilan. This is an open-access article distributed under the terms of the Creative Commons Attribution License (CC BY). The use, distribution or reproduction in other forums is permitted, provided the original author(s) or licensor are credited and that the original publication in this journal is cited, in accordance with accepted academic practice. No use, distribution or reproduction is permitted which does not comply with these terms.
*Correspondence: Adi Lavy, bXIuYWRpLmxhdnlAZ21haWwuY29t
†These authors have contributed equally to this work.