- 1National Health and Environmental Effects Research Laboratory, Atlantic Ecology Division, Office of Research and Development, U.S. Environmental Protection Agency, Narragansett, RI, USA
- 2Division of Fish and Wildlife, Rhode Island Department of Environmental Management, Jamestown, RI, USA
Excessive input of nitrogen to estuaries and coastal waters leads to eutrophication; the resulting organic matter over-enrichment of sediments and seasonal hypoxia of bottom water have significant deleterious effects on benthic community biodiversity, abundance, and biomass. Our goal was to better understand how these losses carry through to impairment of key ecosystem functions of benthic communities. Recent management efforts to address eutrophication have reduced nitrogen loading to several estuaries of the Virginian Biogeographic Province (northeast United States). How the ecosystems will respond remains to be seen. Using Narragansett Bay as an example estuary within this Province, we compared measures of community structure and function from stations in seasonally hypoxic areas with stations in normoxic areas. We analyzed a benthic data set spanning 20 years (1990–2010) and 155 stations, along with ancillary data from other sources. Hypoxic areas had half the species richness, many fewer rare species, lower biomass, and lower secondary production. Benthic communities in the hypoxic areas had a significantly different abundance structure, were at an earlier successional stage, and bioturbated the sediments to a depth about one-fifth that of the normoxic areas. On average, sediments in the hypoxic areas took up more oxygen—used for aerobic metabolism and oxidation of reduced compounds from anaerobic metabolism. Sediments in hypoxic areas released into the overlying water two to three times more ammonium and phosphate. Mean flux of dissolved oxygen into the sediments of hypoxic areas and mean net flux of nitrogen gas (from sediment denitrification) out were slightly higher. Eutrophication-driven over-enrichment of organic matter, along with seasonal hypoxia in the northern part of the Bay have led to degradation of benthic community structure and function, which have serious implications for sustainable provision of ecosystem services. We quantified fifteen stressor-response relationships that can help understand how, following a reduction in nitrogen inputs, a recovery of benthic ecosystem functions in hypoxic areas could proceed.
Introduction
Excessive input of nitrogen to estuaries and coastal waters leads to eutrophication and hypoxia. Organic matter over-enrichment of sediments and seasonal hypoxia of bottom water have well-known deleterious effects on benthic community biodiversity, abundance, and biomass (Diaz and Rosenberg, 1995). How these losses carry through to impairment of key ecosystem functions of benthic communities—and the potential loss of important ecosystem services—are less well-known. Recent management efforts have resulted in reduced nitrogen loading to several estuaries in the northeast United States. In many cases, how the ecosystems will respond is not yet known.
Globally, hypoxia and anoxia are among the most widespread deleterious anthropogenic influences on estuarine and marine ecosystems (Gray et al., 2002; Wu, 2002; Diaz and Rosenberg, 2008; CENR, 2010; Zhang et al., 2010). Although hypoxia and anoxia occur naturally in many ecosystems, increased human-related inputs of nutrients have increased both their frequency and extent (Diaz and Rosenberg, 2008, 2011). In the U.S., almost half of the estuaries are subject to seasonal hypoxia resulting primarily from excess nitrogen loading (Bricker et al., 2007; CENR, 2010). In the Virginian Biogeographic Province along the northeast coast of the United States, eutrophication is one of the most critical contributors to losses of marine biodiversity (NRC, 1995; Bricker et al., 2007; CENR, 2010; USEPA, 2012). Reduction of benthic biodiversity matters because of the consequent changes in ecosystem function (Levin et al., 2009; Carstensen et al., 2014; Gogina et al., 2014) and the services they provide to human well-being (Weslawski et al., 2004; Goulletquer et al., 2014).
Declines in global biodiversity caused by human disturbances in many different biomes have raised concern over the consequences of species loss on the ability of ecosystems to provide services that benefit human well-being (Chapin et al., 2000; Worm et al., 2006; Goulletquer et al., 2014). Ecosystem consequences of altered biodiversity include fewer species interactions, less resistance and resilience to change, lowered resistance to invasions, and less ecosystem functionality (Chapin et al., 2000; Goulletquer et al., 2014). Much theoretical, empirical, and experimental research has been done in the past two decades on the relationship between biodiversity and ecosystem functioning. Results reveal complexity but show a strong positive relationship (e.g., Waldbusser et al., 2004; Balvanera et al., 2006; Solan et al., 2006; Worm et al., 2006; Goulletquer et al., 2014).
In the marine environment, changes in food-web diversity and the resulting trophic cascades have caused large, unexpected, irreversible changes of ecosystem services, such as edible fish and shellfish species, with high environmental, economic, and cultural losses (Goulletquer et al., 2014). Soft-bottom marine habitats, which cover a large proportion of the Earth's surface, support benthic communities with high biodiversity (Snelgrove, 1998). Ecosystem functions driven by benthic biodiversity have global importance, including biogeochemical cycling, metabolism and burial of pollutants, and secondary production (Snelgrove, 1998; Covitch et al., 2004). These functions are directly related to the numerous ecosystem services provided by benthic communities (Snelgrove et al., 1997; Levin et al., 2001; Weslawski et al., 2004; Goulletquer et al., 2014). Some functions, such as those associated with anaerobic processes, are not normally performed by other marine communities (Middelburg and Levin, 2009).
Organic carbon settling to the bottom (direct sources as well as indirect sources from nitrogen-fueled primary production) provides essential food for most bottom organisms. However, loading the bottom waters and sediment habitat with more organic carbon than the benthic community can process aerobically through the heterotrophic degradation of organic matter results in hypoxia and increased sediment carbon content. Over-enrichment of organic matter, even in the absence of hypoxia, can have harmful effects on the benthos and their habitat (Hyland et al., 2005). However, many harmful effects resulting from nutrient over-enrichment are more likely caused by hypoxia rather than organic over-enrichment alone (Gray et al., 2002). Nevertheless, benthic communities in northeast U.S. estuaries typically are exposed to hypoxia only periodically in the summer, whereas those same communities living in sediments over-enriched with organic carbon are exposed to that condition year-round.
In contrast with the overlying water column, hypoxia and anoxia are inherent characteristics of marine sediments; essentially, all marine sediments are anoxic below the top few oxygenated centimeters (Fenchel and Finlay, 1995; Middelburg and Levin, 2009). Sediment anaerobic conditions are necessary for the normal functioning of current-day global biogeochemical cycles of critical elements such as carbon, nitrogen, phosphorus, and sulfur. However, most marine benthic invertebrates require oxygen as the electron acceptor for their metabolism and are limited to a thin centimeters-thick oxygenated surface layer (Middelburg and Levin, 2009). Effects of organic enrichment and hypoxia on marine benthic organisms, populations, and communities have been extensively reviewed (e.g., Diaz and Rosenberg, 1995, 2008; Gray et al., 2002; Wu, 2002; Vaquer-Sunyer and Duarte, 2008; Levin et al., 2009; CENR, 2010; Zhang et al., 2010) and are summarized in Supplementary Table S1. We studied how these effects carry through to affect benthic ecosystem functions and discuss potential implications for ecosystem services.
The goal of this study was to quantify how nitrogen-driven eutrophication and hypoxia degrade benthic community structure and ecosystem functions. We aimed to establish stressor-response relationships that can be used in models to better understand the future functional response of benthic communities to reductions in nitrogen loading. We used Narragansett Bay (Rhode Island and Massachusetts in the northeast U.S.) as an example of a Virginian Biogeographic Province (Cape Cod, Massachusetts, to Cape Hatteras, North Carolina) estuary. The Bay is a relatively data-rich temperate estuary that allows examination of several structural and functional aspects of benthic communities. Further, current reductions in nitrogen loading to Narragansett Bay make it a good case study to address the many questions regarding what effects the reduction will have on the Bay. We expect community structural parameters, such as number of species, abundance, and biomass will follow the classical gradient of decreasing organic enrichment (Pearson and Rosenberg, 1978; Supplementary Figure S1). Our goal was to estimate what to expect in terms of recovered benthic ecosystem functions.
Methods
Study Area and Narragansett Bay Benthos
Narragansett Bay (Figure 1) is a 380 km2 temperate northeastern U.S. estuary with a 4421-km2 watershed holding (in 2010) over 1.9 million people with a mean population density of 434 people per km2. In 2014, 38% of the watershed was urbanized or built-up land use, 40% was forested, and only 5% agricultural (Chintala et al., 2015). Mean water depth is 8.3 m and mean tidal range is 1.1–1.4 m (Pilson, 1985). The Bay has a mean salinity of 27–33 (Pilson, 1985) with relatively small areas of oligohaline (salinity 0–5) or mesohaline (salinity 5–18) waters. Flushing time of the Bay varies from 10–40 days with a mean of 26 days (Pilson, 1985). Benthic species of Narragansett Bay show high biodiversity and are an important functional part of the Bay estuarine ecosystem, where the pelagic and benthic systems of the Bay are closely coupled (Nixon et al., 1976; Beatty, 1991).
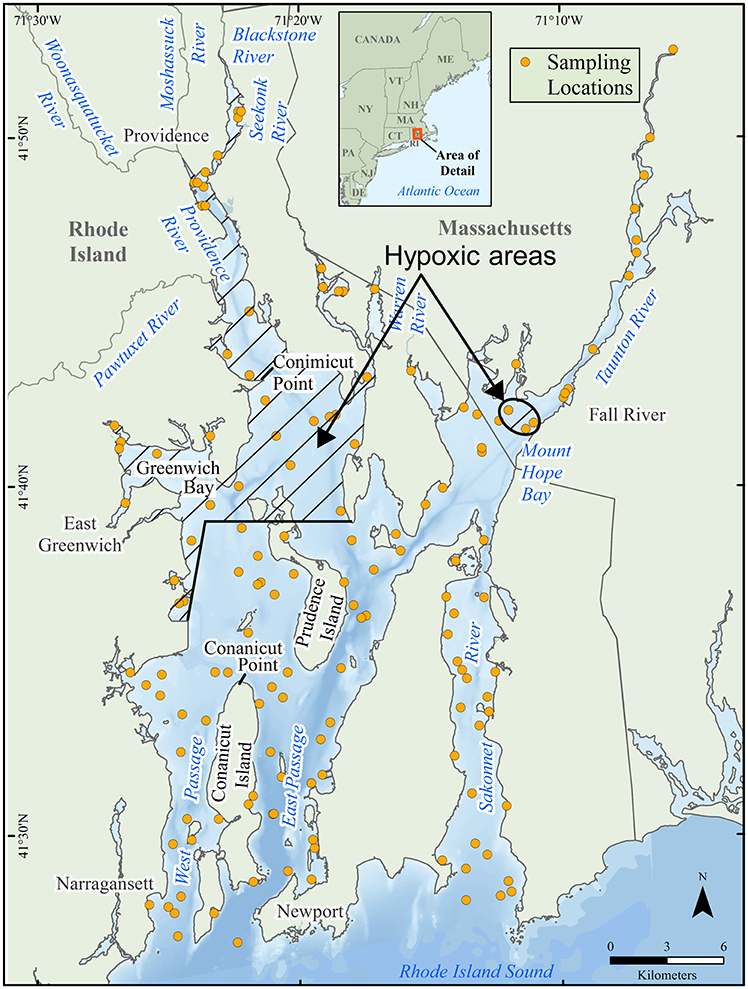
Figure 1. Narragansett Bay showing 155 National Coastal Assessment stations (NCA, 2016). Areas subject to periodic hypoxia are shown with cross-hatching.
Parts of the Bay have undergone eutrophication since the late 1800s (Bricker et al., 2007; Nixon et al., 2008). Sewage is the main source of total nitrogen input. The largest rivers and all the large wastewater treatment facilities (WWTFs) and combined sewer overflows (CSOs) enter the upper end of the Bay, resulting in the highest levels of sediment total organic carbon (TOC) (Murray et al., 2007). Periodic hypoxia, which can occur from May to October, currently affects the upper one-third of the Bay but does not persist for the entire summer season (Codiga et al., 2009). Hypoxic events typically are linked to stratification during the hot summer months of July and August, often when weak neap tidal currents and lack of storms fail to break up stratification, and high water temperatures create favorable conditions for hypoxia (Prell et al., 2004, 2006, 2015; Deacutis et al., 2006; Deacutis, 2008). Summer surveys of DO in the upper half of Narragansett Bay (Prell et al., 2004, 2006, 2015; Deacutis et al., 2006; Insomniacs Day Trippers, 2016) and a network of fixed buoys (BART, 2016) show that the upper part of the Bay experiences periodic hypoxia that can persist days or weeks in the summers of most years. The area <4.8 mg/L (suboxic) can cover up to 100% of the upper part of the Bay, the area < 2.9 mg/L up to 70%, and the area < 1.2 mg/L up to 20% (Deacutis et al., 2006; Melrose et al., 2007; Saarman et al., 2008). Hypoxia and fish kills, first observed in Narragansett Bay in 1898 (Nixon et al., 2008), have occurred nearly every summer since 1994, including the extensive August 2003 event that killed over a million fish, along with numerous benthic invertebrates (RIDEM, 2003; BART, 2016).
We used the following definitions for concentrations of bottom water DO: Anoxic 0–0.1 mg/L; Severely hypoxic < 1.2; Hypoxic > 0.1 to < 2.9; Suboxic ≥ 2.9 to < 4.8; Normoxic ≥ 4.8. For Narragansett Bay, we defined normoxia as bottom water DO concentrations ≥ 4.8 mg/L because lower levels can force behavioral changes, inhibit growth, and kill embryos and larvae—changes that are not normal in the sense of a healthy, fully-functioning, temperate estuarine ecosystem (Diaz and Rosenberg, 1995; USEPA, 2000; Miller et al., 2002; Wu, 2002; Vaquer-Sunyer and Duarte, 2008; Steckbauer et al., 2011; Carstensen et al., 2014).
Data
In 1990, the National Coastal Assessment (NCA, 2016) began sampling estuarine and coastal bottom environments and communities to assess their ecological condition. Starting in 2010, the program continued as the National Coastal Condition Assessment (NCCA). Both programs used a probabilistic design of stations selected randomly, along with a standardized protocol for sample collection and analysis. Samples were taken during a summer index period (July through September) when it was assumed that certain stresses (e.g., hypoxia, elevated temperature) to the benthic community would be high. Benthic macroinvertebrate assemblages were sampled using a 0.04-m2 Young-modified Van Veen grab and samples were sieved with a 0.5-mm mesh. Concurrent sediment samples were taken for grain size, chemical contaminants, and toxicity; physical-chemical properties of the bottom water also were measured. We extracted from the national 1990–2010 data set the 155 stations sampled in Narragansett Bay (Figure 1). Hereafter, we refer to this data set as NCA-NCCA. We used these data mainly for community structural properties; for most functional properties, we analyzed data drawn from other studies, as listed in the following sections. The 1990–2006 data used in the analyses are available online at NCA (2016) and 2010 data are available at NCCA (2016).
Comparison of Structural and Functional Properties of Benthic Communities in Hypoxic Areas with Normoxic Areas of Narragansett Bay
First, to discern the broader regional pattern of how various levels of hypoxia and sediment carbon can put a cap on benthic biodiversity, we plotted species richness (S) against bottom water DO and sediment total organic carbon (TOC). We used 2000–2006 data from 1229 NCA-NCCA estuarine and coastal stations in the Virginian and Acadian Biogeographic Provinces (from Chesapeake Bay to the Canadian border; Hale, 2010). If the level of DO is high, biodiversity can vary widely because of other factors (Supplementary Figure S2). But if DO is low, biodiversity cannot be high. TOC imposes a similar upper limit on potential biodiversity (Supplementary Figure S2). We hypothesized that lost benthic biodiversity because of organic over-enrichment and hypoxia results in reduced ecosystem functions, with implications for ecosystem services. We tested this in Narragansett Bay by comparing hypoxic areas with normoxic areas.
Using a technique applied by other researchers in both Narragansett Bay (Rudnick, 1984; Fulweiler, 2007; Calabretta and Oviatt, 2008; Oviatt, 2008; Schult, 2010) and other estuaries (e.g., Ritter and Montagna, 1999; Montagna and Froeschke, 2009; Gogina et al., 2014), we grouped the data into two categories: Those in seasonally hypoxic areas and those in normoxic areas. We defined seasonally hypoxic areas as those areas with frequent summer episodes of DO < 2.9 mg/L lasting one to several days, occasional episodes of anoxia, and a July-August mean < 4.8 mg/L. These areas were delineated (Figure 1) using hypoxia maps of Prell et al. (2004, 2006); Krahforst and Carullo (2008); Saarman et al. (2008), and data from surveys (Prell et al., 2015) and fixed buoys (BART, 2016). These areas roughly correspond with the four eutrophic zones of Deacutis (2008). The delineation of hypoxic areas is not precise—the intensity, extent, and duration of hypoxia in the Bay varies from year to year; there are spots inside the hypoxic areas that are not hypoxic every year and spots in the normoxic areas that are hypoxic some years. The 1990–2010 data were pooled, as the signal from sewage additions in the upper Bay dominates all other changes (Nixon et al., 2008) and the largest reductions in nitrogen input from the sewage treatment plants began after the last year of the NCA-NCCA dataset. Each area held a variety of habitat types included by the random selection of stations. The means can serve as first approximations for use in models attempting to forecast future benthic conditions as nitrogen inputs decline.
The major stressors on the benthic communities in the Bay, in addition to over-enriched sediments and hypoxia, are sediment contaminants and toxicity (NCA, 2016). Because our objective was to examine the effect of hypoxia and TOC on biodiversity in the absence of other stressors, we removed stations with polluted sediments. This was done by dropping 33 stations where ≥10 heavy metal and organic contaminants in the sediments exceeded the Effects Range Low or ≥1 contaminant exceeded the Effects Range Median (see Hale and Heltshe, 2008 for details), leaving 24 stations in the hypoxic areas and 93 in the normoxic areas. For most of the parameters listed in the following sections, we used a two-sided t-test (SAS ver. 9.4) to compare the mean of the hypoxic areas with the mean of the normoxic areas to test the null hypothesis that there was no significant difference between the two means. When the two variances were equal, we used a pooled test; when unequal, a Satterwaithe test.
Species Biodiversity and Rareness
There were 342 unique taxa (318 identified to the species level) in the entire NCA-NCCA data set spanning 20 years and 155 stations. The ten species most commonly occurring (and the ten most abundant) in hypoxic and normoxic areas in the 1900–2010 NCA-NCCA data set are shown in Table 1. We tested the null hypothesis that the mean species richness of stations in the seasonally hypoxic areas was the same as that in the normoxic areas. Even though the size of the areas was different, the low likelihood of capturing additional species with further sampling (based on species accumulation curves) renders this comparison feasible. Although species richness can vary with salinity, in this case all but 4 (in the upper Taunton River) of the 155 stations had polyhaline or euhaline bottom water, so we did not apply a salinity correction, a conclusion also drawn by Oviatt (2008) in a study of gradients in the Bay.
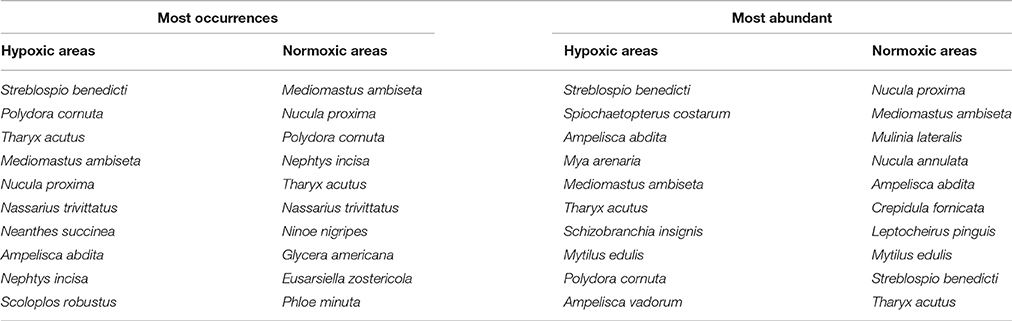
Table 1. Most common (frequency of occurrences) and most abundant benthic macrofauna (> 0.5 mm) in periodically hypoxic areas vs. normoxic areas of Narragansett Bay (NCA-NCCA data set).
The majority of the 342 taxa found were rare; 58% of the 342 taxa had < 25 individuals. We calculated the number of occurrences of each taxon identified to species level in the entire Narragansett Bay NCA-NCCA data set spanning 20 years and 155 stations. Then we calculated, for each station, the number of species with one occurrence (singletons) in the entire data set that occurred at that station. The same was done for species with two occurrences (doubletons).
Species Abundance and Community Structure
Using NCA-NCCA data, we prepared dominance plots (Clarke et al., 2014) for both areas to look at evenness; typically, impacted benthic communities are dominated by a few tolerant species in high numbers. To look at differences in community species abundance structures, we ran an ordination (non-metric multidimensional scaling, or NMDS) on a Bray-Curtis similarity matrix of NCA-NCCA species abundance data (PRIMER 7, PRIMER-E Ltd, Plymouth, UK; Clarke et al., 2014). We used all the species because one of the prominent effects of eutrophication and hypoxia on benthic communities is a sharp reduction in the number of rare species. An analysis of similarity (ANOSIM) was used to check for significance. To help explain why community composition was different between the two areas, we ran the SIMPER routine of PRIMER that identifies the species that mainly contributed to the differences.
Additionally, using data from MERL (2016) and Rudnick (1984), we compared the total abundance of meiofauna (≤ 0.3 mm) at a station in the hypoxic areas (Conimicut Point in the Providence River) with the same taxa at a reference station (North Jamestown) off Conanicut Point in the mid-Bay normoxic area. This station is dominated by the typical Nephtys-Nucula community of southern New England (Table 1) and has been sampled by several different studies.
Biomass and Secondary Production
Other than data from a study of waterfowl feeding on the benthic community, benthic biomass data from Narragansett Bay are scarce. The NCA-NCCA study measured biomass just during the first 4 years, with only one station in the hypoxic area. That station in the Providence River was anoxic on the day of sampling and the only organisms present were small oligochaetes with miniscule measured biomass (0.01 mg). Hence, we were not able to conduct a t-test. We were able to use data from Schult (2010), who sampled four stations in the upper to mid-Bay (0.04 m2 grab, 2.0 mm mesh sieve). Schult found enough taxa so that we could do a species-level station-to-station t-test comparing the Providence River station with the North Jamestown reference station. Data from the waterfowl feeding study included 28 stations throughout the Bay (2.0 mm sieve; 30 species of macrofauna reported; McKinney et al., 2004; R. McKinney, unpublished data) and had adequate sample size to do a t-test on stations in each area. Despite the frequent summer hypoxia, the Schult (2010) station in the hypoxic area near Conimicut Point had high quahog densities because this species can tolerate some hypoxia (Altieri and Witman, 2006). Like Sturdivant et al. (2014), we removed quahogs from both the Schult and McKinney data prior to the t-test.
For calculation of secondary production, we used biomass and bottom water temperature data from NCA-NCCA and Schult (2010) in the formula of Edgar (1990):
P2 = 0.0049 * B0.80*T0.89
where: P2 is secondary production in μg C/d
B is biomass in μg ash-free dry weight
T is temperature of the bottom water in °C
We modified this following the methods of Sturdivant et al. (2014) who, rather than calculating the production of size classes from a series of different mesh sizes, calculated the production of each taxon at a station. We ran a t-test with the Schult (2010) data but were not able to with the NCA-NCCA because of inadequate sample size. Temperature data were not available for the McKinney et al. (2004) study.
Bioturbation and Community Successional Stage
We used two parameters from a Bay-wide sediment profile imaging (SPI) (56 stations) done by Valente et al. (1992): The depth of the apparent redox potential discontinuity (aRPD) layer and the community successional stage (0–3).
Metabolism and Nutrient Cycling
We compared measurements of oxygen, ammonium (NH4), phosphate (PO4), and nitrogen gas (N2) fluxes from five studies (Nixon et al., 1976, 1980; McCaffrey et al., 1980; Elderfield et al., 1981; Fulweiler et al., 2007) at stations in the hypoxic areas with those at stations in the normoxic areas. Benthic communities take up oxygen both for aerobic respiration and for oxidation of reduced compounds such as hydrogen sulfide (H2S). Because these reduced compounds were generated by microbial anaerobic respiration, oxygen uptake can be considered a rough estimate of total community respiration (Middelburg and Levin, 2009).
Results
Species Biodiversity and Rareness
Mean number of species (S) was lower in the hypoxic areas (S = 17) than in the normoxic areas (S = 30, p < 0.001; Table 2). There were 165 species that occurred only once, and 81 species that occurred only twice. Many rare species found in normoxic areas of Narragansett Bay were not present in hypoxic areas. Some species are rare because they are sensitive to disturbances or they are on the edge of their preferred habitat. Others are rare because they were not efficiently captured by the gear used. In total, the hypoxic areas had only 5% as many singletons and 16% as many doubletons as did the noxmoxic areas. Means of stations in the hypoxic areas had a quarter as many singleton species and half as many doubletons (Table 2).
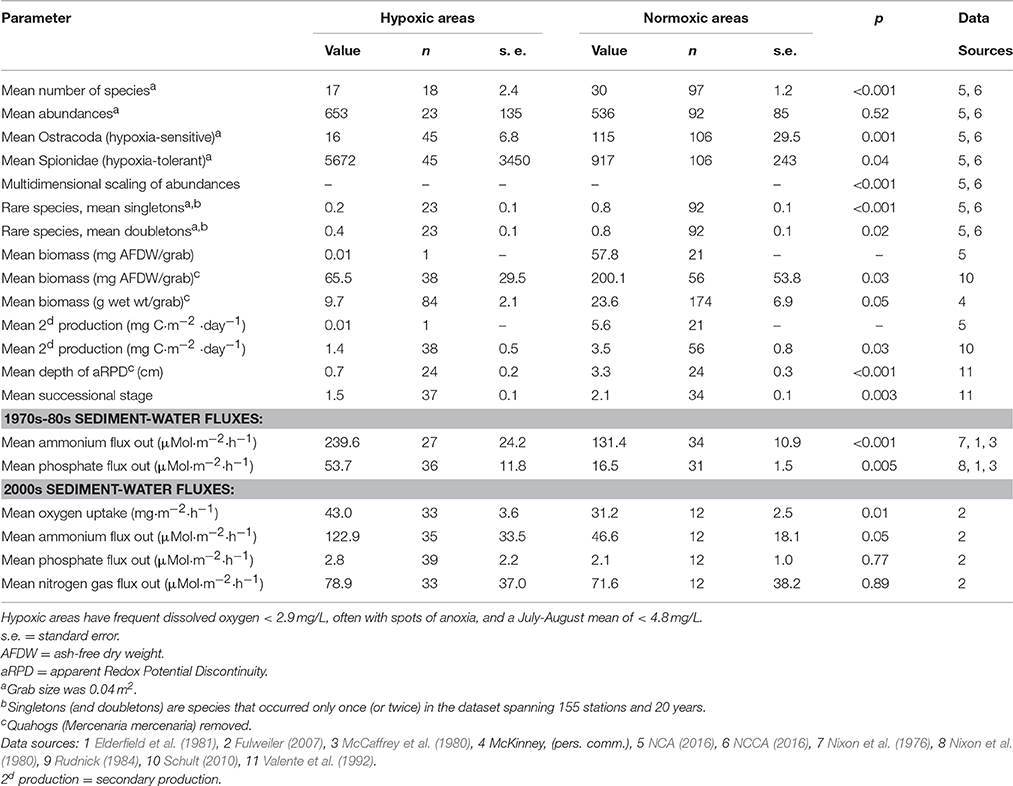
Table 2. Benthic structural and functional parameters in hypoxic areas vs. normoxic areas of Narragansett Bay.
Species Abundance and Community Structure
The dominance plot for species ranked by abundance (Figure 2) showed how, in stressed areas, hypoxia-tolerant species dominated species abundance. It also showed the wide variability and long right-skewed tail comprising rare species that is typical of benthic abundance data. The mean of total station species abundances in the hypoxic areas was higher than in the normoxic areas, but the difference was not significant (p = 0.52). However, mean abundances of hypoxia-sensitive and hypoxia-tolerant species were significantly different. For example, mean abundance of Ostracoda, a group of hypoxia-sensitive crustaceans, was lower in the hypoxic areas (16 vs. 115, Table 2) and mean abundance of Spionidae, a family of polychaetes with several hypoxia-tolerant species, was higher in the hypoxic areas (5672 vs. 917; Table 2). The ordination of the community abundance data showed that although there is overlap, the structure of the benthic communities at stations in the seasonally hypoxic areas was significantly different (p < 0.001) from that at stations outside the area (in Figure 3, three hypoxic stations were off the scale of the plot; these stations were included in the ANOSIM and contributed to the significant difference between stations in the two areas). From the SIMPER analysis, species in the hypoxic areas that contributed most to the dissimilarity included some well-known indicator species of organic enrichment (first four most commonly-occurring species in the second column of Table 1, along with Tubificidae), while those in the normoxic areas contributing the most included Nepthys incisa and Nucula proxima, common benthic assemblage dominants in Narragansett Bay. Regarding meiofauna, total abundance of all species, all years, was 0.6 to 0.7 lower in the hypoxic areas than in normoxic areas.
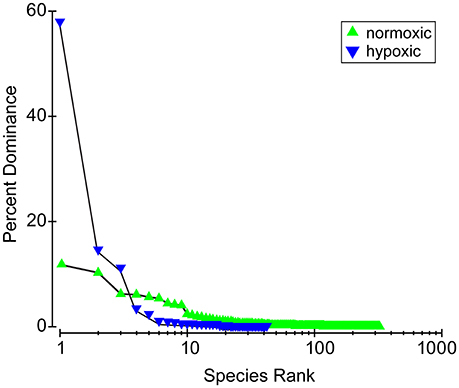
Figure 2. Species dominance curves in hypoxic areas and normoxic areas. Three species dominate the hypoxic areas and the right tail of rare species contributing to biodiversity is much shorter.
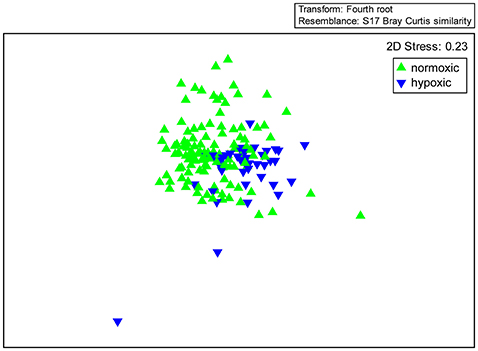
Figure 3. Ordination (non-metric multidimensional scaling) of species abundances in hypoxic and normoxic areas. Another three hypoxic stations were off the scale of this plot.
Biomass and Secondary Production
Mean biomass with the NCA-NCCA data was considerably lower in the hypoxic area than in the normoxic areas (Table 2). With small oligochaetes being the only taxon present at the station in the hypoxic area, we were not able to conduct a statistical test; however, statistics were not needed to conclude that the biomass in the hypoxic area (0.01 mg ash-free dry weight/0.04 m2 grab) was severely diminished relative to the normoxic area (57.8 mg ash-free dry weight). Mean biomass in the Schult (2010) data at the station in the hypoxic areas (ash-free dry wt of 65.5 mg/m2) was substantially lower than the mean in the normoxic areas (203.8 mg/m2; p = 0.03; Table 2). With the waterfowl feeding data (R. McKinney, unpublished data), biomass was 9.7 wet wt g/m2 in hypoxic areas and 23.6 wet wt g/m2 in normoxic areas, significantly different at p = 0.05.
Secondary production estimates here are preliminary because of the limited biomass data. Mean secondary production with the NCA-NCCA data in the hypoxic area was severely depressed (0.01 mg C m−2 day−1, compared with 5.6 mg C m−2 day−1 in the normoxic areas; Table 2). With the Schult (2010) data, mean taxa secondary production at the station in the hypoxic areas (1.4 mg C m−2 d−1) was significantly less (p = 0.03) than the mean in the normoxic areas (3.5 mg C m−2 d−1; Table 2).
Bioturbation and Community Successional Stage
The mean aRPD in the hypoxic areas (0.7 cm) was significantly different (p < 0.001) than in the normoxic areas (3.3 cm; Table 2). This is likely due to a hypoxia-induced loss of large macrofauna, leading to a loss of bioturbation (Valente et al., 1992). In the hypoxic areas, community successional stage was held at a significantly (p = 0.003) earlier stage (1.5 vs. 2.3). These two parameters are illustrated by the sediment profile images taken in the hypoxic and normoxic areas of Narragansett Bay (Supplementary Figure S1).
Metabolism and Nutrient Cycling
Fluxes of oxygen, nitrogen, and phosphate across the sediment water interface are strongly influenced by eutrophication and hypoxia, but different processes can result in either fluxes out of the sediments or into the sediments. Individual stations had fluxes out of the sediments, while other stations in the same area had fluxes into the sediments. Our results report on the net flux (Table 2). In general, uptake of DO and release of NH4 and PO4 by the sediments at stations in the hypoxic areas were higher than those in normoxic areas (Table 2). Oxygen uptake was significantly higher (p = 0.01) in hypoxic areas than in normoxic areas (Table 2). Net N2 flux can be out of the sediments (from denitrification) or into the sediments (N-fixation; Fulweiler and Nixon, 2012) and the mean net fluxes represent a mixture of both processes. Mean net N2 flux from the sediment in the hypoxic areas was slightly higher than in the normoxic areas but the difference was not significant. Phosphate also can be either taken up or released by sediments. In both the 1970s–80s and the 2000s data, PO4 flux from the sediments was higher in hypoxic areas than in normoxic areas; however, the rates in the later data were substantially lower.
Discussion
We found that year-round organic over-enrichment and periodic summer hypoxia in Narragansett Bay decreased benthic biodiversity, abundance, biomass, secondary production, bioturbation, and had strong effects on community structure and successional stage, metabolism, and nutrient cycling. These eutrophication and hypoxia-induced changes in coastal ecosystems can have large socio-economic impacts (Zhang et al., 2010).
Benthic Biodiversity
Consequences of hypoxic areas of Narragansett Bay holding half as many species as normoxic areas are numerous, including impaired capacity to provide food and maintain water quality (Solan et al., 2006; Worm et al., 2006). Major changes in estuarine functions result when benthic species losses lead to a reduction in the types of food available to predators; removal of large bioturbators that rework sediments; loss of suspension-feeding bivalves that clear bottom water; changes in reef or mat habitat; or collapse of facilitative or mutualistic interactions (CENR, 2010; Goulletquer et al., 2014).
Species Abundance and Community Structure
Mean total community abundance in the hypoxic areas was slightly higher than in the normoxic areas, but not significantly so. Intense or prolonged hypoxia can drastically reduce abundance of most benthic groups (e.g., Ritter and Montagna, 1999; Seitz et al., 2009; Sturdivant et al., 2014). Species abundance is important for many functional properties including bioturbation and trophic transfer to predators. However, with increasing stress, total community abundance can initially increase because high abundances of small, opportunistic species can overcome the decline of hypoxic-sensitive species (Supplementary Figure S1). A better measure is the abundance of individual keystone species or of functional groups. For example, hypoxia in the upper reaches of Narragansett Bay has resulted in local and ecological extinction (no longer sufficiently abundant to perform functional roles) of blue mussel reefs (Altieri and Witman, 2006). Loss of foundation species, which dominate an ecological community in biomass and habitat engineering effects, can have wide-reaching consequences (Weslawski et al., 2004). With regard to meiofauna, the substantially lower total abundance in hypoxic areas is likely to have a negative effect on community energy flow because many meiofauna species are prey items for macrofauna species.
The multi-dimensional scaling showing that the hypoxic areas had a significantly different community structure than the normoxic areas has important ramifications for ecosystem functions (Valente et al., 1992; Nilsson and Rosenberg, 2000; Gogina et al., 2014). The structure in the hypoxic areas was one of a community in a significantly earlier successional stage, dominated by small and opportunistic species, and less capable of ecosystem processes such as bioturbation and secondary production. The loss in the hypoxic areas of mature benthic communities characterized by large, long-lived organisms means a loss of important ecosystem functions of secondary production, water filtration, and effects of bioturbation on oxidation and nutrient cycling (Snelgrove, 1998; Weslawski et al., 2004; Rosenberg et al., 2009; Rakocinski, 2012; Norkko et al., 2013). Exposing benthic communities to periodic summertime hypoxia is the underwater equivalent of clear-cutting a forest—it keeps the community in an early successional state that reduces or eliminates the functions performed by large, long-lived, deep-burrowing animals.
Biomass and Secondary Production
All three data sets examined showed a lower biomass in hypoxic areas (after quahogs were removed). While the sample sizes were low in two of the data sets, it is important to understand how biomass and secondary production (a critical ecosystem function) respond. Results of this study generally agreed with other biomass studies (Ritter and Montagna, 1999; Seitz et al., 2009; Sturdivant et al., 2014). A mean biomass in the hypoxic areas of Narragansett Bay that is one-fifth to one-third that of the normoxic areas represents a dramatic reduction in potential food available for higher trophic levels such as fish. It demonstrates that some of the hypoxic areas are far to the right on the Pearson-Rosenberg enrichment gradient. Total biomass can be skewed by the presence of large bivalves such as quahogs which can be abundant in the lower Providence River-Greenwich Bay hypoxic areas. Quahogs can survive the relatively short-term hypoxia of Narragansett Bay and benefit from reduced predation pressure (Altieri, 2008). In the Baltic Sea, the ocean quahog Arctica islandica does the same (Gogina et al., 2014). In Chesapeake Bay, seasonal hypoxia leads to loss of large, multi-year age structure macrofauna and the associated biomass (Sturdivant et al., 2014). Low biomass at DO levels below about 4.5 mg/L provides poor foraging for blue crabs and demersal fish; above 4.5 mg/L, biomass is up to four times greater (Seitz et al., 2009). In Corpus Christi Bay, Texas, Ritter and Montagna (1999) showed a transition point between normoxic and hypoxic benthic communities (based on diversity, abundance, and biomass of adults) at a DO level of 3.5–4.0 mg/L; a similar level (4.0 mg/L) in the Baltic Sea greatly increases benthic biomass over that of lower DO levels (Carstensen et al., 2014).
While our estimates of secondary production by benthic communities are preliminary, both of the studies for which we were able to calculate estimates showed severely depressed secondary production in the hypoxic areas of Narragansett Bay. This cannot support a normal level of energy transfer to fish (Levin et al., 2009; Dolbeth et al., 2012; Gogina et al., 2014). In the Rappahannock River of Chesapeake Bay, Sturdivant et al. (2014) reported that the summer mean daily macrobenthic secondary production at hypoxic (< 2.8 mg/L) sites was significantly lower than at sites with higher DO levels, resulting in 90% less production. Overall, Chesapeake Bay's macrobenthic production is reduced 20–35% as a result of hypoxia during the summer (Sturdivant et al., 2014). In hypoxic areas, energy flow switches from higher trophic levels such as fish and shellfish to anaerobic pathways by microbenthos that are less efficient than aerobic pathways (Diaz and Rosenberg, 1995).
Bioturbation and Bio-Irrigation
A mean depth of the sediment Redox Potential Discontinuity in hypoxic areas of Narragansett Bay that is almost one-fifth that of normoxic areas represents a dramatic reduction in thickness of the bioturbated zone, with serious consequences for biodiversity, organic matter processing, nutrient cycling, and secondary production (Rosenberg et al., 2001; Solan et al., 2004; Levin et al., 2009; Middelburg and Levin, 2009). Additionally, the community in hypoxic areas was at an earlier successional stage that is less capable of bioturbation. At a site in Chesapeake Bay, as hypoxia increased and infauna were exposed to H2S, reduced rates and depth of bioturbation were caused by reductions in burrow production (reduced by 75%), burrow lengths, and burrowing depth (Sturdivant et al., 2014). Bioturbation and bio-irrigation by the large, deep-dwelling species found in successionally mature communities is needed to maintain good quality benthic habitat and is a primary determinant of sediment oxygen concentrations (Levinton, 1995; Solan et al., 2004; Cicchetti et al., 2006; Norling et al., 2007; Middelburg and Levin, 2009).
Metabolism
Sediments in the hypoxic areas of Narragansett Bay, on average, took up more DO from the bottom water than those in the normoxic areas. Oxygen availability in bottom-water is one of the main factors governing benthic metabolic processes. Most oxygen consumption in coastal sediments goes to re-oxidation processes (oxidation of reduced compounds that were produced by earlier anaerobic metabolism), with typically less than 25% of the oxygen consumption going to aerobic respiration (Middelburg and Levin, 2009). Bacterial sulfate reduction dominates anaerobic respiration in most coastal sediments as a result of rich organic matter and high concentrations of sulfate in the bottom water (McCaffrey et al., 1980; Middelburg and Levin, 2009; Zhang et al., 2010). Therefore, the higher DO uptake in the hypoxic areas of Narragansett Bay was likely a result of more anaerobic respiration than that which occurred in sediments in normoxic areas.
Nutrient Cycling
Sediments in hypoxic areas of Narragansett Bay consistently released more NH4 than normoxic areas. Oxygen availability and supply of organic matter to the bottom are the main factors governing nutrient recycling (Nixon et al., 1976; Fulweiler et al., 2007). A positive feedback to eutrophication can occur when low DO conditions inhibit nitrification-denitrification, thereby increasing the flux of NH4 out of the sediments, which stimulates phytoplankton growth and, hence, associated bottom water hypoxia (Levin et al., 2009; Middelburg and Levin, 2009; Zhang et al., 2010; Fulweiler and Nixon, 2012). In the 1970s–1980s, sediments in hypoxic areas released into the overlying water about 3 times more PO4 than in normoxic areas. In the 2000s, PO4 fluxes were much lower, possibly a result of a previous bout of hypoxia not followed by a new input of organic matter. In oxygenated sediments, PO4 is closely bound to iron oxides; when those are reduced under anoxia, PO4 can be released from the sediments at high rates (Middelburg and Levin, 2009; Fulweiler et al., 2010). Repeated short periods of hypoxia (as is common in Narragansett Bay), while not completely eliminating the benthic fauna, can result in successively larger changes in nutrient cycling (Villnäs et al., 2012). Changes in these fluxes that affect primary production can have far reaching consequences for other ecosystem functions (Zhang et al., 2010).
Hypoxia can diminish the ability of benthic communities to provide the essential ecosystem function of regulating nitrogen (Carstensen et al., 2014). The sediment nitrogen cycle is complex and several processes are particularly sensitive to the sediment DO gradient. That the higher net flux of N2 gas from the hypoxic sediments of Narragansett Bay was not statistically different is not surprising (Fulweiler and Heiss, 2014). Both N-fixation and denitrification can occur at a given site and the net N2 flux depends on the balance between the two (Brown, 2013; Fulweiler et al., 2013; Ehrlich, 2014; Fulweiler and Heiss, 2014). In measurements from 2005 to 2013 in Narragansett Bay, Fulweiler and Heiss (2014) found a net positive flux of N2 gas out of the sediments in both hypoxic and normoxic areas. Denitrification rates were significantly higher in the hypoxic areas, while N-fixation rates were not significantly different. Denitrification removes reactive N and has an important role in lessening the effects of eutrophication. However, sediments with hypoxic or anoxic conditions also preserve the enzyme nitrogenase and make N-fixation possible (Fulweiler et al., 2013). High N-fixation rates were found in organically-enriched, severely hypoxic sediments of Greenwich Bay, which implies another positive feedback loop for hypoxia (Ehrlich, 2014).
Recovery of Benthic Ecosystem Functions and Future Prospects for Narragansett Bay
In recent years, improvements in Narragansett Bay WWTFs and CSOs have reduced nutrient inputs and organic loading, with the most dramatic reductions occurring between 2011 and 2014 when total nitrogen loads from 11 WWTFs impacting the upper Bay reached half of the 1995–1996 levels (NBC, 2014; RIDEM, 2015). Krumholz (2012) measured a 15–20% decrease in nitrogen concentrations in the Bay, between 2000 and 2010. How the Narragansett Bay ecosystem will respond when the planned upgrades to the WWTFs and CSOs are completed is a question of much interest (e.g., Nixon et al., 2008; Oviatt, 2008; Nixon et al., 2009), but the effect of this on hypoxia intensity, extent, and duration is not yet clear.
Results from other coastal areas in North America and Europe suggest that a return to lower levels of nutrients and higher levels of DO after reduction of nitrogen inputs can be achieved in many cases (Kemp et al., 2009; Riemann et al., 2015). However, a return to natural levels of DO and sediment TOC could take several years because long-term accumulation of organic matter may first have to be oxidized (Diaz and Rosenberg, 1995; Middelburg and Levin, 2009; Steckbauer et al., 2011). Further, even after a return to normal oxygen conditions, recovery of benthic communities may take many more years because it involves successional dynamics in which colonizing opportunistic species are eventually replaced by equilibrium species (Diaz et al., 2008; Middelburg and Levin, 2009). There may be only a partial recovery of the ecosystem and it may not return to exactly what it was before the input of excessive nutrients and organic matter (Rosenberg et al., 2002; Duarte et al., 2009; Zhang et al., 2010; Riemann et al., 2015). Meanwhile, there is still the question of how benthic communities in the currently normoxic areas of Narragansett Bay will respond to reduced nitrogen loading and the potential decline in organic matter input (Nixon et al., 2008, 2009).
Results shown in Table 2 should be considered initial estimates. Delineation of the hypoxic areas is not absolute, but was based on averages of yearly and seasonal fluctuations using data from several studies. Secondly, although we eliminated as factors sediment contaminants and toxicity (the other major stressors on benthic communities) and bottom water salinity, other unmeasured factors (such as bottom trawling for fish) may be important in local areas. It may be possible in the future, after more data have been gathered by the NCCA monitoring program, to better discriminate between anthropogenic and natural variation. Thirdly, for some of the ecosystem functions, data available from other studies were limited. Nevertheless, the estimates shown in Table 2 generally agree well with values from other studies in the literature. Results shown in Table 2 on the relationships between the stressors of eutrophication and hypoxia and the responses of benthic ecosystem functions can be used with models of alternate future scenarios of nitrogen loading and ecosystem responses (Rashleigh et al., 2015). Similar estuaries in the Virginian Biogeographic Province (Hale, 2010) also have recently experienced reduced nitrogen loading and may follow a similar trajectory of recovery. The results also provide a basis for further development of nutrient and DO critera.
Although our results—and results from studies of benthic community recovery elsewhere—suggest that a return to normoxic conditions will result in a substantial improvement in benthic community ecosystem functions in the hypoxic areas of Narragansett Bay, other factors are at play globally and regionally. Climate change will exacerbate biodiversity loss through ocean warming, acidification, anoxia, and changes in primary productivity (McCauley et al., 2015). Ocean and terrestrial warming can lead to changes in precipitation and river discharge, more land runoff, increased water column stratification, change in winds and circulation, reduced oxygen solubility, and increased respiratory rates, all of which can lead to more hypoxia (Diaz and Rosenberg, 2008; Zhang et al., 2010). Hypoxia in the U.S. and globally is forecast to worsen, with increased occurrence, frequency, intensity, and duration (CENR, 2010; Rabalais et al., 2010; Diaz and Rosenberg, 2011). For the northeast U.S., the climate change expectation is for warmer temperatures and more precipitation (Hayhoe et al., 2008), both of which can exacerbate hypoxia. These changes make local projects to reduce nitrogen input even more important.
Conclusions
A thin layer of life exists at the sediment surface in the world's oceans, comprised of a dynamic, linked system of an oxygenated surface layer and an underlying anoxic layer. This benthic system performs ecosystem functions and services that are essential for biogeochemical cycles and energy flows that support both the marine ecosystem and human well-being. When excess nutrients (primarily nitrogen) from human activities fuel eutrophication that feeds this layer with more organic carbon than the system can process—given the supply rate of oxygen—the boundary between the aerobic and anaerobic realms moves upwards to the sediment surface, leading to diminished biodiversity and benthic ecosystem functioning. In recent years, nitrogen loads to several estuaries in the northeastern U.S. have been reduced and nascent signs of recovery of benthic ecosystems have been observed in some areas. Our results offer an initial glimpse of what a benthic recovery in Narragansett Bay and similar estuaries may look like. If nitrogen inputs continue to decrease, benthic communities in the currently hypoxic areas of Narragansett Bay will gradually look more like those in the normoxic areas (less divergence of stations in the multidimensional scaling; Figure 3). As the organic load decreases, we expect that biodiversity, abundance, and biomass in the currently hypoxic areas will increase as they track backwards along the Pearson and Rosenberg (1978) general model (Supplementary Figure S1). We also predict, on the order of the estimates given in Table 2, more rare species, less dominance of total abundance by opportunistic species, more mature communities with larger animals living at greater depths, increased bioturbation that will lead to increased depth of the sediment oxidized layer, increased secondary production, and a more robust capacity for aerobic metabolism and nutrient recycling. However, other factors such as climate change make it difficult to predict the exact trajectory of recovery of ecosystem functions that will result from reduced nutrient loads. Well-designed monitoring programs—including measurements of benthic community functions, along with more direct measurements of ecosystem services—are required to gather the information needed to adjust management interventions for this changing world.
Author Contributions
SH, GC, and CD made substantial contributions to the conception of the work and analysis of the data; drafted and revised the work, gave final approval, and agree to be accountable for all aspects of the work.
Conflict of Interest Statement
The authors declare that the research was conducted in the absence of any commercial or financial relationships that could be construed as a potential conflict of interest.
Acknowledgments
We thank R. McKinney who provided unpublished benthic biomass data from a study of waterfowl feeding. C. Oviatt provided the MERL data and helpful information on Narragansett Bay benthos and hypoxia. Reviews by J. Grear, A. Oczkowski, B. Rashleigh, and the two peer reviewers improved the manuscript. This is contribution number ORD-015001 of the U.S. Environmental Protection Agency, Office of Research and Development, National Health and Environmental Effects Research Laboratory, Atlantic Ecology Division, Narragansett, RI. The research described in this article has been funded wholly or in part by the U.S. Environmental Protection Agency. This article has been reviewed by the EPA Office of Research and Development, and approved for publication. Mention of trade names or commercial products does not constitute endorsement or recommendation for use.
Supplementary Material
The Supplementary Material for this article can be found online at: http://journal.frontiersin.org/article/10.3389/fmars.2016.00249/full#supplementary-material
References
Altieri, A. H. (2008). Dead zones enhance key fisheries species by providing predation refuge. Ecology 89, 2808–2818. doi: 10.1890/07-0994.1
Altieri, A. H., and Witman, J. D. (2006). Local extinction of a foundation species in a hypoxic estuary: integrating individuals to ecosystem. Ecology 87, 717–730. doi: 10.1890/05-0226
Balvanera, P., Pfisterer, A. B., Buchmann, N., He, J. S., Nakashizuka, T., Raffaelli, D., et al. (2006). Quantifying the evidence for biodiversity effects on ecosystem functioning and services. Ecol. Lett. 9, 1146–1156. doi: 10.1111/j.1461-0248.2006.00963.x
BART [Bay Assessment Response Team]. (2016). Rhode Island Department of Environmental Management. Available online at: www.dem.ri.gov/programs/emergencyresponse/bart/netdata.php
Beatty, L. L. (1991). The Response of Benthic Suspension Feeders and their Grazing Impact on Phytoplankton in Eutrophied Coastal Ecosystems. dissertation, University of Rhode Island, Kingston, RI.
Bricker, S., Longstaff, B., Dennison, W., Jones, A., Boicourt, K., Wicks, C., et al. (2007). Effects of Nutrient Enrichment in the Nation's Estuaries: A Decade of Change. NOAA Coastal Ocean Program Decision Analysis Series No. 26. National Centers for Coastal Ocean Science, Silver Spring, MD.
Brown, S. M. (2013). Using Molecular Tools to Elucidate Controls on Microbes Driving the Nitrogen Cycle in Marine Sediments. dissertation, University of Rhode Island, Kingston, RI.
Calabretta, C. J., and Oviatt, C. A. (2008). The response of benthic macrofauna to anthropogenic stress in Narragansett Bay, Rhode Island: a review of human stressors and assessment of community conditions. Mar. Poll. Bull. 56, 1680–1695. doi: 10.1016/j.marpolbul.2008.07.012
Carstensen, J., Conley, D. J., Bonsdorff, E., Gustafsson, B. G., Hietanen, S., Janas, U., et al. (2014). Hypoxia in the Baltic Sea: biogeochemical cycles, benthic fauna, and management. Ambio 43, 26–36. doi: 10.1007/s13280-013-0474-7
CENR [Committee on Environment Natural Resources]. (2010). Scientific Assessment of Hypoxia in U.S. Coastal Waters. Interagency Working Group on Harmful Algal Blooms, Hypoxia, and Human Health of the Joint Subcommittee on Ocean Science and Technology. Washington, DC.
Chapin, F. S. III., Zavaleta, E. S., Eviner, V. T., Naylor, R. L., Vitousek, P. M., Reynolds, H. L., et al. (2000). Consequences of changing biodiversity. Nature 405, 234–242. doi: 10.1038/35012241
Chintala, M., Ayvazian, S. G., Boothman, W., Cicchetti, G., Coiro, L., Hale, A., et al. (2015). Trend Analysis of Stressors and Ecological Responses, Particularly Nutrients, in the Narragansett Bay Watershed. U.S. Environmental Protection Agency, Narragansett, RI.
Cicchetti, G., Latimer, J. S., Rego, S. A., Nelson, W. G., Bergen, B. J., and Coiro, L. L. (2006). Relationships between near-bottom dissolved oxygen and sediment profile camera measures. J. Mar. Syst. 62, 124–141. doi: 10.1016/j.jmarsys.2006.03.005
Clarke, K. R., Gorley, R. N., Somerfield, P. J., and Warwick, R. M. (2014). Change in Marine Communities: An Approach to Statistical Analysis and Interpretation, 3rd Edn. Devon: PRIMER-E Ltd, Ivybridge.
Codiga, D. L., Stoffel, H. E., Deacutis, C. F., Kiernan, S., and Oviatt, C. A. (2009). Narragansett Bay hypoxic event characteristics based on fixed-site monitoring network time series: intermittency, geographic distribution, spatial synchronicity, and interannual variability. Estuar. Coasts 32, 621–641. doi: 10.1007/s12237-009-9165-9
Covitch, A. P., Austen, M. C., Bärlocher, F., Chauvet, E., Cardinale, B. J., Biles, C. L., et al. (2004). The role of biodiversity in the functioning of freshwater and marine benthic ecosystems. Bioscience 54, 767–775. doi: 10.1641/0006-3568(2004)054[0767:TROBIT]2.0.CO;2
Deacutis, C. F. (2008). “Evidence of ecological impacts from excess nutrients in upper Narragansett Bay,” in Science for Ecosystem-Based Management: Narragansett Bay in the 21st Century, eds A. Desbonnet and B. A. Costa-Pierce (New York, NY: Springer), 349–381.
Deacutis, C. F., Murray, D., Prell, W., Saarman, E., and Korhun, L. (2006). Hypoxia in the upper half of Narragansett, RI, during August 2001 and 2002, in Natural and Anthropogenic Influences on the Mount Hope Bay Ecosystem. Northeastern Nat. 13(Special Issue 4), 173–198. doi: 10.1656/1092-6194(2006)13[173:HITUHO]2.0.CO;2
Diaz, R. J., and Rosenberg, R. (1995). Marine benthic hypoxia: a review of its ecological effects and the behavioural responses of benthic macrofauna. Oceanogr. Mar. Biol Ann. Rev. 33, 245–303.
Diaz, R. J., and Rosenberg, R. (2008). Spreading dead zones and consequences for marine ecosystems. Science 321, 926–929. doi: 10.1126/science.1156401
Diaz, R. J., and Rosenberg, R. (2011). Introduction to environmental and economic consequences of hypoxia. Int. J. Wat. Res. Devel. 27, 71–82. doi: 10.1080/07900627.2010.531379
Diaz, R. J., Rhoads, D. C., Blake, J. A., Kropp, R. K., and Keay, K. E. (2008). Long-term trends of benthic habitats related to reduction in wastewater discharge to Boston Harbor. Estuar. Coasts 31, 1184–1197. doi: 10.1007/s12237-008-9094-z
Dolbeth, M., Cusson, M., Sousa, R., and Pardal, M. A. (2012). Secondary production as a tool for better understanding of aquatic ecosystems. Can. J. Fish. Aquat. Res. 69, 1230–1253. doi: 10.1139/f2012-050
Duarte, C. M., Conley, D. J., Carstensen, J., and Sánchez-Camacho, M. (2009). Return to Neverland: shifting baselines affect eutrophication restoration targets. Estuar. Coasts 32, 29–36. doi: 10.1007/s12237-008-9111-2
Edgar, G. J. (1990). The use of the size structure of benthic macrofaunal communities to estimate faunal biomass and secondary production. J. Exp. Mar. Biol. Ecol. 137(3), 195–214. doi: 10.1016/0022-0981(90)90185-F
Ehrlich, A. L. (2014). Elucidating the Molecular Response of Microbial Nitrogen Fixation in Estuarine Sediments to Hypoxia. master's thesis, University of Rhode Island, Kingston, RI.
Elderfield, H., Luedtke, N., McCaffrey, R. J., and Bender, M. (1981). Benthic flux studies in Narrgansett Bay. Am. J. Sci. 281, 768–787. doi: 10.2475/ajs.281.6.768
Fenchel, T., and Finlay, B. J. (1995). Ecology and Evolution in Anoxic Worlds. Oxford: Oxford University Press.
Fulweiler, R. W. (2007). The Impact of Climate Change on Benthic-Pelagic Coupling and the Biogeochemical Cycling of Narragansett Bay, R.I. dissertation, University of Rhode Island, Kingston, RI.
Fulweiler, R. W., and Heiss, R. W. (2014). (Nearly) A decade of directly measured sediment N2 fluxes – what can Narragansett Bay tell us about the global ocean nitrogen budget? Oceanography 27, 184–195. doi: 10.5670/oceanog.2014.22
Fulweiler, R. W., and Nixon, S. W. (2012). Net sediment N2 fluxes in a southern New England estuary – variations in space and time. Biogeochemistry 111, 111–124. doi: 10.1007/s10533-011-9660-5
Fulweiler, R. W., Brown, S. M., Nixon, S. W., and Jenkins, B. D. (2013). Evidence and conceptual model for the co-occurrence of nitrogen fixation and denitrification in heterotrophic marine sediments. Mar. Ecol. Prog. Ser. 482, 57–68. doi: 10.3354/meps10240
Fulweiler, R. W., Nixon, S. W., and Buckley, B. A. (2010). Spatial and temporal variability of benthic oxygen demand and nutrient regeneration in an anthropogenically impacted New England estuary. Estuar. Coasts 33:1377–1390. doi: 10.1007/s12237-009-9260-y
Fulweiler, R. W., Nixon, S. W., Buckley, B. A., and Granger, S. L. (2007). Reversal of the net nitrogen flux in coastal marine sediments. Nature 448, 180–182. doi: 10.1038/nature05963
Gogina, M., Darr, A., and Zettler, M. L. (2014). Approach to assess consequences of hypoxia disturbance events for benthic ecosystem functioning. J. Mar. Syst. 129:203–213. doi: 10.1016/j.jmarsys.2013.06.001
Goulletquer, P., Gros, P., Boeuf, G., and Weber, J. (2014). Biodiversity in the Marine Environment. Springer: Cham
Gray, J. S., Wu, R. S., and Or, Y. Y. (2002). Effects of hypoxia and organic enrichment on the coastal marine environment. Mar. Ecol. Prog. Ser. 238, 249–279. doi: 10.3354/meps238249
Hale, S. S. (2010). Biogeographical patterns of marine benthic macroinvertebrates along the Atlantic coast of the northeastern USA. Estuar. Coasts 33, 1039–1053. doi: 10.1007/s12237-010-9332-z
Hale, S. S., and Heltshe, J. F. (2008). Signals from the benthos: development and evaluation of a benthic index for the nearshore Gulf of Maine. Ecol. Indic. 8, 338–350. doi: 10.1016/j.ecolind.2007.04.004
Hayhoe, K., Wake, C., Anderson, B., Liang, X.-Z., Maurer, E., Zhu, J., et al. (2008). Regional climate change projections for the Northeast USA. Mitig. Adapt. Strat. Glob. Change 13, 425–436. doi: 10.1007/s11027-007-9133-2
Hyland, J., Balthis, L., Karakassis, I., Magni, P., Petrov, A., Shine, J., et al. (2005). Organic carbon content of sediments as an indicator of stress in the marine benthos. Mar. Ecol. Prog. Ser. 295, 91–103. doi: 10.3354/meps295091
Insomniacs Day Trippers. (2016). Surveys of Narragansett Bay Dissolved Oxygen. Available online at: http://www.geo.brown.edu/georesearch/insomniacs
Kemp, W. M., Testa, J. M., Conley, D. J., Gilbert, D., and Hagy, J. D. (2009). Temporal responses of coastal hypoxia to nutrient loading and physical controls. Biogeosciences 6, 2985–3008. doi: 10.5194/bg-6-2985-2009
Krahforst, C., and Carullo, M. (2008). “An ecosystem-based perspective of Mount Hope Bay” in Science for Ecosystem-Based Management: Narragansett Bay in the 21st Century, eds A. Desbonnet and B. A. Costa-Pierce (New York, NY: Springer), 383–417.
Krumholz, J. S. (2012). Spatial and Temporal Patterns in Nutrient Standing Stock and Mass-Balance in Response to Load Reductions in a Temperate Estuary. dissertation, University of Rhode Island, Kingston, RI.
Levin, L. A., Boesch, D. F., Covich, A., Dahm, C., Erseus, C., Ewel, K. C., et al. (2001). The function of marine critical transition zones and the importance of sediment biodiversity. Ecosystems 4, 430–451. doi: 10.1007/s10021-001-0021-4
Levin, L. A., Ekau, W., Gooday, A. J., Jorissen, F., Middelburg, J. J., Naqvi, S. W. A., et al. (2009). Effects of natural and human-induced hypoxia on coastal benthos. Biogeosciences, 6, 2063–2098. doi: 10.5194/bg-6-2063-2009
Levinton, J. (1995). “Bioturbators as ecosystem engineers: Control of the sediment fabric, inter-individual interactions, and material fluxes,” in Linking Species and Ecosystems, eds C. G. Jones and J. H. Lawton (New York, NY: Chapman and Hall), 29–36.
McCaffrey, R. J., Myers, A. C., Davey, E., Morrison, G., Bender, M., Luedtke, N., et al. (1980). The relation between pore water chemistry and benthic fluxes of nutrients and manganeses in Narragansett Bay, Rhode Island. Limnol. Oceanogr. 25, 31–44. doi: 10.4319/lo.1980.25.1.0031
McCauley, D. J., Pinsky, M. L., Palumbi, S. R., Estes, J. A., Joyce, F. H., and Warner, R. R. (2015). Marine defaunation: animal loss in the global ocean. Science 347, 1255641-1–1255641-7. doi: 10.1126/science.1255641
McKinney, R. A., Glatt, S. M., and McWilliams, S. R. (2004). Allometric length-weight relationships for benthic prey of aquatic wildlife in coastal marine habitats. Wildlife Biol. 10, 241–249.
Melrose, D. C., Oviatt, C. A., and Berman, M. S. (2007). Hypoxic events in Narragansett Bay, Rhode Island, during the summer of 2001. Estuar. Coasts 30, 47–53. doi: 10.1007/BF02782966
MERL [Marine Ecosystems Research Laboratory]. (2016). Available online at: http://www.gso.uri.edu/merl/merl.html
Middelburg, J. J., and Levin, L. A. (2009). Coastal hypoxia and sediment biogeochemistry. Biogeosciences 6, 1273–1293. doi: 10.5194/bg-6-1273-2009
Miller, D. C., Poucher, S. L., and Coiro, L. (2002). Determination of lethal dissolved oxygen levels for selected marine and estuarine fishes, crustaceans, and a bivalve. Mar. Biol. 140, 287–296. doi: 10.1007/s002270100702
Montagna, P. A., and Froeschke, J. (2009). Long-term biological effects of coastal hypoxia in Corpus Christi Bay, Texas, USA. J. Exp. Mar. Biol. Ecol. 381, S21–S30. doi: 10.1016/j.jembe.2009.07.006
Murray, D. W., Prell, W. L., Rincon, C. E., and Saarman, E. (2007). Physical Property and Chemical Characteristics of Surface Grab Samples from Narragansett Bay and the Providence and Seekonk Rivers, a Summary of the Brown University Narragansett Bay Sediment Project (BUNBSP). Narragansett Bay Estuary Program, NBEP-2007-127, Providence, RI.
NBC [Narragansett Bay Commission]. (2014). Five Years and 5 Billion Gallons: Evaluating the Narragansett Bay Commission CSA Abatement Project. Report, Providence, RI.
NCA [National Coastal Assessment]. (2016). U.S. Environmental Protection Agency. Available online at: https://archive.epa.gov/emap/archive-emap/web/html/index-78.html
NCCA [National Coastal Condition Assessment]. (2016). Office of Water, U.S. Environmental Protection Agency, Washington, DC. Available online at: https://www.epa.gov/national-aquatic-resource-surveys/data-national-aquatic-resource-surveys
Nilsson, H. C., and Rosenberg, R. (2000). Succession in marine benthic habitats and fauna in response to oxygen deficiency: analysed by sediment profile-imaging and by grab samples. Mar. Ecol. Prog. Ser. 197, 139–148. doi: 10.3354/meps197139
Nixon, S. W., Buckley, B. A., Granger, S. L., Harris, L. A., Oczkowski, A. J., Fulweiler, R. W., et al. (2008). “Nitrogen and phosphorus inputs to Narragansett Bay: Past, present, and future,” in Science for Ecosystem-Based Management: Narragansett Bay in the 21st Century, eds A. Desbonnet and B. A. Costa-Pierce (New York, NY: Springer), 101–175.
Nixon, S. W., Fulweiler, R. W., Buckley, B. A., Granger, S. L., Nowicki, B. L., and Henry, K. M. (2009). The impact of changing climate on phenology, productivity, and benthic-pelagic coupling in Narragansett Bay. Estuar. Coast. Shelf Sci. 82, 1–18. doi: 10.1016/j.ecss.2008.12.016
Nixon, S. W., Kelly, J. R., Furnas, B. N., Oviatt, C. A., and Hale, S. S. (1980). “Phosphorus regeneration and the metabolism of coastal marine bottom communities” in Marine Benthic Dynamics, eds K. R. Tenore and B. C. Coull (Columbia, SC: University of South Carolina Press), 219–242.
Nixon, S. W., Oviatt, C. A., and Hale, S. S. (1976). “Nitrogen regeneration and the metabolism of coastal marine bottom communities,” in The Role of Terrestrial and Aquatic Organisms in Decomposition Processes, eds J. M. Anderson and A. Macfadyen (Oxford: Blackwell), 269–283.
Norkko, A., Villnäs, A., Norkko, J., Valanko, S., and Pilditch, C. (2013). Size matters: implications of the loss of large individuals for ecosystem function. Sci. Rep. 3:2646. doi: 10.1038/srep02646
Norling, K., Rosenberg, R., Hulth, S., Grémare, A., and Bonsdorff, E. (2007). Importance of functional biodiversity and species-specific traits of benthic fauna for ecosystem functions in marine sediment. Mar. Ecol. Prog. Ser. 332, 11–23. doi: 10.3354/meps332011
NRC [National Research Council]. (1995). Understanding Marine Biodiversity. Washington, DC: National Academy Press.
Oviatt, C. A. (2008). “Impacts of nutrients on Narragansett Bay productivity: A gradient approach,” in Science for Ecosystem-Based Management: Narragansett Bay in the 21st Century, eds A. Desbonnet and B. A. Costa-Pierce (New York, NY: Springer), 523–543.
Pearson, T. H., and Rosenberg, R. (1978). Macrobenthic succession in relation to organic enrichment and pollution of the marine environment. Oceanogr. Mar. Biol. Ann. Rev. 16, 229–311.
Pilson, M. E. Q. (1985). On the residence time of water in Narragansett Bay. Estuaries 8, 2–14. doi: 10.2307/1352116
Prell, W. L., Murray, D. W., and Howell, P. (2015). Reanalysis of the Narragansett Bay Spatial Survey (Day Trippers) Water Column Data for Temperature, Salinity, Density, and Dissolved Oxygen (2005 to 2013). Narragansett Bay Estuary Program, MBEP201501.
Prell, W., Murray, D., and Deacutis, C. (2006). Summer-Season Survey of Dissolved Oxygen in Upper Narragansett Bay Beginning in 2005. Available online at: http://www.geo.brown.edu/georesearch/insomniacs
Prell, W., Saarman, E., Murray, D., and Deacutis, C. (2004). Summer-Season Nighttime Surveys of Dissolved Oxygen in Upper Narragansett Bay (1999-2003). Available online at: http://www.geo.brown.edu/georesearch/insomniacs
Rabalais, N. N., Diaz, R. J., Levin, L. A., Turner, R. E., Gilbert, D., and Zhang, J. (2010). Dynamics and distribution of natural and human-caused hypoxia. Biogeosciences 7, 585–619. doi: 10.5194/bg-7-585-2010
Rakocinski, C. F. (2012). Evaluating macrobenthic process indicators in relation to organic enrichment and hypoxia. Ecol. Indic. 13, 1–12. doi: 10.1016/j.ecolind.2011.04.031
Rashleigh, B., Walker, H., Gleason, T., Abdelrhman, M., Charlestra, L., Dettmann, E., et al. (2015). Quantitative Models Describing Past and Current Nutrient Fluxes and Associated Ecosystem Level Responses in the Narragansett Bay Ecosystem. U.S. Environmental Protection Agency, Narragansett, RI
RIDEM [Rhode Island Department of Environmental Management]. (2003). The Greenwich Bay Fish Kill–August 2003: Causes, Impacts and Responses. Rhode Island Department of Environmental Management, Providence, RI. Available online at: http://www.dem.ri.gov/pubs/fishkill.pdf
RIDEM [Rhode Island Department of Environmental Management]. (2015). Estimated Nitrogen Loads to Upper Narragansett Bay (early 2000s versus 2014): Adapting Nixon et al. (1995, 2008) Bay-Wide Estimates to the Upper Bay. RIDEM, Office of Water Resources, Providence, RI.
Riemann, B., Carstensen, J., Dahl, K., Fossing, H., Hansen, J. W., Jakogbsen, H. H., et al. (2015). Recovery of Danish coastal systems after reductions in nutrient loading: a holistic approach. Estuar. Coasts 39, 82–97. doi: 10.1007/s12237-015-9980-0
Ritter, C., and Montagna, P. A. (1999). Seasonal hypoxia and models of benthic response in a Texas Bay. Estuaries 22, 7–20. doi: 10.2307/1352922
Rosenberg, R., Agrenius, S., Hellman, B., Nilsson, H. C., and Norling, K. (2002). Recovery of marine benthic habitats and fauna in a Swedish fjord following improved oxygen conditions. Mar. Ecol. Prog. Ser. 234, 43–53. doi: 10.3354/meps234043
Rosenberg, R., Magnusson, M., and Nilsson, H. C. (2009). Temporal and spatial changes in marine benthic habitats in relation to the EU Water Framework Directive: the use of sediment profile imagery. Mar. Poll. Bull. 58, 565–572. doi: 10.1016/j.marpolbul.2008.11.023
Rosenberg, R., Nilsson, H. C., and Diaz, R. J. (2001). Response of benthic fauna and changing sediment redox profiles over a hypoxic gradient. Estuar. Coast. Shelf Sci. 53, 343–350. doi: 10.1006/ecss.2001.0810
Rudnick, D. T. (1984). Seasonality of Community Structure and Carbon Flow in Narragansett Bay Sediments. dissertation, University of Rhode Island, Kingston, RI.
Saarman, E., Prell, W. L., Murray, D. W., and Deacutis, C. F. (2008). “Summer bottom water dissolved oxygen in upper Narragansett Bay,” in Science for Ecosystem-Based Management: Narragansett Bay in the 21st Century, eds A. Desbonnet and B. A. Costa-Pierce (New York, NY: Springer), 325–347.
Schult, M. (2010). An Exploration of Factors Influencing Benthic Macrofauna (> 2.0 mm) Assemblage Patterns within Narragansett Bay. master's thesis, University of Rhode Island, Kingston, RI.
Seitz, R. D., Dauer, D. M., Llanso, R. J., and Long, W. C. (2009). Broad-scale effects of hypoxia on benthic community structure in Chesapeake Bay. J. Exp. Mar. Biol. Ecol. 381, S4–S12. doi: 10.1016/j.jembe.2009.07.004
Snelgrove, P. V. R. (1998). The biodiversity of macrofaunal organisms in marine sediments. Biodivers. Conserv. 7, 1123–1132. doi: 10.1023/A:1008867313340
Snelgrove, P. V. R., Blackburn, T. H., Hutchings, P., Alongi, D., Grassle, J. F., Hummel, H., et al. (1997). The importance of marine biodiversity in ecosystem processes. Ambio 26, 578–583.
Solan, M., Cardinale, B. J., Downing, A. L., Engelhardt, K. A. M., Ruesink, J. L., and Srivastava, D. S. (2004). Extinction and ecosystem function in the marine benthos. (2004). Science 306, 1177–1180. doi: 10.1126/science.1103960
Solan, M., Raffaelli, D. J., Paterson, D. M., White, P. C. L., and Pierce, J. G. (2006). Marine biodiversity and ecosystem function: empirical approaches and future research needs. Mar. Ecol. Prog. Ser. 311, 275–178. doi: 10.3354/meps311175
Steckbauer, A., Duarte, C. M., Carstensen, J., Vaquer-Sunyer, R., and Conley, D. J. (2011). Ecosystem impacts of hypoxia: thresholds of hypoxia and pathways to recovery. Environ. Res. Lett. 6, 1–12. doi: 10.1088/1748-9326/6/2/025003
Sturdivant, S. K., Diaz, R. J., Llanso, R., and Dauer, D. M. (2014). Relationship between hypoxia and macrobenthic production in Chesapeake Bay. Estuar. Coasts 37, 1219–1232. doi: 10.1007/s12237-013-9763-4
USEPA. (2000). Ambient Aquatic Life Water Quality Criteria for Dissolved Oxygen (saltwater): Cape Cod to Cape Hatteras. EPA-822-R-00-012. U.S. Environmental Protection Agency, Office of Water, Washington, DC, and Office of Research and Development, Narragansett, RI, USA.
USEPA. (2012). National Coastal Condition Report IV. EPA-842-R-10-003. U.S. Environmental Protection Agency, Office of Research and Development/Office of Water, Washington, DC, USA.
Valente, R. M., Rhoads, D. C., Germano, J. D., and Cabelli, V. J. (1992). Mapping of benthic enrichment patterns in Narragansett Bay, Rhode Island. Estuaries 15, 1–17. doi: 10.2307/1352705
Vaquer-Sunyer, R., and Duarte, C. M. (2008). Thresholds of hypoxia for marine biodiversity. Proc. Nat. Acad. Sci.U.S.A. 105, 15452–15457. doi: 10.1073/pnas.0803833105
Villnäs, A., Norkko, J., Lukkari, K., Hewitt, J., and Norkko, A. (2012). Consequences of increasing hypoxic disturbance on benthic communities and ecosystem functioning. PLOS ONE 7:e44920. doi: 10.1371/journal.pone.0044920
Waldbusser, G. G., Marinelli, R. L., Whitlatch, R. B., and Visscher, P. T. (2004). The effects of infaunal biodiversity on biogeochemistry of coastal marine sediments. Limnol. Oceanogr. 49, 1482–1492. doi: 10.4319/lo.2004.49.5.1482
Weslawski, J. M., Snelgrove, P. V. R., Levin, L. A., Austen, M. C., Kneib, R. T., Illiffe, T. M., et al. (2004). “Marine sedimentary biota as providers of ecosystem goods and services,” in Sustaining Biodiversity and Ecosystem Services in Soils and Sediments, ed D. H. Wall (Washington, DC: Island Press), 73–98.
Worm, B., Barbier, E. B., Beaumont, N., Duffy, J. E., Folke, C., Halpern, B. S., et al. (2006). Impacts of biodiversity loss on ocean ecosystem services. Science 314, 787–790. doi: 10.1126/science.1132294
Wu, R. S. (2002). Hypoxia: from molecular responses to ecosystem responses. Mar. Poll. Bull. 45, 35–45. doi: 10.1016/S0025-326X(02)00061-9
Keywords: marine benthic communities, biodiversity, ecosystem functions, eutrophication, hypoxia, Narragansett Bay, Virginian Biogeographic Province
Citation: Hale SS, Cicchetti G and Deacutis CF (2016) Eutrophication and Hypoxia Diminish Ecosystem Functions of Benthic Communities in a New England Estuary. Front. Mar. Sci. 3:249. doi: 10.3389/fmars.2016.00249
Received: 12 August 2016; Accepted: 15 November 2016;
Published: 29 November 2016.
Edited by:
Christos Dimitrios Arvanitidis, Hellenic Centre for Marine Research, GreeceReviewed by:
Jan Warzocha, National Marine Fisheries Research Institute in Gdynia, PolandJan Marcin Weslawski, Institute of Oceanology (PAN), Poland
Copyright © 2016 Hale, Cicchetti and Deacutis. This is an open-access article distributed under the terms of the Creative Commons Attribution License (CC BY). The use, distribution or reproduction in other forums is permitted, provided the original author(s) or licensor are credited and that the original publication in this journal is cited, in accordance with accepted academic practice. No use, distribution or reproduction is permitted which does not comply with these terms.
*Correspondence: Stephen S. Hale, aGFsZS5zdGVwaGVuQGVwYS5nb3Y=