- 1The Leon H. Charney School of Marine Sciences, University of Haifa, Mount Carmel, Haifa, Israel
- 2Department of Evolution, Systematics and Ecology, Hebrew University of Jerusalem, Jerusalem, Israel
- 3Department of Natural Sciences, City University of New York, Baruch College and The Graduate Center, New York, NY, USA
- 4Department of Plant and Environmental Sciences, Hebrew University of Jerusalem, Jerusalem, Israel
- 5Interuniversity Institute for Marine Sciences, Eilat, Israel
Photosynthetic coral reef structures extend from the shallow sundrenched waters to the dimly lit, “twilight” mesophotic depths. For their resident endosymbiotic dinoflagellates, primarily from the genus Symbiodinium spp., this represents a photic environment that varies ~15-fold in intensity and also differs in spectral composition. We examined photosynthesis in the scleractinian coral Stylophora pistillata in shallow (3 m) and mesophotic settings (65 m) in the northern Red Sea. Symbiodinium spp. in corals originating from the mesophotic environment consistently performed below their photosynthetic compensation point and also exhibited distinct light harvesting antenna organization. In addition, the non-photochemical quenching activity of Symbiodinium spp. from mesophotic corals was shown to be considerably lower than those found in shallow corals, showing they have fewer defenses to high-light settings. Over a period of almost 4 years, we extensively utilized closed circuit Trimix rebreather diving to perform the study. Phylogenetic analysis showed that shallow corals (3 m) transplanted to a deep reef environment (65 m) maintained their initial Symbiodinium spp. community (clade A), rather than taking on deep low-light clades (clade C), demonstrating that shallow S. pistillata acclimate to low-light mesophotic environments while maintaining their shallow photosynthetic traits. Mesophotic corals exhibited static depth-related chlorophyll content per cell, a decrease in PSI activity and enhanced sigmoidal fluorescence rise kinetics. The sigmoidal fluorescence rise kinetics we observed in mesophotic corals is an indication of energy transfer between photosynthetic units. We postulate that at mesophotic depths, a community of adapted Symbiodinium spp. utilize a unique adaptation to lower light conditions by shifting their light harvesting to a PSII based system, where PSII is structured near PSI, with additional PCP soluble antenna also trapping light that is funneled to the PSI reaction center. In this study, we provide evidence that mesophotic Symbiodinium spp. have developed novel adaptive low-light characteristics consisting of a cooperative system for excitation energy transfer between photosynthetic units that maximizes light utilization.
Introduction
Scleractinian corals build the framework of the world's tropical reefs and their growth relies primarily on photosynthetic dinoflagellate endosymbionts from the genus Symbiodinium (Jones et al., 1998). These photosymbionts reside within the corals endoderm and produce photosynthates that are translocated to the host (Muscatine et al., 1975; Falkowski and Dubinsky, 1981). While shallow reefs have been extensively studied, the deeper mesophotic environment (>30 m) has been much less examined due to difficulty to access, such as the need for technical diving or remotely operated vehicles. Light intensity decays exponentially with depth (Gordon, 1989) and therefore, the mesophotic reef environment, known as the “twilight zone,” is characterized by light fluxes that vary seasonally between 5 and 100 μmol photons m−2 s−1. This dim mesophotic setting, therefore, receives 15–300 times less of a photon flux than shallow reef counterparts ~1500 μmol photons m−2 s−1 (Levy et al., 2003). The low light flux in the mesophotic region impacts corals photosynthetic and calcification rates and limits their vertical distribution throughout the photic zone (Wells, 1957; Dubinsky et al., 1984; Franklin et al., 1996; Jones et al., 1998). In addition, deeper reefs receive narrower spectra, primarily blue light spectra centered at 467 nm, than the wider spectral range of shallow waters (Dishon et al., 2013). In mesophotic, low-light conditions, corals have been shown to shift their energetic budget to heterotrophic predation (Muscatine and Kaplan, 1994; Einbinder et al., 2009). Symbiodinium has also been shown to acclimate to low-light regimes (Cohen and Dubinsky, 2015). The current understanding is that two mechanisms are involved: (1) an increase in the effective antenna size—antenna pigments per photosynthetic unit (PSU) (Falkowski and Dubinsky, 1981; Franklin et al., 1996) and (2) an increase of photosynthetic units (PSU) per cell (Chang et al., 1983; Prézelin, 1987; Iglesias-Prieto and Trench, 1997; Pratchett et al., 2008; Hennige et al., 2009; Hutchings et al., 2009; Lesser et al., 2010). However, there have also been studies that have reported no significant changes in the Symbiodinium chlorophyll content at depths below 25 m (Titlyanov et al., 2002; Mass et al., 2007; Lesser et al., 2010).
In shallow high light conditions thermal dissipation, Non photochemical Quenching (NPQ) provides a mechanism that protects Symbiodinium photosynthetic apparatus from excess photosynthetic active radiation (PAR) damage (Hoegh-Guldberg and Jones, 1999). Dinoflagellates contain two types of photosynthetic antenna: A membrane bound intrinsic antenna–acpPC (Chl a-Chl c2-peridinin protein complex) as shown in Figure 1 (Niedzwiedzki et al., 2014); and a soluble membrane complex PCP (peridinin-Chl a protein). NPQ was shown to be associated with the conversion of diadinoxanthin to diatoxanthin (Brown, 1997). Xanthophyll epoxidation and de-epoxidation decreases photon flux to PSII reaction centers and thus prevents over reduction of the quinone acceptors by reducing the functional absorption cross-section of PSII reaction centers (σPSII). In corals, the quencher in the NPQ processes was suggested to reside in the apcCP antenna (Iglesias-Prieto and Trench, 1997; Kanazawa et al., 2014).
NPQ has a finite capacity and may reach saturation while excess electrons continue to reduce the electron transport chain (Brown et al., 1999; Dustan, 1999; Smith et al., 2005). Alternative sinks for electrons, other than CO2 assimilation, may provide additional protection mechanisms to help prevent over reduction at high light intensities. PSI can directly reduce O2 via the Mehler reaction to produce superoxide radicals that are rapidly converted within the chloroplast to hydrogen peroxide by superoxide dismutase (Jones et al., 1998). Hydrogen peroxide is then detoxified to produce water by ascorbate peroxidase, which oxidizes ascorbate to monodehydroascorbate (Asada, 1999).
While there is a wealth of information on short-term processes of light regime change acclimation mechanisms, long-term processes accounting for the diversity of both symbionts and hosts have only recently been explored (Iglesias-Prieto et al., 2004; Bongaerts et al., 2011, 2013; Cooper et al., 2011; van Oppen et al., 2011; Brazeau et al., 2013; Nir et al., 2014). Most indicate distinct deep and shallow symbionts types, with a few generalist types (Lesser et al., 2010).
In this study, we examined Stylophora pistillata at 65 and 3 m, as well as those slowly transplanted from shallow to deep, in an attempt to elucidate what long-term acclimation or adaptation processes photosynthetic dinoflagellates possess in high and low light environments. We are also investigated potential mechanisms of efficient energy transfer at mesophotic depths.
Materials and Methods
In order to investigate the photosynthetic characteristics of mesophotic and shallow corals we conducted a series of analyses on intact corals and their isolated Symbiodinium and determined their net and gross photosynthesis rates as a function of irradiance (PI), PSII quantum yield, non-photochemical quenching (NPQ) parameters, chlorophyll fluorescence emission spectra and PSI activity.
Sample Collection and Preparation for Analysis
Coral samples were collected in the Northern Red Sea (29⋅30′N, 34⋅56′E) from a gradient of 3 to 65 m along the fore reef slope. Branches of the hermatypic corals S. pistillata (wide depth range, 0–70 m) (Fricke and Schuhmacher, 1983) were collected from their natural habitats using closed circuit Trimix rebreather systems (Megalodon™). To distinguish between adaptive or acclimation responses, over almost 4 years (June 2004-April 2008), we gradually transferred (5 m/2 weeks) mesophotic S. pistillata from a depth of 65 to 5 m, and shallow S. pistillata from 5 m to a final depth of 65 m. The corals then remained at either depth until the conclusion of the experiment when they were removed for analysis. Samples were collected in light-proof black bags and brought to the incubation area where light intensity was controlled to duplicate source depths. The collection was conducted under permit number 31944 from the Israel Nature and Parks Authority. Samples were held for less than 18 h, and then transferred for further analysis.
Fluorescence Parameters (PSII)
Chlorophyll fluorescence parameters were measured using a Fluorescence Induction and Relaxation (FIRe) fluorometer (Satlantic) and a PAM imaging system fluorometer (Walz, GmbH, Effeltrich, Germany). The corals were dark-adapted for 30 min prior to measurements. A single saturating pulse (4000 μmol photons m−2 s−1, white-light, 500 ms for the PAM and 10,000 μmol photons m−2 s−1, blue-light, 80 μs for the FIRe) was triggered, and PSII maximal fluorescence (Fm) was measured.
NPQ was calculated as described in Schreiber (2004) where NPQ = (Fm − )/. was obtained after steady state fluorescence was achieved under an actinic light intensity of (0–1000 μmol photons m−2 s−1 for the PAM and a 1000 μmol photons m−2 s−1 (Schott KL2500LCD) for the FIRe measurement.
Oxygen Evolution and Consumption (Net and Gross Photosynthesis)
A quadrupole mass spectrometer (Balzers QMG 421) with a membrane inlet (MIMS) was used to measure dissolved gas in a climate and light controlled chaimber (3.3 ml volume). Simultaneous measurements of argon and mass 14 concentration were used to assess the stability of the system as described in Tchernov et al. (1997, 2001). The action medium consisted of artificial sea water (ASW) pH 8.2 with 2 mM total dissolved inorganic carbon.
PSI Relative Function through Oxygen Consumption
Fresh symbionts were extracted from coral fragments and placed in the action medium that consisted of ASW pH 8.2 with 2 mM total dissolved inorganic carbon. The algae were incubated in the dark in the presence of 10–5 M (3-(3,4-dichlorophenyl)-1,1-dimethylurea) DCMU (D2425 Sigma) for 20 min. When there was no detectable oxygen evolution under illumination at saturating light intensity (400 μmol photons m−2 s−1) lights were turned off and 30 μM (sodium 2,6-dichloroindophenol) DCIP, (33125 Sigma), 0.3 mM sodium ascorbate (A7631 Sigma), and 70 μM methyl viologen (856177 Sigma) were added (Kyle et al., 1984). The FIZ were transferred into a temperature controlled (26⋅C ± 0.5) MIMS chamber. Upon illumination, oxygen was monitored on line using the MIMS as described in Tchernov et al. (1997, 2001). The rate of oxygen consumption was normalized to chlorophyll concentration.
Pigments Analysis
Coral fragments (2 cm) were airbrushed and purified using the same protocol as Stochaj and Grossman (1997) and resuspended in 100% methanol. After ensuring identical samples (chlorophyll concentration), we followed the protocol of Rogers and Marcovich (2007) for pigment HPLC analysis. The samples were incubated in 4⋅C for 2 h and were vortexed for 2 min every 30 min. The samples were incubated overnight in the presence of ammonium acetate (pH 7.2, −20⋅C). The mixture was vortexed again and centrifuged (14,000 rpm, 4⋅C, 20 min). The pellet was discarded and the supernatant was injected into a HPLC equipped with a reverse-phased column (Spherisorb ODS-2 column -silica 5 μm, 3.2 mm × 250 mm (Phenomenex®) analysis.
Photosynthesis/Irradiance Measurements
Coral fragments were airbrushed to release the Symbiodinium symbionts, cleaned via triple washing with 0.2 μm filtered seawater and then placed in a temperature-controlled chamber (2.8 ml) at 26⋅C in artificial seawater (pH 8.2) supplemented with 2 mM dissolved inorganic carbon (S5761 Sigma). For illumination, Schott© KL2500LCD unit with two optic fibers were connected to special optic ports to the chamber. The various chemicals supplied to the cell suspension were introduced via a top injection port. In order to measure respiration during light photosynthesis, isotopic labeling oxygen method incorporated as in Bender et al. (1987). The sample was spiked with O, incubated in light, and measured for labeled oxygen dilution. Detection was performed by a Membrane Inlet Mass Spectrometry (MIMS) apparatus. As all the labeled O2 is contained in a single well-defined dissolved gas phase and the ambient O2 pool assumed to be much larger, only a negligible amount of O2 will be recycled by respiration during the incubation. For normalization, chlorophyll was extracted using 90% acetone, as in Jeffrey and Humphrey (1975). PSI activity values were calculated from the initial slopes.
Phylogenetic Analysis
Phylogenetic analysis of the ITS2 sequences (316 bp) using parsimony (PAUP 4) algorithm carried out with 26 representative sequences of seven clades and three sequences obtained from this study (deep, shallow and transplant, Supplementary Table 1). The ITS2 sequence of Gymnodinium aureolum was used as an out-group. Values (%) of 500 bootstrap replicates are given at branching points for parsimony/distance analyses. Corals from shallow water and transplant present zooxanthellae from clade A while the symbiont of the deep-water cluster with clade c.
Results
Dynamics of the Photosynthetic Light Response
For the study of the dynamics of the photosynthetic apparatus we used four groups of corals: shallow and mesophotic coral fragments and two depth transplants groups (3 and 65 m, Figure 2). NPQ activity was measured in S. pistillata (3 and 65 m), and was up to five times higher in shallow corals than in the mesophotic corals (Figure 3 and Table 1).
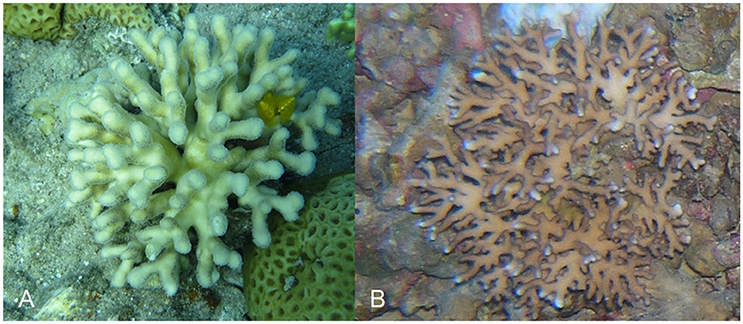
Figure 2. Example of Stylophora pistillata from shallow and mesophotic environments. (A) 3 m; (B) 65 m.
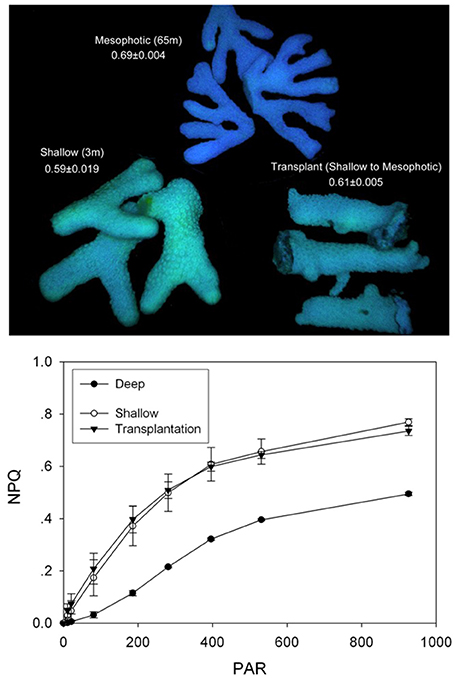
Figure 3. NPQ analysis of mesophotic, shallow and transplanted corals. Measurements were performed using PAM imaging system. The top panel presents a false color image of the coral fragments. The values indicated are Fv/Fm average calculated from three independent fragments. The bottom panel presents NPQ values as a function of incident light intensity for the three coral fragment groups. Mesophotic corals symbionts lose a small fraction of excessive absorbed energy to photoprotective measures.
The transfer of 65 m mesophotic Stylophora colonies to shallow 5 m environments resulted in lethal responses, despite a slow assent rate (5 m every 2 weeks). This is in agreement with (Baker, 2003) that indicates mortality of deeper colonies upon ascending transplant experiments. Yet, the transfer of shallow corals to the mesophotic region was successful. For shallow corals transplanted to 65 m, a marked difference in NPQ from the mesophotic corals was observed, indicating a shallow-type response (Figure 3). Phylogenetic sampling showed that transplanted corals retain their original Symbiodinium type. This differed from the non-transplanted corals as well as from control corals that were transplanted laterally along the same depth (Supplementary Figure 1). The different symbiont population discovered at mesophotic depths hints that it diverged from shallow population to provide an adaptive advantage. These results prompted us to take a closer mechanistic look at the differences in the function of the photosynthetic apparatus.
Organization of the Photosynthetic Apparatus
The rates of oxygen production and uptake reactions were studied in detail using the Membrane Inlet Mass-Spectrometry technique (MIMS). Using MIMS determined that S. pistillata from 65 m performed below their photosynthetic compensation point (where oxygen uptake and production rates are equal) at the natural ambient low-light intensities (Figure 4). Shallow corals performed close to their photosynthetic Pmax in their ambient high-light conditions. The Pmax of mesophotic corals from 65 m was ~1.6-fold lower than that of their shallow counterparts (Table 1), a finding in agreement with (Falkowski and Dubinsky, 1981). Mesophotic corals did not exhibit a decrease in oxygen production when subjected to high-light intensities of nearly two orders of magnitude higher than their natural light environment (Figure 4). The relatively low Pmax is in accordance with the prevalent shade-adapted response in photosynthetic organisms. Mesophotic corals displayed a low initial rate constant (α), comparable to shallow corals (Table 1). This differs from the typical response of shade-adapted species that exhibit lower Pmax at low-light intensities and retain high photosynthetic efficiency (α), due to an increased antenna size (Björkman, 1966).
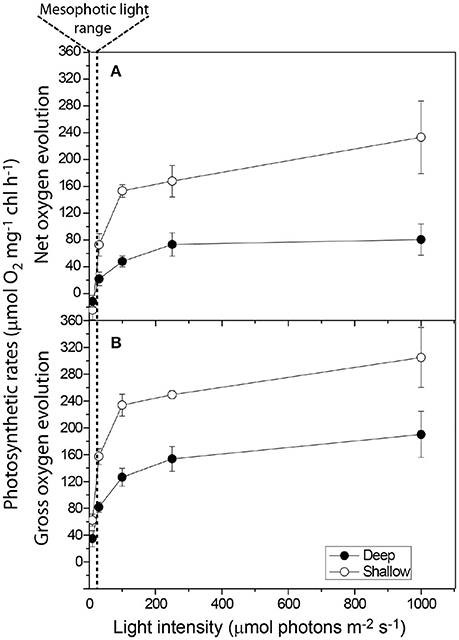
Figure 4. Photosynthesis-Irradiance measurements of the initial mesophotic (65 m) and shallow (3 m) corals Symbiodinium sp. depicting (A) Net Photosynthesis and (B) Gross photosynthesis. Mesophotic symbionts do not experience dynamic photoinhibition at shallow light intensities.
Using the Fluorescence Induction and Relaxation (FIRe) approach (Kolber et al., 1998), we found that mesophotic PSII traps energy better than shallow PSII; and its variable fluorescence magnitude is higher than the shallow symbionts (Table 1). However, mesophotic QA-reoxidation time constant (τ) was slightly slower than the shallow PSU constant (Table 1,) and the absorption cross-section (σP680) was significantly lower (Table 1), implying an overall smaller PSU size.
The photosynthetic pigments concentration ratios were obtained via High Performance Liquid Chromatography (HPLC) (Table 1). Mesophotic Symbiodinium contain substantially less antenna pigments on a chlorophyll basis. The percent decrease was greater for Chlorophyll C then for Peridinin (34 and 18%, respectively). This data suggests a shift to smaller antenna size in mesophotic corals, with a composition favoring membrane anchored PCP over membrane embedded apcCP. Partial electron transport measurements of freshly isolated Symbiodinium (FIZ) were performed using a membrane inlet mass-spectrometer (MIMS). A ratio of 2.7 ± 0.7 in PSI activity was recorded between shallow and mesophotic samples (Table 1), suggesting reduced PSI content in mesophotic samples.
Energy Transfer between Antenna and Reaction Centres
Fluorescence rise kinetics in the presence of DCMU was measured to investigate the dynamics of PSII and antenna energy transfer processes. In the presence of DCMU, blocking electron transport at the QB site, fluorescence emission kinetics reflects the interplay of antenna excitation migration and subsequent PSII charge separation. Fluorescence rise kinetics of deep corals was strongly sigmoidal as compared to the shallow corals (Figure 5). The sigmoidal nature of the mesophotic corals fluorescence kinetics extended their rise time compared to shallow corals, the difference being most pronounced under low light intensities (Figure 5).
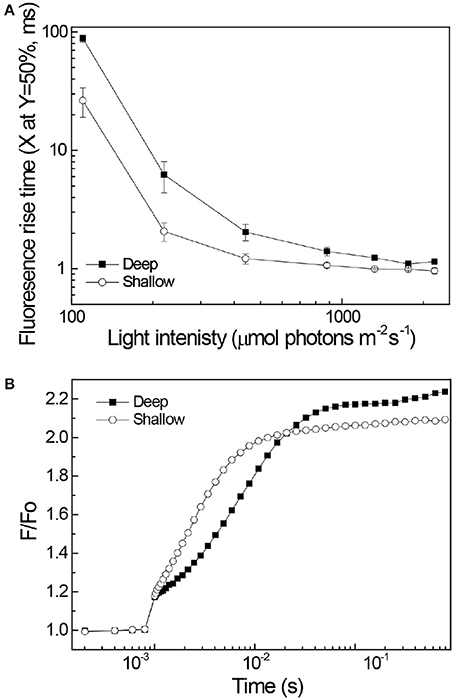
Figure 5. Chlorophyll fluorescence rise kinetics. The actinic light was turned on at 10−3 s. (A) Shallow corals (3 m) are represented by open symbols, mesophotic corals (65 m) depth are represented by closed symbols. Curves fitted to a sigmoid trandline (R2 > 0.97 for all curves). The fluorescence rise half time, extracted from the sigmoidal fit, for deep and shallow corals is plotted as a function of the actinic light intensity. Standard deviation values calculated from three samples for each depth. Values represent the time for the signal to reach 50% of its maximum excitation. (B) An example for the raw data where 220 μmole photons m−2 s−1 red light excitation (620 nm) was used.
Discussion
The combination of static depth-related chlorophyll content per cell (Mass et al., 2007) with a distinct decrease in PSI activity, and enhanced sigmoidal fluorescence rise kinetics suggests a distinct organization of the photosynthetic apparatus of mesophotic corals (Figure 6). In the photosynthetic apparatus, PSI supports the linear electron transport process leading to the generation of ATP and NADPH and cyclic electron transport processes affecting ATP/NADPH ratios. Cyclic processes are considered as means for adjusting the redox poise and as a protective mechanism preventing excess excitation induced damages (Munekage et al., 2004; Nawrocki et al., 2015). At low light intensities such protection is not required.
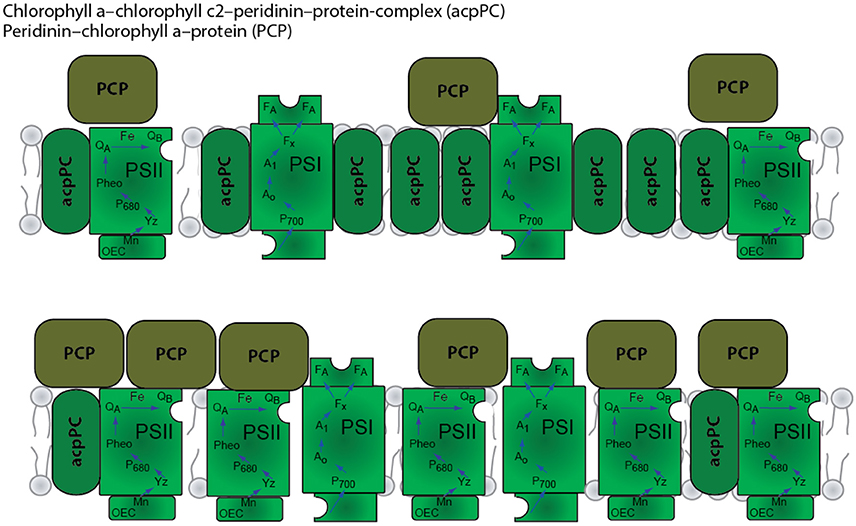
Figure 6. Schematic model of the organization of the photosynthetic apparatus in shallow (top) and mesophotic/deep (bottom) corals.
The theoretical limit (Falkowski and Dubinsky, 1981) for enhancing light absorption through an increase in the effective antenna cross section was calculated to be 1650 ± 175 Chl a per P700 (Falkowski and Dubinsky, 1981) at a depth equivalent to 30 m (where light intensity is equated to the light intensity at the depth the coral was extracted). This mechanism, therefore, cannot be efficient in the deeper mesophotic reef environment. An increase in PSU per cell may be effective for low-light acclimation, as described by several groups (Chang et al., 1983; Prézelin, 1987; Iglesias-Prieto and Trench, 1997; Hutchings et al., 2009). In this study, as well as in previous work (Mass et al., 2007) evidence of an acclimation pathway mechanism was not found at mesophotic depths.
PSII's shallow energetic trap allows exactions to be distributed between closed and open reaction centers. This creates an efficient low-light energy transfer scheme that is displayed in our data as a sigmoid fluorescence rise kinetics curve, shown only in the deep corals. The sigmoidal fluorescence rise kinetics that we observed in mesophotic corals is an indication of energy transfer between photosynthetic units (Trissl et al., 1993; Lavergne and Trissl, 1995). Under low-light intensities, this energy transfer increases photosynthetic quantum efficiency (Joliot and Joliot, 1964, 2003). The lower PSI content and the shift from a membrane embedded to a membrane-attached light harvesting system can facilitate this type of cooperativity. We postulate that at mesophotic depths, a community of adapted Symbiodinium spp. occupies most of the corals and they utilize a unique adaptation to the “twilight” conditions by shifting their light harvesting to a PSII based system, where PSII is structured near PSI, with additional PCP soluble antenna also trapping light that is funneled to the PSI reaction center. Our genetic data suggest that mesophotic S. pistillata have one species of symbionts that maintain these traits. Shallow corals and those transplanted from shallow to deep kept their shallow traits, such as high NPQ that serves as a line of defense against photoinhibitory damage (Papageorgiou and Govindjee, 1968; Takahashi et al., 2008). These finding points to an adaptive mechanism in mesophotic corals, rather than the previously noted acclimation strategies. Other species of mesophotic corals and other photosynthetic organisms living in perpetually low-light environments should be examined to determine if they also share this novel, energy efficient low-light photosynthesis strategy.
Author Contributions
Planned the study: SE, DG, ES, OL, NK, and DT. Performed Pigments Analysis: SE, ES, OL, NK, and DT. Performed Photosynthesis/Irradiance Measurements: SE, ES, OL, NK, and DT. Wrote the manuscript: SE, DG, ES, OL, NK, and DT.
Conflict of Interest Statement
The authors declare that the research was conducted in the absence of any commercial or financial relationships that could be construed as a potential conflict of interest.
The reviewer GE declared a shared affiliation, though no other collaboration, with one of the authors OL to the handling Editor, who ensured that the process nevertheless met the standards of a fair and objective review.
Acknowledgments
We thank K. Zendbank for assisting in molecular work and the Interuniversity Institute for Marine Sciences and staff in Eilat, Israel, for contributing to field studies. We greatly thank E. Brokovitch and O. Ben-Sharput for participating in the technical dives. The research was supported by the Israel Science Foundation grant # 981/05 to DT, the Bundesministerium für Bildung und Forschung, Germany, grant # 1923–1300 to DT and the US National Science Foundation grant # 0920572 and 1556213 to DG.
Supplementary Material
The Supplementary Material for this article can be found online at: http://journal.frontiersin.org/article/10.3389/fmars.2016.00195
References
Asada, K. (1999). The water-water cycle in chloroplasts: scavenging of active oxygens and dissipation of excess photons. Annu. Rev. Plant Physiol. Plant Mol. Biol. 50, 601–639. doi: 10.1146/annurev.arplant.50.1.601
Baker, A. C. (2003). Flexibility and specificity in coral-algal symbiosis: diversity, ecology, and biogeography of symbiodinium. Annu. Rev. Ecol. Evol. Syst. 34, 661–689. doi: 10.1146/annurev.ecolsys.34.011802.132417
Bender, M., Grande, K., Johnson, K., Marra, J., Williams, P., Sieburth, J., et al. (1987). A comparison of four methods for determining planktonic community production. Limnol. Oceanogr. 32, 1085–1098. doi: 10.4319/lo.1987.32.5.1085
Björkman, O. (1966). The effect of oxygen concentration on photosynthesis in higher plants. Physiol. Plant. 19, 618–633. doi: 10.1111/j.1399-3054.1966.tb07046.x
Bongaerts, P., Frade, P. R., Ogier, J. J., Hay, K. B., van Bleijswijk, J., Englebert, N., et al. (2013). Sharing the slope: depth partitioning of agariciid corals and associated Symbiodinium across shallow and mesophotic habitats (2-60 m) on a Caribbean reef. BMC Evol. Biol. 13:205. doi: 10.1186/1471-2148-13-205
Bongaerts, P., Sampayo, E. M., Bridge, T. C. L., Ridgway, T., Vermeulen, F., Englebert, N., et al. (2011). Symbiodinium diversity in mesophotic coral communities on the Great Barrier Reef: a first assessment. Mar. Ecol. Prog. Ser. 439, 117–126. doi: 10.3354/meps09315
Brazeau, D. A., Lesser, M. P., and Slattery, M. (2013). Genetic structure in the coral, Montastraea cavernosa: assessing genetic differentiation among and within Mesophotic reefs. PLoS ONE 8:e65845. doi: 10.1371/journal.pone.0065845
Brown, B. E. (1997). Coral bleaching: causes and consequences. Coral Reefs 16, S129–S138. doi: 10.1007/s003380050249
Brown, B. E., Ambarsari, I., Warner, M. E., Fitt, W. K., Dunne, R. P., Gibb, S. W., et al. (1999). Diurnal changes in photochemical efficiency and xanthophyll concentrations in shallow water reef corals : evidence for photoinhibition and photoprotection. Coral Reefs 18, 99–105. doi: 10.1007/s003380050163
Chang, S. S., Prézelin, B. B., and Trench, R. K. (1983). Mechanisms of photoadaptation in three strains of the symbiotic dinoflagellate Symbiodinium microadriaticum. Mar. Biol. 76, 219–229. doi: 10.1007/BF00393021
Cohen, I., and Dubinsky, Z. (2015). Long term photoacclimation responses of the coral Stylophora pistillata to reciprocal deep to shallow transplantation: photosynthesis and calcification. Front. Mar. Sci. 2:45. doi: 10.3389/fmars.2015.00045
Cooper, T. F., Ulstrup, K. E., Dandan, S. S., Heyward, A. J., Kühl, M., Muirhead, A., et al. (2011). Niche specialization of reef-building corals in the mesophotic zone: metabolic trade-offs between divergent Symbiodinium types. Proc. Biol. Sci. 278, 1840–1850. doi: 10.1098/rspb.2010.2321
Dishon, G., Dubinsky, Z., Fine, M., and Iluz, D. (2013). Underwater light field patterns in subtropical coastal waters: a case study from the Gulf of Eilat (Aqaba). Isr. J. Plant Sci. 60, 265–275. doi: 10.1560/IJPS.60.1-2.265
Dubinsky, Z., Falkowski, P. G., Porter, J. W., and Muscatine, L. (1984). Absorption and utilization of radiant energy by light- and shade-adapted colonies of the hermatypic coral Stylophora pistillata. Proc. R. Soc. Lond. Ser. B Biol. Sci. 222, 203–214. doi: 10.1098/rspb.1984.0059
Dustan, P. (1999). Distribution of zooxanthellae and photosynthetic chloroplast pigments of the reef-building coral montastrea annularis ellis and solander in relation to depth on a West Indian Coral Reef. Bull. Mar. Sci. 29, 79–95.
Einbinder, S., Mass, T., Brokovich, E., Dubinsky, Z., Erez, J., and Tchernov, D. (2009). Changes in morphology and diet of the coral Stylophora pistillata along a depth gradient. Mar. Ecol. Prog. Ser. 381, 167–174. doi: 10.3354/meps07908
Falkowski, P. G., and Dubinsky, Z. (1981). Light-shade adaptation of Stylophora pistillata, a hermatypic coral from the Gulf of Eilat. Nature 289, 172–174. doi: 10.1038/289172a0
Franklin, L. A., Seaton, G. G. R., Lovelock, C. E., and Larkum, A. W. D. (1996). Photoinhibition of photosynthesis on a coral reef. Plant Cell Environ. 19, 825–836. doi: 10.1111/j.1365-3040.1996.tb00419.x
Fricke, H. W., and Schuhmacher, H. (1983). The depth limits of red sea stony corals: an ecophysiological problem (a deep diving survey by submersible). Mar. Ecol. 4, 163–194. doi: 10.1111/j.1439-0485.1983.tb00294.x
Gordon, H. R. (1989). Can the Lambert-Beer law be applied to the diffuse attenuation coefficient of ocean water? Limnol. Oceanogr. 34, 1389–1409. doi: 10.4319/lo.1989.34.8.1389
Hennige, S., Suggett, D., Warner, M., McDougall, K., and Smith, D. (2009). Photobiology of symbiodinium revisited: bio-physical and bio-optical signatures. Coral Reefs 28, 179–195. doi: 10.1007/s00338-008-0444-x
Hoegh-Guldberg, O., and Jones, R. (1999). Photoinhibition and photoprotection in symbiotic dinoflagellates from reef-building corals. Mar. Ecol. Prog. Ser. 183, 73–86. doi: 10.3354/meps183073
Hutchings, P., Kingsford, M. J., and Hoegh-Guldberg, O. (eds.). (2009). The Great Barrier Reef: Biology, Environment and Management, 1st Edn. Collingwood, VIC: Springer Netherlands.
Iglesias-Prieto, R., Beltrán, V. H., LaJeunesse, T. C., Reyes-Bonilla, H., and Thomé, P. E. (2004). Different algal symbionts explain the vertical distribution of dominant reef corals in the eastern Pacific. Proc. Biol. Sci. 271, 1757–1763. doi: 10.1098/rspb.2004.2757
Iglesias-Prieto, R., and Trench, R. K. (1997). Acclimation and adaptation to irradiance in symbiotic dinoflagellates. II. Response of chlorophyll-protein complexes to different photon-flux densities. Mar. Biol. 130, 23–33. doi: 10.1007/s002270050221
Jeffrey, S. W., and Humphrey, G. F. (1975). New spectrophotometric equations for determining chlorophylls a, b, c1 and c2 in higher plants, algae and natural phytoplankton. Biochem. Physiol. Pflanz. 167, 191–194.
Joliot, A., and Joliot, P. (1964). Etude cinétique de la réaction photochimique libérant l'oxygène au cours de la photosynthèse. C.R. Acad. Sci. Paris 258, 4622–4625.
Joliot, P., and Joliot, A. (2003). Excitation transfer between photosynthetic units: the 1964 experiment. Photosynth. Res. 76, 241–245. doi: 10.1023/A:1024908829819
Jones, R. J., Hoegh-Guldberg, O., Larkum, A. W. D., and Schreiber, U. (1998). Temperature-induced bleaching of corals begins with impairment of the CO2 fixation mechanism in zooxanthellae. Plant Cell Environ. 21, 1219–1230. doi: 10.1046/j.1365-3040.1998.00345.x
Kanazawa, A., Blanchard, G. J., Szabó, M., Ralph, P. J., and Kramer, D. M. (2014). The site of regulation of light capture in Symbiodinium: does the peridinin-chlorophyll a-protein detach to regulate light capture? Biochim. Biophys. Acta 1837, 1227–1234. doi: 10.1016/j.bbabio.2014.03.019
Kolber, Z. S., Prášil, O., and Falkowski, P. G. (1998). Measurements of variable chlorophyll fluorescence using fast repetition rate techniques: defining methodology and experimental protocols. Biochim. Biophys. Acta 1367, 88–106.
Kyle, D. J., Ohad, I., and Arntzen, C. J. (1984). Membrane protein damage and repair: selective loss of a quinone-protein function in chloroplast membranes. Proc. Natl. Acad. Sci. U.S.A. 81, 4070–4074.
Lavergne, J., and Trissl, H. W. (1995). Theory of fluorescence induction in photosystem II: derivation of analytical expressions in a model including exciton-radical-pair equilibrium and restricted energy transfer between photosynthetic units. Biophys. J. 68, 2474–2492. doi: 10.1016/S0006-3495(95)80429-7
Lesser, M. P., Slattery, M., Stat, M., Ojimi, M., Gates, R. D., and Grottoli, A. (2010). Photoacclimatization by the coral Montastraea cavernosa in the mesophotic zone: light, food, and genetics. Ecology 91, 990–1003. doi: 10.1890/09-0313.1
Levy, O., Dubinsky, Z., and Achituv, Y. (2003). Photobehavior of stony corals: responses to light spectra and intensity. J. Exp. Biol. 206, 4041–4049. doi: 10.1242/jeb.00622
Mass, T., Einbinder, S., Brokovich, E., Shashar, N., Vago, R., Erez, J., et al. (2007). Photoacclimation of Stylophora pistillata to light extremes: metabolism and calcification. Mar. Ecol. Prog. Ser. 334, 93–102. doi: 10.3354/meps334093
Munekage, Y., Hashimoto, M., Miyake, C., Tomizawa, K., Endo, T., Tasaka, M., et al. (2004). Cyclic electron flow around photosystem I is essential for photosynthesis. Nature 429, 579–582. doi: 10.1038/nature02598
Muscatine, L., and Kaplan, I. R. (1994). Resource partitioning by reef corals as determined from stable isotope composition II. 15N of zooxanthellae and animal tissue versus depth. Pacific Sci. 48, 304–312.
Muscatine, L., Pool, R. R., and Trench, R. K. (1975). Symbiosis of algae and invertebrates: aspects of the symbiont surface and the host-symbiont interface. Trans. Am. Microsc. Soc. 94, 450–469. doi: 10.2307/3225518
Nawrocki, W. J., Tourasse, N. J., Taly, A., Rappaport, F., and Wollman, F.-A. (2015). The plastid terminal oxidase: its elusive function points to multiple contributions to plastid physiology. Annu. Rev. Plant Biol. 66, 49–74. doi: 10.1146/annurev-arplant-043014-114744
Niedzwiedzki, D. M., Jiang, J., Lo, C. S., and Blankenship, R. E. (2014). Spectroscopic properties of the Chlorophyll a-Chlorophyll c 2-Peridinin-Protein-Complex (acpPC) from the coral symbiotic dinoflagellate Symbiodinium. Photosynth. Res. 120, 125–139. doi: 10.1007/s11120-013-9794-5
Nir, O., Gruber, D. F., Shemesh, E., Glasser, E., and Tchernov, D. (2014). Seasonal mesophotic coral bleaching of Stylophora pistillata in the Northern Red Sea. PLoS ONE 9:e84968. doi: 10.1371/journal.pone.0084968
Papageorgiou, G., and Govindjee (1968). Light-induced changes in the fluorescence yield of chlorophyll a in vivo. II. Chlorella pyrenoidosa. Biophys. J. 8, 1316–1328. doi: 10.1016/S0006-3495(68)86558-0
Pratchett, M. S., Munday, P. L., Wilson, S. K., Graham, N. A. J., Cinner, J. E., Bellwood, D. R., et al. (2008). Effects of climate-induced coral bleaching on coral-reef fishes - ecological and economic consequences. Oceanogr. Mar. Biol. Annu. Rev. 46, 251–296. doi: 10.1201/9781420065756.ch6
Prézelin, B. (1987). “Photosynthetic physiology of dinoflagellates,” in The Biology of dinoflagellates, ed F. J. R. Taylor (Oxford: Blackwell Scientific), 174–223.
Rogers, J. E., and Marcovich, D. (2007). A simple method for the extraction and quantification of photopigments from Symbiodinium spp. J. Exp. Mar. Biol. Ecol. 353, 191–197. doi: 10.1016/j.jembe.2007.08.022
Schreiber, U. (2004). “Pulse-Amplitude-Modulation (PAM) fluorometry and saturation pulse method: an overview,” in Chlorophyll a Fluorescence: A Signature of Photosynthesis, eds G. Papageorgiou and Govindjee (Dordrecht: Springer), 279–319.
Smith, D. J., Suggett, D. J., and Baker, N. R. (2005). Is photoinhibition of zooxanthellae photosynthesis the primary cause of thermal bleaching in corals? Glob. Chang. Biol. 11, 1–11. doi: 10.1111/j.1529-8817.2003.00895.x
Stochaj, W. R., and Grossman, A. R. (1997). Differences in the protein profiles of cultured and endosymbiotic Symbiodinium sp. (Pyrrophyta) from the anemone Aiptasia pallida (Anthozoa). J. Phycol. 33, 44–53. doi: 10.1111/j.0022-3646.1997.00044.x
Takahashi, S., Whitney, S., Itoh, S., Maruyama, T., and Badger, M. (2008). Heat stress causes inhibition of the de novo synthesis of antenna proteins and photobleaching in cultured Symbiodinium. Proc. Natl. Acad. Sci. U.S.A. 105, 4203–4208. doi: 10.1073/pnas.0708554105
Tchernov, D., Hassidim, M., Luz, B., Sukenik, A., Reinhold, L., and Kaplan, A. (1997). Sustained net CO2 evolution during photosynthesis by marine microorganism. Curr. Biol. 7, 723–728. doi: 10.1016/S0960-9822(06)00330-7
Tchernov, D., Helman, Y., Keren, N., Luz, B., Ohad, I., Reinhold, L., et al. (2001). Passive entry of CO2 and its energy-dependent intracellular conversion to HCO3– in cyanobacteria are driven by a photosystem I-generated ΔμH+. J. Biol. Chem. 276, 23450–23455. doi: 10.1074/jbc.M101973200
Titlyanov, E., Titlyanova, T., and Yamazato, K. (2002). Acclimation of symbiotic reef-building corals to extremely low light. Symbiosis 33, 125–143.
Trissl, H. W., Gao, Y., and Wulf, K. (1993). Theoretical fluorescence induction curves derived from coupled differential equations describing the primary photochemistry of photosystem II by an exciton-radical pair equilibrium. Biophys. J. 64, 974–988. doi: 10.1016/S0006-3495(93)81463-2
van Oppen, M. J. H., Bongaerts, P., Underwood, J. N., Peplow, L. M., and Cooper, T. F. (2011). The role of deep reefs in shallow reef recovery: an assessment of vertical connectivity in a brooding coral from west and east Australia. Mol. Ecol. 20, 1647–1660. doi: 10.1111/j.1365-294X.2011.05050.x
Keywords: Symbiodinium spp., mesophotic coral reef, photosynthesis, low-light acclimation, excitation energy transfer
Citation: Einbinder S, Gruber DF, Salomon E, Liran O, Keren N and Tchernov D (2016) Novel Adaptive Photosynthetic Characteristics of Mesophotic Symbiotic Microalgae within the Reef-Building Coral, Stylophora pistillata. Front. Mar. Sci. 3:195. doi: 10.3389/fmars.2016.00195
Received: 01 July 2016; Accepted: 26 September 2016;
Published: 17 October 2016.
Edited by:
Daniel Wangpraseurt, University of Copenhagen, DenmarkReviewed by:
Susana Enríquez, National Autonomous University of Mexico, SpainLeïla Ezzat, Scientific Centre of Monaco, Monaco
Gal Eyal, Tel Aviv University and The Interuniversity Institute for Marine Sciences in Eilat, Israel
Copyright © 2016 Einbinder, Gruber, Salomon, Liran, Keren and Tchernov. This is an open-access article distributed under the terms of the Creative Commons Attribution License (CC BY). The use, distribution or reproduction in other forums is permitted, provided the original author(s) or licensor are credited and that the original publication in this journal is cited, in accordance with accepted academic practice. No use, distribution or reproduction is permitted which does not comply with these terms.
*Correspondence: Dan Tchernov, ZHRjaGVybm92QHVuaXYuaGFpZmEuYWMuaWw=