- 1Department of Environmental Sciences, Macquarie University, North Ryde, NSW, Australia
- 2Ocean and Earth Science, National Oceanography Centre, University of Southampton, Southampton, UK
- 3Plentziako itsas Estazioa, University of the Basque Country (PiE-UPV/EHU), Plentzia-Bizkaia, Spain
- 4School of Civil, Environmental and Mining Engineering, University of Western Australia, Crawley, WA, Australia
- 5Damara WA, Innaloo, WA, Australia
- 6Geoscience Division, Secretariat of the Pacific Community, Suva, Fiji
- 7Department of Applied Geology, Curtin University, Bentley, WA, Australia
- 8The UWA Oceans Institute and School of Plant Biology, University of Western Australia, Crawley, WA, Australia
- 9RPS MetOcean, Jolimont, WA, Australia
- 10School of Earth and Environment, University of Western Australia, Crawley, WA, Australia
- 11Centre of Offshore Foundation Systems, University of Western Australia, Crawley, WA, Australia
“Packaging” coastal sediment transport into discrete temporal and spatial scale bands is necessary for measurement programs, modeling, and design. However, determining how to best measure and parameterize information, to transfer between scales, is not trivial. An overview is provided of the major complexities in transferring information on coastal sediment transport between scales. Key considerations that recur in the literature include: interaction between sediment transport and morphology; the influence of biota; episodic sediment transport; and recovery time-scales. The influence of bedforms and landforms, as well as sediment–biota interactions, varies with spatio-temporal scale. In some situations, episodic sediment dynamics is the main contributor to long-term sediment transport. Such events can also significantly alter biogeochemical and ecological processes, which interact with sediments. The impact of such episodic events is fundamentally influenced by recovery time-scales, which vary spatially. For the various approaches to scaling (e.g., bottom-up, aggregation, spatial hierarchies), there is a need for fundamental research on the assumptions inherent in each approach.
Introduction
In all areas of theoretical and applied science and engineering, processes are described over a spectrum of temporal and spatial scales, to provide focus within the spatio-temporal framework of available data. This is reflected in hierarchical governance entities and coastal stakeholder groups, which influence at different (overlapping) scales (Swaney et al., 2012). How to transfer knowledge between discrete scale bands is a recurring issue (Church, 1996; Capobianco et al., 1998; Gracia et al., 2005; Roelvink, 2006). Landscapes are characterized by different properties at each scale of observation (Slaymaker et al., 2009). Each scale includes: (a) cumulative effects of lower levels and (b) emergent properties. Although emergent properties cannot be disregarded, large-scale sediment dynamics must, fundamentally, be cumulative of smaller scales (Cowell et al., 1995; Nicholls et al., 2014). However, data from smaller scales typically cannot be summed to describe larger-scale-dynamics. Measured and modeled data provide only a proxy for sediment transport, due to dependence on factors such as sampling technique, resolution, and range. Engineering and management activities often require spatial and temporal data extrapolation, to project lives of many decades. In addition, sediment dynamics governs, and is governed by, interactions between sediment, biota, physical, and chemical processes. As a result, chemical and biological processes are subject to unique spatio-temporal scales, not related purely to physical grain-interactions (Mann and Lazier, 2013). This contribution provides an overview of the key complexities in transferring information on coastal sediment dynamics, between spatial and temporal scales.
Spectrum of Scales
Morphological changes are cumulative of smaller scales, and depend upon the magnitude and frequency of drivers; thus, systematic understanding of coastal change must be organized by scale (Nicholls et al., 2014). Larson and Kraus (1995) described “compatible space-time scales,” within which feasible calculations can be undertaken (Figure 1). It is generally assumed that small and large spatial-scale changes are due to short- and long-term processes, respectively (Stive et al., 1991; Larson and Kraus, 1994; List et al., 2006). Larger-scale processes are used to provide boundary conditions (Stive et al., 2002), and smaller-scale processes are considered to have negligible influence at the larger scale (Stive et al., 1991). However, it can be difficult and expensive to measure large-scale (tens of kilometers or more) coastal response, to short-term (days) events (List et al., 2006). Therefore, there are relatively few observations investigating how information of coastal sediment transport relates between scales. Moreover, most scale descriptions focus upon natural (autonomous) variability, in the absence of human and engineering intervention (Stive et al., 2002). “Packaging” coastal processes into discrete scale bands is a convenient approach to analysis (Woodroffe, 2003); however, determining how to best measure and parameterize information to transfer between discrete scales is not trivial.
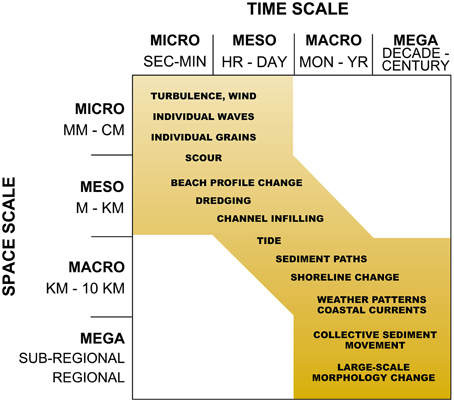
Figure 1. Compatible temporal and spatial scales for sediment transport and beach morphology. White spaces indicate combined space-time scales considered to be incompatible, or unfeasible (redrawn from Larson and Kraus, 1995, with permission from Elsevier). There are various similar diagrams of this type in the literature.
Considerations in Scale Transfer
Key issues in transferring information on coastal sediment transport, between scales, are considered here, including interaction between sediment transport and morphology; the influence of biota; episodic sediment transport; and recovery time-scales.
Influence of Morphology on Sediment Transport
Landforms and bedforms have important feedbacks with sediment transport. However, such features are not measured if the resolution or range is too low. Holman (2001) presented “classes” (scales) of nearshore morphology, using the alongshore wave number (inverse wavelength) as the length scale, with frequency as the time-scale. Time-scales varied from several wave periods (ripples), to decades, over some six orders of magnitude. However, because the complex and non-linear nearshore physical processes are still not clearly understood, morphological changes leading to the range of shapes, and features at different scales is almost impossible to predict (Caballeria et al., 2002). Bedforms have been deduced as being due to forcing by regular structures in the hydrodynamics, in so-called template models (van Enckevort et al., 2004). Whilst this approach has been able to predict shapes and initial longshore spacing in some cases, it failed in extensive field tests (Holland and Holman, 1996; Masselink and Pattiaratchi, 1998). Other approaches use linear and non-linear self-organization models (Blondeaux, 1990; Hulscher et al., 1993; Calvete et al., 2001; Caballeria et al., 2002). This allows for positive feedback between the hydrodynamics and morphology; this can lead to bedforms that do not correspond directly to scaling in the hydrodynamics.
Eulerian-Lagrangian frameworks may provide a useful analog for variation in sediment transport due to hydrodynamic forcing, with scale. Evaluation of sediment dynamics near to grain-scale is analogous to an Eulerian perspective. The Lagrangian analog at larger scales occurs with reference to spatio-temporal organization, developed by hydrodynamics, sediment transport, and morphology. From grain-scale upward, this organization may be characteristic of bedforms and landforms. This distinction reflects partly the approach of different disciplines to coastal sediment dynamics. For example, the grain-scale (Eulerian analog) is used more commonly by a sediment dynamicist, compared to a geomorphologist, who may take a landform approach (Lagrangian analog).
Sediment–biota Interactions
The approaches outlined above focus on the physical nature of sediments, which actually should be conceived as the integration of physical, chemical, and biological processes. To illustrate this point, we discuss two examples of the influence of: (1) seagrass and (2) capitellid worms, on sediment transport.
Sediment transport in complex benthic ecosystems, such as seagrass meadows, is difficult to describe, either qualitatively or quantitatively. This is partly due to the multiple scales of transport (Figure 1). Four such scales in seagrass meadows are:
(1) The stem scale (~O; cm): Significant erosion can occur around individual seagrass stems, similar to around engineered structures (Figure 2A). This has tremendous implications for the stability of individual shoots in seagrass recruitment or transplanting.
(2) The canopy scale (LC, ~O; 0.1–1 m): This represents the scale of turbulence generated at the top of the seagrass canopy in a steady flow (Figure 2B). In short canopies, the canopy height (h) sets the canopy scale (LC ≈ 3–4 h). In tall canopies, this scale is set by the density of the canopy, decreasing with increasing density (Ghisalberti, 2010). There is significant sediment transport on this canopy scale in aquatic canopies.
(3) The “patch” scale (~O; 1–10 m): Sediment transport on the horizontal scale of a patch of vegetation (Figure 2C) impacts patch growth shape (Follett and Nepf, 2012; Ortiz et al., 2013).
(4) The meadow scale (~O; 0.1–1 km): Sediment transport on the scale of an entire seagrass meadow has the potential to create large-scale bedforms (Figure 2D).
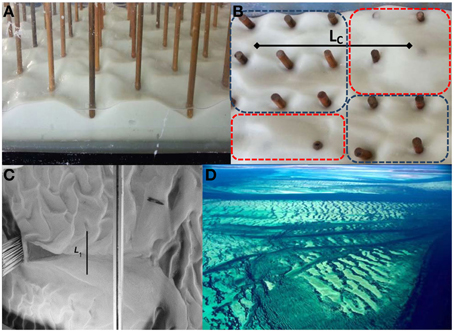
Figure 2. Demonstrations of scales of sediment transport in seagrass meadows. (A) Stem-scale scour in the laboratory (Ng, 2013); (B) Canopy-scale heterogeneity of sediment transport in a uniform array in the laboratory (adapted from Ng, 2013). Alternating zones of strong sediment deposition (red zones, often burying canopy elements) and sediment erosion (blue zones), separated by a distance of the order of LC, are evident; (C) Panoramic view of patch-scale sediment transport in the laboratory. The patch on the left-hand side (flow is from left to right) creates strong scour within the patch, deposition behind the patch, and patch-driven bedforms (taken from Follett and Nepf, 2012); (D) Fauré Sill (500 km2) in Shark Bay, Western Australia, which has formed through thousands of years of sediment deposition within a seagrass meadow (Davies, 1970; image taken by Western Australian Department of Parks and Wildlife).
Physical properties of sediments (e.g., grain size distribution, water content, compaction, shear strength, and bottom stability) are influenced by the feeding, behavior, density and biomass of benthic organisms, and vice versa (Rhoads and Boyer, 1982). For example, sediments colonized by capitellid particles can be repackaged into large fecal pellets that persist for years (Horng and Taghon, 1999); modifying spatial and time trends in coastal sediment transport and morphology, as well as sediment biogeochemistry (e.g., persistence of chemical contaminants). A challenging conceptual approach for transferring knowledge between scales in coastal sediment dynamics would benefit from including chemical and biological factors, together with hydrodynamics and grain interactions; otherwise, models' consistency will remain limited. However, spatial and temporal scales in ecological processes may not correlate linearly, and can “jump” between or appear at different scales at the same time, such as long-lived species and their structures which can cause memory effects in ecosystems (Swaney et al., 2012).
Episodic and Extreme Sediment Transport
Coastal sediment transport can be episodic (Collins and Balson, 2007), in response to infrequent and high magnitude extreme events (Hume and Schalk, 1967), and more frequent and lower magnitude “nuisance level” events. Wright (1987) stated that it is the long-term, net sediment transport fluxes and storages, which are reflected in gross coastal morphology and shoreface stratigraphy. Short periods of rapid forcing, such as due to storms and tsunamis, can be more significant to total and long-term sediment transport than longer periods of weaker forcing (Soulsby, 1983, 1997; Larcombe, 2007). For example, energetic storms may move large boulders, and floods can shift otherwise stable channel positions in deltas (Woodroffe, 2003). Similarly, sand transport paths may be reversed in direction during storm events (Pattiaratchi and Collins, 1984). However, large amounts of sediment transport can also occur during relatively calm conditions (List and Farris, 1999; Larcombe, 2007). In some situations, because extreme events are infrequent, they make a relatively small contribution to the overall sediment dynamics (Soulsby, 1987). On the other hand, episodic events can be the main driver of sediment sources and transport to some areas, such as on some beaches, e.g., in the U.S. (Seymour and Castel, 1985); and on some continental shelves such as in the North Sea (Soulsby, 1987). Also, episodic erosion of coastal cliffs is the dominant sediment source to many coasts (McCave, 1987; Woodroffe, 2003; Violante, 2009). Furthermore, non-extreme events can control the sediment state around engineered structures, with consequences for the resilience to extreme events. For example, non-extreme events can create local scour and self-lowering of seabed pipelines, improving stability under the hydrodynamic loading of subsequent extreme events (Leckie et al., 2015). The geomorphological significance of episodic sediment transport events is determined by the energy of the event, compared to the resilience of the system (at different scales) to change (Woodroffe, 2003).
Understanding the effects of episodic sediment transport events is important when evaluating the impacts of human-induced sediment disturbances (Erftemeijer et al., 2012). This is especially so in areas where the relative contribution of extreme events to sediment transport is greater than that from ambient conditions, such as the middle shelf, and parts of the inner shelf, of the Great Barrier Reef (Larcombe and Carter, 2004). One human-driven cause of episodic sediment transport is dredging operations. Although the scale of dredging has expanded rapidly over the last two decades, in terms of volumes, size, and duration of individual and multiple dredging projects (de Jong et al., 2005), bio-physical impacts appear to remain localized. As such, they should be viewed in perspective against natural sediment disturbances, which can be several orders of magnitude greater (Gao and Collins, 1995; Larcombe et al., 1995), and have timescales of many decades. However, repeated dredging that does not permit sufficient recovery time between operations, can draw significant quantities of fine sediments out of sediment-starved estuaries, leading to erosion of tidal flats and channel instability (Kirby, 2013). Extreme episodes of sediment transport in close proximity to highly industrialized or urbanized areas, can also re-mobilize toxic chemicals, which modifies coastal ecosystems and, hence, sediment dynamics (Einsporn et al., 2005). Severity increases as the frequency of toxic incidents increases, whether due to increased engineering activities or climate change, because the recovery time for biological systems would be insufficient (Wölz et al., 2009). In some situations, natural and human-induced episodes of extreme sediment transport dominate, which must be considered when establishing spatio-temporal data coverage (Coco et al., 2014).
Recovery Time-scales Following Extreme Events
The potential for coastal sediment dynamics to be affected by an event such as a storm, depends upon the balance between event frequency and recovery time-scale (Ferreira, 2005; Coco et al., 2014). Understanding of the coast and beach-recovery processes is much less developed than for erosive conditions. It is generally agreed that recovery by accretion of sediment tends to occur much more slowly than erosion. Several studies suggest recovery time-scales vary significantly with location (Muñoz-Perez and Medina, 2010; Gallop et al., 2012), with beach-erosion recovery time-scales, for example, ranging from days (Birkemeier, 1979), through days and months (Wang et al., 2006), to years (Thom and Hall, 1991). The large spatial variation in recovery between and within sites needs to be considered in the measurement and scaling of coastal sediment dynamics.
Approaches to Scale Transfer
Various approaches have been suggested and used to transfer information on coastal sediment dynamics between scales. These focus largely on the physical properties of sediment, for multi-scale numerical modeling (Roelvink and Reniers, 2012). Data-driven modeling uses derived spatio-temporal relationships between coastal measurements, and oceanographic or atmospheric variables, to project coastal change. This inherently includes scales related to sampling, resolution and range, of both the coastal measurements, and environmental variables. Relationships are generally deduced statistically and may be linear (Larson et al., 2003) or non-linear (Southgate et al., 2003). Linear techniques include bulk statistics, such as means or tend correlations, Fourier analysis, empirical orthogonal functions (Winant et al., 1975), canonical correlational analysis (Karunarathna et al., 2012), and principal oscillation patterns (Różyński, 2002). Non-linear techniques include time-delay embedding (Packard et al., 1980), (multichannel) singular spectrum analysis (Różyński, 2005), forecasting signatures (Rubin, 1992), fractal analysis (Baas, 2002), and neural networks (Weigand, 1994). There are major challenges in ensuring that correlations between variables remain coherent through projection scales. Non-linear techniques go some way to ensuring this, but understanding the non-linearity in coastal systems is non-trivial; there is often a lack of sufficient data coverage to apply these techniques; and there is a need for interpretation of such methods to coastal science, as they are scattered across a range of disciplines (Southgate et al., 2003).
Bottom-up approaches to scale-transfer attempt to understand, and predict, larger-scale coastal sediment dynamics from measurements or models at smaller scales. However, there are various difficulties (de Vriend, 1991a,b; Larson and Kraus, 1995), including imbalanced data availability with scale (Southgate et al., 2003). Another key problem is how to bridge the gap between small-scale and larger-scale processes, such as developing relationships between grain-scale numerical models and bedform development (Sonu, 1968). It is also a challenge to determine how to integrate sediment–biota interactions, for which the spatio-temporal scales may not align with the physical scales (Mann and Lazier, 2013).
Larson and Kraus (1995) demonstrated that quantitative approaches to one-dimensional, cross-shore sediment transport, and morphology can be undertaken at different scales, which are not necessarily contradictory. Nevertheless, it was emphasized that still it is likely to be inappropriate to assume that relationships at large scales are simplifications of the smaller scale. Similar examples of reconciliation of approaches have been given between mesoscale and macroscale, and macroscale to megascale processes, with these scales defined in Figure 1. This principle is related to morphological scaling factors used in numerical modeling (Lesser et al., 2004). The morphological factor multiplies changes in bed sediments, which extends the morphological time-step by increasing the amplitude of short-term changes such as due to tides and waves (Roelvink, 2006). There are several other ways to undertake such morphological updating to allow longer-term modeling, which are discussed in depth by Roelvink (2006), and Roelvink and Reniers (2012). However, each method has problems, thus selecting a suitable morphological factor is not trivial, depending on the site characteristics, and is based usually on best-judgment and sensitivity testing (Lesser et al., 2004).
Aggregated approaches were developed to avoid some of the problems of up-scaling (de Vriend, 1998). Aggregation is the non-trivial process of adding or otherwise combining components (Capobianco et al., 1998). The concept of filtering has been discussed previously to handle the scaling of physics and morphology in delta systems (Capobianco et al., 1998). Various types can be used: where it may be assumed that “representative inputs” of small-scale processes can describe long-term residual effects (input and output filtering); or filtering of processes to permit model reformulation to a larger scale, without describing small-scale effects (formal process filtering), or that which facilitates attempts to qualitatively describe phenomena, without physical descriptions (behavior-orientated modeling).
Capobianco et al. (1998) summarized the three methods used for scaling, where: small-scale mathematics are up-scaled using a statistical expectation operator, that incorporates the small-scale variability (partial transformation); the system is divided into smaller, relatively homogenous parts (partitioning), and the smaller-scale equations are applied to each partition; and re-calibration of the smaller-scale relationships to larger-scales. However, each method can lead to: extremely complex mathematics; subjectivity in selecting partitions, which also require a method for adjusting the parameters for each partition; and large-scale data are not always available for re-calibration. Aggregated approaches provide a pathway for upscaling of information. However, there is a need for more fundamental research on the assumptions of “filtering” smaller scale processes, and how to incorporate non-linear relationships, between scales.
Spatial hierarchies have developed in response to situations where coastal management has jurisdictions at different scales. Therefore, they have an extensive history of being related to governance, with scale-transfer being addressed through aggregation and disaggregation. However, complexities to aggregation develop when transitions of either governance, or active coastal processes, are not aligned. Consequently, the concept of natural management units was developed, where compartments are identified based upon transitions of coastal processes. Applied to multiple processes that are active at different temporal scales, spatial hierarchies may be developed. Hierarchical spatial frameworks have developed in parallel in various parts of the world (e.g., Rosati, 2005; Cooper and Pontee, 2006; Whitehouse et al., 2009). The most generally applied approach is sediment cells, which are identified as self-contained units where little or no sediment movement occurs across cell boundaries (Department for Environment, Food and Rural Affairs (DEFRA), 2006; Whitehouse et al., 2009; George et al., 2015). For less compartmentalized coasts, cells become increasingly notional with greater sediment exchange across boundaries. Sediment cells provide a framework for defining and estimating sediment budgets. The changing nature of cell boundaries at different scales has been illustrated for the Western Australian region, when considered on a cell hierarchy derived from geologic and geomorphic information (Eliot et al., 2011; Stul et al., 2014).
The coastal-tract approach is a framework for scale aggregation, using a contiguous hierarchy of morphological units (Cowell et al., 2003a,b; Stive et al., 2009). The approach uses alongshore characteristics relevant to processes active at discrete temporal scales, to facilitate aggregation. Spatial homogeneity or spatially-balanced heterogeneity may therefore be used to identify hierarchical units that can independently be described as a system, with cross-shore balance including sedimentary processes in estuarine basins and over the adjacent continental shelf. Conservation of sediment volume at each level of the hierarchy constrains morphological responses on a given scale (Stive, 2003), with sediment distributed between smaller spatial units. Coastal advance or retreat is determined quantitatively by the balance between sediment availability and accommodation space (Nicholls et al., 2014). Spatial hierarchy frameworks provide a pathway for upscaling or downscaling, built around factual geologic and geomorphic information. This may reduce dependence on simplifications such as the zero-sum cross-shore transport, often applied to decadal scale coastal change (Eliot et al., 2013).
Concluding Remarks
This contribution provides an overview of the key complexities in transferring information on coastal sediment transport, between discrete spatial and temporal scale bands. Some major issues are: how to consider interaction between grains; sediment–biota interactions; the incorporation of episodic transport; and the establishment of erosion “recovery” time-scales. The influence of bedforms and landforms, as well as sediment–biota interactions varies with spatio-temporal scale. Progress may be made in understanding how to best transfer information from grain-scale, to larger scale processes. In some situations, natural and human-induced episodes of extreme sediment transport can dominate, which must be considered when establishing spatio-temporal data resolution and range. The impact of erosive events is also influenced fundamentally by recovery time-scales, which display significant site-to-site variation. For the various approaches to scaling (e.g., bottom-up, aggregation, spatial hierarchies), there is a need for more fundamental research on the assumptions inherent to their various approaches to scaling.
Author Contributions
All authors presented at or contributed to a workshop on scales in coastal sediment dynamics held at the University of Western Australia, led by CP and MC. The theme of this contribution is based on these collective presentations, and the resulting discussions. SG led the writing of this submission. All authors revised critically the draft manuscript, approving the final version for submission.
Conflict of Interest Statement
The authors declare that the research was conducted in the absence of any commercial or financial relationships that could be construed as a potential conflict of interest.
Acknowledgments
This paper is dedicated to the memory of Professor LC, a quiet achiever in the sometimes broad and raucous field of marine and coastal science. LC was a much valued friend and mentor to his colleagues and students. We will miss his thoughtful and open minded voice. This contribution is the result of a workshop on Temporal and Spatial Scales in Sediment Dynamics, hosted by The University of Western Australia (UWA), Institute of Advanced Studies. MC was funded through the UWA Institute of Advanced Studies “Professor-at-Large” program.
References
Baas, A. C. W. (2002). Chaos, fractals and self-organization in coastal geomorphology: simulating dune landscapes in vegetated environments. Geomorphology 48, 309–328. doi: 10.1016/S0169-555X(02)00187-3
Birkemeier, W. A. (1979). The effects of the 19 December 1977 coastal storm on beaches in North Carolina and New Jersey. Shore Beach 47, 7–15.
Blondeaux, P. (1990). Sand ripples under sea waves. Part 1. Ripple formation. J. Fluid Mech. 218, 1–17. doi: 10.1017/S0022112090000908
Caballeria, M., Coco, G., Falques, A., and Huntley, D. A. (2002). Self-organisation mechanisms for the formation of nearshore crescentic and transverse sand bars. J. Fluid Mech. 465, 379–410. doi: 10.1017/S002211200200112X
Calvete, D., Falques, A., de Swart, H. E., and Walgreen, M. (2001). Modelling the formation of shoreface-connected ridges on storm-dominated inner shelves. J. Fluid Mech. 441, 169–193. doi: 10.1017/S0022112001004815
Capobianco, M., Stive, M. J. F., Jiménez, J. A., and Sanchez-Arcilla, A. (1998). Towards the definition of budget models for the evolution of deltas. J. Coast. Conserv. 4, 7–16. doi: 10.1007/BF02806484
Church, M. (1996). “Space, time and the mountain: how do we order what we see?,” in The Scientific Nature of Geomorphology, eds B. L. Rhoads and E. C. Thorn (Chichester: John Wiley), 147–170.
Coco, G., Senechal, N., Rejas, A., Bryan, K. R., Capo, S., Parisot, J. P., et al. (2014). Beach response to a sequence of extreme storms. Geomorphology 204, 493–501. doi: 10.1016/j.geomorph.2013.08.028
Collins, M. B., and Balson, P. S. (2007). “Coastal and shelf sediment transport: an introduction,” in Coastal and Shelf Sediment Transport, eds P. S. Balson and M. B. Collins (London: Geological Society of London), 1–5. Special Publications 274.
Cooper, N. J., and Pontee, N. I. (2006). Appraisal and evolution of the littoral ‘sediment cell’ concept in applied coastal management: experiences from England and Wales. Ocean Coast. Manag. 49, 498–510. doi: 10.1016/j.ocecoaman.2006.04.003
Cowell, P. J., Roy, P. S., and Jones, R. A. (1995). Simulation of large-scale coastal change using a morphological behaviour model. Mar. Geol. 126, 45–61. doi: 10.1016/0025-3227(95)00065-7
Cowell, P. J., Stive, M. J. F., Niedoroda, A. W., de Vriend, H. J., Swift, D. J. P., Kaminsky, G. M., et al. (2003a). The coastal-tract (Part 1): a conceptual approach to aggregated modeling of low-order coastal change. J. Coast. Res. 19, 812–827.
Cowell, P. J., Stive, M. J. F., Niedoroda, A. W., Swift, D. J. P., de Vriend, H. J., Buijsman, M. C., et al. (2003b). The coastal-tract (Part 2): applications of aggregated modeling of lower-order coastal change. J. Coast. Res. 19, 828–848.
Davies, G. R. (1970). Carbonate bank sedimentation, eastern Shark Bay, Western Australia. Am. Soc. Pet. Geol. Memoir 13, 85–168.
de Jong, R., van Gelderen, P., Lindo, M., and Fernandez, J. (2005). “Dubai's extreme reclamations,” in Paper Presented at the CEDA Dredging Days 2005 Conference (Rotterdam), 2–4.
Department for Environment, Food Rural Affairs (DEFRA). (2006). Shoreline Management Plan Guidance. London: Department for Environment, Food and Rural Affairs.
de Vriend, H. J. (1991a). Mathematical modelling and large-scale coastal behaviour, Part 1: physical processes. J. Hydraulic Res. 29, 727–740. doi: 10.1080/00221689109498955
de Vriend, H. J. (1991b). Mathematical modelling and large-scale coastal behaviour, Part 2: predictive models. J. Hydraulic Res. 29, 741–753. doi: 10.1080/00221689109498956
de Vriend, H. J. (1998). “On the predictability of coastal morphology,” in Third European Proceedings Marine Science and Technology Conference, ed K. G. Barthel (Luxemburg: European Communities), 289–300.
Einsporn, S., Broeg, K., and Koehler, A. (2005). The Elbe flood 2002—toxic effects of transported contaminants in flatfish and mussels of the Wadden Sea. Mar. Pollut. Bull. 50, 423–429. doi: 10.1016/j.marpolbul.2004.11.027
Eliot, I., Nutt, C., Gozzard, B., Higgins, M., Buckley, E., and Bowyer, J. (2011). Coastal Compartments of Western Australia: A Physical Framework for Marine and Coastal Planning. Report 80–82. Damara WA Pty Ltd. Report to the Departments of Environmental and Conservation, Planning and Transport, Environmental Protection Authority.
Eliot, M., Stul, T., and Eliot, I. (2013). “Revisiting landforms in coastal engineering,” in Proceedings of Coasts and Ports (Sydney, NSW), 11–13.
Erftemeijer, P. L. A., Riegl, B., Hoeksema, B. W., and Todd, P. A. (2012). Environmental impacts of dredging and other sediment disturbances on corals: a review. Mar. Pollut. Bull. 64, 1737–1765. doi: 10.1016/j.marpolbul.2012.05.008
Ferreira, O. (2005). Storm groups versus extreme single storms: predicted erosion and management consequences. J. Coast. Res. Spec. Issue 42, 221–227.
Follett, E. M., and Nepf, H. M. (2012). Sediment patterns near a model patch of reedy emergent vegetation. Geomorphology 179, 141–151. doi: 10.1016/j.geomorph.2012.08.006
Gallop, S. L., Bosserelle, C., Eliot, I., and Pattiaratchi, C. B. (2012). The influence of limestone reefs on storm erosion and recovery of a perched beach. Cont. Shelf Res. 47, 16–27. doi: 10.1016/j.csr.2012.08.001
Gao, S., and Collins, M. B. (1995). On the physical aspects of the ‘design with nature’ principle in coastal management. Ocean Coast. Manag. 26, 163–175. doi: 10.1016/0964-5691(95)00013-R
George, D. A., Largier, J. L., Storlazzi, C. D., and Barnard, P. L. (2015). Classification of rocky headlands in California with relevance to littoral cell boundary definition. Mar. Geol. 369, 137–152. doi: 10.1016/j.margeo.2015.08.010
Ghisalberti, M. (2010). The three-dimensionality of obstructed shear flows. Environ. Fluid Mech. 10, 329–343. doi: 10.1007/s10652-009-9161-4
Gracia, F. J., Anfuso, G., Benavente, J., Del Río, L., Domínguez, L., and Martínez, J. A. (2005). Monitoring coastal erosion at different temporal scales on sandy beaches: application to the Spanish Gulf of Cadiz coast. J. Coast. Res. Spec. Issue 49, 22–27.
Holland, K. T., and Holman, R. A. (1996). Field observations of beach cusps and swash motions. Mar. Geol. 134, 77–93. doi: 10.1016/0025-3227(96)00025-4
Holman, R. A. (2001). “Pattern formation in the nearshore,” in River, Coastal and Estuarine Morphodynamics, eds G. Seminara and P. Blondeaux (Berlin: Springer-Verlag), 141–162.
Horng, C.-Y., and Taghon, G. L. (1999). Effects of contaminated sediments on particle size selection by the polychaete Capitella sp. I. J. Exp. Mar. Biol. Ecol. 242, 41–57. doi: 10.1016/S0022-0981(99)00093-3
Hulscher, S. J. M. H., de Swart, H. E., and de Vriend, H. J. (1993). The generation of offshore tidal sand banks and sand waves. Cont. Shelf Res. 13, 1183–1204. doi: 10.1016/0278-4343(93)90048-3
Hume, J. D., and Schalk, M. (1967). Shoreline processes near Barrow, Alaska: a comparison of the normal and the catastrophic. Arctic 20, 86–103. doi: 10.14430/arctic3285
Karunarathna, H., Horillo-Caraballo, J. M., Ranansinghe, R., Short, A. D., and Reeve, D. E. (2012). An analysis of the cross-shore beach morphodynamics of a sandy and composite gravel beach. Mar. Geol. 299–302, 33–42. doi: 10.1016/j.margeo.2011.12.011
Kirby, R. (2013). Managing industrialised coastal fine sediment systems. Ocean Coast. Manag. 79, 2–9. doi: 10.1016/j.ocecoaman.2012.05.011
Larcombe, P. (2007). “Continental shelf environments,” in Environmental Sedimentology, eds C. Perry and K. Taylor (Malden, MA: Blackwell Publishing), 351–388.
Larcombe, P., and Carter, R. M. (2004). Cyclone pumping, sediment partitioning and the development of the great barrier reef shelf system: a review. Quart. Sci. Rev. 23, 107–135. doi: 10.1016/j.quascirev.2003.10.003
Larcombe, P., Ridd, P. V., Prytz, A., and Wilson, B. (1995). Factors controlling suspended sediment on inner-shelf coral reefs, Townsville, Australia. Coral Reefs 14, 163–171. doi: 10.1007/BF00367235
Larson, M., Capobianco, M., Jansen, H., Różyński, G., Southgate, H. N., Stive, M., et al. (2003). Analysis and modeling of field data on coastal morphological evolution over yearly and decadal time scales. Part 1: background and linear techniques. J. Coast. Res. 19, 760–775.
Larson, M., and Kraus, N. C. (1994). Temporal and spatial scales of beach profile change, Duck, North Carolina. Mar. Geol. 117, 75–94. doi: 10.1016/0025-3227(94)90007-8
Larson, M., and Kraus, N. C. (1995). Prediction of cross-shore sediment transport at different spatial and temporal scales. Mar. Geol. 126, 111–127. doi: 10.1016/0025-3227(95)00068-A
Leckie, S., Draper, S., White, D. J., Cheng, L., and Fogliani, N. (2015). Lifelong embedment and spanning of a pipeline on a mobile seabed. Coast. Eng. 95, 130–146. doi: 10.1016/j.coastaleng.2014.10.003
Lesser, G. R., Roelvink, J. A., van Kester, J. A. T. M., and Stelling, G. S. (2004). Development and validation of a three-dimensional morphological model. Coast. Eng. 51, 883–915. doi: 10.1016/j.coastaleng.2004.07.014
List, J. H., and Farris, A. S. (1999). “Large-scale shoreline response to storms and fair weather,” in Proceedings of Coastal Sediments'99, American Society of Civil Engineering (Reston, VA), 1324–1338.
List, J. H., Farris, A. S., and Sullivan, C. (2006). Reversing storm hotspots on sandy beaches: spatial and temporal characteristics. Mar. Geol. 226, 261–279. doi: 10.1016/j.margeo.2005.10.003
Mann, K., and Lazier, J. (2013). Dynamics of Marine Ecosystems: Biological-Physical Interactions in the Oceans. New Jersey, NJ: Wiley-Blackwell.
Masselink, G., and Pattiaratchi, C. B. (1998). Morphological evolution of beach cusps and associated swash circulation patterns. Mar. Geol. 146, 93–113. doi: 10.1016/S0025-3227(97)00129-1
McCave, I. N. (1987). Fine sediment sources and sinks around the East Anglian Coast (UK). J. Geol. Soc. Lond. 144, 149–152. doi: 10.1144/gsjgs.144.1.0149
Muñoz-Perez, J. J., and Medina, R. (2010). Comparison of long-, medium- and short-term variations of beach profiles with and without submerged geological control. Coast. Eng. 57, 241–251. doi: 10.1016/j.coastaleng.2009.09.011
Ng, S. (2013). A Laboratory Study of Sediment Transport in Vegetated Environments using Rigid Cylinders. Honours thesis, School of Environmental Systems Engineering, University of Western Australia.
Nicholls, R. J., Stive, M. J. F., and Tol, R. S. J. (2014). “Coping with coastal change,” in The Scientific Coastal Environments and Global Change, eds G. Masselink and R. Gehrels (Chichester: John Wiley & Sons, Ltd.), 410–431.
Ortiz, A. C., Ashton, A., and Nepf, H. (2013). Mean and turbulent velocity fields near rigid and fleixble plants and the implications for deposition. J. Geophys. Res. 118, 2585–2599. doi: 10.1002/2013JF002858
Packard, N. H., Crutchfield, J. P., Farmer, J. D., and Shaw, R. S. (1980). Geometry from a time series. Phys. Rev. Lett. 45, 712–716. doi: 10.1103/PhysRevLett.45.712
Pattiaratchi, C. B., and Collins, M. B. (1984). Sediment transport under the combined influence of waves and currents: a case study from the Northern Bristol Channel, U.K. Mar. Geol. 56, 27–40. doi: 10.1016/0025-3227(84)90004-5
Rhoads, D. C., and Boyer, L. F. (1982). The effects of marine benthos on physical properties of sediments. Topics Geobiol. 100, 3–52. doi: 10.1007/978-1-4757-1317-6_1
Roelvink, J. A. (2006). Coastal morphodynamic evolution techniques. Coast. Eng. 53, 277–287. doi: 10.1016/j.coastaleng.2005.10.015
Roelvink, J. A., and Reniers, A. J. H. M. (2012). A Guide to Modelling Coastal Morphology. Singapore: World Scientific.
Rosati, J. D. (2005). Concepts in sediment budgets. J. Coast. Res. 21, 307–322. doi: 10.2112/02-475A.1
Różyński, G. (2005). Long-term shoreline response of a nontidal barred coast. Coast. Eng. 52, 79–91. doi: 10.1016/j.coastaleng.2004.09.007
Różyński, G., and Jansen, H. (2002). Modeling nearshore bed topography with principal oscillation patterns. J. Waterway Port Coast. Ocean Eng. 128, 202–215. doi: 10.1061/(ASCE)0733-950X(2002)128:5(202)
Rubin, D. M. (1992). Use of forecasting signatures to help distinguish periodicity, randomness, and chaos in ripples and other spatial patterns. Chaos 2, 525–535. doi: 10.1063/1.165894
Seymour, R. J., and Castel, D. (1985). Episodicity in longshore sediment transport. J. Waterway Port Coast. Ocean Eng. 111, 542–551. doi: 10.1061/(ASCE)0733-950X(1985)111:3(542)
Slaymaker, O., Spencer, T., and Dadson, S. (2009). “Landscape and landscape-scale processes as the unfilled niche in the global environmental change debate: an introduction,” in Geomorphology and Global Environmental Change, eds O. Slaymaker, T. Spencer, and C. Embleton-Hamann (Cambridge: Cambridge University Press), 1–36.
Sonu, C. J. (1968). “Collective movement of sediment in littoral environment,” in Proceedings of the 11th International Conference on Coastal Engineering, ASCE (New York, NY), 373–400.
Soulsby, R. (1983). “The bottom boundary layer of shelf seas,” in Physical Oceanography of Coastal and Shelf Seas, ed B. Johns (Amsterdam: Elsevier), 189–266.
Soulsby, R. L. (1987). The Relative Contributions of Waves and Tidal Currents to Marine Sediment Transport. Report No. SR 124, Hydraulics Research, Wallingford, CT.
Southgate, H. N., Wijnberg, K. M., Larson, M., Capobianco, M., and Jansen, H. (2003). Analysis of field data of coastal morphological evolution over yearly and decadal timescales. Part 2: non-linear techniques. J. Coast. Res. 19, 776–789.
Stive, M. J. F. (2003). “Advances in morphodynamics of coasts and lagoons,” in Proceedings of the International Conference on Estuaries and Coasts (Hangzhou), 23–35.
Stive, M. J. F., Aarninkhof, S. G. J., Hamm, L., Hanson, H., Larson, M., Wijnberg, K. M., et al. (2002). Variability of shore and shoreline evolution. Coast. Eng. 47, 211–235. doi: 10.1016/S0378-3839(02)00126-6
Stive, M. J. F., Cowell, P. J., and Nicholls, R. J. (2009). “Impact of global environmental change on beaches, cliffs and deltas,” in Geomorphology and Global Environmental Change, eds O. Slaymaker, T. Spencer, and C. Embleton-Hamann (Cambridge: Cambridge University Press), 158–179.
Stive, M. J. F., Roelvink, D. J. A., and de Vriend, H. J. (1991). “Large-scale coastal evolution concept,” in Proceedings of the 22nd International Conference on Coastal Engineering ASCE (New York, NY), 1962–1974.
Stul, T., Gozzard, J. R., Eliot, I. G., and Eliot, M. J. (2014). Coastal Sediment Cells for the Pilbara Region between Giralia and Beebingarra Creek, Western Australia. Report prepared by Seashore Engineering Pty. Ltd. and Geological Survey of Western Australia for the Western Australian Department of Transport, Fremantle.
Swaney, D. P., Humborg, C., Emeis, K., Kannen, A., Silvert, W., Tettf, P., et al. (2012). Five critical questions of scale for the coastal zone. Estuar. Coast. Shelf Sci. 96, 9–21. doi: 10.1016/j.ecss.2011.04.010
Thom, B. G., and Hall, W. (1991). Behaviour of beach profiles during accretion and erosion dominated periods. Earth Surf. Process. Landforms 16, 113–127. doi: 10.1002/esp.3290160203
van Enckevort, I. M. J., Ruessink, B. G., Coco, G., Suzuki, K., Turner, I. L., Plant, N. G., et al. (2004). Observations of nearshore cresentic sandbars. J. Geophys. Res. 109, C06028. doi: 10.1029/2003JC002214
Violante, C. (2009). Rocky coast: geological constraints for hazard assessment. Geol. Soc. Lond. Spec. Publ. 322, 1–31. doi: 10.1144/SP322.1
Wang, P., Kirby, J. H., Haber, J. D., Horwitz, M. H., Knorr, P. O., and Krock, J. R. (2006). Morphological and sedimentological impacts of hurricane Ivan and immediate post-storm beach recovery along the northwestern Florida barrier-island coasts. J. Coast. Res. 6, 1382–1402. doi: 10.2112/05-0440.1
Weigand, A. S. (1994). “Paradigm changes in prediction,” in Chaos and Forecasting, Proceedings of the Royal Society Discussion Meeting, ed H. Tong (Singapore: World Scientific), 145–160.
Whitehouse, R., Balson, P., Beech, N., Brampton, A., Blott, S., Burningham, H., et al. (2009). Characterisation and Prediction of Large-scale, Long-term Change of Coastal Geomorphological Behaviours: Final Science Report. Science Report SC060074/SR1. Joint DEFRA and Environment Agency Food and Coastal Erosion Risk Management R&D Programme, Environment Agency and Department for Environment, Food and Rural Affairs, UK.
Winant, C. D., Inman, D. L., and Nordstrom, C. E. (1975). Description of seasonal beach changes using empirical eigenfunctions. J. Geophys. Res. 80, 1979–1986. doi: 10.1029/JC080i015p01979
Wölz, J., Cofalla, C., Hudjetz, S., Roger, S., Brinkmann, M., Schmidt, B., et al. (2009). In search for the ecological and toxicological relevance of sediment re-mobilisation and transport during flood events. J. Soils Sediments 9, 1–5. doi: 10.1007/s11368-008-0050-0
Woodroffe, C. D. (2003). Coasts: Form, Process and Evolution. Cambridge: Cambridge University Press.
Keywords: sediment transport, coastal morphodynamics, temporal scales, spatial scales, sediment cells, episodic sediment transport, sediment-biota interactions, scale transfer
Citation: Gallop SL, Collins M, Pattiaratchi CB, Eliot MJ, Bosserelle C, Ghisalberti M, Collins LB, Eliot I, Erftemeijer PLA, Larcombe P, Marigómez I, Stul T and White DJ (2015) Challenges in transferring knowledge between scales in coastal sediment dynamics. Front. Mar. Sci. 2:82. doi: 10.3389/fmars.2015.00082
Received: 24 August 2015; Accepted: 01 October 2015;
Published: 20 October 2015.
Edited by:
Pengfei Xue, Michigan Technological University, USAReviewed by:
Vanesa Magar, Centro de Investigacion Cientifica y Educacion Superior de Ensenada, MexicoGangfeng Ma, Old Dominion University, USA
Copyright © 2015 Gallop, Collins, Pattiaratchi, Eliot, Bosserelle, Ghisalberti, Collins, Eliot, Erftemeijer, Larcombe, Marigómez, Stul and White. This is an open-access article distributed under the terms of the Creative Commons Attribution License (CC BY). The use, distribution or reproduction in other forums is permitted, provided the original author(s) or licensor are credited and that the original publication in this journal is cited, in accordance with accepted academic practice. No use, distribution or reproduction is permitted which does not comply with these terms.
*Correspondence: Shari L. Gallop, c2hhcmkuZ2FsbG9wQG1xLmVkdS5hdQ==