- 1Plant Functional Biology and Climate Change Cluster, University of Technology, Sydney, Sydney, NSW, Australia
- 2Marine Biological Section, Department of Biology, University of Copenhagen, Helsingør, Denmark
- 3Department of Bioscience - Microbiology, Aarhus University, Aarhus, Denmark
The O2 budget of seagrasses is regulated by a complex interaction between several sources and sinks, which is strongly regulated by light availability and mass transfer over the diffusive boundary layer (DBL) surrounding the plant. Epiphyte growth on leaves may thus strongly affect the O2 availability of the seagrass plant and its capability to aerate its rhizosphere as a defense against plant toxins. We used electrochemical and fiber-optic microsensors to quantify the O2 flux, DBL, and light microclimate around leaves with and without filamentous algal epiphytes. We also quantified the below-ground radial O2 loss (ROL) from roots (~1 mm from the root-apex) to elucidate how this below-ground oxic microzone was affected by the presence of epiphytes. Epiphyte-cover on seagrass leaves (~21% areal cover) resulted in reduced light quality and quantity for photosynthesis, thus leading to reduced plant fitness. A ~4 times thicker DBL around leaves with epiphyte-cover impeded gas (and nutrient) exchange with the surrounding water-column and thus the amount of O2 passively diffusing down to the below-ground tissue through the aerenchyma in darkness. During light exposure of the leaves, radial oxygen loss from the below-ground tissue was ~2 times higher from plants without epiphyte-cover. In contrast, no O2 was detectable at the surface of the root-cap tissue of plants with epiphyte-cover during darkness, leaving the plants more susceptible to sulfide intrusion. Epiphyte growth on seagrass leaves thus has a negative effect on the light climate during daytime and O2 supply in darkness, hampering the plants performance and thereby reducing the oxidation capability of its below-ground tissue.
Introduction
Seagrasses are angiosperms that form coastal habitats of prime importance for marine biodiversity and carbon sequestration (Duarte, 2001; Duarte et al., 2005). Over the past century, seagrasses have faced an alarming global decline, owing to both direct and indirect human interference (Robblee et al., 1991; Zieman et al., 1999; Seddon et al., 2000; Plus et al., 2003; Orth et al., 2006). Seagrasses inhabit organic rich, reduced sediments and the exposure of their below-ground biomass to sediment-derived hydrogen sulfide (H2S), as a result of inadequate internal aeration due to low water-column O2 levels during darkness, has been identified as a key factor in seagrass die-back events (Greve et al., 2003; Borum et al., 2005; Brodersen et al., 2015). Hydrogen sulfide is produced in reduced sediment through bacterial sulfate reduction, which is considered the quantitatively most important anaerobic degradation process in coastal marine sediment (Jørgensen, 1982). H2S is a phytotoxin that leads to chemical asphyxiation, due to a strong chemical binding with cytochrome c in the mitochondrial electron transport chain (Eghbal et al., 2004; Pérez-Pérez et al., 2012; Lamers et al., 2013). If H2S reaches the root tissue surface it may enter the lacunar system of the seagrass plant via lipid-solution permeation of the plasmalemma (Raven and Scrimgeour, 1997). Such H2S intrusion into the below-ground tissue of seagrasses has mainly been related to inadequate internal aeration during night-time, as a result of a low water-column O2 content and thus a decrease in the diffusive O2 supply from the surrounding water-column (Pedersen et al., 2004; Borum et al., 2005). The amount of O2 passively diffusing into the leaves from the water-column during darkness, is thus highly dependent on the water-column O2 content, but is also strongly affected by other factors such as the DBL thickness (Binzer et al., 2005; Borum et al., 2006) and the leaf surface area. The DBL surrounds all aquatic surfaces, such as seagrass leaves, and functions as a diffusive barrier to the exchange of gasses and nutrients with the surrounding water-column by impeding water motions toward the leaf tissue surface (Jørgensen and Revsbech, 1985). The width and thus the mass transfer impedance of the DBL depends on factors such as the surface topography and the flow velocity, where e.g., relative low flow rates and uneven surfaces increases the thickness of the DBL (Jørgensen and Des Marais, 1990); both parameters are highly affected by epiphyte growth on the leaf surface.
Light availability is the key environmental factor regulating photosynthesis and thus the O2 supply during day-time, and small decreases in irradiance can cause significant declines in the growth and distribution of seagrasses (Burkholder et al., 2007; Ralph et al., 2007). In eutrophic coastal waters, light can be attenuated up to 100-fold in the upper 1–4 m of the water column, often with dramatic changes in the spectral composition (Sand-Jensen and Borum, 1991). Therefore, rooted macrophytes are often spatially limited to biotopes with sufficient light exposure, i.e., water depths experiencing a minimum of 10% of surface irradiance for temperate seagrasses (Borum, 1983; Duarte, 1991). Eutrophication can stimulate epiphyte colonization on seagrass leaves (Richardson, 2006) potentially affecting the light availability for the plant. Epiphytes may thus have a major impact on the photosynthetic O2 evolution of rooted macrophytes, such as seagrasses (Sand-Jensen, 1977).
The O2 budget of seagrass plants is regulated by a complex interaction between several sources and sinks. Sources encompass photosynthetic O2 evolution in leaves during day-time and passive diffusion of O2 into the leaves from the water-column in darkness. Sinks encompass the total O2 demand of the surrounding sediment, including bacterial respiration and chemical reactions with reduced compounds, as well as the plants own respiratory needs. The amount of O2 produced or passively diffusing into the leaves is affected by external physical factors such as the light availability for underwater photosynthesis, the flow-dependent thickness of the DBL and the water-column O2 content, whereas the sinks are highly affected by elevated seawater temperatures and the quantity of accessible organic matter in the rhizosphere (Pedersen et al., 2004; Binzer et al., 2005; Borum et al., 2006; Raun and Borum, 2013).
The O2 is transported from the above-ground tissue to the below-ground tissue through the aerenchyma, i.e., an internal gas-filled lacunar system, whereby plants support aerobic metabolism in their root-system and provide protection against reduced toxic compounds such as H2S and Fe2+ (Armstrong, 1979; Borum et al., 2006). Some of the transported O2 is leaked to the rhizosphere as the so-called radial oxygen loss (ROL), especially at the basal leaf meristems, root-shoot junctions and root-caps (Koren et al., 2015). During non-stressful environmental conditions, ROL maintains a ~0.5 mm wide oxic microzone around the leaking areas that continuously oxidizes the surrounding sediment and thus alters the immediate sediment biogeochemistry in the seagrass rhizosphere (Pedersen et al., 1998; Jensen et al., 2005; Brodersen et al., 2015). This chemical defense mechanism is, however, negatively affected by over-night water-column hypoxia (Brodersen et al., 2015).
Seagrass morphology is an important controlling factor affecting the likelihood of H2S intrusion into seagrasses, where a higher above- to below-ground biomass ratio positively affects the seagrasses oxidation capacity and reduces the risk of H2S intrusion (Frederiksen et al., 2006). Seagrass roots possess structural barriers to ROL in mature root tissue regions such as Casparian band-like structures of suberin in the hypodermis (Barnabas, 1996). Such barriers to ROL in the basal-parts of seagrass roots increase the intra-plant O2 transport to the active apical root meristem and therefore are very important for seagrass root metabolism.
In this study, we used electrochemical and fiber-optic microsensors to investigate effects of epiphyte-cover on seagrass leaves on the below-ground aeration of the rhizosphere of the seagrass Zostera marina kept in a custom-made split flow-chamber with natural sediment. This microenvironmental approach allowed us to (i) analyse the DBL and light microclimate around seagrass leaves with- and without epiphytes, and (ii) correlate changes in these above-ground micro-environmental parameters with changes in the ROL from the root-caps, and thereby, the oxidation capacity of the below-ground tissue.
Materials and Methods
Seagrass and Sediment Sampling
Marine sediment and Z. marina specimens with and without leaf epiphyte-cover were collected from shallow coastal waters (< 2 m depth) at Aggersund, Limfjorden, Denmark. After sampling, plants and sediment were transported to a nearby field station (Rønbjerg Marine Biological Station, Aarhus University, Denmark), where they were kept in constantly aerated water reservoirs prior to experiments. Seagrass specimens with similar above- and below-ground biomass ratios were selected from the reservoirs and gently washed free of adhering sediment before transferred to the experimental split flow-chamber (see below; Brodersen et al., 2014). In the following, seagrasses with epiphyte-cover refer to plants with ~21% areal cover of filamentous algal epiphytes on leaves in contrast to seagrasses without visible leaf epiphyte-cover. The above- to below-ground biomass ratio was 1.0 and 0.8 of selected plants with and without leaf epiphyte-cover, respectively, based on g DW values obtained after drying the plants in an oven at 60°C until a constant weight was reached.
Experimental Setup
Plants were horizontally positioned in the flow-chamber (one plant at a time) with the leaf canopy in the free flowing water phase compartment and the below-ground biomass transplanted in homogenized sediment from the sampling site in the adjoining “sediment” compartment (Figure 1). An anoxic water column (~2 cm depth) functioned as a liquid-phase diffusion barrier to O2 intrusion over the sediment compartment of the flow chamber, as preliminary studies had shown a constant loss/efflux of reduced compounds such as H2S from the sediment during incubation. Illumination of the leaf canopy was provided by a fiber-optic tungsten halogen lamp (KL-2500LCD, Schott GmbH, Germany). The downwelling photon irradiance (PAR, 400–700 nm) at the leaf surface was measured with a spherical quantum sensor (US-SQS/L, Walz GmbH, Germany) connected to a calibrated quantum irradiance meter (ULM-500, Walz GmbH, Germany). A constant flow (~0.5 cm s−1) of aerated seawater (~22°C, Salinity = 30) was maintained in the seawater compartment of the flow chamber by means of a pump submersed in an aerated seawater reservoir (Figure 1).
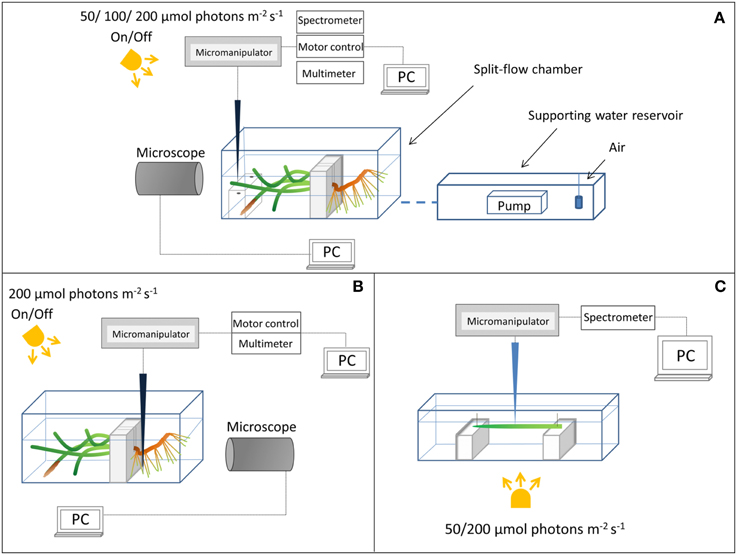
Figure 1. Schematic diagram of the experimental setups. (A) Above-ground light and O2 microsensor measurements. (B) Measurements on the below-ground chemical microenvironment with Clark-type O2 microsensors. (C) Measuring light transmission spectra at the seagrass leaf surface.
Light and O2 Measurements
We used scalar irradiance microprobes (sphere diameter 50 μm; manufactured by a modified procedure of Lassen et al., 1992; Rickelt et al., submitted) to quantify the light microenvironment around leaves of Z. marina with- and without epiphyte cover under two different irradiance levels (50 and 200 μmol photons m−2 s−1; Figure 1A). The scalar irradiance microprobe was connected to a fiber-optic spectrometer (USB 2000+, Ocean Optics, USA), interfaced to a PC running spectral acquisition software (SpectraSuite, Ocean Optics, USA). We measured vertical profiles of spectral scalar irradiance in 0.1 mm steps from the leaf surface to 1 mm above the leaf surface, and in 1 mm steps from 1 to 10 mm from the leaf surface. To quantify the downwelling irradiance, we recorded spectra of the vertically incident light with the scalar irradiance microprobe tip positioned over a black non-reflective light well at the same position and distance in the light beam as the seagrass tissue surface; in a collimated light field the downwelling- and scalar irradiance are identical (Kühl and Jørgensen, 1994).
Clark-type O2 microsensors (OX-10 and OX-50, Unisense A/S, Aarhus, Denmark; Revsbech, 1989) with a fast response time (< 0.5 s) and low stirring sensitivity (< 2–3%) were used to measure (i) the radial O2 loss (ROL) from the below-ground biomass of Z. marina (~1 mm from the root-apex; Figure 1B), and (ii) the O2 concentration at and toward the leaf surface (Figure 1A). The O2 microsensors were linearly calibrated from signal readings in 100% air saturated seawater and anoxic seawater (by addition of ascorbate) at experimental temperature and salinity; prior to calibrations and measurements in natural sediment, the microsensors were pre-contaminated with sulfide, i.e., they were pre-polarized in a Na2S solution, to avoid drifting calibrations during experiments.
Microsensors were mounted on a motorized micromanipulator (Unisense A/S, Denmark) and connected to a PC-interfaced microsensor multimeter (Unisense A/S, Denmark); both were controlled by dedicated data acquisition and positioning software (SensorTrace Pro, Unisense A/S, Denmark). Microsensors and microprobes were carefully positioned at the tissue surface (defined as 0 μm) by manual operation of the micromanipulator, while observing the microsensor tip and tissue surface with a USB microscope (AD7013MZT, DinoLite, AnMo Electronics Corp., Taiwan). When positioning the O2 microsensors at the below-ground tissue surface, a root from the first root-bundle was first gently un-covered from sediment before manually moving the microsensor to the surface of the root-cap, where after the root was gently covered again with sediment. Steady state O2 levels at the below-ground tissue surface were re-established after ~3 h (data not shown). Microprofiles of O2 concentration were measured in depth increments of 50 μm.
Light Calculations
To quantify PAR, we integrated the measured scalar irradiance spectra over 400–700 nm and calculated the fractions of incident PAR irradiance for each measured depth position. By multiplying with the known incident photon irradiance (in μmol photons m−2 s−1), measured with a calibrated quantum irradiance meter (ULM-500, Walz GmbH, Germany) equipped with a spherical quantum sensor (US-SQS/L, Walz GmbH, Germany), absolute photon scalar irradiance levels in each depth could be calculated as:
where E(PAR)z is the PAR photon scalar irradiance in depth z, Az is the wavelength integrated signal in depth z, AD is the wavelength integrated downwelling irradiance, and Ed is the downwelling photon irradiance (in μmol photons m−2 s−1).
Since the leaves of Z. marina were ~50 μm thick, it was not possible to measure internal light gradients in the leaves with microprobes. Instead we measured the spectral attenuation of light through leaves with and without epiphyte cover. A leaf, with- or without epiphytes, was positioned in a transparent acrylate chamber illuminated from below and with the incident irradiance determined as above (Figure 1C). Concomitantly, the microprobe was positioned at the abaxial surface of the leaf and the transmitted spectra were recorded on leaves with- and without epiphytes.
Flux Calculations
The O2 flux between the leaf surface and the surrounding seawater was calculated using Fick's first law of diffusion:
where D0 is the molecular diffusion coefficient of O2 in seawater at experimental temperature and salinity (2.0845·10−5 cm−2 s−1; tabulated values available at www.unisense.com), and is the slope of the linear O2 concentration gradient within the DBL.
A cylindrical version of Fick's first law of diffusion, described by Steen-Knudsen (2002), was used to calculate the ROL from the below-ground tissue surface (assuming a homogenous and cylinder-shaped O2 loss from the roots):
where φ is the porosity of the sediment and φD0 estimates the diffusivity of O2 within the sediment at experimental temperature and salinity, r is the radius of the root, and C1 and C2 are the O2 concentrations measured at the radial distances r1 and r2, respectively. Porosity was determined from the weight loss of wet sediment from the sampling site (known initial volume and weight) after drying at 60°C until a constant weight was reached (Porosity = 0.51).
Statistical Procedures
Data were tested for normality (Shapiro-Wilk) and equal variance prior to statistical analysis. Student's t-tests were used to compare treatments (with- or without leaf epiphytes) on data that met the above-mentioned assumptions. Mann-Whitney Rank Sum tests were used on data lacking normality and/or equal variance. A Two-Way ANOVA was performed to examine the influence of leaf epiphyte-cover and incident irradiance on O2 fluxes across the leaf tissue surface (Table S1). Analysis of covariance (ANCOVA) was used to examine the effect of leaf epiphytes on scalar irradiance with distance from the leaf surface as a covariant. The significance level was set to p < 0.05. Statistical tests were performed in SigmaPlot and SPSS.
Results
Light Climate
Our observations on the light microclimate around the leaves of Z. marina revealed that epiphyte cover affect the quantity and quality of light reaching the seagrass leaf.
In the presence of epiphytes, photon scalar irradiance (PAR, 400–700 nm) on the surface of seagrass leaves was reduced by 54 and 92% under a downwelling photon irradiance of 50 and 200 μmol photons m−2 s−1, respectively (Figure 2). Without epiphytes, we observed a 3 and 4% increase in photon scalar irradiance at incident irradiance levels of 50 and 200 μmol photons m−2 s−1, respectively. Analysis of covariance (ANCOVA) confirmed significant difference in the scalar irradiance at the leaf tissue surface of plants with leaf epiphyte cover as compared to plants without leaf epiphyte cover (p < 0.01), as well as between photon scalar irradiance measured at z = 10 mm and z = 0 mm for plants with leaf epiphyte cover (p < 0.01). No significant difference was found between photon scalar irradiance measured at z = 10 mm and z = 0 mm for plants without leaf epiphyte cover (p > 0.05).
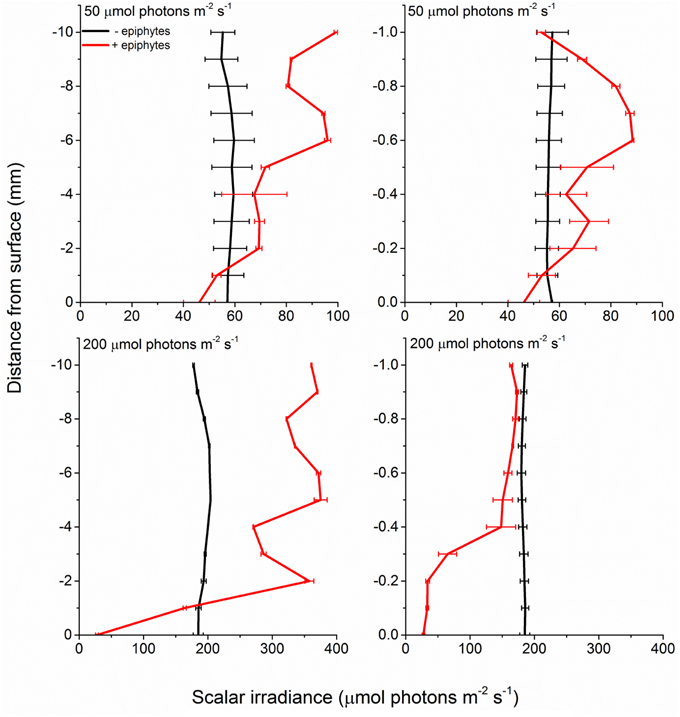
Figure 2. Profiles of photon scalar irradiance measured at two different downwelling photon irradiances (50- and 200 μmol photons m−2 s−1) on Z. marina leaves with- and without epiphyte cover. Left panels show the scalar irradiance 0–10 mm from the leaf surface measured in 1 mm steps. Right panels show the scalar irradiance 0–1 mm from the leaf surface measured in 0.1 mm steps (enlarged plots of the scalar irradiance showed in the left panels). Data points represents means ± S.D. n = 3; leaf level replicates.
The decrease in scalar irradiance in the upper canopy (1–10 mm above the leaf surface) was uniform across wavelengths in the PAR region, while a spectral shift became evident in the lower canopy (0–1 mm above the leaf surface) with blue light and light around 675 nm being absorbed preferentially (Figure 3). However, approaching the surface of the seagrass leaf we also observed an enhanced absorption around 625 nm indicative of phycocyanin found in cyanobacteria.
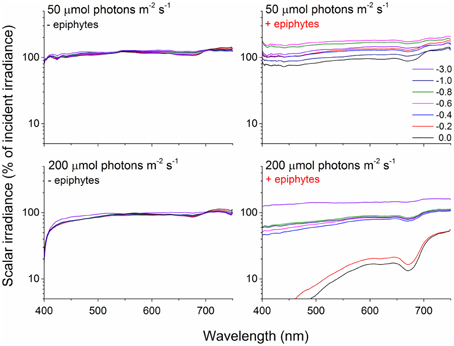
Figure 3. Spectral scalar irradiance measured over Z. marina leaves under an incident irradiance of 50 and 200 μmol photons m−2 s−1 with- (right panels) and without epiphytes (left panels). Colored lines represents spectra collected at the given depths in mm above the leaf surface expressed as % of incident irradiance on a log-scale. n = 3; leaf level replicates.
This was further clarified in the seagrass light transmission spectra (Figure 4) where, in the absence of epiphytes, mainly actinic light and light around 675 nm were absorbed, corresponding to the absorption spectrum of Chl a. In the presence of epiphytes there was a profound decrease in all wavelengths in the PAR region leading to a reduction in the transmitted light with 71 and 88% (downwelling photon irradiance of 50 and 200 μmol photons m−2 s−1, respectively). Students t-tests performed at 425, 560, and 675 nm (except at 425 nm under an incident irradiance of 50 μmol photons m−2 s−1, where a Mann-Whitney test was performed due to data lacking normality; p < 0.05) confirmed significant difference in the transmitted light spectra between plants with leaf epiphyte cover and plants without leaf epiphyte cover (p < 0.01). In addition there was a relatively larger absorption of green light in the presence of epiphytes, evident from a change in the ratio of wavelengths 560:675 nm from six without epiphytes to three with epiphytes suggesting absorption from accessory epiphyte pigments.
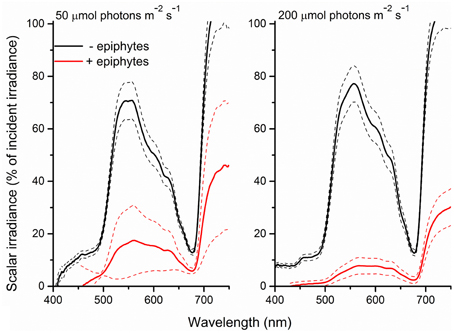
Figure 4. Spectra of scalar irradiance transmitted through Z. marina leaves with- (red line) and without (black line) epiphyte cover and at two different downwelling photon irradiances (50- and 200-μmol photons m−2 s−1). Dashed lines represents ± S.D. n = 4; leaf level replicates.
Diffusive Boundary Layer and Photosynthesis
The O2 concentration microprofiles at the Z. marina leaf tissue surface revealed a ~4 times thicker DBL around leaves with epiphyte-cover as compared to leaves without epiphyte-cover, i.e., an increase in the DBL thickness from ~350 to 1400 μm (Figure 5). During darkness, passive diffusion of O2 from the surrounding water-column resulted in a constant influx of O2 into leaves both with and without epiphyte-cover, supporting the below-ground tissue with O2 (Figure 5; Table 1). However, the thick DBL around leaves with epiphyte-cover impeded the diffusive O2 supply in darkness as compared to plants without epiphyte-cover [seen as a reduction in the seagrass leaf surface O2 concentration from ~198 to 51 μmol L−1 (Student's t-test, p < 0.001); Figure 5], leaving these plants more vulnerable to low water-column O2 contents at night-time.
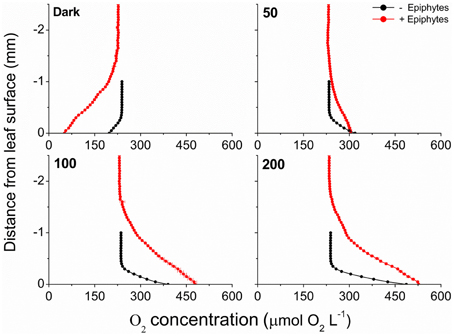
Figure 5. Vertical microprofiles of the O2 concentration measured toward the leaf surface under four different incident photon irradiances (0, 50, 100, and 200 μmol photons m−2 s−1). Red symbols and lines represent leaves with 21% epiphyte-cover, Black symbols and lines represent leaves without epiphyte-cover. y = 0 indicates the leaf surface. Symbols and errors bars represent means ± SD. n = 3–4; leaf level replicates.
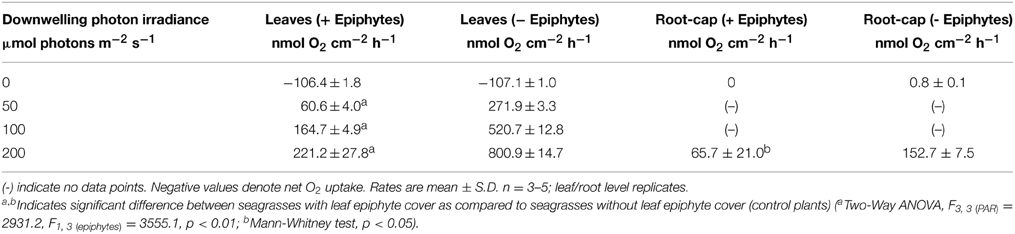
Table 1. O2 fluxes across the leaf surface and radial O2 loss from the root-cap (~1 mm from the root-apex).
Net O2 production increased with increasing photon irradiance, as a result of enhanced shoot photosynthesis (Figure 5). The lower light availability for plants with epiphyte-cover resulted in relatively lower net photosynthesis rates, and the compensation irradiance increased from ~12 to 27 μmol photons m−2 s−1 for plants with epiphyte-cover (Figure 6; Table 1). Despite the lower net photosynthesis in plants with leaf epiphyte-cover, there was a higher build-up of O2 on the tissue surface under moderate photon irradiances (100 μmol photons m−2 s−1) as compared to plants without leaf epiphyte-cover, owing to limited gas exchange with the surrounding water-column as a result of the enhanced DBL thickness.
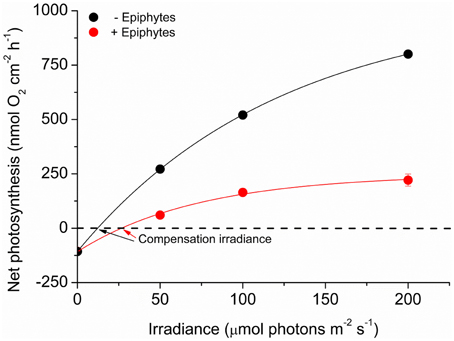
Figure 6. Net photosynthesis rates as a function of downwelling photon irradiance. Rates were calculated for the four different incident irradiances (0, 50, 100, and 200 μmol photons m−2 s−1) and were fitted with a hyperbolic tangent function (Webb et al., 1974) with an added term to account for respiration (Spilling et al., 2010) (R2 = 0.99). Red symbols and line represent leaves with ~21% epiphyte-cover. Black symbols and line represent leaves without epiphyte-cover. Error bars are ±SD. n = 3–4; leaf level replicates.
Radial O2 Loss
We used the measured steady state O2 microprofiles around the root-cap of Z. marina with and without leaf epiphyte-cover (Figure 7), to calculate the radial O2 flux into the surrounding sediment. In light, we calculated the ROL from the root-cap to be 65.7 nmol O2 cm−2 h−1 from plants with leaf epiphyte-cover as compared to 152.7 nmol O2 cm−2 h−1 from plants without leaf epiphyte-cover (Table 1). The ROL maintained a ~300 μm thick oxic microzone around the root-cap of Z. marina (Figure 7). In darkness, the ROL from the root-cap dramatically decreased to 0 nmol O2 cm−2 h−1 in plants with leaf epiphyte-cover (i.e., no O2 was detectable at the root surface during darkness; Figure 7), and 0.8 nmol O2 cm−2 h−1 in plants without leaf epiphyte-cover (Table 1). Epiphyte-covered plants did thus lose their oxic microshield against H2S intrusion in darkness.
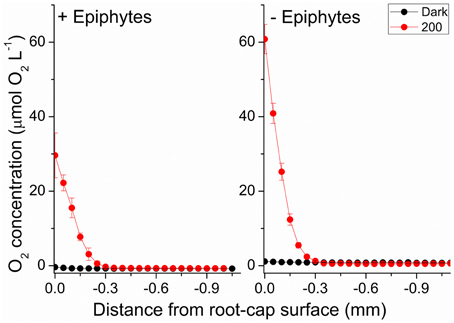
Figure 7. Radial O2 loss from the root-cap of Z. marina (~1 mm from the root-apex) to the immediate rhizosphere measured at two different photon irradiances (0 and 200 μmol photons m−2 s−1). Left panel show radial O2 loss from seagrass with leaf epiphyte-cover, right panel show radial O2 loss from seagrass without leaf epiphyte-cover. X = 0 indicates the root surface. Error bars are ±SD. n = 3–5; root level replicates.
Discussion
Our results provide clear experimental evidence that epiphyte growth on Z. marina leaves reduces both light quantity and quality reaching the seagrass leaf, thereby impeding the overall plant performance during day-time. Furthermore, leaf epiphyte-cover lead to an enhanced thickness of the DBLs surrounding the leaves, thus impeding the exchange of gasses and essential nutrients with the ambient water-column. In darkness, this resulted in a negative effect on the intra-plant O2 status that subsequently reduced the oxidation capability of the below-ground tissue, thereby rendering plants more vulnerable to sediment-produced reduced phytotoxic compounds, such as H2S.
Light Microenvironment and Shoot Photosynthesis
Light availability on the surface of the leaves of Z. marina covered by epiphytes was dramatically decreased compared to leaves without epiphytes in agreement with previous studies (Drake et al., 2003; Pedersen et al., 2014). Effectively, this means that a higher downwelling photon irradiance is needed to meet the compensation irradiance for the epiphyte covered leaf (Figure 6). We expected a larger change in the spectral quality of light reaching the leaf surface through the epiphyte canopy, but as the generation time of unicellular and filamentous algae colonizing the seagrass are short relative to the seagrass leaves, there might have been a large proportion of dead epiphytes thus acting as particulate organic matter with a more uniform light attenuation (Figure 3, upper right). However, in the lower epiphyte canopy (0–1 mm above the seagrass surface) there was a non-uniform attenuation of light leading to a strong reduction in blue light reaching the seagrass surface (Figure 3, lower right). In the transmittance spectra, we saw a disproportionate large amount of green light being attenuated in the presence of epiphytes indicating the presence of a community possessing accessory pigments able to utilize green light, such as red algal or cyanobacterial phycobiliproteins.
Although a large proportion of the green light was attenuated by epiphytes, blue and red light were almost completely removed, leaving the plant in a light environment with predominately green light which is less effectively absorbed by Chl a. Thus, both quality and especially the quantity of light were diminished in the presence of epiphytes thereby leaving the plant for longer periods near the minimal light requirement for growth, which is high in Z. marina (~20% of surface irradiance; Dennison et al., 1993). A recent study showed ~90% reduction in biomass under prolonged diminished light conditions, comparable to the decrease in light shown here (Kim et al., 2015). It has been speculated that the high minimum light requirement for growth reflects that seagrasses often grow in anoxic, sulfide-rich sediments (Ralph et al., 2007). The presence of sulfide results in decreased photosynthesis and increased O2 consumption in the dark (Goodman et al., 1995; Holmer and Bondgaard, 2001), which means that more light is needed to drive a sufficient photosynthetic O2 supply to maintain positive growth. Diminished light conditions due to epiphyte cover can thus reduce the fitness of the plant.
The ~4 times enhanced DBL thickness around leaves with epiphyte-cover adversely affected the internal O2 supply to the below-ground tissue at night-time. In addition, it lead to a build-up of O2 at the leaves surface under high incident photon irradiance (≥100 μmol photons m−2 s−1; Figure 5), which potentially could lead to enhanced photorespiration (as surplus internal O2 molecules may bind competitively to RuBisCO instead of CO2 resulting in decreased CO2 fixation and reduced photosynthetic efficiency) and/or internal oxidative stress (Maberly, 2014). At low photon irradiance (50 μmol photons m−2 s−1), the reduced light availability and lower photosynthetic activity, seemed to counter-balance this internal O2 build-up caused by the insulating DBL (Figure 5). Furthermore, the epiphytes themselves, i.e., filamentous algal epiphytes and most probably leaf- and filamentous algal epiphyte-associated bacterial communities, contribute with oxygenic photosynthesis and respiration, thereby further enhancing the O2 consumption at the leaf surface during night-time. Correspondingly, we found a ~2 times higher compensation irradiance of plants with leaf epiphyte-cover, as compared to plants without epiphyte-cover (Figure 6). This may be a very important factor during prolonged events of poor light conditions, such as during dredging operations and eutrophication, making plants with leaf epiphyte-cover more prone to sulfide invasion as a result of inadequate internal aeration (Pedersen et al., 2004; Borum et al., 2005). The generally reduced net photosynthesis rates of plants with epiphyte-cover (Figure 6), was most likely a combined result of the poor light conditions and a limited influx of CO2 from the surrounding water-column. Such DBL-induced limited gas exchange with the ambient water-column can lead to inorganic carbon limitation enhancing photorespiration (e.g., Maberly, 2014) thereby impeding shoot photosynthesis.
Light-driven O2 Microdynamics in the Rhizosphere
Photosynthetic O2 evolution resulted in the establishment of a ~300 μm wide oxic microzone around the root-cap of Z. marina at the approximate position of the apical root meristem (Figure 7). Plants with epiphyte-cover exhibited a negative effect on the below-ground tissue oxidation capacity with ~2 times lower ROL from the root-apex during light stimulation of the leaf canopy, as compared to plants without leaf epiphyte-cover. Although the ROL in light from the root-cap of plants with and without epiphyte-cover were of similar magnitude to fluxes previously reported by Jensen et al. (2005; Table 1), a lower oxidation capability of the below-ground tissue will almost certainly have a negative effect on the overall plants performance. ROL has been shown to improve the chemical conditions in the immediate rhizosphere of seagrasses due to enhanced sulfide reoxidation (Brodersen et al., 2015). The oxic microshield at the root-cap surface can thus protect the apical root meristem from reduced phytotoxic compounds, such as H2S, through chemical re-oxidation with O2.
Dark O2 Microdynamics in the Rhizosphere
During darkness, no O2 was detected at the root-cap surface of plants with leaf epiphyte-cover, indicative of inadequate internal aeration in contrast to plants without leaf epiphyte-cover, where low levels of O2 were detectable at the root-cap surface during darkness (Figure 7; Table 1). Such breakdown of the oxic microshield in presence of epiphytes on seagrass leaves can be of great importance, as a shift to anaerobic metabolism in the root-system results in a much less efficient energy utilization than with aerobic metabolism, as anaerobic conditions inhibit the translocation of carbohydrates supporting plant metabolism (Zimmerman and Alberte, 1996; Greve et al., 2003). Previous studies of Z. marina have shown that the ROL from the root-apex persists during darkness at a much higher flux rate (up to 16.2 nmol O2 cm−2 h−1 measured 2 mm behind the root-apex) than reported in this study (Jensen et al., 2005; Frederiksen and Glud, 2006). This apparent discrepancy may be explained by bacterial colonization of the root-cap surface consuming the small amounts of leaked O2 through microbial respiration and/or by ferrous sulfide (FeS) and iron plaques. Sulfate reducing bacteria have thus previously been isolated from surface-sterilized roots of Z. marina (Nielsen et al., 1999; Finster et al., 2001).
Interestingly, the root-cap mediated O2 leakage to the rhizosphere may also be important for plant-beneficial root-associated microbial processes, such as H2S re-oxidation, in addition to simply detoxifying reduced substances in the immediate rhizosphere through spontaneous chemical reactions. Bacterially-mediated H2S oxidation is about 10.000–100.000 times faster than the chemical reaction alone (Jørgensen and Postgate, 1982) and therefore has potential to be of high value for the plants. It has been suggested that H2S oxidation also takes place inside the plant (Holmer et al., 2005; Holmer and Hasler-Sheetal, 2014), as seagrass exposed to high sediment H2S levels showed internal accumulation of elemental sulfur that is an intermediate in the sulfide oxidation. This process is, however, driven by simple chemical reactions between H2S and O2 and is not mediated by intra-plant enzymes or bacteria (Pedersen et al., 2004) as seen in some marine invertebrates (Grieshaber and Völkel, 1998).
The lower light availability for photosynthesis of plants with filamentous algal epiphyte-cover seemed to be the key factor behind the lower ROL from the root-cap (Figure 7), as a result of the relative lower net photosynthesis rates and thereby lower O2 production in leaves, as compared to plants without epiphyte-cover (Figure 6). This might seem obvious, but the DBL-induced impedance of O2 exchange with the water-column of plants with epiphyte-cover, could also have resulted in an enhancement in the aerenchymal O2 level (seen as the build-up in the surface O2 concentration on Figure 5) and thereby a concomitant higher ROL from the root-apex, but this effect was apparently overruled by lower seagrass photosynthesis due to epiphyte shading and/or inorganic carbon limitation due to increased DBL thickness.
Burnell et al. (2014) recently demonstrated that high incident photon irradiance (~200 μmol photons m−2 s−1) in combination with elevated water-column CO2 concentrations (up to 900 μl L−1, representing future predictions of enhanced water-column CO2 levels) had a negative effect on seagrass biomass and leaf growth, as compared to low light conditions. The observed negative growth response to combined high CO2 and light conditions appeared to be closely related to overgrowth of seagrass leaves with filamentous algal epiphytes. This finding supports our microsensor measurements demonstrating the negative effects of leaf epiphyte-cover on the intra-plant O2 status and the below-ground tissue oxidation capacity. Epiphyte-impeeded O2 evolution in seagrass leaves causing reduced internal aeration and increased H2S intrusion may result in enhanced seagrass mortality if unfavorable light conditions persist for longer periods of time. This emphasizes the importance of minimizing nutrient loading into seagrass inhabited marine coastal waters, as eutrophication often leads to poor light conditions, low water quality, algal blooms and enhanced night-time O2 consumption in the water column.
In conclusion, the present study shows that epiphyte-cover of seagrass leaves leads to reduced oxidation capability of the below-ground tissue, due to a combined result of lower light availability and thicker DBLs around leaves, impeding seagrass photosynthesis. This synergetic negative effect on the plants performance, resulted in a ~2 times higher compensation irradiance in Z. marina leaving epiphyte-covered seagrasses more vulnerable to H2S invasion during prolonged events of poor light conditions in the surrounding water-column. Seagrasses with leaf epiphyte-cover are thus more prone to anthropogenic impacts and activity in coastal environments, as leaf epiphytes reduce their resilience toward environmental disturbances.
Author Contributions
KB, ML, LP, and MK designed the research. KB, ML, and LP conducted the experiments. KB, ML, LP, and MK analyzed the data. KB and ML wrote the manuscript with editorial help from MK. All authors have given approval to the final version of the manuscript. The authors declare no competing financial interest.
Conflict of Interest Statement
The authors declare that the research was conducted in the absence of any commercial or financial relationships that could be construed as a potential conflict of interest.
Acknowledgments
We thank Unisense A/S, the microbiology group at Aarhus University and Johan F. Kraft for providing the microsensor equipment used in this study. We thank Lars F. Rickelt for manufacturing the scalar irradiance microsensors and Peter Ralph (UTS) for financial support of KB. The research was funded by grants from the Augustinus Foundation, P. A. Fiskers Fund and Jorck and Wife's Fund (KB), the Danish Council for Independent Research |Natural Sciences (MK), and the Australian Research Council (ARC LP 110200454) (MK).
Supplementary Material
The Supplementary Material for this article can be found online at: http://journal.frontiersin.org/article/10.3389/fmars.2015.00058
References
Barnabas, A. D. (1996). Casparian band-like structures in the root hypodermis of some aquatic angiosperms. Aquat. Bot. 55, 217–225. doi: 10.1016/S0304-3770(96)01072-8
Binzer, T., Borum, J., and Pedersen, O. (2005). Flow velocity affects internal oxygen conditions in the seagrass Cymodocea nodosa. Aquat. Bot. 83, 239–247. doi: 10.1016/j.aquabot.2005.07.001
Borum, J. (1983). “The quantitative role of macrophytes, epiphytes, and phytoplankton under different nutrient conditions in Roskilde Fjord, Denmark,” in Proceedings of the International Symposium on Aquatic Macrophytes (Nijmegen), 35–40.
Borum, J., Pedersen, O., Greve, T. M., Frankovich, T. A., Zieman, J. C., Fourqurean, J. W., et al. (2005). The potential role of plant oxygen and sulphide dynamics in die-off events of the tropical seagrass, Thalassia testudinum. J. Ecol. 93, 148–158. doi: 10.1111/j.1365-2745.2004.00943.x
Borum, J., Sand-Jensen, K., Binzer, T., Pedersen, O., and Greve, T. (2006). “Oxygen movement in seagrasses,” in Seagrasses: Biology, Ecology and Conservation, eds A. W. D. Larkum, J. R. Orth, and C. M. Duarte (Dordrecht; Berlin: Springer), 255–270.
Brodersen, K. E., Nielsen, D. A., Ralph, P. J., and Kühl, M. (2014). A split flow chamber with artificial sediment to examine the below-ground microenvironment of aquatic macrophytes. Mar. Biol. 161, 2921–2930. doi: 10.1007/s00227-014-2542-3
Brodersen, K. E., Nielsen, D. A., Ralph, P. J., and Kühl, M. (2015). Oxic microshield and local pH enhancement protects Zostera muelleri from sediment derived hydrogen sulphide. New Phytol. 205, 1264–1276. doi: 10.1111/nph.13124
Burkholder, J. M., Tomasko, D. A., and Touchette, B. W. (2007). Seagrasses and eutrophication. J. Exp. Mar. Biol. Ecol. 350, 46–72. doi: 10.1016/j.jembe.2007.06.024
Burnell, O. W., Russell, B. D., Irving, A. D., and Connell, S. D. (2014). Seagrass response to CO2 contingent on epiphytic algae: indirect effects can overwhelm direct effects. Oecologia 176, 871–882. doi: 10.1007/s00442-014-3054-z
Dennison, W. J., Orth, R. J., Moore, K. A., Stevenson, J. C., Carter, V., Kollar, S., et al. (1993). Assessing water quality with submerged aquatic vegetation. Bioscience 43, 86–94. doi: 10.2307/1311969
Drake, L. A., Dobbs, F. C., and Zimmerman, R. C. (2003). Effects of epiphyte load on optical properties and photosynthetic potential of the seagrasses Thalassia testudinum Banks ex König and Zostera marina L. Limnol. Ocenogr. 48, 456–463. doi: 10.4319/lo.2003.48.1_part_2.0456
Duarte, C. M. (1991). Seagrass depth limits. Aquat. Bot. 40, 363–377. doi: 10.1016/0304-3770(91)90081-F
Duarte, C. M. (2001). “Seagrass ecosystems,” in Encyclopedia of Biodiversity, Vol 5, ed S. L. Leven (San Diego, CA: Academic Press), 254–268. doi: 10.1016/B0-12-226865-2/00241-8
Duarte, C. M., Middelburg, J. J., and Caraco, N. (2005). Major role of marine vegetation on the oceanic carbon cycle. Biogeosciences 2, 1–8. doi: 10.5194/bg-2-1-2005
Eghbal, M. A., Pennefather, P. S., and O'Brien, P. J. (2004). H2S cytotoxicity mechanism involves reactive oxygen species formation and mitochondrial depolarisation. Toxicology 203, 69–76. doi: 10.1016/j.tox.2004.05.020
Finster, K., Thomsen, T. R., and Ramsing, N. B. (2001). Desulfomusa hansenii gen. nov., sp. nov., a novel marine propionate-degrading, sulfate-reducing bacterium isolated from Zostera marina roots. Int. J. Syst. Evol. Microbiol. 51, 2055–2061. doi: 10.1099/00207713-51-6-2055
Frederiksen, M. S., and Glud, R. N. (2006). Oxygen dynamics in the rhizosphere of Zostera marina: a two-dimensional planar optode study. Limnol. Oceanogr. 51, 1072–1083. doi: 10.4319/lo.2006.51.2.1072
Frederiksen, M. S., Holmer, M., Borum, J., and Kennedy, H. (2006). Temporal and spatial variation of sulfide invasion in eelgrass (Zostera marina) as reflected by its sulfur isotopic composition. Limnol. Oceanogr. 51, 2308–2318. doi: 10.4319/lo.2006.51.5.2308
Goodman, J. L., Moore, K. A., and Dennison, W. C. (1995). Photosynthetic responses of eelgrass (Zostera marina L.) to light and sediment sulfide in a shallow barrier island lagoon. Aquat. Bot. 50, 37–47. doi: 10.1016/0304-3770(94)00444-Q
Greve, T. M., Borum, J., and Pedersen, O. (2003). Meristematic oxygen variability in eelgrass (Zostera marina). Limnol. Oceanogr. 48, 210–216. doi: 10.4319/lo.2003.48.1.0210
Grieshaber, M. K., and Völkel, S. (1998). Animal adaptations for tolerance and exploitation of poisonous sulfide. Annu. Rev. Physiol. 60, 33–53. doi: 10.1146/annurev.physiol.60.1.33
Holmer, M., and Bondgaard, E. J. (2001). Photosynthetic and growth response of eelgrass to low oxygen and high sulfide concentrations during hypoxic events. Aquat. Bot. 70, 29–38. doi: 10.1016/S0304-3770(00)00142-X
Holmer, M., Frederiksen, M., and Møllegaard, H. (2005). Sulfur accumulation in eelgrass (Zostera marina) and effect of sulfur on eelgrass growth. Aquat. Bot. 81, 367–379. doi: 10.1016/j.aquabot.2004.12.006
Holmer, M., and Hasler-Sheetal, H. (2014). Sulfide intrusion in seagrasses assessed by stable sulfur isotopes – a synthesis of current results. Front. Mar. Sci. 1:64. doi: 10.3389/fmars.2014.00064
Jensen, S. I., Kühl, M., Glud, R. N., Jørgensen, L. B., and Prieme, A. (2005). Oxic microzones and radial oxygen loss from roots of Zostera marina. Mar. Ecol. Prog. Ser. 293, 49–58. doi: 10.3354/meps293049
Jørgensen, B. B. (1982). Mineralization of organic matter in the sea bed - the role of sulfate reduction. Nature 296, 643–645. doi: 10.1038/296643a0
Jørgensen, B. B., and Des Marais, D. J. (1990). The diffusive boundary layer of sediments: oxygen microgradients over a microbial mat. Limnol. Oceanogr. 35, 1343–1355. doi: 10.4319/lo.1990.35.6.1343
Jørgensen, B. B., and Postgate, J. R. (1982). Ecology of the bacteria of the Sulfur cycle with special reference to anoxic oxic interface environments. Philos. Trans. R. Soc. Lond. B Biol. Sci. 298, 543–561. doi: 10.1098/rstb.1982.0096
Jørgensen, B. B., and Revsbech, N. P. (1985). Diffusive boundary layers and the oxygen uptake of sediments and detritus. Limnol. Oceanogr. 30, 111–122. doi: 10.4319/lo.1985.30.1.0111
Kim, Y. K., Kim, S. H., and Lee, K. S. (2015). Seasonal growth responses of the seagrass Zostera marina under severely diminished light conditions. Estuar. Coasts 38, 558–568. doi: 10.1007/s12237-014-9833-2
Koren, K., Brodersen, K. E., Jakobsen, S. L., and Kühl, M. (2015). Optical sensor nanoparticles in artificial sediments – a new tool to visualize O2 dynamics around the rhizome and roots of seagrasses. Environ. Sci. Technol. 49, 2286–2292. doi: 10.1021/es505734b
Kühl, M., and Jørgensen, B. B. (1994). The light-field of microbenthic communities - radiance distribution and microscale optics of sandy coastal sediments. Limnol. Oceanogr. 39, 1368–1398. doi: 10.4319/lo.1994.39.6.1368
Lamers, L. P., Govers, L. L., Janssen, I. C., Geurts, J. J., Van der Welle, M. E., Van Katwijk, M. M., et al. (2013). Sulfide as a soil phytotoxin—a review. Front. Plant Sci. 4:268. doi: 10.3389/fpls.2013.00268
Lassen, C., Ploug, H., and Jørgensen, B. B. (1992). A fiberoptic scalar irradiance microsensor - application for spectral light measurements in sediments. FEMS Microbiol. Ecol. 86, 247–254. doi: 10.1111/j.1574-6968.1992.tb04816.x
Maberly, S. C. (2014). The fitness of the environments of air and water for photosynthesis, growth, reproduction and dispersal of photoautotrophs: an evolutionary and biogeochemical perspective. Aquat. Bot. 118, 4–13. doi: 10.1016/j.aquabot.2014.06.014
Nielsen, J. T., Liesack, W., and Finster, K. (1999). Desulfovibrio zosterae sp. nov., a new sulphate reducer isolated from surface-sterilized roots of the seagrass Zostera marina. Int. J. Syst. Evol. Microbiol. 49, 859–865.
Orth, R. J., Carruthers, T. J., Dennison, W. C., Duarte, C. M., Fourqurean, J. W., Heck, K. L., et al. (2006). A global crisis for seagrass ecosystems. Bioscience 56, 987–996. doi: 10.1641/0006-3568(2006)56[987:AGCFSE]2.0.CO;2
Pedersen, M. F., Nejrup, L. B., Pedersen, T. M., and Frederiksen, S. (2014). Sub canopy light conditions only allow low annual net productivity of epiphytic algae on kelp Laminaria hyperborea. Mar. Ecol. Prog. Ser. 516, 163–176. doi: 10.3354/meps11019
Pedersen, O., Binzer, T., and Borum, J. (2004). Sulphide intrusion in eelgrass (Zostera marina L.). Plant Cell Environ. 27, 595–602. doi: 10.1111/j.1365-3040.2004.01173.x
Pedersen, O., Borum, J., Duarte, C. M., and Fortes, M. D. (1998). Oxygen dynamics in the rhizosphere of Cymodocea rotundata. Mar. Ecol. Prog. Ser. 169, 283–288. doi: 10.3354/meps169283
Pérez-Pérez, M. E., Lemaire, S. D., and Crespo, J. L. (2012). Reactive oxygen species and autophagy in plants and algae. Plant Physiol. 160, 156–164. doi: 10.1104/pp.112.199992
Plus, M., Deslous-Paoli, J.-M., and Dagault, F. (2003). Seagrass (Zostera marina L.) bed recolonisation after anoxia-induced full mortality. Aquat. Bot. 77, 121–134. doi: 10.1016/S0304-3770(03)00089-5
Ralph, P. J., Durako, M. J., Enriquez, S., Collier, C. J., and Doblin, M. A. (2007). Impact of light limitation on seagrasses. J. Exp. Mar. Biol. Ecol. 350, 176–193. doi: 10.1016/j.jembe.2007.06.017
Raun, A. L., and Borum, J. (2013). Combined impact of water column oxygen and temperature on internal oxygen status and growth of Zostera marina seedlings and adult shoots. J. Exp. Mar. Biol. Ecol. 441, 16–22. doi: 10.1016/j.jembe.2013.01.014
Raven, J. A., and Scrimgeour, C. M. (1997). The influence of anoxia on plants of saline habitats with special reference to the Sulfur cycle. Ann. Bot. 79, 79–86. doi: 10.1093/oxfordjournals.aob.a010309
Revsbech, N. P. (1989). An oxygen microsensor with a guard cathode. Limnol. Oceanogr. 34, 474–478. doi: 10.4319/lo.1989.34.2.0474
Richardson, S. L. (2006). Response of epiphytic foraminiferal communities to natural eutrophication in seagrass habitats off Man O'War Cay, Belize. Mar. Ecol. 27, 404–416. doi: 10.1111/j.1439-0485.2006.00096.x
Robblee, M. B., Barber, T. R., Carlson, P. R. Jr., Durako, M. J., Fourqurean, J. W., Muehlstein, L. K., et al. (1991). Mass mortality of the tropical seagrass Thalassia testudinum in Florida Bay (USA). Mar. Ecol. Prog. Ser. 71, 297–299.
Sand-Jensen, K. (1977). Effect of epiphytes on eelgrass photosynthesis. Aquat. Bot. 3, 55–63. doi: 10.1016/0304-3770(77)90004-3
Sand-Jensen, K., and Borum, J. (1991). Interactions among phytoplankton, periphyton, and macrophytes in temperate freshwaters and estuaries. Aquat. Bot. 41, 137–175. doi: 10.1016/0304-3770(91)90042-4
Seddon, S., Connolly, R., and Edyvane, K. S. (2000). Large-scale seagrass dieback in northern Spencer Gulf, South Australia. Aquat. Bot. 66, 297–310. doi: 10.1016/S0304-3770(99)00080-7
Spilling, K., Titelman, J., Greve, T. M., and Kühl, M. (2010). Microsensor measurements of the external and internal microenvironment of Fucus vesiculosus (Phaeophyceae). J. Phycol. 46, 1350–1355. doi: 10.1111/j.1529-8817.2010.00894.x
Steen-Knudsen, O. (2002). Biological Membranes: Theory of Transport, Potentials and Electric Impulses. Cambridge, UK: Cambridge University Press.
Webb, W. L., Newton, M., and Starr, D. (1974). Carbon-dioxide exchange of Alnus-rubra - mathematical-model. Oecologia 17, 281–291. doi: 10.1007/BF00345747
Zieman, J. C., Fourqurean, J. W., and Frankovich, T. A. (1999). Seagrass die-off in Florida Bay: long-term trends in abundance and growth of turtle grass, Thalassia testudinum. Estuaries 22, 460–470. doi: 10.2307/1353211
Keywords: epiphyte-cover, light, diffusive boundary layer, radial O2 loss, oxic microshield, microenvironment
Citation: Brodersen KE, Lichtenberg M, Paz L-C and Kühl M (2015) Epiphyte-cover on seagrass (Zostera marina L.) leaves impedes plant performance and radial O2 loss from the below-ground tissue. Front. Mar. Sci. 2:58. doi: 10.3389/fmars.2015.00058
Received: 15 May 2015; Accepted: 03 August 2015;
Published: 20 August 2015.
Edited by:
Christos Dimitrios Arvanitidis, Hellenic Centre for Marine Research, GreeceReviewed by:
Jose M. Riascos, Universidad de Antofagasta, ChileEugenia T. Apostolaki, Hellenic Centre for Marine Research, Greece
Copyright © 2015 Brodersen, Lichtenberg, Paz and Kühl. This is an open-access article distributed under the terms of the Creative Commons Attribution License (CC BY). The use, distribution or reproduction in other forums is permitted, provided the original author(s) or licensor are credited and that the original publication in this journal is cited, in accordance with accepted academic practice. No use, distribution or reproduction is permitted which does not comply with these terms.
*Correspondence: Kasper E. Brodersen, Plant Functional Biology and Climate Change Cluster (C3), Faculty of Science, University of Technology Sydney, PO Box 123, Broadway, NSW 2007, Australia, kasper.e.brodersen@student.uts.edu.au; Website: elgetti.wordpress.com
†These authors have contributed equally to this work.