- 1Key Laboratory of Coastal Zone Environmental Processes and Ecological Remediation, Yantai Institute of Coastal Zone Research, Chinese Academy of Sciences, Yantai, China
- 2Department of Marine Chemistry, National Marine Environmental Monitoring Center, Dalian, China
Both offshore oil-gas exploration and marine methane hydrate recovery can trigger massive CH4 release from seafloor. During upward transportation of CH4 plume through water column, CH4 is subjected to dissolution and microbial consumption despite the protection of hydrate and oil coating on bubbles surface. The ultimate CH4 degassing to the atmosphere appears to be water-depth dependent. In shallow oceans with water depth less than 100 m, the natural or human-induced leakages or both lead to significant sea-to-air CH4 degassing from 3.00 to 1.36 × 105 μ mol m−2 d−1. To quantify the human-perturbation induced CH4 degassing, the combination of top-down modeling and bottom-up calculations is essential due to spatial and temporal variability of diffusion and ebullition at water-air interface.
Introduction
Methane (CH4) is an infrared-active trace gas that plays an important role in Earth's climate system (Lelieveld et al., 1998). Despite its low mole fraction in the atmosphere (1.803 ppm in 2011), CH4 is the second largest contributor (17%, after carbon dioxide) to the total radiative forcing caused by the well-mixed greenhouse gases (IPCC, 2013). The continuous increase of atmospheric CH4 in the past decades implies an imbalance between CH4 sources and sinks and prompts urgent questions about the causes (Cicerone and Oremland, 1988; Kirschke et al., 2013; Nisbet et al., 2014).
Although earlier researchers suggest that natural emissions of CH4 from oceans may only contribute 1% to the global atmospheric methane budget (Bange et al., 1994; Judd et al., 2002; U.S. EPA, 2012), recently the oceanic CH4 release has received increasing attention under the context of global warming induced Arctic changes (e.g., Whiteman et al., 2013). So far, understanding the maintaining mechanism and variability of the sea-to-air flux of CH4 remains a huge challenge since most potential source areas of CH4 are still insufficiently sampled in global oceans (Achterberg, 2014). It is worth noting that marine sediments serve as the global largest reservoir of CH4 (Kvenvolden, 2002; Milkov, 2004). If a substantial amount of CH4 were released from seafloor, the atmospheric CH4 concentration would rise dramatically.
As strong anthropogenic perturbations, offshore oil-gas explorations and marine methane hydrate explorations may play an important role in triggering the CH4 release from seafloor. The well sites, processing plants, storage tanks, transmission compressor stations, and distribution systems may act as “super-emitter” CH4 sources, which have been considered to be a cause for larger CH4 emissions from North American natural gas systems than official estimates (Brandt et al., 2014).
Nowadays preventing CH4 release from vented and flared natural gas has received considerable attention (e.g., Elvidge et al., 2009; U.S. GAO, 2010), while the CH4 degassing associated with episodic CH4 leakage from seafloor is also identified (e.g., Du et al., 2014; Zhang et al., 2014). This mini review summarized recent progresses regarding the CH4 leakage from seafloor caused by offshore oil-gas and marine methane hydrate explorations and its degassing to the atmosphere.
Offshore Oil-Gas Exploration and CH4 Leakage
To meet energy demands in both developed and emerging economies, the offshore oil and gas exploration has received international attentions in recent decades. In 2012, the offshore discovery accounted for 90% of world newly increased reserves (Zhao et al., 2014). The newly discovered oil-gas reservoirs concentrated in the coastal shallow waters (Figure 1). In addition to offshore boom in the Gulf of Mexico, substantial exploration and development has taken place along coasts of Brazil and the Africa. Interests in the oil-gas exploration in the more challenging Arctic area are also increasing.
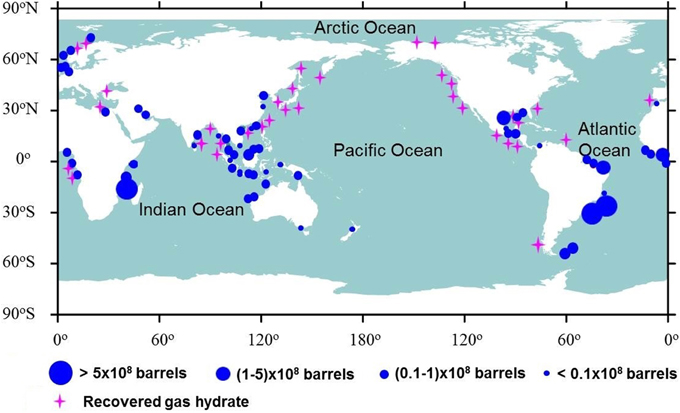
Figure 1. The global new discoveries of offshore oil-gas exploration (Zhao et al., 2014) and an inventory of marine methane hydrate deposits (Ruppel and Noserale, 2012). Purple star symbols show the gas hydrates that have been discovered, while blue dots show those offshore oil-gas discoveries in 2012.
Oil and gas can enter the marine environment by natural seepage and/or by leaks and spills caused by human activities (extraction, transportation, etc.). The natural crude-oil seeps scatter in global coastal seas and the amount of global seepage is estimated to be 600,000 tons per year (Kvenvolden and Cooper, 2003). Natural gas seepage has been considered to be the cause of strong subsurface CH4 anomaly in the Davis Strait, North Atlantic Ocean (Punshon et al., 2014).
Compared to natural seepage, marine oil and gas extraction can cause disastrous oil spills and leakages accompanied by massive CH4 injections into the overlying water column. Some “landmark” accidents include Ekofish B blowout in the North Sea in 1977, Ixtoc I blowout in the Gulf of Mexico in 1979, Adriatic IV blowout in the Mediterranean Sea in 2004, Montara blowout in the Timor Sea in 2009, and Macondo blowout in the Gulf of Mexico in 2010 (Christou and Konstantinidou, 2012). Additionally, CH4 leakage may occur at any subsea facility, such as the pipeline, the flange, the valve and the weld (Vrålstad et al., 2011). CH4 emissions from oil and gas systems (including coastal and offshore) were estimated to be 23% of total global anthropogenic emissions in 2010 and they are expected to grow 26% from 2010 to 2030 (U. S. EPA, 2012).
Undoubtedly, reducing CH4 emission from oil and gas systems has environmental and economic benefits, especially in the Arctic. This issue has been seriously considered by some national governments and non-governmental organizations (e.g., the Global Methane Initiative, https://www.globalmethane.org/).
Marine Methane Hydrate Exploration and Potential CH4 Leakage
Huge quantities of CH4 are stored in continental margins in the form of methane hydrates under a delicate balance of low temperature (around 0°C) and high hydrostatic pressure (a few MPa) (Sloan and Koh, 2008). Methane hydrate (also called gas hydrate) is an ice-like crystalline form of water and low-molecular-weight gas (mainly CH4) with a nominal composition of (CH4)4(H2O)23. One cubic meter of fully saturated methane hydrate solid contains ~164 m3 of CH4 gas at the standard temperature and pressure (Kvenvolden, 1993).
The methane hydrate has been found on nearly all major continental shelves (Figure 1). Its reserves are estimated to be 3000–4000 times today's atmospheric CH4 reservoir (MacDonald, 1990; Blunier, 2000) or twice the existing reserves of all fossil fuels including coal, oil and natural gas (Kvenvolden, 1993, 2002).
Interest in methane hydrate has been growing rapidly since global resources of conventional oil and gas are on the decline and methane hydrate has emerged as a potential resource to make up the expected shortfall of conventional oil and gas (Kvenvolden, 1993; Makogon et al., 2007; Makogon, 2010). The Gas Hydrate Joint Industry Project in the Gulf of Mexico has confirmed the occurrence of methane hydrates below the seafloor of Gulf of Mexico (Boswell et al., 2012). In early 2012, the U.S. and Japan completed a successful field trial of methane hydrate production technologies in the North Slope of Alaska (http://energy.gov/). In March 2013, Japan's Methane Hydrate R&D Program conducted an experimental operation and succeeded to produce a steady CH4 flow southeast of the Atsumi peninsula, Japan (http://www.jogmec.go.jp/). Other earlier global drilling efforts and production test studies were summarized by Ruppel (2011). However, so far no large-scale commercial CH4 production from methane hydrate deposits has been reported due to scientific and technical challenges and economic viability.
Methane hydrate may serve as an important factor affecting global climate change because they are unstable and subject to dissociation due to slight temperature or pressure change, causing catastrophic seafloor failure and massive (gigaton scale) CH4 release into overlying ocean-atmosphere system (Kennett et al., 2000; Paull et al., 2003). The release of large volumes of CH4 to the atmosphere could in theory aggravate climate warming and trigger more methane hydrates to destabilize, creating a positive feedback loop. The positive interaction between climate and methane hydrate has been considered to be a cause in triggering the Palaeogene hyperthermal events, an abrupt period of global warming (4–8°C temperature rise) between 57 and 50 million years ago (Dickens et al., 1995; Kaiho et al., 1996; Gu et al., 2011) and the Late Quaternary (400,000–10,000 years ago) climate change (Kennett et al., 2003).
Without anthropogenic perturbation, most of the world's methane hydrate deposits should remain stable for the next few thousand years (Ruppel and Noserale, 2012). However, marine methane hydrate dissociation in response to ocean warming has been reported in many coastal seas, including the offshore Costa Rica (Crutchley et al., 2014), the offshore southwestern Japan (Bangs et al., 2010), the south Kara Sea shelf (Portnov et al., 2013), the northern U.S. Atlantic margin (Skarke et al., 2014), the offshore Svalbard (Westbrook et al., 2009; Marín-Moreno et al., 2013; Berndt et al., 2014) and sub-sea permafrost-associated methane hydrates in the east Siberian Arctic shelf (Shakhova et al., 2010). U.S. EPA (2012) suggested that the increase of CH4 leakage from methane hydrate due to ocean warming may have overcome the barrier of water column and resulted in a significant atmospheric CH4 load. How to evaluate the risks of the future commercial exploitation induced submarine geohazards and the consequently massive CH4 release remains an open question.
Behavior of Seafloor-Released CH4
The seafloor-released CH4 will migrate upward through the water column either as dissolved CH4 or as bubble CH4. The rising CH4 plume in the water column tends to get weak since some CH4 are subject to dissolution and microbially-mediated aerobic and anaerobic oxidation (Greinert et al., 2006; Reeburgh, 2007; Römer et al., 2012). The remaining fraction can reach atmosphere through diffusion or ebullition, which is determined by water depth, stratification, and microbiological processes within the water column (Schmale et al., 2005; Mau et al., 2007). Clarifying the behavior of CH4 in the water column is critical to constrain the hazard potential of offshore drilling activities.
For CH4 bubbles emanated from seafloor, the upward migration and dissolution of CH4 is highly dependent on hydrate or oil coverage on bubbles surface. Both field and lab experiments have demonstrated that CH4 bubbles are likely to be coated by methane hydrate within the local methane hydrate stable field (MHSF), which can significantly decrease but does not halt CH4 dissolution (Rehder et al., 2002, 2009; Warzinski et al., 2014). The bubbles usually dissolve rapidly at the upper boundary of the local MHSF, which is jointly determined by water temperature, salinity, and gas composition (Römer et al., 2014). When CH4 spills are accompanied by oil, oil coating around rising bubbles also impedes dissolution, thereby enhancing the likelihood of CH4 release to the atmosphere (De Beukelaer et al., 2003; Leifer and MacDonald, 2003; Körber et al., 2014).
In deep waters, the scenario is outlined by the Deepwater Horizon oil spill event. From 20 April to 15 July 2010, 9.14 × 109 to 1.25 × 1010 moles of CH4 were injected into deep waters (~1500 m) of Gulf of Mexico (Kessler et al., 2011). However, only a few (less than 0.01%) of the seafloor-released CH4 escaped into atmosphere (Yvon-Lewis et al., 2011). The CH4 plume was trapped below 800 m depth with CH4 concentrations roughly 20–50 times as high as background levels and the microbial CH4 oxidation rate quantified to be 10 nmol d−1 (the median value) therein (Valentine et al., 2010). The trapped CH4 was consumed by methanotrophic bacteria within ~120 days from the onset of release, resulting in significant oxygen anomaly in deep waters (Valentine et al., 2010; Kessler et al., 2011). By comparing the amounts of CH4 microbial consumed and degassed to the atmosphere, Kessler et al. (2011) suggested that methanotrophic bacterial communities act as a dynamic biofilter that respond quickly and efficiently to the seafloor CH4 leakage.
In the waters with depth around the upper boundary of local MHSZ, no oil-gas spill event has been reported so the scenario can only be described by some simulated experiments. A gas blowout experiment was conducted in the Norwegian Sea in 2000, during which ~4 × 105 mole of CH4 was discharged into the water column of 844 m depth. Since no methane hydrates shell was formed around gas bubbles, the gas dissolved quickly in the water column and no gas bubble was observed at sea surface (Johansen et al., 2003). Schmale et al. (2011) assumed a massive short-term injection of CH4 release (1.1 × 1010 mole) at depth of 700 m in the Black Sea. They modeled that CH4 was effectively buffered by microbial consumption and hammered by water column stratification. The simulated CH4 release in depth resulted in only a 2–3% increase in the sea-to-air flux.
The shallow-ocean CH4 leakage presents a quite different scenario. By studying oil spills in the Bohai Sea (China) with water depth less than 30 m, Zhang et al. (2014) revealed that the seafloor-released CH4 can break through the summer stratification, increasing sea surface CH4 concentration by up to 4.7 times and enhancing local CH4 outgassing by up to 14.6 times. However, the function of methanotrophs in this shallow sea is still unknown.
According to McGinnis et al. (2006), most marine sources of the atmospheric CH4 are located in shallow oceans with water depth less than 100 m. This is coincident with the surface-water CH4 distribution in the Black Sea, where elevated CH4 concentrations were only observed above seeps areas with depth <100 m and no significant imprint was observed above high-intensity seeps with water column deeper than 150 m (Schmale et al., 2005). Summarizing sea-to-air CH4 fluxes from marine environments affected by oil-gas exploration and methane hydrate dissociation (Table 1), we can also find that shallow areas (depth of leakage < 100 m) present intensive CH4 fluxes of 3.00–1.36 × 105 μ mol m−2 d−1, whereas deeper areas show weaker values of −3.65–800 μmol m−2 d−1. It is worthy of noting that Solomon et al. (2009) observed that intensive CH4 bubble plumes ascend from depth 550–600 m to sea surface in the Gulf of Mexico, leading to intensive degassing (up to 104 μ mol m−2 d−1). So water depth may not serve as the only threshold of the CH4 degassing.
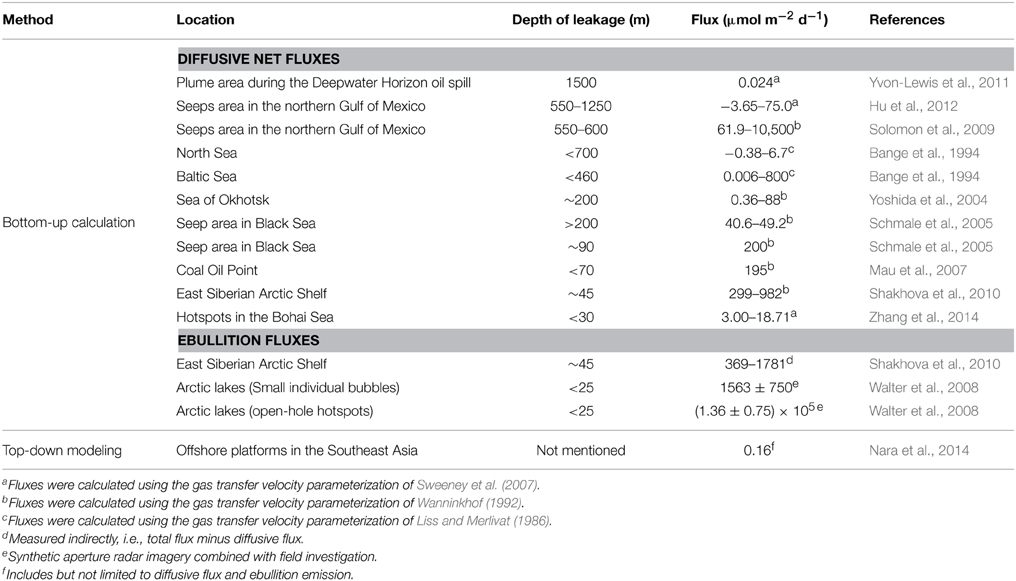
Table 1. Sea-to-air CH4 fluxes from marine environments affected by oil-gas exploration and methane hydrate dissociation.
Quantifying CH4 Degassing
Leakage of CH4 from seafloor triggered by offshore explorations may contribute directly to atmospheric CH4 budgets and possibly accelerate global warming (Jiang et al., 2006; Biastoch et al., 2011). However, so far the global or regional sea-to-air CH4 fluxes are poorly constrained, leading to uncertainties in carbon cycle and climate models.
The approaches to quantify CH4 degassing generally fall into two categories: “bottom-up” calculations and “top-down” modeling (also known as inverse modeling). The bottom-up estimation of the CH4 leakage rate is based on summing up emissions from different types of known sources (Olivier, 2002). CH4 can enter the atmosphere by diffusion of dissolved CH4 across the water-air interface or by direct migration of bubbles (if still contains CH4). As for diffusion, the sea-air flux is estimated using a stagnant laminar layer model proposed by Liss and Slater (1974). Its reliability lies not only on gas transfer velocity and hydrodynamic conditions (Wanninkhof et al., 2009; Johnson et al., 2011), but also on the spatial resolution of sample collections. The surface CH4 emission hot spots (i.e., areas of high flux) need to be covered (Du et al., 2014; Zhang et al., 2014). Compared to diffusion, ebullition of CH4-containing bubbles, especially a small fraction of the largest bubbles, may release larger amounts of CH4 and exhibits much greater spatiotemporal variation (Greinert and Nützel, 2004; McGinnis et al., 2014; Xiao et al., 2014; DelSontro et al., 2015). Floating chamber method has been widely used for direct measurement of both diffusive and ebullitions fluxes at water-air interface, but mostly in lakes and estuaries. Its utilization at sea is greatly challenged due to insufficient temporal and spatial coverage. At extreme shallow coastal waters (<5 m), a submerged chamber device has been developed for in-situ and on-line measurement of CH4 flux from seafloor (Di et al., 2014). In the Arctic regions, synthetic aperture radar imagery has been used to locate point-source CH4 ebullition from ice-covered lakes (Walter et al., 2008). Some numerical models have also been developed to quantify direct bubble transport of CH4 (Leifer and Patro, 2002; McGinnis et al., 2006; Yamamoto et al., 2009). Yet more efforts are needed to better quantify CH4 ebullition flux to the atmosphere on the spot, especially at ice-free seas.
Top-down modeling is based on atmospheric CH4 measurements, atmospheric models, and statistical tools to invert concentration fields into parameters that provide the estimation of CH4 emissions and sinks (Olivier, 2002). The remote-sensing-based top-down approach has been used to quantify CH4 emission fluxes from the North American inland natural gas sites (Kort et al., 2014; Schneising et al., 2014). So far only a few similar investigations are designed to constrain CH4 emissions from offshore oil-gas production. For example, Nara et al. (2014) have estimated CH4 emission rates from offshore oil and gas platforms in the Southeast Asia based on their observed atmospheric CH4 peaks and a mass balance approach. Similar atmospheric CH4 peaks were also revealed at two regional atmospheric background monitoring sites adjacent to the Bohai Sea shortly after an oil-spill accident in June–August 2011 (Zhang et al., 2014). The field-data-based top-down approach is worth to be seriously applied.
Satellite observations are able to monitor gas emissions down to the point-source scale (Velazco et al., 2011). Airborne imaging spectrometry has the potential to provide high resolution mapping of marine CH4 emissions from point sources (Thorpe et al., 2013). So time-resolved satellite observation supplemented by aircraft and ground-based measurements may provide a feasible method to quantify the large scale CH4 emission from offshore oil-gas exploration areas.
According to the limited literature data that have already been summarized in Table 1, ebullition fluxes generally show much higher values than diffusive ones and both of them show great spatial variation. However, so far ebullition measurements are seldom conducted at sea, probably due to their episodic occurrence and lack of method, especially at ice-free seas. Inverse modeling observations are even fewer. Therefore, the current investigations from a few scattered sites are quite insufficient to obtain a global estimation of the CH4 emission triggered by offshore oil-gas and methane hydrate explorations.
Summary
Massive CH4 leakage from seafloor can be triggered by both offshore oil-gas explorations and marine methane hydrate recovery. Both field investigations and model results showed that CH4 leakage from seafloor, especially from shallow seafloor with depth less than 100 m, may have affected the atmospheric CH4 budget. The shallow-ocean CH4 degassing should be considered in the policy-making on the greenhouse gas mitigation and pollution abatement. While the potential consequence of possible massive CH4 release due to commercial methane hydrate exploration in the near future is still unknown and should be fully considered.
To quantify the oceanic CH4 degassing regionally or globally, the combination of “bottom-up” calculation and “top-down” modeling is recommended. However, field monitoring approaches still need to be developed to evaluate CH4 ebullition fluxes at sea.
Conflict of Interest Statement
The authors declare that the research was conducted in the absence of any commercial or financial relationships that could be construed as a potential conflict of interest.
Acknowledgments
This research was jointly supported by Key Laboratory for Ecological Environment in Coastal Areas, State Oceanic Administration of China (contract: 201203) and by National Natural Science Foundation of China (grant 41006040).
References
Achterberg, E. P. (2014). Grand challenges in marine biogeochemistry. Front. Mar. Sci. 1:7. doi: 10.3389/fmars.2014.00007
Bange, H. W., Bartell, U. H., Rapsomanikis, S., and Andreae, M. O. (1994). Methane in the Baltic and North Seas and a reassessment of the marine emissions of methane. Global Biogeochem. Cycles 8, 465–480. doi: 10.1029/94GB02181
Bangs, N. L., Hornbach, M. J., Moore, G. F., and Park, J. O. (2010). Massive methane release triggered by seafloor erosion offshore southwestern Japan. Geology 38, 1019–1022. doi: 10.1130/G31491.1
Berndt, C., Feseker, T., Treude, T., Krastel, S., Lievetrau, V., Niemann, H., et al. (2014). Temporal constraints on hydrate-controlled methane seepage off Svalbard. Science 343, 284–287. doi: 10.1126/science.1246298
Biastoch, A., Treude, T., Rupke, L. H., Riebesell, U., Roth, C., Burwicz, E. B., et al. (2011). Rising Arctic Ocean temperatures cause gas hydrate destabilization and ocean acidification. Geophys. Res. Lett. 38, L08602. doi: 10.1029/2011GL047222
Blunier, T. (2000). “Frozen” methane escapes from the sea floor. Science 288, 68–69. doi: 10.1126/science.288.5463.68
Boswell, R., Collett, T. S., Frye, M., Shedd, W., McConnell, D. R., and Shelander, D. (2012). Subsurface gas hydrates in the northern Gulf of Mexico. Mar. Petrol. Geol. 34, 4–30. doi: 10.1016/j.marpetgeo.2011.10.003
Brandt, A. R., Heath, G. A., Kort, E. A., O'Sullivan, F., Pétron, G., Jordaan, S. M., et al. (2014). Methane leaks from North American natural gas systems. Science 343, 733–735. doi: 10.1126/science.1247045
Christou, M., and Konstantinidou, M. (2012). Safety of Offshore Oil and Gas Operations: Lessons from Past Accident Analysis: Ensuring EU Hydrocarbon Supply Through Better Control of Major Hazards. Publication JRC77767. Joint Research Centre, European Commission.
Cicerone, R. J., and Oremland, R. S. (1988). Biogeochemical aspects of atmospheric methane. Global Biogeochem. Cycles 2, 299–327. doi: 10.1029/GB002i004p00299
Crutchley, G. J., Klaeschenb, D., Planertb, L., Bialasb, J., Berndtb, C., Papenbergb, C., et al. (2014). The impact of fluid advection on gas hydrate stability: investigations at sites of methane seepage offshore Costa Rica. Earth Planet. Sci. Lett. 401, 95–109. doi: 10.1016/j.epsl.2014.05.045
De Beukelaer, S. M., MacDonald, I. R., Guinnasso, N. L., and Murray, J. A. (2003). Distinct side-scan sonar, RADARSAT SAR, and acoustic profiler signatures of gas and oil seeps on the Gulf of Mexico slope. Geo Mar. Lett. 23, 177–186. doi: 10.1007/s00367-003-0139-9
DelSontro, T., McGinnis, D. F., Wehrli, B., and Ostrovsky, I. (2015). Size does matter: importance of large bubbles and small-scale hot spots for methane transport. Environ. Sci. Technol. 49, 1268–1276. doi: 10.1021/es5054286
Di, P. F., Dong, F., and Chen, D. F. (2014). In-situ and on-line measurement of gas flux at a hydrocarbon seep from the northern South China Sea. Cont. Shelf Res. 81, 80–87. doi: 10.1016/j.csr.2014.04.001
Dickens, G. R., O'Neil, J. R., Rea, D. K., and Owen, R. M. (1995). Dissociation of oceanic methane hydrate as a cause of the carbon isotope excursion at the end of the Paleocene. Paleoceanography 10, 965–971. doi: 10.1029/95PA02087
Du, M., Yvon-Lewis, S., Garcia-Tigreros, F., Valentine, D. L., Mendes, S. D., and Kessler, J. D. (2014). High resolution measurements of methane and carbon dioxide in surface waters over a natural seep reveal dynamics of dissolved phase air-sea flux. Environ. Sci. Technol. 48, 10165–10173. doi: 10.1021/es5017813
Elvidge, C. D., Ziskin, D., Baugh, K. E., Tuttle, B. T., Ghosh, T., Pack, D. W., et al. (2009). A fifteen year record of global natural gas flaring derived from satellite data. Energies 2, 595–622. doi: 10.3390/en20300595
Greinert, J., Artemov, Y., Egorov, V., Batist, M. D., and McGinnis, D. (2006). 1300-m-high rising bubbles from mud volcanoes at 2080 m in the Black Sea: hydroacoustic characteristics and temporal variability. Earth Planet. Sci. Lett. 244, 1–15. doi: 10.1016/j.epsl.2006.02.011
Greinert, J., and Nützel, B. (2004). Hydroacoustic experiments to establish a method for the determination of methane bubble fluxes at cold seeps. Geo Mar. Lett. 24, 75-85. doi: 10.1007/s00367-003-0165-7
Gu, G. S., Dickens, G. R., Bhatnagar, G., Colwell, F. S., Hirasaki, G. J., and Chapman, W. G. (2011). Abundant Early Palaeogene marine gas hydrates despite warm deep-ocean temperatures. Nat. Geosci. 4, 848–851. doi: 10.1038/ngeo1301
Hu, L., Yvon-Lewis, S. A., Kessler, J. D., and MacDonald, I. R. (2012). Methane fluxes to the atmosphere from deepwater hydrocarbon seeps in the northern Gulf of Mexico, J. Geophys. Res. 117, C01009. doi: 10.1029/2011JC007208
IPCC. (2013). “Climate change 2013: the physical science basis,” in Contribution of Working Group I to the Fifth Assessment Report of the Intergovernmental Panel on Climate Change. Cambridge: Cambridge University Press.
Jiang, G. Q., Shi, X. Y., and Zhang, S. H. (2006). Methane seeps, methane hydrate destabilization, and the late Neoproterozoic postglacial cap carbonates. Chin. Sci. Bull. 51, 1152–1173. doi: 10.1007/s11434-006-1152-y
Johansen, Ø., Rye, H., and Cooper, C. (2003). DeepSpill—Field study of a simulated oil and gas blowout in deep water. Spill Sci. Technol. B 8, 433–443. doi: 10.1016/S1353-2561(02)00123-8
Johnson, M. T., Hughes, C., Bell, T. G., and Liss, P. S. (2011). “A Rumsfeldian analysis of uncertainty in air-sea gas exchange,” in Gas Transfer at Water Surfaces 2010, eds S. Komori, W. McGillis, and R. Kurose (Kyoto: Kyoto University Press), 464–485.
Judd, A. G., Hovland, M., Dimitrov, L. I., García, G., and Jukesn, V. (2002). The geological methane budget at continental margins and its influence on climate change, Geofluids 2, 109–126. doi: 10.1046/j.1468-8123.2002.00027.x
Kaiho, K., Arinobu, T., Ishiwatari, R., Morgans, H. E. G., Okada, H., Takeda, N., et al. (1996). Latest Paleocene benthic foraminiferal extinction and environmental changes at Tawanui, New Zealand. Paleoceanography 11, 447–465. doi: 10.1029/96PA01021
Kennett, J. P., Cannariato, K. G., Hendy, I. L., and Behl, R. J. (2000). Carbon isotopic evidence for methane hydrate instability during Quaternary interstadials. Science 288, 128–133. doi: 10.1126/science.288.5463.128
Kennett, J. P., Cannariato, K. G., Hendy, I. L., and Behl, R. J. (2003). Methane Hydrates in Quaternary Climate Change: The Clathrate Gun Hypothesis. Washington, DC: American Geophysical Union.
Kessler, J. D., Valentine, D. L., Redmond, M. C., Du, M., Chan, E. W., Mendes, S. D., et al. (2011). A persistent oxygen anomaly reveals the fate of spilled methane in the deep Gulf of Mexico. Science 331, 312–315. doi: 10.1126/science.1199697
Kirschke, S., Bousquet, P., Ciais, P., Saunois, M., Canadell, J. G., Dlugokencky, E. J., et al. (2013). Three decades of global methane sources and sinks. Nat. Geosci. 6, 813–823. doi: 10.1038/ngeo1955
Körber, J. H., Sahling, H., Pape, T., dos Santos, F. C., MacDonald, I. R., and Bohrmann, G. (2014). Natural oil seepage at Kobuleti Ridge, eastern Black Sea. Mar. Petrol. Geol. 50, 68–82. doi: 10.1016/j.marpetgeo.2013.11.007
Kort, E. A., Frankenberg, C., Costigan, K. R., Lindenmaier, R., Dubey, M. K., and Wunch, D. (2014). Four corners: the largest US methane anomaly viewed from space, Geophys. Res. Lett. 41, 6898–6903. doi: 10.1002/2014GL061503
Kvenvolden, K. A. (1993). Gas hydrates: geological perspective and global change. Rev. Geophy. 31, 173–187. doi: 10.1029/93RG00268
Kvenvolden, K. A. (2002). Methane hydrate in the global organic carbon cycle. Terra. Nova. 14, 302–306. doi: 10.1046/j.1365-3121.2002.00414.x
Kvenvolden, K. A., and Cooper, C. K. (2003). Natural seepage of crude oil into the marine environment. Geo Mar. Lett. 23, 140–146. doi: 10.1007/s00367-003-0135-0
Leifer, I., and MacDonald, I. (2003). Dynamics of the gas flux from shallow gas hydrate deposits: interaction between oily hydrate bubbles and the oceanic environment. Earth Planet. Sci. Lett. 210, 411–424. doi: 10.1016/S0012-821X(03)00173-0
Leifer, I., and Patro, R. K. (2002). The bubble mechanism for methane transport from the shallow sea bed to the surface: a review and sensitivity study, Cont. Shelf Res. 22, 2409–2428. doi: 10.1016/S0278-4343(02)00065-1
Lelieveld, J., Crutzen, P. J., and Dentener, F. J. (1998). Changing concentration, lifetime and climate forcing of atmospheric methane. Tellus B Chem. Phys. Meteorol. 50, 128–150. doi: 10.1034/j.1600-0889.1998.t01-1-00002.x
Liss, P. S., and Merlivat, L. (1986). “Air-sea gas exchange rates: introduction and synthesis,” in The Role of Air-Sea Exchange in Geochemical Cycling, ed P. Buat-Menard (New York, NY: Springer), 113–127.
Liss, P. S., and Slater, P. G. (1974). Flux of gases across the air-sea interface. Nature 247, 181–184. doi: 10.1038/247181a0
MacDonald, G. J. (1990). Role of methane clathrates in past and future climates. Clim. Chang. 16, 247–281. doi: 10.1007/BF00144504
Makogon, Y. F. (2010). Natural gas hydrates—a promising source of energy. J. Nat. Gas Sci. Eng. 2, 49–59. doi: 10.1016/j.jngse.2009.12.004
Makogon, Y. F., Holditch, S. A., and Makogon, T. Y. (2007). Natural gas-hydrates—A potential energy source for the 21st century. J. Petrol. Sci. Eng. 56, 14–31. doi: 10.1016/j.petrol.2005.10.009
Marín-Moreno, H., Minshull, T. A., Westbrook, G. K., Sinha, B., and Sarkar, S. (2013). The response of methane hydrate beneath the seabed offshore Svalbard to ocean warming during the next three centuries. Geophys. Res. Lett. 40, 5159–5163. doi: 10.1002/grl.50985
Mau, S., Valentine, D., Clark, J., Reed, J., Camilli, R., and Washburn, L. (2007). Dissolved methane distributions and air-sea flux in the plume of a massive seep field, Coal Oil Point, California. Geophys. Res. Lett. 34, L22603. doi: 10.1029/2007GL031344
McGinnis, D. F., Greinert, J., Artemov, Y., Beaubien, S. E., and Wüest, A. (2006). Fate of rising methane bubbles in stratified waters: how much methane reaches the atmosphere? J. Geophys. Res. 111, C09007. doi: 10.1029/2005JC003183
McGinnis, D. F., Kirillin, G., Tang, K. W., Flury, S., Bodmer, P., Engelhardt, C., et al. (2014). Enhancing surface methane fluxes from an oligotrophic lake: exploring the microbubble hypothesis. Environ. Sci. Technol. 49, 873–880. doi: 10.1021/es503385d
Milkov, A. V. (2004). Global estimates of hydrate-bound gas in marine sediments: how much is really out there? Earth Sci. Rev. 66, 183–197. doi: 10.1016/j.earscirev.2003.11.002
Nara, H., Tanimoto, H., Tohjima, Y., Mukai, H., Nojiri, Y., and Machida, T. (2014). Emissions of methane from off shore oil and gas platforms in Southeast Asia. Sci. Rep. 4:6503. doi: 10.1038/srep06503
Nisbet, E. G., Dlugokencky, E. J., and Bousquet, P. (2014). Methane on the rise—Again. Science 343, 493–495. doi: 10.1126/science.1247828
Olivier, J. G. J. (2002). On the Quality of Global Emission Inventories: Approaches, Methodologies, Input Data, and Uncertainties. Ph.D. thesis, Utrecht University, Wilco BV, Amersfoort.
Paull, C. K., Brewer, P. G., Ussler, W., Peltzer, E. T., Rehder, G., and Clague, D. (2003). An experiment demonstrating that marine slumping is a mechanism to transfer methane from seafloor gas-hydrate deposits into the upper ocean and atmosphere. Geo Mar. Lett. 22, 198–203. doi: 10.1007/s00367-002-0113-y
Portnov, A., Smith, A. J., Mienert, J., Cherkashov, G., Rekant, P., Semenov, P., et al. (2013). Offshore permafrost decay and massive seabed methane escape in water depths > 20m at the South Kara Sea shelf. Geophys. Res. Lett. 40, 3962–3967. doi: 10.1002/grl.50735
Punshon, S., Azetsu-Scott, K., and Lee, C. M. (2014). On the distribution of dissolved methane in Davis Strait, North Atlantic Ocean. Mar. Chem. 161, 20–25. doi: 10.1016/j.marchem.2014.02.004
Reeburgh, W. S. (2007). Oceanic methane biogeochemistry. Chem. Rev. 107, 486–513. doi: 10.1021/cr050362v
Rehder, G., Brewer, P. G., Peltzer, E. T., and Friederich, G. (2002). Enhanced lifetime of methane bubble streams within the deep ocean. Geophys. Res. Lett. 29, 1731. doi: 10.1029/2001GL013966
Rehder, G., Leifer, I., Brewer, P. G., Friederich, G., and Peltzer, E. T. (2009). Controls on methane bubble dissolution inside and outside the hydrate stability field from open ocean field experiments and numerical modeling. Mar. Chem. 114, 19–30. doi: 10.1016/j.marchem.2009.03.004
Römer, M., Sahling, H., Pape, T., Bahr, A., Feseker, T., Wintersteller, P., et al. (2012). Geological control and magnitude of methane ebullition from a high-flux seep area in the Black Sea—the Kerch seep area. Mar. Geol. 319–322, 57–74. doi: 10.1016/j.margeo.2012.07.005
Römer, M., Sahling, H., Pape, T., dos Santos Ferreira, C., Wenzhöfer, F., Boetius, A., et al. (2014). Methane fluxes and carbonate deposits at a cold seep area of the Central Nile Deep Sea Fan, Eastern Mediterranean Sea. Mar. Geol. 347, 27–42. doi: 10.1016/j.margeo.2013.10.011
Ruppel, C. (2011). “Mehtane hydrates and the future of natural gas,” in MITEI Natural Gas Report (Supplementary paper No. 4), MIT, MA. Available online at: https://mitei.mit.edu/system/files/Supplementary_Paper_SP_2_4_Hydrates.pdf
Ruppel, C., and Noserale, D. (2012). Gas Hydrate and Climate Warming—Why a Methane Catastrophe is Unlikely. Sound Waves (USGS Newsletter). Available online at: http://soundwaves.usgs.gov/2012/06/
Schmale, O., Greinert, J., and Rehder, G. (2005). Methane emission from high-intensity marine gas seeps in the Black Sea into the atmosphere. Geophys. Res. Lett. 32, L07609. doi: 10.1029/2004GL021138
Schmale, O., Haeckel, M., and McGinnis, D. F. (2011). Response of the Black Sea methane budget to massive short-term submarine inputs of methane. Biogeosciences 8, 911–918. doi: 10.5194/bg-8-911-2011
Schneising, O., Burrows, J. P., Dickerson, R. R., Buchwitz, M., Reuter, M., and Bovensmann, H. (2014). Remote sensing of fugitive methane emissions from oil and gas production in North American tight geologic formations. Earth's Future 2, 1–11. doi: 10.1002/2014EF000265
Shakhova, N., Semiletov, I., Salyuk, A., Yusupov, V., Kosmach, D., and Gustafsson, Ö. (2010). Extenvisve methane venting to the atmosphere from sediments of the east Siberian Arctic shelf. Science 327, 1246–1250. doi: 10.1126/science.1182221
Skarke, A., Ruppel, C., Kodis, M., Brothers, D., and Lobecker, E. (2014). Widespread methane leakage from the sea floor on the northern US Atlantic margin. Nat. Geosci. 7, 657–661. doi: 10.1038/ngeo2232
Sloan, E. D., and Koh, C. A. (2008). Clathrate Hydrates of Natural Gases, 3rd Edn. New York, NY: CRC Press.
Solomon, E. A., Kastner, M., MacDonald, I. R., and Leifer, I. (2009). Considerable methane fluxes to the atmosphere from hydrocarbon seeps in the Gulf of Mexico. Nat. Geosci. 2, 561–565. doi: 10.1038/ngeo574
Sweeney, C., Gloor, E., Jacobson, A. R., Key, R. M., McKinley, G., Sarmiento, J. L., et al. (2007). Constraining global air-sea gas exchange for CO2 with recent bomb 14C measurements. Global Biogeochem. Cycles, 21, GB2015. doi: 10.1029/2006GB002784
Thorpe, A. K., Roverts, D. A., Bradley, E. S., Funk, C. C., Dennison, P. E., and Leifer, I. (2013). High resolution mapping of methane emissions from marine and terrestrial sources using a Cluster-Tuned Matched Filter technique and imaging spectrometry. Remote Sens. Environ. 134, 305–308. doi: 10.1016/j.rse.2013.03.018
U.S. EPA. (2012). Global Anthropogenic Non-CO2 Greenhouse Gas Emissions: 1990–2030. United States Environmental Protection Agency (EPA) Report (Washington, DC). Available online at: http://www.epa.gov/climatechange/Downloads/EPAactivities/Summary_Global_NonCO2_Projections_Dec2012.pdf
U.S. GAO. (2010). Federal Oil and Gas Leases: Opportunities Exist to Capture Vented and Flared Natural Gas, which would Increase Royalty Payments and Reduce Greenhouse Gases. United States Government Accountability Office (U.S. GAO) Report to Congressional Requesters. GAO-11-34. (Washington, DC). Available online at: http://www.gao.gov/products/GAO-11-34
Valentine, D. L., Kessler, J. D., Redmond, M. C., Mendes, S. D., Heintz, M. B., et al. (2010). Propane respiration jump-starts microbial response to a deep oil spill. Science 330, 208–211. doi: 10.1126/science.1196830
Velazco, V. A., Buchwitz, M., Bovensmann, H., Reuter, M., Schneising, O., Heymann, J., et al. (2011). Towards space based verification of CO2 emissions from strong localized sources: fossil fuel power plant emissions as seen by a CarbonSat constellation. Atmos. Meas. Tech. 4, 2809–2822. doi: 10.5194/amt-4-2809-2011
Vrålstad, T., Melbye, A. G., Carlsen, I. M., and Llewelyn, D. (2011). Comparison of leak-detection technologies for continuous monitoring of subsea-production templates. SPE Proj. Facil. Constr. 6, 96–103. doi: 10.2118/136590-PA
Walter, K. M., Engram, M., Duguay, C. R., Jeffries, M. O., and Chapin, F. S. (2008). The potential use of synthetic aperture radar for estimating methane ebullition from Arctic lakes. J. Am. Water Res. Ass. 44, 305–315. doi: 10.1111/j.1752-1688.2007.00163.x
Wanninkhof, R. (1992). Relationship between wind speed and gas exchange over the ocean. J. Geophys. Res. 97, 7373–7382. doi: 10.1029/92JC00188
Wanninkhof, R., Asher, W. E., Ho, D. T., Sweeney, C., and McGillis, W. R. (2009). Advances in quantifying air–sea gas exchange and environmental forcing. Annu. Rev. Mar. Sci. 1, 213–244. doi: 10.1146/annurev.marine.010908.163742
Warzinski, R. P., Lynn, R., Haljasmaa, I., Leifer, I., Shaffer, F., Anderson, B. J., et al. (2014). Dynamic morphology of gas hydrates on a methane bubble in water: observations and new insights for hydrate film models. Geophys. Res. Lett. 41, 6841–6847. doi: 10.1002/2014GL061665
Westbrook, G. K., Thatcher, K. E., Rohling, E. J., Piotrowski, A. M., Pälike, H., Osborne, A. H., et al. (2009). Escape of methane gas from the seabed along the West Spitsbergen continental margin. Geophys. Res. Lett. 36, L15608. doi: 10.1029/2009GL039191
Whiteman, G., Hope, C., and Wadhams, P. (2013). Vast costs of Arctic change. Nature 499, 401–403. doi: 10.1038/499401a
Xiao, S. B., Yang, H., Liu, D. F., Zhang, C., Lei, D., Wang, Y. C., et al. (2014). Gas transfer velocities of methane and carbon dioxide in a subtropical shallow pond. Tellus B 66:23795. doi: 10.3402/tellusb.v66.23795
Yamamoto, A., Yamanaka, Y., and Tajika, E. (2009). Modeling of methane bubbles released from large sea-floor area: condition required for methane emission to the atmosphere. Earth Planet. Sci. Lett. 284, 590–598. doi: 10.1016/j.epsl.2009.05.026
Yoshida, O., Inoue, H. Y., Watanabe, S., Noriki, S., and Wakatsuchi, M. (2004). Methane in the western part of the Sea of Okhotsk in 1998–2000. J. Geophys. Res. 109, C09S12. doi: 10.1029/2003JC001910
Yvon-Lewis, S. A., Hu, L., and Kessler, J. (2011). Methane fux to the atmosphere from the deepwater horizon oil disaster. Geophys. Res. Lett. 38, L01602. doi: 10.1029/2010GL045928
Keywords: methane, seafloor leakage, offshore oil-gas exploration, hydrate, coastal process
Citation: Zhang Y and Zhai W-D (2015) Shallow-ocean methane leakage and degassing to the atmosphere: triggered by offshore oil-gas and methane hydrate explorations. Front. Mar. Sci. 2:34. doi: 10.3389/fmars.2015.00034
Received: 11 February 2015; Accepted: 19 May 2015;
Published: 29 May 2015.
Edited by:
Sunil Kumar Singh, Physical Research Laboratory, IndiaReviewed by:
Arvind Singh, GEOMAR Helmholtz Centre for Ocean Research Kiel, GermanySanjeev Kumar, Physical Research Laboratory, India
Copyright © 2015 Zhang and Zhai. This is an open-access article distributed under the terms of the Creative Commons Attribution License (CC BY). The use, distribution or reproduction in other forums is permitted, provided the original author(s) or licensor are credited and that the original publication in this journal is cited, in accordance with accepted academic practice. No use, distribution or reproduction is permitted which does not comply with these terms.
*Correspondence: Yong Zhang, Key Laboratory of Coastal Zone Environmental Processes and Ecological Remediation, Yantai Institute of Coastal Zone Research, Chinese Academy of Sciences, 17, Chunhui Road, Laishan District, Yantai 264003, China,eXpoYW5nQHlpYy5hYy5jbg==