- 1Department of Ecology, Faculty of Science, University of Málaga, Málaga, Spain
- 2Laboratory of Botany, Faculty of Pharmacy, University of Barcelona, Barcelona, Spain
- 3Marine Biology and Ecology Research Centre, Plymouth University, Plymouth, UK
- 4Department of Botany, University Federal of Santa Catarina, Florianopolis, Brazil
- 5Dipartimento di Scienze della Terra e del Mare, University of Palermo, Palermo, Italy
- 6Institute for Marine and Antarctic Studies, University of Tasmania, Hobart, TAS, Australia
- 7School of Earth and Environment and ARC Centre of Excellence for Coral Reef Studies, University of Western Australia, Crawley, WA, Australia
Ocean acidification may benefit algae that are able to capitalize on increased carbon availability for photosynthesis, but it is expected to have adverse effects on calcified algae through dissolution. Shifts in dominance between primary producers will have knock-on effects on marine ecosystems and will likely vary regionally, depending on factors such as irradiance (light vs. shade) and nutrient levels (oligotrophic vs. eutrophic). Thus experiments are needed to evaluate interactive effects of combined stressors in the field. In this study, we investigated the physiological responses of macroalgae near a CO2 seep in oligotrophic waters off Vulcano (Italy). The algae were incubated in situ at 0.2 m depth using a combination of three mean CO2 levels (500, 700–800 and 1200 μatm CO2), two light levels (100 and 70% of surface irradiance) and two nutrient levels of N, P, and K (enriched vs. non-enriched treatments) in the non-calcified macroalga Cystoseira compressa (Phaeophyceae, Fucales) and calcified Padina pavonica (Phaeophyceae, Dictyotales). A suite of biochemical assays and in vivo chlorophyll a fluorescence parameters showed that elevated CO2 levels benefitted both of these algae, although their responses varied depending on light and nutrient availability. In C. compressa, elevated CO2 treatments resulted in higher carbon content and antioxidant activity in shaded conditions both with and without nutrient enrichment—they had more Chla, phenols and fucoxanthin with nutrient enrichment and higher quantum yield (Fv/Fm) and photosynthetic efficiency (αETR) without nutrient enrichment. In P. pavonica, elevated CO2 treatments had higher carbon content, Fv/Fm, αETR, and Chla regardless of nutrient levels—they had higher concentrations of phenolic compounds in nutrient enriched, fully-lit conditions and more antioxidants in shaded, nutrient enriched conditions. Nitrogen content increased significantly in fertilized treatments, confirming that these algae were nutrient limited in this oligotrophic part of the Mediterranean. Our findings strengthen evidence that brown algae can be expected to proliferate as the oceans acidify where physicochemical conditions, such as nutrient levels and light, permit.
Introduction
Ocean acidification due to increased atmospheric CO2 levels is altering the concentrations of dissolved inorganic carbon (DIC) in surface waters; CO2−3 levels are falling, which is expected to corrode marine carbonates, whilst CO2 and HCO−3 levels are rising which can stimulate photosynthesis (Connell et al., 2013; Cornwall et al., 2015). As some primary producers are better able to capitalize on increasing carbon availability than others, this is expected to alter marine communities (Hepburn et al., 2011; Connell et al., 2013; Koch et al., 2013; Gaylord et al., 2015). In the Mediterranean, surveys of coastal CO2 seeps have repeatedly shown that coralline algae and sea urchins become less common as pH and CO2−3 fall, whereas brown algae, such as Cystoseira spp., Dictyota spp., Sargassum vulgare and Padina pavonica, proliferate as CO2 and HCO−3 levels rise (Porzio et al., 2011; Baggini et al., 2014). The ways in which ocean acidification affects communities of primary producers are likely to vary regionally, depending on the species present and abiotic factors such as temperature, light and nutrient availability (Giordano et al., 2005; Brodie et al., 2014; Hofmann et al., 2014).
To begin to understand the influence of physicochemical factors on the responses of macroalgae to ocean acidification, we grew common types of brown algae (from the Families Fucales and Dictyotales) at CO2 seeps in a multifactorial experiment in which we manipulated light (irradiance) and nutrient levels. At low light levels, macroalgae are thought to be more likely to rely on carbon uptake via diffusion than use energetically expensive carbon concentrating mechanisms (Raven and Beardall, 2014; Raven et al., 2014) which has led to the idea that any benefits of ocean acidification on growth would only be seen at lower light levels for the majority of species (Hepburn et al., 2011). However, ocean acidification also has the potential to damage photoprotective mechanisms which kick-in at high light levels (Pierangelini et al., 2014). Algae minimize damage from high irradiance by down-regulating photosystems-they also produce chemicals, such as phenolic compounds in the brown algae, which screen ultraviolet light and dissipate energy (Figueroa et al., 2014a). In oligotrophic waters, such as those of the Mediterranean, nutrient availability generally limits macroalgal growth (Ferreira et al., 2011), photosynthetic capacity (Pérez-Lloréns et al., 1996) and photoprotective mechanisms (Celis-Plá et al., 2014a).
Our study centers upon a highly oligotrophic region (the Tyrrhenian Sea) which is undergoing rapid changes in carbonate chemistry coupled with coastal eutrophication and increased land run-off (Oviedo et al., 2015). In this region, as with elsewhere in the world, canopy-forming brown algae have undergone a decline in abundance due to anthropogenic perturbation (Scherner et al., 2013; Strain et al., 2014; Yesson et al., 2015). Here, we investigate the interactive effects of increasing CO2 levels and eutrophication on Cystoseira compressa and Padina pavonica using a pH gradient caused by volcanic seeps. These species were chosen because they are abundant around shallow Mediterranean CO2 seeps (Baggini et al., 2014), because Cystoseira spp. are indicators of high water quality in the Mediterranean (Bermejo et al., 2013) and since Padina spp. tolerate loss of external calcification as CO2 levels increase (Johnson et al., 2012; Pettit et al., 2015).
Macroalgal responses to ocean acidification may well depend upon their nutrient metabolism, which can vary widely between species (Hofmann et al., 2014; Hurd et al., 2014). Here, we compared interspecific physiological and biochemical responses to ocean acidification under different light and nutrient levels using standard methods for the study of multiple physical stressors in algae (Martínez et al., 2012; Celis-Plá et al., 2014a). Our hypothesis was that both brown algal species would benefit from ocean acidification in shaded conditions when nutrient levels were elevated. We expected that high light levels would inhibit photosystems, and that any benefits from high CO2 would only occur if sufficient nutrients were available. If this were true we expected to observe increased photosynthetic activity (using electron transport rates and carbon content as a proxy) and increases in phenolic and antioxidant production in shaded nutrient-enriched treatments.
Materials and Methods
Experimental Design
Macroalgal incubations took place from 19 to 22 March 2013, along a CO2 gradient near Vulcano, Italy (Figure 1; Boatta et al., 2013). Cystoseira compressa and Padina pavonica were collected at 0.5 m depth from a reference zone. Thalli (5 g fresh weight) were held in individual mesh cylinders (15 cm long × 5 cm in diameter) set 1 m apart and suspended at 0.2 m depth off a floating line that was anchored to the seabed perpendicular to the coast. This array was replicated at an ambient CO2 site (ca 500 μatm CO2), a medium CO2 site (ca 700–800 μatm CO2) and a high CO2 site (ca 1200 μatm CO2) (Table 1).
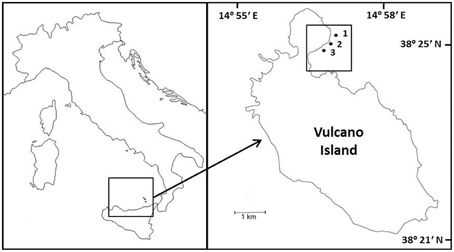
Figure 1. Sample sites and location of experiments on Cystoseira compressa and Padina pavonica off Vulcano, Italy; (1) Ambient CO2 site (ca 500 μatm CO2), (2) Medium CO2 site (ca 700–800 μatm CO2) and (3) High CO2 (ca 1200 μatm CO2).
Each CO2 zone had 12 replicates per treatment per species (nutrient enriched + ambient light or 100%PAB, i.e., 100% of surface irradiance defined as PAB irradiance (PAR + UVR), nutrient enriched + shaded light or 70%PAB, i.e., 70% of surface irradiance defined as PAB irradiance (PAR + UVR), non-enriched + ambient light or 100%PAB, non-enriched shaded light or 70%PAB). Light levels were manipulated using a 1 mm2 size pore mesh that reduced light levels to 70% of that of the unshaded treatments. The filter we used does not modify the light spectra (Aphalo et al., 2013). Mesh bags containing 100 g of a slow-release fertilizer comprising 17% N (NH+4 and NO−3), 17% P (P2O5) and 17% K (Multicote®, Haifa Chemicals, USA) were attached below nutrient enriched cylinders. For the non-enriched treatments, a bag with 100 g of sand was used as a control. The nutrient treatments were set 20 m apart from each other so that non-enriched treatments were unaffected.
Environmental Conditions
The seawater carbonate system was monitored at each site (Table 1). A 556 MPS YSI (Yellow Springs, USA) probe was used to measure salinity, pH and temperature (°C). The pH sensor was calibrated using NBS scale standard buffers. On 20th March 2013, water samples for total alkalinity (TA) were strained through 0.2 μm filters, poisoned with 0.05 ml of 50% HgCl2, and then stored in the dark at 4°C. Three replicates were analyzed at 25°C using a titrator (Mettler Toledo, Inc.). The pH was measured at 0.02 ml increments of 0.1 N HCl.
Total alkalinity was calculated from the Gran function applied to pH variations from 4.2 to 3.0, from the slope of the curve HCl volume vs. pH. The pCO2 and the saturation state of aragonite were calculated from pHNBS, TA, temperature and salinity using the CO2 SYS package (Pierrot and Wallace, 2006), using the constants of Roy et al. (1993) and Dickson (1990). Saturation state (Ω) is the ion product of calcium and carbonate ion concentrations as:
The apparent solubility product K'sp depends on temperature, salinity, pressure, and the particular mineral phase (e.g. calcite and aragonite in this case).
Irradiance was monitored at the sea surface at two wavelength bands using PAR (QSO-SUN 2.5V) and UV-A (USB-SU 100, Onset Computer Corporation, Massachusetts, USA) sensors sealed in a water proof box (OtterBox3000). Water temperature was monitored using a HOBO logger (Onset Computer Corporation, Massachusetts, USA). The nutrient enrichment caused by the release of the fertilizer was assessed taking triplicate seawater samples at both enriched and non-enriched sites. Seawater was strained using portable GF/F filters (Whatman International. Ltd., Maidstone, UK) then transported to the laboratory inside an isotherm bag (4°C, in darkness), and kept at −20°C. Nitrate (NO−3) was determined using an automated analyzer (SanPlus++ System, SKALAR, Breda, Netherlands) applying standard colorimetric procedures (Koroleff, 1983).
Physiological and Biochemical Variables
Several physiological variables were obtained from the algae within each cylinder at the end of the experiment. These variables were also measured in C. compressa and P. pavonica from ambient CO2 site (500 μatm) populations at 0.5 m depth. Carbon and nitrogen contents were determined using an element analyzer CNHS-932 model (LECO Corporation, Michigan, USA).
In vivo chlorophyll a fluorescence associated with Photosystem II was determined by using a portable pulse amplitude modulated (PAM) fluorometer (Diving-PAM, Walz GmbH, Germany). Macroalgal thalli were collected from natural populations (initial time) and after 4 days of incubation in the experiment (for each treatment or cylinder), and were put in 10 mL incubation chambers to obtain rapid light curves for each treatment. Fo and Fm were measured after 15 min in darkness to obtain the maximum quantum yield (Fv/Fm) being Fv = Fm–Fo, Fo the basal fluorescence of 15 min dark adapted thalli and Fm maximal fluorescence after a saturation light pulse of >4000 μmol m−2 s−1 (Schreiber et al., 1995). The electron transport rate (ETR) was determined after 20 s exposure in eight increasing irradiances of white light (halogen lamp provided by the Diving-PAM). The ETR was calculated according to Schreiber et al. (1995) as follows:
where ΔF/F′m is the effective quantum yield, being ΔF = Fm′−Ft (Ft is the intrinsic fluorescence of alga incubated in light and Fm′ is the maximal fluorescence reached after a saturation pulse of algae incubated in light), E is the incident PAR irradiance expressed in μmol photons m−2 s−1, A is the thallus absorptance as the fraction of incident irradiance that is absorbed by the algae (see Figueroa et al., 2003) and FII is the fraction of chlorophyll related to PSII (400–700 nm) being 0.8 in brown macroalgae (Figueroa et al., 2014a). ETR parameters as maximum electron transport rate (ETRmax) and the initial slope of ETR vs. irradiance function (αETR) as estimator of photosynthetic efficiency were obtained from the tangential function reported by Eilers and Peeters (1988). Finally, the saturation irradiance for ETR (EkETR) was calculated from the intercept between ETRmax and αETR. Non-photochemical quenching (NPQ) was calculated according to Schreiber et al. (1995) as:
Maximal NPQ (NPQmax) and the initial slope of NPQ vs. irradiance function (αNPQ) were obtained from the tangential function of NPQ vs. irradiance function according to Eilers and Peeters (1988).
Pigments were extracted from 20 mg fresh weight of thalli using 2 mL of 100% acetone and analyzed using an ultra-high-performance liquid chromatographer (Shimadzu Corp., Kyoto, Japan) equipped with a photodiode array detector to measure peaks in the range 350–800 nm. After extraction samples were centrifuged at 16200 g for 5 min (Sorvall Legend Micro 17, Thermo Scientific, Langenselbold, Germany) and then the extracts were filtered (0.22 μm nylon filters). The separation, was achieved with one column C-18 reversed phase (Shim-pack XR-ODS column; 3.0 × 75 mm i. d.; 2.2 μm particle size; Shimadzu, Kyoto, Japan) protected by a guard column TR-C-160 K1 (Teknokroma, Barcelona, Spain). The carotenoid composition was determined according to García-Plazaola and Becerril (1999) with some modifications (García-Plazaola et al., 2012), using commercial standards (DHI LAB Products). The mobile phase consisted of two components: Solvent A, acetonitrile: methanol: Tris buffer (0.1 M, pH 8) (84:2:14); and solvent B, methanol: ethyl acetate (68:32). The pigments were eluted using a linear gradient from 100% A to 100% B for the first 7 min, followed by an isocratic elution with 100% B for the next 4 min. This was followed by a 50 s linear gradient from 100% B to 100% A and an isocratic elution with 100% B for the next 3 min to allow the column to re-equilibrate with solvent A, prior to the next injection.
Total phenolic compounds were determined using 0.25 g fresh weight samples pulverized with a mortar and pestle with sand and 2.5 mL of 80% methanol. After keeping the samples overnight at 4°C, the mixture was centrifuged at 2253 g for 30 min at 4°C, and then the supernatant was collected. Total phenolic compounds were determined colorimetrically using Folin-Ciocalteu reagent and phloroglucinol (1,3,5-trihydroxybenzene, Sigma P-3502) as standard. Finally the absorbance was determined at 760 nm using a spectrophotometer (UV Mini-1240, Shimadzu) (Celis-Plá et al., 2014b). Total phenolic content was expressed as mg g−1 DW after determining the fresh to dry weight ratio in the tissue (5.2 for C. compressa and 4.5 P. pavonica, respectively). The results are expressed as average ± SE from three replicates of each treatment. Antioxidant activity was measured on polyphenol extracts according to Blois (1958); 150 μL of DPPH (2,2-diphenyl-1-picrylhydrazyil) prepared in 90% methanol were added to each extract. The reaction was complete after 30 min in darkness at ambient temperature (~20°), and the absorbance was read at 517 nm in a spectrophotometer (UVmini-1240, Shimadzu). The calibration curve made from DPPH was used to calculate the remaining concentration of DPPH in the reaction mixture after incubation. Values of DPPH concentration (mM) were plotted against plant extract concentration expressed as the EC50 value (oxidation index, mg DW mL−1) required to scavenge 50% of the DPPH in the reaction mixture. Ascorbic acid was used as a control (Celis-Plá et al., 2014b).
Statistical Analysis
The effects of the in situ treatments on the physiological responses of C. compressa and P. pavonica were assessed using analysis of variance. Three fixed factors were considered: Site with three levels: ambient CO2 site, medium CO2 and high CO2, Irradiance with two levels: 70 and 100% of surface irradiance (PAR + UVR irradiance), and two nutrient levels; enriched (N+) and non-enriched (N). This design allowed us to test interactive and additive effects of the variables on physiological responses after the 4 day experimental period. Student Newman Keuls tests (SNK) were performed on significant ANOVA interactions. Homogeneity of variance was tested using Cochran tests and by visual inspection of the residuals. All data conformed to homogeneity of variance. Analyses were performed by using SPSS v.21 (IBM, USA).
Results
Environmental Conditions
Cystoseira compressa and Padina pavonica were abundant at all three stations; P. pavonica was visibly less calcified at the site with the highest levels of CO2. The seawater temperature was about15°C and the salinity was 38 at all stations; at the Ambient site, mean pH was 8.11, at the Medium CO2 site (700–800 μatm), mean pH was 7.97 and at the High CO2 site (1200 μatm), it was 7.86 (Table 1).
The average daily irradiance for the experimental period was 5360 kJ m−2 for PAR and 666 kJ m−2 for UVA. The nutrient enriched treatments had approximately 100 times the nitrate concentration of the ambient seawater; ambient vs. enriched ratios were 0.16 ± 0.04 vs. 106.17 ± 9.37 μM for the ambient site, 0.13 ± 0.01 vs. 106.33 ± 9.37 μM at the medium CO2 site and 0.25 μM ± 0.01 vs. 106.42 ± 9.37 μM at the high CO2 site (mean ± SE, n = 3).
Physiological and Biochemical Responses
The carbon content of C. compressa increased with increasing CO2, whereas in P. pavonica showed interactive effects between all factors. Carbon, in P. pavonica showed maximal values 279.9 ± 6.5 with increased CO2, in non-enrichment enriched treatments and minimal values 225.3 ± 2.4 mg g−1 DW with decreased CO2, non-nutrient enriched and 70%PAB conditions (Table 2, Table S1). The nitrogen content of C. compressa was greatest in the high CO2, nutrient enriched and 70%PAB treatment (Figure 2A, Figure S1); conversely, in P. pavonica the nitrogen content was highest at the reference site, ambient CO2 treatment (Figure 2B, Figure S1). The ratio C:N of C. compressa did not show significant differences between treatments (Figure 3A, Figure S1), whereas the ratio in P. pavonica showed significant effects for CO2 levels and nutrient enrichment showed maximal values (19.5 ± 5.8) with increased CO2, non-nutrient enriched in 100%PAB conditions and minimal values 15.9 ± 0.5 in medium CO2, nutrient enrichment and 70%PAB conditions (Figure 3B, Figure S1).
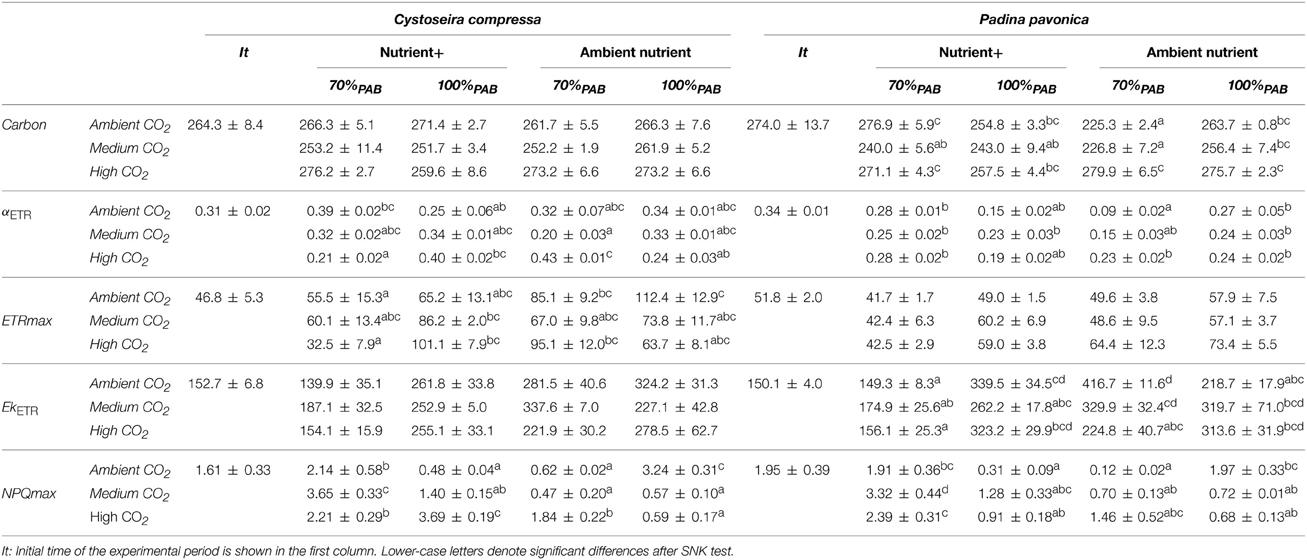
Table 2. Carbon content, photosynthetic efficiency (αETR), maximal electron transport rate (ETRmax), expressed in μmol m−2 s−1), irradiance of saturation of ETR (EkETR), maximal non-photochemical quenching (NPQmax) (mean values ± SE, n = 3) of Cystoseira compressa and Padina pavonica in relation to Irradiance (70%PAB: low irradiance and 100%PAB: ambient irradiance), Nutrients (Nutrient+ and Ambient Nutrient) and CO2 (ambient CO2 site: 500 μatm, Medium CO2 site: 700–800 μatm and High CO2: 1200 μatm) treatments.
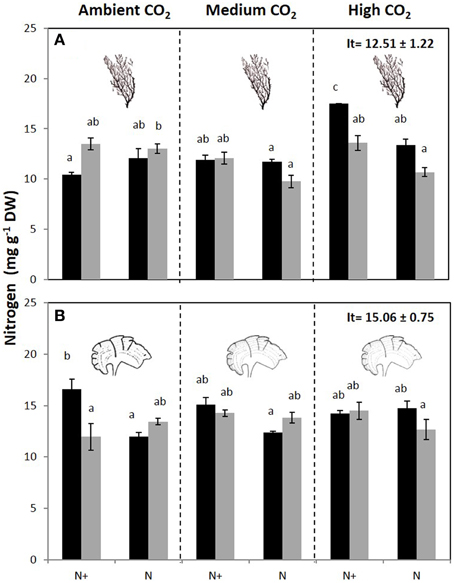
Figure 2. Nitrogen content (mg g−1 DW) (mean ± SE, n = 3) in (A) Cystoseira compressa and (B) Padina pavonica at Ambient CO2 (ca 500 μatm, left boxes), Medium CO2 (ca 700–800 μatm, middle boxes) and High CO2 (ca 1200 μatm, right boxes), in Irradiance and Nutrient treatments. Dark bars indicate 70%PAB (low irradiance), gray bars indicate 100%PAB (ambient irradiance). N+ and N indicate nutrient enriched and non-enriched treatments respectively. Upper values in right box indicate initial time values (It: mean ± SE, n = 3). Lower-case letters denote significant differences after an SNK test.
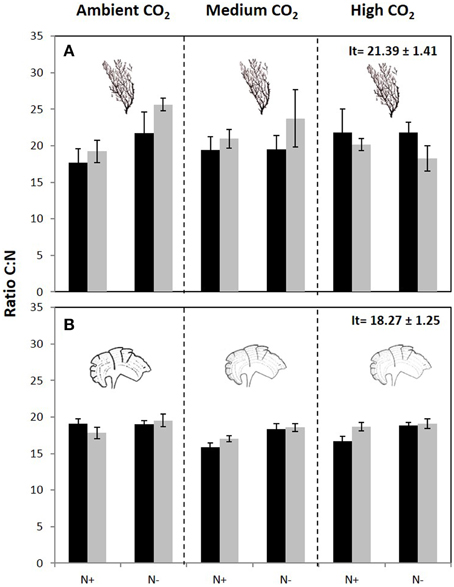
Figure 3. C:N ratio (mean ± SE, n = 3) in (A) Cystoseira compressa and (B) Padina pavonica at Ambient CO2 (ca 500 μatm, left boxes), Medium CO2 (ca 700–800 μatm, middle boxes) and High CO2 (ca 1200 μatm, right boxes), in Irradiance and Nutrient treatments. Dark bars indicate 70%PAB (low irradiance), gray bars indicate 100%PAB (ambient irradiance). N+ and N indicate nutrient enriched and non-enriched treatments respectively. Upper values in right box indicate initial time values (It: mean ± SE, n = 3).
The maximal quantum yield (Fv/Fm) was significantly different between CO2 treatments, nutrient and irradiance in both macroalgae (Figure 4, Figure S2). In C. compressa, the Fv/Fm was greatest in 70%PAB treatments with high CO2, and non-enriched enrichment (Figure 4A, Figure S2), but in P. pavonica this was greatest in the nutrient enriched treatments (Figure 4B, Figure S2). The αETR values also varied significantly between treatments in both species (Table 2, Table S2). In C. compressa, αETR was greatest in 70%PAB treatments at high CO2 with non-enriched enrichment; in P. pavonica αETR was greatest in the high CO2 conditions (Table 2, Table S2). ETRmax in C. compressa was highest in high CO2, 70%PAB and non-nutrient enrichment, also in 100%PAB and nutrient enrichment, and also this was higher with decreased CO2, 100%PAB, in non-nutrient enrichment. In P. pavonica, ETRmax varied significantly depending on nutrient and irradiance, without interactions (Table 2, Table S2). In contrast, the EkETR in C. compressa had one significant interaction among nutrient x irradiance. P. pavonica had significant interactions between CO2 level, nutrient and irradiance. The EkETR, in C. compressa, was greatest in the 100%PAB treatments that had no CO2 or nutrient enrichment, but in P. pavonica EkETR was greatest in 70%PAB conditions (Table 2, Table S2). In both species, the maximal non-photochemical quenching (NPQmax) was affected by the interaction of all factors. In C. compressa, NPQmax increased significantly with increasing CO2 conditions, under nutrient enriched and 100%PAB, also increased in ca 700–800 μatm but in 70%PAB. As well as, NPQmax increased under ambient CO2 conditions in 100%PAB in nutrient non-enriched. Finally, in P. pavonica, the NPQmax was significantly higher in 70%PAB at 700 μatm CO2 treatment with nutrient enrichment (Table 2, Table S2).
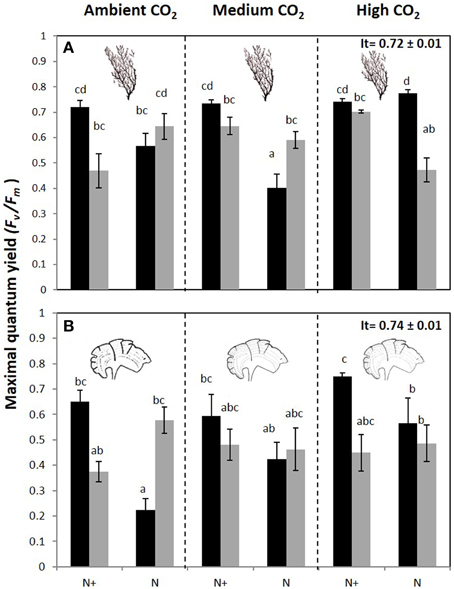
Figure 4. Maximal quantum yield or Fv/Fm (mean ± SE, n = 3) in (A) Cystoseira compressa and (B) Padina pavonica at Ambient CO2 (ca 500 μatm, left boxes), Medium CO2 (ca 700–800 μatm, middle boxes) and High CO2 (ca 1200 μatm, right boxes), in Irradiance and Nutrient treatments. Dark bars indicate 70%PAB (low irradiance), and gray bars indicate 100%PAB (ambient irradiance). N+ and N indicate nutrient enriched and non-enriched treatments respectively. Upper values in right box indicate initial time values (It: mean ± SE, n = 3). Lower-case letters denote significant differences after an SNK test.
Nutrient enrichment increased Chla significantly in C. compressa. In contrast, in P. pavonica significant differences were found for the following interactions: CO2 level × nutrient, CO2 level × irradiance and nutrient × irradiance (Table 3, Table S3). The same occurred for Chlc in P. pavonica; but there was no significant difference in C. compressa (Table 3, Table S3). The carotenoids, fucoxanthin and violaxanthin in C. compressa did not differ among factors (Table 3, Table S3) but in P. pavonica the fucoxanthin and violaxanthin contents were affected by the interaction of all factors. Fucoxanthin increased in 70%PAB, non-enriched treatments in ambient CO2 whereas violoxanthin levels were highest in 70%PAB, ca 700–800 μatm CO2, nutrient enriched treatment (Table 3, Table S3).
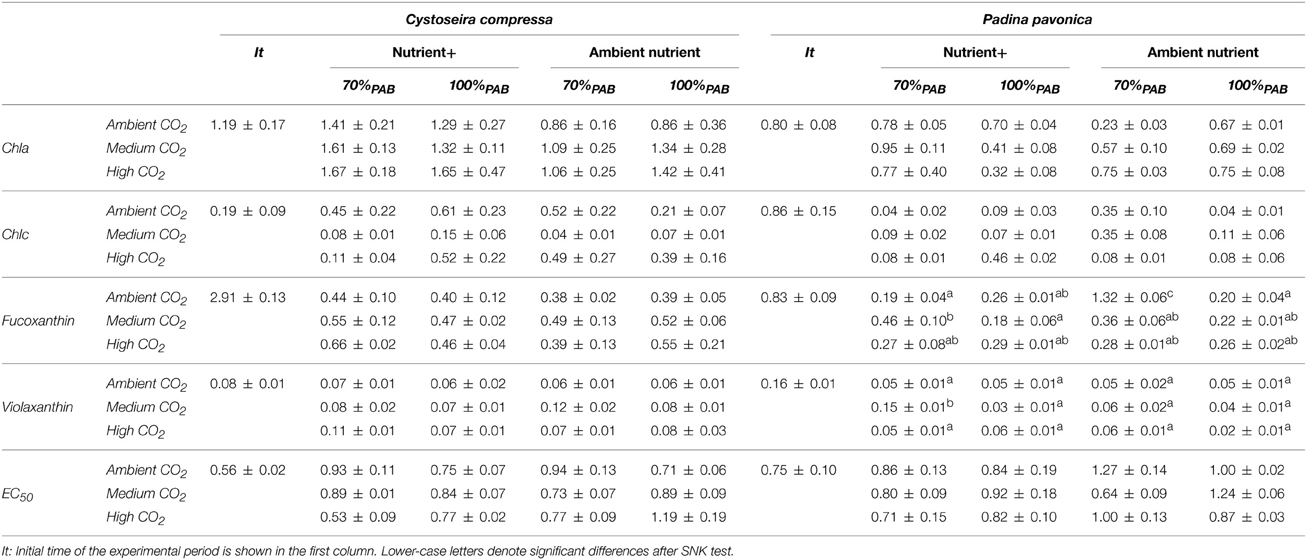
Table 3. Pigment contents; Chlorophyll a (Chla), chlorophyll c (Chlc), Fuxocanthin and Violaxanthin contents are expressed in mg g−1 DW and Antioxidant activity expressed as EC50 in mg DW mL−1 (mean values ± SE, n = 3) of Cystoseira compressa and Padina pavonica in relation to Irradiance (70%PAB: low irradiance and 100%PAB: ambient irradiance), Nutrient (Nutrient+ and Ambient Nutrient) and CO2 (ambient CO2 site: 500 μatm, Medium CO2 site:700-800 μatm and High CO2: 1200 μatm) treatments.
Phenolic content (PC) was affected by the interaction of all factors in both species (Figure 5, Figure S4). In C. compressa, PC was highest in CO2 and nutrient enriched conditions (Figure 5A, Figure S4). In P. pavonica at 1200 μatm CO2, PC was high in 100%PAB and nutrient enriched treatments and in 70%PAB treatments non-nutrient enrichment (Figure 5B, Figure S4). Antioxidant activity (EC50) showed a significant interaction between CO2 level × nutrient and CO2 level × irradiance in C. compressa; however in P. pavonica the only significant difference found in antioxidant activity was between CO2 level and irradiance. In C. compressa and P. pavonica, EC50 was lowest (i.e., it had higher antioxidant activity) in the high CO2, 70%PAB light conditions and nutrient enriched treatments (Table 3, Table S4).
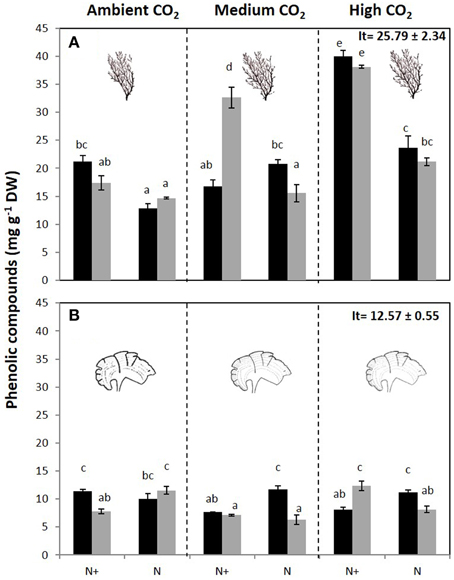
Figure 5. Total phenolic compounds (mg g−1 DW) (mean ± SE, n = 3) in (A) Cystoseira compressa and (B) Padina pavonica from Ambient CO2 (ca 500 μatm, left boxes), Medium CO2 (ca 700–800 μatm, middle boxes) and High CO2 (ca 1200 μatm, right boxes), in Irradiance and Nutrient treatments. Dark bars indicate 70%PAB (low irradiance), gray bars indicate 100%PAB (ambient irradiance). N+ and N indicate nutrient enriched and non-enriched treatments respectively. Upper values in right box indicate initial time values (It: mean ± SE, n = 3). Lower-case letters denote significant differences after an SNK test.
Discussion
Recent reviews surmise that ocean acidification is likely to increase macroalgal productivity due to beneficial effects of increased dissolved inorganic carbon (DIC) levels which can stimulate the growth of algae and allows them to divert more resources into anti-herbivore and photo-protective compounds (Harley et al., 2012; Brodie et al., 2014). Here we show that calcified and non-calcified macroalgae can indeed benefit physiologically from increases in DIC, but that the benefits, and the extent of the algal response, depend upon nutrient and light availability. Figure 6 summarizes our projections that brown macroalgal stands will both proliferate in the shallows (because of up-regulation of anti-herbivore and photo-protective compounds) and extend deeper due to a combination of ocean acidification and anthropogenic nutrient input, whereas other work on Mediterranean CO2 seeps has established that sea urchins and coralline algae are adversely affected by acidification (Baggini et al., 2014). In vivo chlorophyll a fluorescence parameters (maximal quantum yield or Fv/Fm and maximal electron transport rate or ETRmax) and algal biochemical composition (Chla, total phenolic compounds and antioxidant activity, %C) helps explain the dominance of phaeophytes at a variety of coastal Mediterranean CO2 seeps. Increases in brown macroalgal cover at CO2 seep sites are probably due to a combination of the direct stimulus of increased DIC for photosynthesis for species with inefficient carbon concentrating mechanisms (CCMs), and decreased grazing since sea urchins for example are excluded by hypercapnia (Calosi et al., 2013).
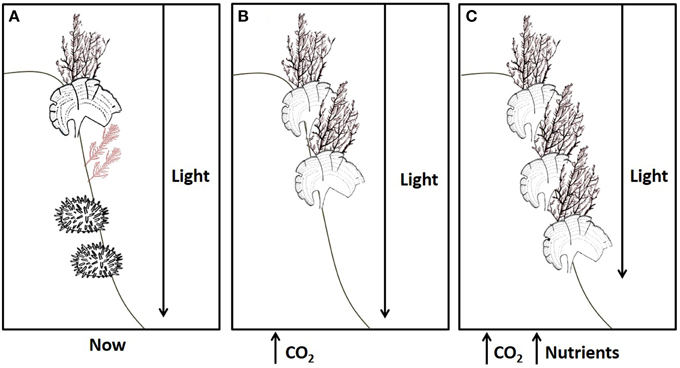
Figure 6. The effects of ocean acidification on rocky shore communities will depend on nutrient levels. (A) A typical brown algal canopy with coralline algae and sea urchins at present-day levels of CO2, nutrients and light. (B) Increased CO2 in oligotrophic conditions has adverse impacts on most calcified organisms, such as coralline algae and sea urchins, yet dense brown algal stands persist at shallow depths despite corrosion of facultatively calcified algae, such as Padina. (C) Ocean acidification remains detrimental to most calcified organisms, but when this is combined with nutrient enrichment fleshy algae can flourish since they are able to take full advantage of increases in CO2, extending deeper into the photic zone despite increased turbidity.
Other Mediterranean seep locations show similar trends to Vulcano, with increases in Cystoseira and Padina species at elevated CO2 locations compared to reference locations (Johnson et al., 2012; Baggini et al., 2014). Work in other regions has also shown that ocean acidification can directly benefit some macroalgae, such as Gracilaria lemaneiformis in China (Zou and Gao, 2009) and mat-forming Feldmannia spp. in Australia (Russell et al., 2011), as well as canopy-forming phaeophytes such as Nereocystis luetkeana and Macrocystis pyrifera (Swanson and Fox, 2007; Roleda et al., 2012). We found that the benefits of increased DIC were even more pronounced when combined with increased nutrients. This is what we expected, given that macroalgae tend to be nutrient-limited in oligotrophic waters such as those of the Mediterranean Sea (Ferreira et al., 2011). Both our study species increased electron transport rates and the accumulation of photoprotectors when exposed to a Nitrogen Phosphorus Potassium fertilizer, but these were short-term experiments with macroalgae grown in isolation. We suspect that chronic eutrophication combined with ocean acidification may benefit more opportunistic algal groups, to the detriment of brown macroalgae based on research by Russell et al. (2009) and Falkenberg et al. (2013). In our study, C. compressa and P. pavonica had increased carbon content at elevated CO2, which was augmented by increases in a range of other physiological parameters when nutrient levels were also increased. The Fv/Fm ratio was highest at increased CO2 concentrations with no nutrient enrichment in C. compressa, but highest at increased CO2 with nutrient enrichment for P. pavonica (Figure 4). The maximal photosynthetic activity (ETRmax) in C. compressa was reduced at high nutrient levels in shaded conditions but in fully lit conditions nutrients did not have significant effects under high DIC conditions. In other Cystoseira species, such as C. tamariscifolia, both Fv/Fm and ETRmax also decrease in nutrient enriched treatments in field experiments at various depths (Celis-Plá et al., 2014a). In another experiment, Celis-Plá et al. (2014b) found the highest ETRmax in C. tamariscifolia in thalli with the lowest internal nitrogen stores i.e., winter compared to summer grown algae. On the other hand, EkNPQ increased in all cases with the increased CO2 as an acclimation to high light levels. On this basis, it is clear that the responses of coastal macroalgal communities to ocean acidification will depend on nutrient availability, and will be species-specific. Given these results we expect that in temperate waters, brown algae will benefit from increases in CO2 if sufficient nutrients are available (Johnson et al., 2012). However, as with all ecology, we can expect that there will be a region-specific balancing act. We show here that in oligotrophic conditions brown macroalgae were unable to take full advantage of increased inorganic carbon availability. There is added complexity when we consider that many regions have experienced a die-back of canopy-forming brown algae due to excess nutrients or sedimentation (Strain et al., 2014); ocean acidification may exacerbate this problem since increased DIC may further benefit those algae that presently compete with fucoids and kelps in eutrophic conditions (Connell et al., 2013).
Light quantity and quality drive physiological processes in macroalgae (Hanelt and López-Figueroa, 2012), so we were not surprised to find that shading affected their responses to ocean acidification. We anticipated two outcomes of the effects of light: we expected ETR rates to be higher as the most obvious response to light, but we also expected low-light macroalgae to increase ETR rates and %C when transplanted to higher CO2 concentrations. Our first expectations were met, as maximum quantum yield, photosynthetic efficiency, irradiance of saturation and non-photochemical quenching for chlorophyll fluorescence all increased at higher light levels and were, at times, amplified by increasing CO2 and nutrient levels. The only instance where our second expectation was met was for P. pavonica under ambient nutrients, which had significantly higher %C (and non-significantly higher ETRmax) when transplanted to elevated CO2 sites. Previous studies at the same sites found elevated ETRmax when comparing P. pavonica at an elevated CO2 site compared to an ambient CO2 site (Johnson et al., 2012). If the duration of our experiment had been longer, our transplanted P. pavonica may also have significantly increased their ETRmax. Our results emphasize the likelihood that ocean acidification will act upon primary production differently at different latitudes and depths, not always according to our expectations. This is important since increases in land nutrient run-off, due to changes in land use and/or rainfall, are altering light levels in coastal waters (Scherner et al., 2013).
One of the most important photoprotective mechanisms available to algae is an ability to dissipate excess thermal energy (Adams et al., 2006). Thermal dissipation measured as non-photochemical PSII fluorescence quenching (NPQ) is triggered by the trans-thylakoidal proton gradient (ΔpH) and zeaxanthin (ZEA) synthesis through the xanthophyll cycle (Gilmore et al., 1994) and is recognized as the most important photoprotective mechanisms in higher plants and several algal divisions (Rodrigues et al., 2002). Fucoxanthin and violaxanthin levels were not affected in C. compressa whereas in P. pavonica fucoxanthin and violaxanthin increased under 70%PAB conditions, nutrient enrichment and medium CO2 levels. We used NPQmax as an indicator of photoprotective energy dissipation efficiency (Celis-Plá et al., 2014b), and we also measured phenolic content and antioxidant activity (EC50), both of which can be used as photoprotectors (Celis-Plá et al., 2014a). In C. compressa and P. pavonica NPQmax was higher in all shaded treatments with nutrient enrichment, but not in the fully lit treatments, indicating higher photoprotection when nutrients were elevated and light was reduced. Phenols usually accumulated under higher irradiance and (for C. compressa) higher CO2 treatments, as per past studies on kelp grown at high CO2 (Swanson and Fox, 2007), or measured under higher irradiance (Connan et al., 2004). However, the effects of CO2 on autotroph phenol production are not straight forward, as previous work has shown that both seagrass (Arnold et al., 2012) and the macroalga Cystoseira tamariscifolia (Figueroa et al., 2014b) decrease phenol production when CO2 increased. In C. compressa and P. pavonica, antioxidant activity and EC50 were affected by the interactions between light levels and CO2. EC50 tended to be higher in shaded, high CO2 treatments with and without nutrient addition, suggesting a positive correlation with phenolic compounds and their use as antioxidants to prevent photodamage. Together, NPQmax, phenol production and EC50 indicate that in elevated CO2 conditions some species will have a higher capacity for photoprotection.
Macroalgae regulate their biochemical composition to changes in solar radiation (Bischof et al., 2006; Figueroa et al., 2014a,b). Whilst light obviously affects photosynthesis, other variables such pH, nutrients and the availability of different DIC species all have the potential to affect photosynthetic rates (Raven and Beardall, 2014). As interactions among such factors will determine the success of algal species and the amount of primary productivity in any time and place, it is crucial to know how the effects of ocean acidification are modified by other key drivers of photosynthesis. Research similar to our study, but with more species, in more locations and for longer durations, is clearly required before solid conclusions can be made with respect to the effects of ocean acidification on macroalgal productivity.
In conclusion, our study shows that ongoing ocean acidification can be expected to increase photosynthetic efficiency and algal productivity. The magnitude of these effects, and the species that benefit, will depend on light and nutrient levels. We show that C. compressa and P. pavonica are able to benefit from an increase in CO2 levels, rapidly changing their physiology and biochemical composition over 3 day alterations in DIC, irradiance and nutrients. These factors had interactive effects on photosynthetic and photoprotective systems in both species and help explain why brown algae proliferate at CO2 seeps. Longer-term growth studies involving algal interactions would be useful: we remain concerned that chronic eutrophication combined with ocean acidification may benefit more opportunistic algal groups to the detriment of canopy-forming brown macroalgae. As ocean acidification is not happening in isolation, but alongside a plethora of other anthropogenic changes, an understanding of the interactive effects of multiple stressors is critical to plan for global ocean change. We have shown that elevated CO2 levels can enhance brown algal productivity, and may boost the kelp and fucoid forests of the planet, but the effects will depend upon interactions with other physicochemical parameters such as light and nutrient availability.
Conflict of Interest Statement
The authors declare that the research was conducted in the absence of any commercial or financial relationships that could be construed as a potential conflict of interest.
Acknowledgments
This work was supported by the Junta de Andalucía (Project RNM-5750) and by the research group RNM-295. The NERC/Defra/DECC UK Ocean Acidification research programme and Save Our Seas Foundation contributed to fieldwork expenses (this video https://vimeo.com/101795615 shows the field site). PC gratefully acknowledges financial support from “Becas-Chile” (CONICYT) fellowship of doctorate of the Ministry of Education of the Republic of Chile. P. A. Horta is also grateful to CNPq grants, CAPES (PNADB/visitant professor fellowship) and INCT-MC to additional financial support.
Supplementary Material
The Supplementary Material for this article can be found online at: http://journal.frontiersin.org/article/10.3389/fmars.2015.00026/abstract
References
Adams, W. W. I. I. I., Zarter, C. R., Mueh, K. E., Amiard, V. S. E., and Demmig-Adams, B. (2006). “Energy dissipation and photoinhibition: a continuum of photoprotection,” in Photoprotection, Photoinhibition, Gene Regulation, and Environment, eds B. Demmig-Adams, W. W. III. Adams, A, Mattoo (Dordrecht: Springer), 49–64.
Aphalo, P. J., Albert, A., McLeod, A., Heikkilä, A., Gómez, I., Figueroa, F. L., et al. (2013). “2 Manipulating UV radiation,” in Beyond the Visible A Handbook of Best Practice in Plant UV Photobiology, eds P. J. Aphalo, A. Albert, L. O. Björn, A. McLeod, T. M. Robson, and E. Rosenqvist (COST Action FA0906 UV4growth, Helsinki: University of Helsinki, Division of Plant Biology), 35–70.
Arnold, T., Mealey, C., Leahey, H., Miller, A. W., Hall-Spencer, J. M., Milazzo, M., et al. (2012). Ocean acidification and the loss of phenolic substances in marine plants. PLoS ONE 7:e35107. doi: 10.1371/journal.pone.0035107
Baggini, C., Salomidi, M., Voutsinas, E., Bray, L., Krasakopoulou, E., and Hall-Spencer, J. M. (2014). Seasonality affects macroalgal community response to increases in pCO2. PLoS ONE 9:e106520. doi: 10.1371/journal.pone.0106520
Bermejo, R., De la Fuente, G., Vergara, J. J., and Hernández, I. (2013). Application of the CARLIT index along a biogeographical gradient in the Alboran Sea (European Coast). Mar. Pollut. Bull. 72, 107–118. doi: 10.1016/j.marpolbul.2013.04.011
Bischof, K., Gómez, I., Molis, M., Hanelt, D., Karsten, U., Lüder, U., et al. (2006). Ultraviolet radiation shapes seaweed communities. Rev. Environ. Sci. Biot. 5, 141–166. doi: 10.1007/s11157-006-0002-3
Blois, M. S. (1958). Antioxidant determinations by the use of a stable free radical. Nature 181, 1199–1200. doi: 10.1038/1811199a0
Boatta, F., D'Alessandro, W., Gagliano, A. L., Liotta, M., Milazzo, M., Rodolfo-Metalpa, R., et al. (2013). Geochemical survey of Levante Bay, Vulcano Island (Italy) and its suitability as a natural laboratory for the study of ocean acidification. Mar. Pollut. Bull. 73, 485–494. doi: 10.1016/j.marpolbul.2013.01.029
Brodie, J., Williamson, C., Smale, D. A., Kamenos, N. A., Mieszkowska, N., Santos, R., et al. (2014). The future of the northeast Atlantic benthic flora in a high CO2 world. Ecol. Evol. 4, 2787–2798. doi: 10.1002/ece3.1105
Calosi, P., Rastrick, S. P. S., Graziano, M., Thomas, S. C., Baggini, C., Carter, H. A., et al. (2013). Distribution of sea urchins living near shallow water CO2 vents is dependent upon species acid-base and ion-regulatory abilities. Mar. Poll. Bull. 73, 470–484 doi: 10.1016/j.marpolbul.2012.11.040
Celis-Plá, P. S. M., Korbee, N., Gómez-Garreta, A., and Figueroa, F. L. (2014b). Seasonal photoacclimation patterns in the intertidal macroalga Cystoseira tamariscifolia (Ochrophyta). Sci. Mar. 78, 377–388. doi: 10.3989/scimar.04053.05A
Celis-Plá, P. S. M., Martínez, B., Quintano, E., García-Sánchez, M., Pedersen, A., Navarro, N. P., et al. (2014a). Short-term ecophysiological and biochemical responses of Cystoseira tamariscifolia and Ellisolandia elongata to changes in solar irradiance and nutrient levels. Aquat. Biol. 22, 227–243. doi: 10.3354/ab00573
Connan, S., Goulard, F., Stiger, V., Deslandes, E., and Ar-Gall, E. (2004). Interspecific and temporal variation in phlorotannin levels in an assemblage of brown algae. Bot. Mar. 47, 410–416. doi: 10.1515/BOT.2004.057
Connell, S. D., Kroeker, K. J., Fabricius, K. E., Kline, D. I., and Russell, B. D. (2013). The other ocean acidification problem: CO2 as a resource among competitors for ecosystem dominance. Philos. Trans. R. Soc. Lond. B Biol. Sci. 368:20120442. doi: 10.1098/rstb.2012.0442
Cornwall, C. E., Revill, A. T., and Hurd, C. L. (2015). High prevalence of diffusive uptake of CO2 by macroalgae in a temperate subtidal ecosystem. Photosynth. Res. 124, 181–190. doi: 10.1007/s11120-015-0114-0
Dickson, A. G. (1990). Standard potential of the reaction: AgCl(s) + 1/2 H2(g) = Ag(s) + HCl(aq), and the standard acidity constant of the ion HSO−4 in synthetic seawater from 273.15 to 318.15 KJ. J. Chem. Thermodyn. 22, 113–127. doi: 10.1016/0021-9614(90)90074-Z
Eilers, P. H. C., and Peeters, J. C. H. (1988). A model for the relationship between light intensity and the rate of photosynthesis in phytoplankton. Ecol. Model. 42, 199–215. doi: 10.1016/0304-3800(88)90057-9
Falkenberg, L. J., Connell, S. D., and Russell, B. D. (2013). Disrupting the effects of synergies between stressors: improved water quality dampens the effects of future CO2 on a marine habitat. J. Appl. Ecol. 50, 51–58. doi: 10.1111/1365-2664.12019
Ferreira, J. G., Andersen, J. H., Borja, A., Bricker, S. B., Camp, J., Cardoso da Silva, M., et al. (2011). Overview of eutrophication indicators to assess environmental status within the European Marine Strategy Framework Directive. Estuar. Coast. Shelf. S. 93, 117–131. doi: 10.1016/j.ecss.2011.03.014
Figueroa, F. L., Conde-Álvarez, R., and Gómez, I. (2003). Relations between electron transport rates determined by pulse amplitude modulated chlorophyll fluorescence and oxygen evolution in macroalgae under different light conditions. Photosynth. Res. 75, 259–275. doi: 10.1023/A:1023936313544
Figueroa, F. L., Dominguez-González, B., and Korbee, N. (2014a). Vulnerability and acclimation to increased UVB in the three intertidal macroalgae of different morpho-functional groups. Mar. Env. Res. 101, 8–21. doi: 10.1016/j.marenvres.2014.01.009
Figueroa, F. L., Malta, E.-J., Bonomi-Barufi, J., Conde-Álvarez, R., Nitschke, U., and Arenas, F. (2014b). Short-term effects of increasing CO2, nitrate and temperature on three Mediterranean macroalgae: biochemical composition. Aquat. Biol. 22, 177–193. doi: 10.3354/ab00610
García-Plazaola, J. I., and Becerril, J. M. (1999). A rapid HPLC method to measure lipophilic antioxidants in stressed plants: simultaneous determination of carotenoids and tocopherols. Phytochem. Anal. 10, 307–313.
García-Plazaola, J. I., Fernandez-Marin, B., and Porcar-Castell, A. (2012). Thermal energy dissipation and xanthophyll cycles beyond the Arabidopsis model. Photosynth. Res. 113, 89–103. doi: 10.1007/s11120-012-9760-7
Gaylord, B., Kroeker, K. J., Sunday, J. M., Anderson, K. M., Barry, J. P., Brown, N. E., et al. (2015). Ocean acidification through the lens of ecological theory. Ecology 96, 3–15. doi: 10.1890/14-0802.1
Gilmore, A. M., Mohanty, N., and Yamamoto, H. Y. (1994). Epoxidation of zeaxanthin and antheraxanthin reverses non-photochemical quenching of photosystem II chlorophyll a fluorescence in the presence of trans-thylakoid DpH. FEBS. Lett. 390, 271–274. doi: 10.1016/0014-5793(94)00784-5
Giordano, M., Beardall, J., and Raven, J. A. (2005). CO2 concentrating mechanisms in algae: mechanisms, environmental modulation, and evolution. Annu. Rev. Plant Biol. 56, 99–131. doi: 10.1146/annurev.arplant.56.032604.144052
Hanelt, D., and López-Figueroa, F. (2012). “Physiological and photomorphogenic effects of light of marine macrophytes,” in Seaweed Biology Ecological Studies, eds C. Wienke and K. Bischof (Verlag: Springer), 3–23. doi: 10.1007/978-3-642-28451-9_1
Harley, C. D. G., Anderson, K. M., Demes, K. W., Jorve, J. P., Kordas, R. L., and Coyle, T. A. (2012). Effects of climate change on global seaweed communities. J. Phycol. 48, 1064–1078. doi: 10.1111/j.1529-8817.2012.01224.x
Hepburn, C. D., Pritchard, D. W., Cornwall, C. E., Mcleod, R. J., Beardall, J., Raven, J. A., et al. (2011). Diversity of carbon use strategies in a kelp forest community: implications for a high CO2 ocean. Glob. Chan. Biol. 17, 2488–2497. doi: 10.1111/j.1365-2486.2011.02411.x
Hofmann, L. C., Heiden, J., Bischof, K., and Teichberg, M. (2014). Nutrient availability affects the response of the calcifying chlorophyte Halimeda opuntia (L.) J.V. Lamouroux to low pH. Planta 239, 231–242. doi: 10.1007/s00425-013-1982-1
Hurd, C. L., Harrison, P. J., Bischof, K., and Lobban, C. S. (2014). Seaweed Ecology and Physiology, 2nd Edn. Cambridge: Cambridge University Press.
Johnson, V. R., Russell, B. D., Fabricius, K. E., Brownlee, C., and Hall-Spencer, J. M. (2012). Temperate and tropical brown macroalgae thrive, despite decalcification, along natural CO2 gradients. Glob. Chan. Biol. 18, 2792–2803. doi: 10.1111/j.1365-2486.2012.02716.x
Koch, M., Bowes, G., Ross, C., and Zhang, X. (2013). Climate change and ocean acidification effects on seagrasses and marine macroalgae. Glob. Chan. Biol. 19, 103–132. doi: 10.1111/j.1365-2486.2012.02791.x
Koroleff, F. (1983). “Determination of phosphorus,” in Methods of Seawater Analysis: Second, revised and extended edition, eds K. Grasshoff, M. Ehrhardt, K. Kremling (Weinheim: Verlag Chemie), 125–139.
Martínez, B., Arenas, F., Rubal, M., Burgués, S., Esteban, R., García-Plazaola, I., et al. (2012). Physical factors driving intertidal macroalgae distribution: physiological stress of a dominant fucoid at its southern limit. Oecologia 170, 341–353. doi: 10.1007/s00442-012-2324-x
Oviedo, A. M., Ziveri, P., Álvarez, M., and Tanhua, T. (2015). Is coccolithophore distribution in the Mediterranean Sea related to seawater carbonate chemistry? Ocean Sci. Discuss. 11, 613–653. doi: 10.5194/osd-11-613-2014
Pérez-Lloréns, J. L., Vergara, J. J., Pino, R. R., Hernandez, I., Peralta, G., and Niell, F. X. (1996). The effect of photoacclimation on the photosynthetic physiology of Ulva curvata and Ulva rotun data (Ulvales, Chlorophyta). Eur. J. Phycol. 31, 349–359. doi: 10.1080/09670269600651581
Pettit, L. R., Smart, C. W., Hart, M. B., Milazzo, M., and Hall-Spencer, J. M. (2015). Seaweed fails to prevent ocean acidification impact on foraminifera along a shallow-water CO2 gradient. Ecol. Evol. 5, 1784–1793. doi: 10.1002/ece3.1475
Pierangelini, M., Stojkovic, S., Orr, P. T., and Beardall, J. (2014). Elevated CO2 causes changes in the photosynthetic apparatus of a toxic cyanobacterium, Cylindrospermopsis raciborskii. J. Plant Physiol. 171, 1091–1098. doi: 10.1016/j.jplph.2014.04.003
Pierrot, D. E., and Wallace, D. W. R. (2006). MS Excel Program Developed for CO2 System Calculations. ORNL/CDIAC-105a. Carbon Dioxide Information Analysis Center, Oak Ridge National Laboratory. Oak Ridge, TN: U.S. Department of Energy.
Porzio, L., Buia, M. C., and Hall-Spencer, J. M. (2011). Effects of ocean acidification on macroalgal communities. J. Exp. Mar. Biol. Ecol. 400, 278–287. doi: 10.1016/j.jembe.2011.02.011
Raven, J. A., and Beardall, J. (2014). CO2 concentrating mechanisms and environmental change. Aquat. Bot. 118, 24–37. doi: 10.1016/j.aquabot.2014.05.008
Raven, J. A., Beardall, J., and Giordano, M. (2014). Energy costs of carbon dioxide concentrating mechanisms in aquatic organisms. Photosynth. Res. 121, 111–124. doi: 10.1007/s11120-013-9962-7
Rodrigues, M. A., Dos Santos, C. P., Young, A. J., Strbac, D., and Hall, D. O. (2002). A smaller and impaired xanthophyll cycle makes the deep sea macroalgae Laminaria abyssalis (Phaeophyceae) highly sensitive to daylight when compared with shallow water Laminaria digitata. J. Phycol. 38, 939–947. doi: 10.1046/j.1529-8817.2002.t01-1-01231.x
Roleda, M. Y., Morris, J. N., McGraw, C. M., and Hurd, C. L. (2012). Ocean acidification and seaweed reproduction: increased CO2 ameliorates the negative effect of lowered pH on meiospore germination in the giant kelp Macrocystis pyrifera (Laminariales, Phaeophyceae). Glob. Chan. Biol. 18, 854–864. doi: 10.1111/j.1365-2486.2011.02594.x
Roy, R. N., Roy, L. N., Vogel, K. M., Porter-Moore, C., Pearson, T., Good, C. E., et al. (1993). The dissociation constants of carbonic acid in seawater at salinities 5 to 45 and temperatures 0 to 45° C. Mar. Chem. 4, 249–267. doi: 10.1016/0304-4203(93)90207-5
Russell, B. D., Pasasrelli, C. A., and Connell, S. D. (2011). Forecasted CO2 modifies the influence of light in shaping subtidal habitat. J. Phycol. 47, 744–752. doi: 10.1111/j.1529-8817.2011.01002.x
Russell, B. D., Thompson, J. I., Falkenberg, L. J., and Connell, S. D. (2009). Synergistic effects of climate change and local stressors: CO2 and nutrient-driven change in subtidal rocky habitats. Glob. Chan. Biol. 15, 2153–2162. doi: 10.1111/j.1365-2486.2009.01886.x
Scherner, F., Horta, P. A., Oliveira, E. C., Simonassi, J. C., Hall-Spencer, J. M., Chow, F., et al. (2013). Coastal urbanization leads to remarkable seaweed species loss and community shifts along the SW Atlantic. Mar. Poll. Bull. 76, 106–115. doi: 10.1016/j.marpolbul.2013.09.019
Schreiber, U., Endo, T., Mi, H., and Asada, K. (1995). Quenching analysis of chlorophyll fluorescence by the saturation pulse method: particular aspects relating to the study of eukaryotic algae and cyanobacteria. Plant Cell. Physiol. 36, 873–882.
Strain, E. M. A., Thomson, R. J., Micheli, F., Mancuso, F. P., and Airoldi, L. (2014). Identifying the interacting roles of stressors in driving the global loss of canopy-forming to mat-forming algae in marine ecosystems. Glob. Chan. Biol. 20, 3300–3312. doi: 10.1111/gcb.12619
Swanson, A. K., and Fox, C. H. (2007). Altered kelp (Laminariales) phlorotannins and growth under elevated carbon dioxide and ultraviolet-B treatments can influence associated intertidal food webs. Glob. Chan. Biol. 13, 1696–1709. doi: 10.1111/j.1365-2486.2007.01384.x
Yesson, C., Bush, L. E., Davies, A. J., Maggs, C. A., and Brodie, J. (2015). Large brown seaweeds of the British Isles: evidence of changes in abundance over four decades. Estuar. Coast. Shelf. S. 155, 167–175. doi: 10.1016/j.ecss.2015.01.008
Keywords: ocean acidification, macroalgae, photosynthesis, phenolic compounds, nutrient availability
Citation: Celis-Plá PSM, Hall-Spencer JM, Horta PA, Milazzo M, Korbee N, Cornwall CE and Figueroa FL (2015) Macroalgal responses to ocean acidification depend on nutrient and light levels. Front. Mar. Sci. 2:26. doi: 10.3389/fmars.2015.00026
Received: 05 March 2015; Accepted: 04 May 2015;
Published: 22 May 2015.
Edited by:
Iris Eline Hendriks, University of the Balearic Islands, SpainReviewed by:
Peng Jin, King Abdullah University of Science and Technology, Saudi ArabiaDorte Krause-Jensen, Aarhus University, Denmark
Copyright © 2015 Celis-Plá, Hall-Spencer, Horta, Milazzo, Korbee, Cornwall and Figueroa. This is an open-access article distributed under the terms of the Creative Commons Attribution License (CC BY). The use, distribution or reproduction in other forums is permitted, provided the original author(s) or licensor are credited and that the original publication in this journal is cited, in accordance with accepted academic practice. No use, distribution or reproduction is permitted which does not comply with these terms.
*Correspondence: Paula S. M. Celis-Plá, Department of Ecology, Faculty of Science, University of Málaga, Campus teatinos s/n, 29016 Málaga, Spain,cGF1bGFjZWxpc3BsYUB1bWEuZXM=