- 1Faculty of Veterinary Medicine, University of Ibadan, Ibadan, Nigeria
- 2Department of Pharmaceutical Microbiology, Pharmacy, University of Ibadan, Ibadan, Nigeria
- 3Biotechnology Division, Rubber Research Institute of Nigeria, Benin City, Nigeria
- 4Research and Development, Healthy Africans Platform, Ibadan, Nigeria
- 5Department of Veterinary Biochemistry, Nigeria College of Veterinary Medicine, University of Ibadan, Ibadan, Nigeria
- 6Department of Epidemiology and Population Health, School of Public Health and Information Sciences, University of Louisville, Kentucky, KY, United States
- 7Department of Biochemistry, Federal, Abeokuta, Nigeria
- 8Department of Physiology, University of Ibadan, Ibadan, Nigeria
- 9Department of Microbiology, Chuckuemeka Odumegwu Ojukwu University, Anambra State, Anambra, Nigeria
The (re)emergence of several infectious zoonoses underlines the need for the re-evaluation of the transmission patterns and key players responsible for effective inter-species transfer of diseases. Anthrax is caused by Bacillus anthracis, a zoonotic rod-shaped, Gram-positive, spore-forming bacterium that is highly fatal to both human and animal populations. B. anthracis is widespread across several regions of the world, including Africa, Asia, southern Europe, North and South America, and Australia, and it has a remarkably high attendant impact on the sustainability and profitability of livestock. The current trend in the global distribution of anthrax necessitates an urgent contextual understanding of the key drivers of the spread of B. anthracis in different parts of the world toward the end goal of an anthrax-free world. The understanding of the drivers is integral for the development of control and preventive measures, and also the development of agents such as therapeutics and vaccines against B. anthracis. This review presents a holistic description of the transmission pattern and epidemiology of B. anthracis, and updates on the diagnostic techniques and approaches available for the detection of B. anthracis. In addition, this review highlights plausible prevention and control strategies for the bacterium. This review further underscores the need for participatory epidemiology, hygiene, and safety protocols, the establishment of comprehensive surveillance systems, and global collaborative efforts toward vaccine development as critical steps in controlling anthrax.
1 Introduction
Anthrax is an important pathogenic disease caused by Bacillus anthracis, a zoonotic rod-shaped, Gram-positive, spore-forming bacterium (1–3). The history of anthrax dates to the 1870s; it was during this period that Robert Koch discovered the bacterium. Other researchers including Louis Pasteur, Élie Metchnikoff, Max Sterne, and Harry Smith, to mention a few, contributed immensely to the current understanding of B. anthracis, including its phagocytic characteristics, zoonotic nature, toxin production, and dispersal (1–3). Interestingly, B. anthracis has also been established as a bioweapon, and was used as such for the first time in the United States in an incident in 2001 when anthrax spores were mailed to certain government officials. This was the first recorded intentional use of a bacterium as a bioweapon and was associated with the infection of 22 people, including 12 mail handlers, and the death of five people (Antonio, 2013). B. anthracis is now classified as a tier 1 biological agent as there is a very high risk associated with its misuse and it has significant potential to cause mass casualties or have a devastating effect on the economy, critical infrastructure, or public confidence; therefore, it poses a severe threat to public health and safety (4, 5; Zacchia and Schmitt, 2001). This review aims to elucidate the different host, environment, and pathogen factors contributing to the persistence and spread of B. anthracis and the occurrence of anthrax in the environment. This is pertinent because of the current wave of anthrax across both developing and developed nations across the world. Furthermore, the persistence and possible increase in trends of global warming in complicating the (re)emergence of anthrax warrant an urgent need for public understanding of the dynamics of anthrax in the environment.
1.1 Bacillus anthracis: phylogenetic characteristics
Bacillus anthracis belongs to the Firmicutes phylum in the family Bacillaceae, genus Bacillus, and Bacillus cereus group. The B. cereus group consists of B. anthracis, B. cereus, B. mycoides, B. pseudomycoides, B. thuringiensis, B. weihenstephanensis, and B. cytotoxicus, all of which are closely related phenotypically, pathogenically, symptomatically, ecologically, and also in terms of host preference (2, 3, 6, 7). Phylogenetically, the B. cereus group of bacteria is divided into three distinct clades that show partial barriers to interclade gene flow. Strains of B. anthracis that are highly clonal are restricted to clade 1, together with the highly virulent emetic toxin (cereluid synthetase)-producing B. cereus strains (3).
The most important member of the group is B. anthracis, which has two large plasmids encoding the most important virulence factors (i.e., toxins and capsule) (3). The cells of B. anthracis are rod shaped and quite large, with the rods ranging in size from 1 × 3 μm to 1.3 × 10 μm (1–3).
Bacillus anthracis primarily infects ungulates and other mammals, including humans (8–11). The toxins produced by B. anthracis are encoded on a pathogenicity island in the 183-kb pXO1 plasmid, whereas the poly-γ-D-glutamic acid capsule is synthesized by the capBCADE operon located in the 96-kb pXO2 plasmid (7; Antonio, 2013; 12–14) (Figure 1).
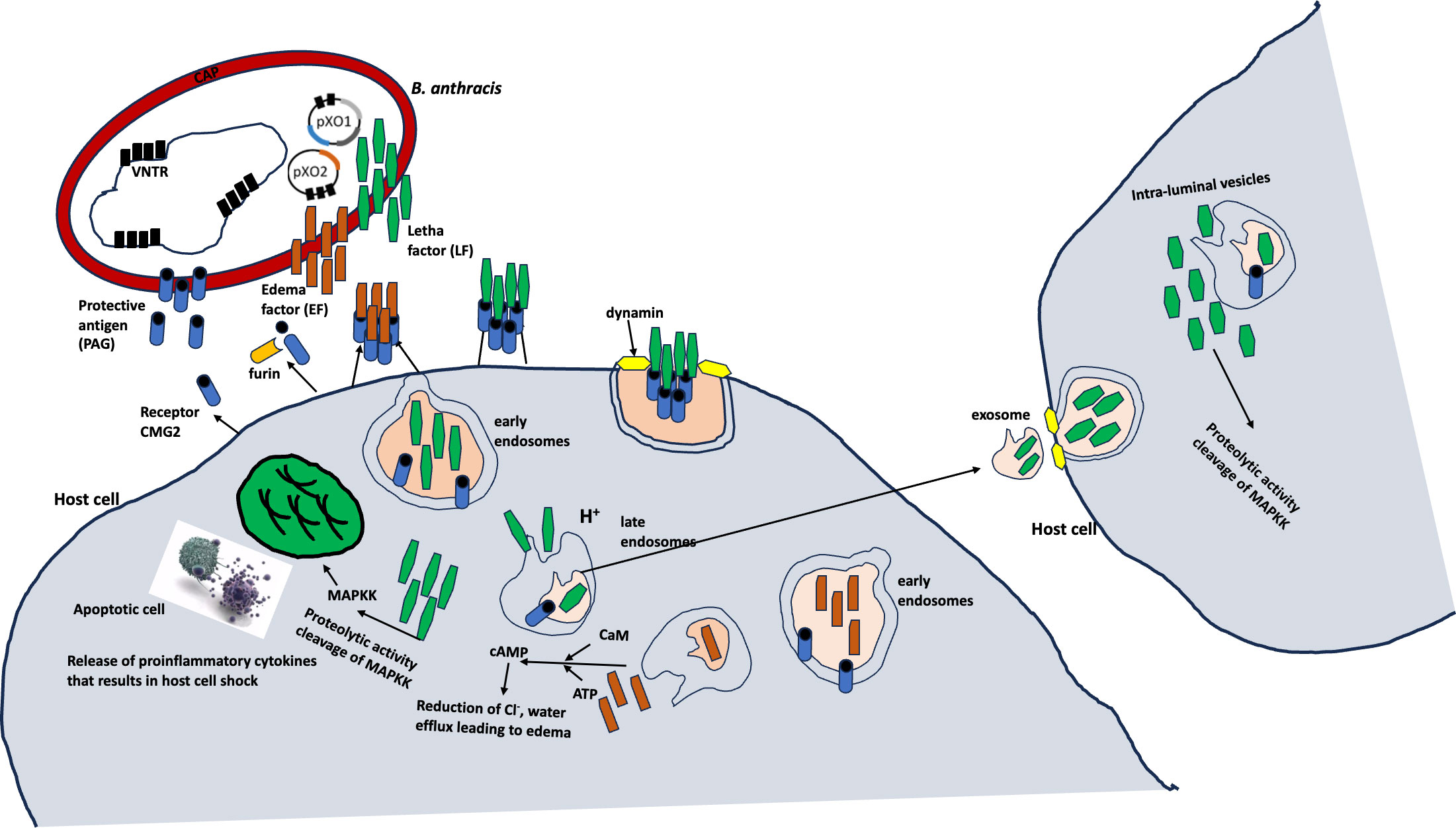
Figure 1 Molecular mechanisms of B anthracis (7).
1.2 Methodology
The research methodology for this review was accessed by reviewing papers downloaded from Scopus® (Elsevier, Amsterdam, the Netherlands), ScienceDirect® (Elsevier), Google Scholar (Google Inc., Mountain View, CA, USA), PubMed® (National Library of Medicine, Bethesda, MD, USA), and Embase® (Elsevier). These databases were searched for the keywords “Anthrax”, “Biological Agents”, and “Public Health Implications” in papers published between 1940 and 2023.
2 Epidemiology and transmission of anthrax
Bacillus anthracis exists in two distinct forms (phases), known as the vegetative and sporulated forms/phases (7). This is a unique attribute of B. anthracis, as it exists principally in the spore form in the environment, where it can be dormant without replication over a long time (15). The environmental maintenance of anthrax over a long time is the least understood part of its life cycle. Despite the integral role of the environment, knowledge about the survival and activity of B. anthracis outside a host is still limited.
2.1 Environmental reservoir
Many pieces of evidence have revealed the persistence of anthrax spores in the environment over several years, especially in soil with a slightly alkaline pH and a higher organic matter and calcium content (Turner et al., 2016, 16, Williams et al., 2013). The germination of the spores into the vegetative form occurs in the host when the spores are taken up via inhalation, ingestion, and cutaneous means (7, 15; Turner et al., 2016). The germination and replication of B. anthracis spores in the host are potentiated by the ability of the bacterium to escape the host’s immune cells due to its toxin and capsule production (17). These virulence factors are integral to not only the B. anthracis invasion but also to the systemic dissemination through regional lymph nodes associated with infection sites (5). The disseminated B. anthracis grows exponentially, leading to large numbers in the bloodstream, which in turn contributes to a rapid lethality due to the high volume of toxin production.
The toxins produced by B. anthracis are three polypeptides that form two classical types, that is, lethal and edema toxins. The lethal (LF) and edema (ET) toxins have similar receptor-binding components and protective antigens. LF is a zinc metalloprotease (18–20), and EF is a calmodulin-dependent adenylate cyclase (18–20). The toxins target cellular pathways, inhibiting the host’s response and causing lethality.
2.2 Natural infections in herbivores
Anthrax occurs most commonly in nature when herbivores feed on contaminated vegetation with B. anthracis spores from previously dead animals (9–11). Anthrax fatality is caused by acute intoxication and massive bacteremia resulting from the progression of the three basic disease forms including cutaneous, gastrointestinal, and inhalation, which corresponds to the route of exposure, mostly in humans.
The cutaneous form is the most common and fatal anthrax form, which is associated with edema and necrosis and the consequential black eschar sign in infected individuals; it has a mortality rate of approximately 20% in untreated individuals (21). The gastrointestinal form is considered rare and is characterized by flu-like symptoms. The inhalation form is uniquely important because of the relative ease of transmission of the B. anthracis spores in the environment in an aerosolized form, rapid progression of the diseases caused by them, and potential mortality of up to 100% associated with it, which is similar to that of a classical bioweapon bacterium. The inhalation form is also important because of its non-specific symptoms, usually flu-like in nature, and consequentially the possibility of its leading to sepsis and septic shock, such as vacuolar collapse (5, 11, 21).
2.3 Occurrences of anthrax
The occurrence and epidemiology of anthrax globally can be captured within the context of the disease outbreak occurring across different nations of the world. Historically, the occurrence of anthrax dates back to 1979 with an outbreak in Sverdlovsk, Russia, an incident believed to have been caused by the accidental release of B. anthracis spores from a secret military facility, leading to over 100 people being exposed to the spores, and to at least 64 dying within a few weeks of exposure (22). In the early 2000s, the most popular US case, which is known to be a terror attack on the political classes, occurred with anthrax spores reportedly being mailed to several news media offices targeting US senators, eventually leading to the death of five people and the infection of approximately 17 people (Antonio, 2013; 4, 5; Zacchia and Schmitt, 2001). In India, human cases of anthrax affecting approximately seven people were reported in the largest outbreak of anthrax in 2014 (23) (Figure 3).
Furthermore, in Russia, nearly 100 people were hospitalized owing to an anthrax outbreak among nomads. In Ukraine, between 2005 and 2022, a total of 267 cases of anthrax were reported (approximately 251 cases reported in domestic animals and 16 cases reported in wild animals), of which 28 cases were confirmed positive for B. anthracis (24). According to the World Organisation for Animal Health (WOAH), the highest number of cases was reported in 2018 (WOAH, 2018). Interestingly, most of these reported cases were confirmed from samples obtained from the soil which were believed to have been potentiated by the presence of several biothermal pits and burial grounds of cattle. These pockets of outbreaks underscore the global distribution of anthrax across many continents of the world (Figure 2).
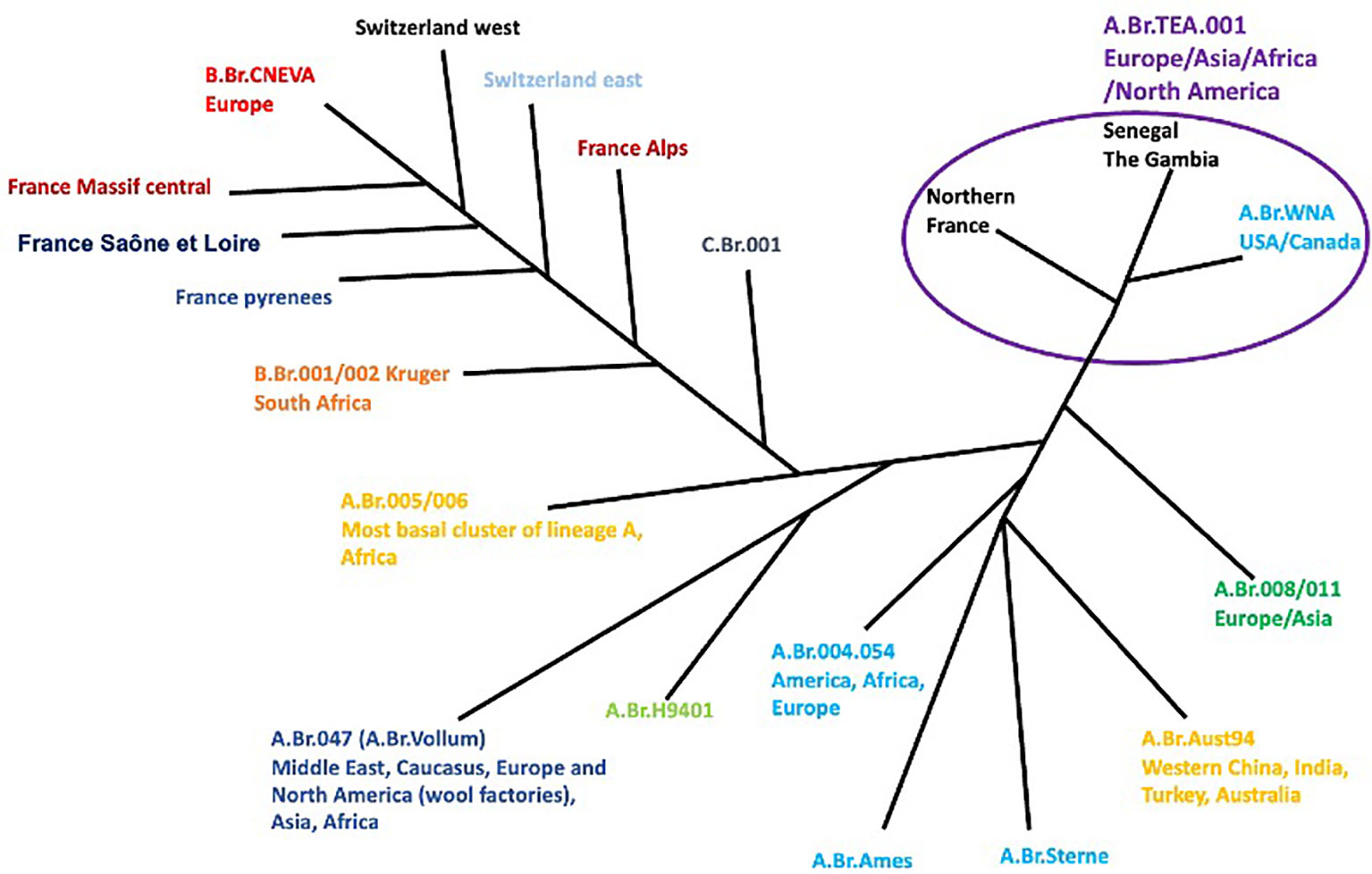
Figure 2 Phylogeography of worldwide B anthracis strains (7).
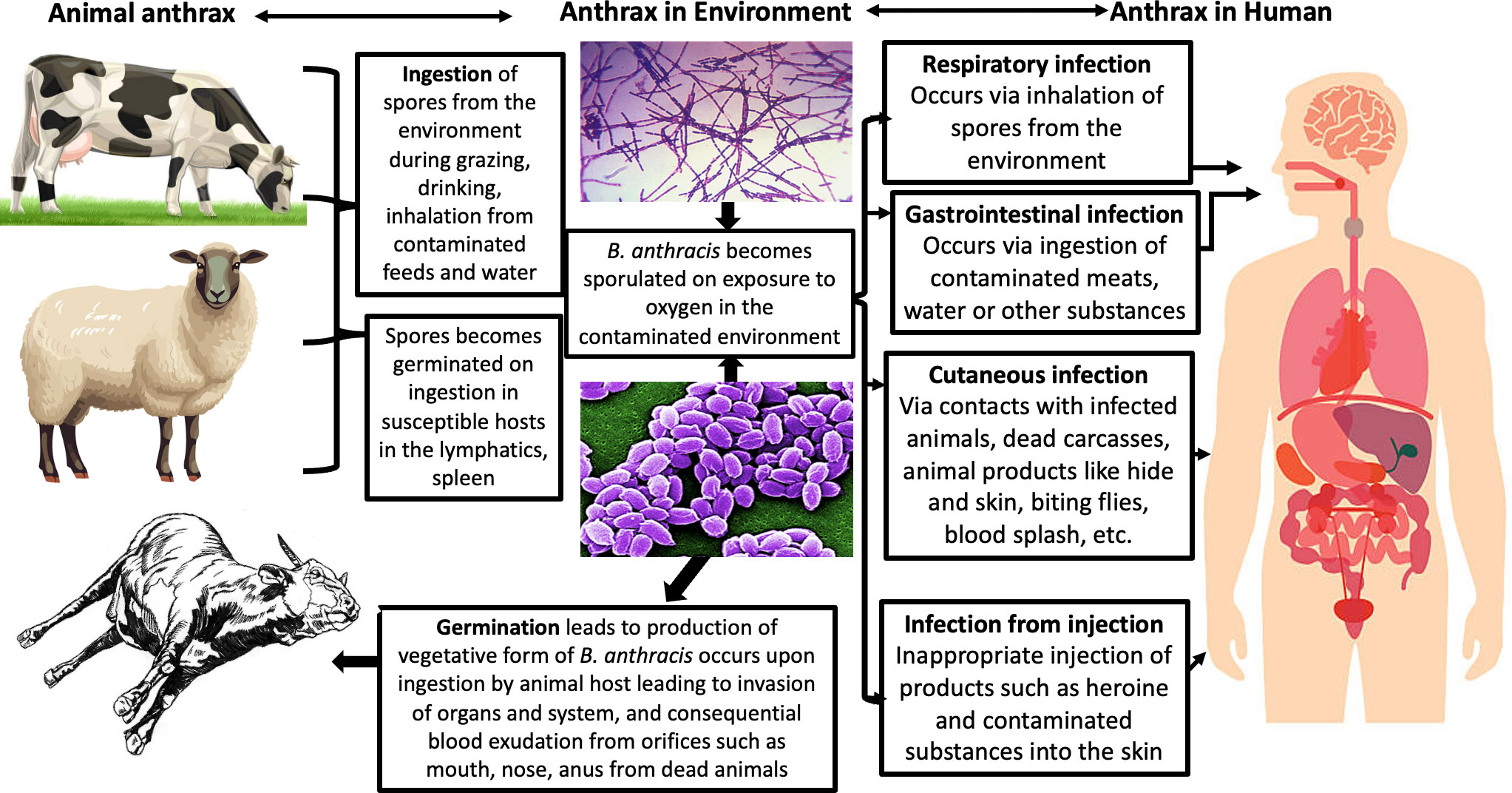
Figure 3 Transmission of anthrax (25).
In recent news, there are currently global panics underscored by more rapid spread and more diverse occurrences/reports of anthrax in different nations of the world across Africa, America, Asia, and Australia (Ndolo et al., 2020). In the United States, according to the WOAH monthly update, the state of Minnesota confirmed its first case of anthrax in a decade in Kittson County, which affected multiple cattle and a horse, and the Grant County of North Dakota State reported its first case in 2023 in cattle belonging to a Grant County beef herd.
Currently, Canada has reported confirmed 18 cases (WOAH, 2023). Indonesia has reported approximately 12 deaths consisting of six cattle and six goats, with approximately 85 people testing positive but asymptomatic (WOAH, 2023). In Spain, there are currently approximately 65 suspected cases, with six confirmed deaths in cattle (WOAH, 2023). According to the confirmatory tests obtained from the National Veterinary Research Institute (NVRI), VOM, Plateau State, Nigeria, Nigeria’s index case has been identified, which is characterized by the sudden death of eight animals (including cattle, sheep, and goats) with observed bleeding from orifices without clots (26).
In Ghana, there are currently approximately 97 confirmed cases of anthrax across six districts (WOAH, 2023). Furthermore, Kenya has reported approximately 15 cases so far, with three deaths across five sub-counties. Underscoring the zoonotic potential of anthrax, Russia confirmed human infection in Tuva affecting approximately six people with a history of visitation to a shepherd’s camp and contact with animals reported not to have been vaccinated (WOAH, 2023). The current global trends and distribution associated with the outbreaks of anthrax between 2022 and 2023 alone are worrisome and warrant increasing the awareness of the populace. The presence of anthrax across national and continental borders portends its importance and the high risks it poses to both human and animal populations. Increasing global movement and the existence of climate change characterized by increasing changes in temperature is another possible driver of anthrax globally (WOAH, 2023).
3 Formation of biofilms as a mechanism of Bacillus anthracis virulence
Biofilms are complex, multicellular communities of microorganisms that attach to surfaces and are encompassed in a self-produced extracellular polymeric matrix, which mainly consists of proteins, nucleic acids, and polysaccharides (27, 28). A key feature of bacterial biofilms is their contribution to bacterial virulence through the promotion of survival and persistence in hostile environments, enhancing resistance to antimicrobial agents, and facilitating chronic infections (29, 30).
Bacillus anthracis has been reported to readily form robust biofilms both under both static and shear conditions (31–33). The United States Environmental Protection Agency (EPA) reported that biofilm formation is one of the mechanisms utilized by B. anthracis to remain persistent in the soil. Furthermore, it is an essential aspect of its lifecycle and virulence (EPA, 2014). These biofilms can resist several antimicrobial agents, making B. anthracis particularly challenging to eradicate, which is a property independent of its sporulation function (31). The capability to form biofilms allows the pathogen to persist in the environment, facilitating the cycle of infection.
Moreover, research has demonstrated that the interaction between B. anthracis and bacteriophages leads to the emergence of lysogens, which are phenotypically altered bacteria with significantly improved survival capabilities. These lysogens are associated with enhanced exopolysaccharide expression and biofilm formation, further intensifying the resilience and survival capabilities of B. anthracis in soil and also in anoxic earthworm guts (33, 34).
On a molecular level, Ser/Thr protein kinase (PrkC) plays a critical role in the formation of B. anthracis biofilms. PrkC phosphorylates the chaperon protein GroEL, promoting interaction with GroES and facilitating the assembly and disassembly of structures critical to biofilm formation. Notably, the disruption of the PrkC function compromises biofilm formation, signifying the importance of the molecular signaling in this process (35).
Genetic studies by Schuch and Fischetti revealed that the genes bcp25, bcp26, wip38, and wip39 were instrumental in inducing high-level exopolysaccharide expression, leading to enhanced biofilm formation. This relationship was particularly evident in Bcp1 and Wip4 infections, signifying a strong correlation between genetic factors and biofilm development (33). Biofilm formation serves as a pivotal strategy in B. anthracis virulence by augmenting its survival in hostile environments, enabling its resistance to antimicrobial agents, facilitating its persistent environmental presence, and, ultimately, contributing to the persistent and chronic nature of anthrax infections.
4 Global warming as a driver of anthrax
The environment, especially the soil, is currently known as the most important reservoir for B. anthracis. B. anthracis characteristically alternates between the dormant sporulated state in the environment and the vegetative replicating state in the host (7, 15). Specifically, an ideal soil is characterized by low-lying depressions, or eroded shallow and deep rich alluvial soils with high organic and calcium contents and a pH ranging between 6 and 8 serves as a suitable condition for the growth of B. anthracis (15, 36). These factors play key roles in the maintenance and persistence of B. anthracis for a long period of time because they increase its resistance to heat and desiccation (7). These climatic factors also serve as enablers of adequate host–pathogen–environment interaction. Sporulation occurs when the bacterium encounters free oxygen in the air, especially as exudates are released from dead or dying animals (15).
The phenomenon of global warming has the potential to exert an influence on the occurrence and dispersion of infectious diseases such as anthrax. However, it is crucial to acknowledge that this relationship is characterized by a complex and varied nature. The bacterial disease known as anthrax is predominantly caused by the microorganism B. anthracis. The occurrence of this disease is determined by a complex interplay of various elements, including environmental conditions, ecological dynamics, and human activities. The potential linkage between global warming and anthrax is worth exploring (37).
The potential impact of global warming on anthrax can manifest through the process of permafrost thawing. In certain geographical areas, spores of anthrax have been found to remain viable within frozen soil for extended periods of time, spanning several decades or even millennia. With the increase in temperatures leading to the thawing of permafrost, there is a possibility of the release of these spores into the surrounding environment, which might potentially result in the exposure of both wild and domestic animals to the disease (15). The phenomenon of global warming has the potential to induce modifications within ecosystems, which manifest as alterations in vegetation patterns, shifts in wildlife migration routes, and changes in the accessibility of water resources. The alterations have the potential to impact the dispersion of anthrax throughout wildlife communities, given that bacterial spores can persist in the environment and are consumed by herbivorous animals (15).
The process of increased animal stress can be attributed to the escalation of temperatures and alterations in climate patterns. The presence of stress in wildlife and livestock has been observed to have a detrimental effect on their immune systems, rendering them more vulnerable to many illnesses, including anthrax. This phenomenon has the potential to result in a higher frequency of breakouts within the regions that are impacted (38). The expanded range of disease vectors can be influenced by global warming, leading to changes in their distribution and behavior. These vectors include insects and other arthropods. Although anthrax is not primarily transmitted by vectors, alterations in the distribution and behavior of these species can have an indirect impact on the ecological dynamics of the illness (37).
The impact of human activities on land usage, such as deforestation and alterations prompted by global warming, can also result in increased proximity among humans, cattle, and wildlife that may serve as carriers of anthrax. The increased level of interaction between humans and wildlife has the potential to facilitate a greater occurrence of anthrax transmission from wildlife to both humans and domestic animals (7).
It is imperative to acknowledge that the dynamics of anthrax are influenced by a multitude of causes, with global warming being one of them. The management of land utilization, implementation of livestock immunization programs, and enforcement of public health measures are pivotal in effectively mitigating the transmission of the illness. Comprehending and effectively resolving the intricate relationship among climate change, ecological variables, and infectious illnesses such as anthrax is of utmost importance in formulating efficacious methods to alleviate their negative impacts on both animal and human populations.
Temperature and precipitation both contribute to the factors affecting the sporulation of B. anthracis spores at the onset of an outbreak with varying prolonged periods of hot, dry weather that are usually followed by heavy rains and flooding, or with the onset of rains ending a period of drought (15, 37, 39). The seasonal dynamics, human activities, and animal density play key roles in the occurrence of anthrax-related outbreaks, making anthrax a more complicated disease for humans and animals.
Global climate change has become increasingly concerning as it continues to change the dynamics of disease outbreaks, including the (re)emergence of diseases. Climate change, specifically in the form of dramatic temperature increases and extreme weather, impacts both the terrestrial and aquatic ecosystems, changing soil composition and nutrient availability, vegetation, distribution of both wild and domestic animals, and also animal density and movements (16, 38). The increasing number of climate-related stresses on the environment contribute to the change in the host–pathogen interactions, which consequently causes a change in disease dynamics. Anthrax is able to gain momentum, especially in most grasslands, because of the fragility and vulnerability of grasslands to the consequences of climate change (40, 41). The continual needs of the grasslands and the density of livestock, including their movement in search of pasture during scarcity, serve as a driver that increases the risk of exposure of naive cattle to anthrax (37, 39).
The presence of suitable pH and organic carbon alongside calcium further aids the germination of the anthrax spores while also contributing to optimal grassland ecosystems and agricultural cultivation (15; Gaitanis et al., 2015, Wall et al., 2015; 37). This type of environment, which naturally attracts a high density of livestock because of the numerous pastures/vegetation utilizable by livestock, serves as an important springboard for an increased risk of anthrax.
Increasing temperature anomalies have been linked to increased suitability for B. anthracis, and they also contribute to water stress (15). The divergence of temperature over the years, especially around the latitudes, is now known to change habitat suitability across intercontinental regions and contribute immensely to the emergence of diseases such as anthrax. The warming temperature trends not only change grassland diversity and increase livestock movement, but also increase the melting rate of permafrost in the Arctic, which in turn leads to the release of spores from dead frozen animals into the environment (42, 43). These spores then become available for livestock for ingestion, and they germinate and thus cause infection. On another note, the warming temperature in the arid shrubland in the middle or southern latitudes, especially in the summer, favors the dissemination of anthrax spores, particularly when there is intermittent precipitation (Gaitanis et al., 2015, Wall et al., 2015). Furthermore, the increasing temperature also affects the host by increasing disease immunocompetence, which eventually increases disease susceptibility in the host (44). This is due to the significant impact of stress on both the host’s immunity and the enhanced chains of disease transmission.
Climate change is undoubtedly a huge contributor to the trends currently predominantly caused by anthrax. The significant roles of temperature and consequential stressful environmental conditions, including water stress and grassland as drivers of anthrax in a population, could be a major reason for the (re)emergence of anthrax beyond the norm (16, 45). The impact of climate change on infectious diseases is indeed inexhaustible, especially for anthrax, the distribution of which is driven by salient environmental changes. Climate change complicates anthrax more because of the force with which it exerts changes on animal and human distribution and movement, the availability of foods and consequential increasing search for foods, and the increasing exposure of humans and animals to anthrax spores.
5 Diagnosis of anthrax
5.1 Conventional methods of detecting Bacillus anthracis
Prompt and accurate identification is key to anthrax diagnosis. The accuracy of the employed diagnostic tool(s) determines the health outcomes of human and animal populations, especially in the context of the surveillance of anthrax. The detection of anthrax utilizes a combination of different tools which is based on the history of exposure, clinical presentations, and examinations. The identification of B. anthracis is carried out using laboratory methods such as culture, microscopy, radiology, DNA, and antigen and antibody detection methods.
The history of animal or human exposure to dead infected animals or tissues from them serves as one of the vital tools for anthrax diagnosis. Animals having a history of contact with infected animals or animal products during movement and grazing are presumptively diagnosed and must be handled carefully. The presence of signs of classical symptoms, including sudden death and signs of cutaneous, gastrointestinal, and respiratory lesions can be used to diagnose animals (9–11).
Conventional diagnostic methods, including bacterial culture, motility and hemolytic assays, staining methods, gamma lysis, and antibiotic susceptibility testing methods, are all integral, foundational, gold standard methods needed to make a reliable molecular diagnosis.
In brief, the clinical diagnosis of anthrax is based on classical signs, including cutaneous black eschar caused by the edema toxins around lesions, and also by other orogastric and inhalational clinical presentations, such as severe sore throat (5, 11, 21).
The microscopic detection of B. anthracis is another foundational technique that focuses on the bacteria’s characteristic phenotypic appearance, including its large size, rectangular shape, and the presence of spores and the antiphagocytic capsule, which can be observed using light microscopy or electron microscopy after Gram or Wright staining (Friedlander, 1999). This method reveals the presence of Gram-positive rod-shaped organisms, and the presence of a capsule which surrounds virulent forms of the bacterium can be confirmed using polychrome methylene blue or Indian ink, whereas staining with methylene blue reveals blue-black, square-tipped bacilli surrounded by a pink capsule (25).
5.1.1 Culture-based methods
These traditional techniques involve the isolation and identification of the bacterium through cultivation on selective media. Culture-based methods rely on the use of specific growth media to isolate B. anthracis. Blood agar, with or without added antimicrobial agents, is commonly employed as a primary selective medium. This allows the growth of B. anthracis while inhibiting the growth of other bacteria. Another selective medium is polymyxin-lysozyme-EDTA-thallous acetate (PLET) on which B. anthracis grows as a characteristic small, white, domed and circular colony, but this medium has been banned in some countries due to the highly toxic thallous acetate constituent (46, 47). The limited accessibility to these cultured-based methods indicates the need for novel methods for the prompt diagnosis of anthrax. Other media, such as R & F® anthrax chromogenic agar (ChrA) containing cycloheximide, polymyxin B, and X-indoxyl-choline phosphate (X-CP), are currently being employed as alternatives to PLET (Doganay, 2017; 25). The growth of B. anthracis on agar plates with characteristic colonies appearing large, smooth, and opaque, with a characteristic ground-glass appearance is diagnostic (25). The colonies may also exhibit hemolysis on blood agar, known as the “Medusa head” effect, due to the bacterium’s ability to produce the anthrax toxin.
Gram staining is a valuable technique in culture-based methods for detecting B. anthracis. The bacterium appears as large, Gram-positive rods under a microscope. This staining pattern, combined with colony morphology, aids in the preliminary identification of the pathogen. The B. anthracis capsules formed to escape phagocytic activity in the host can be detected using the M’Fadyean staining procedure, which causes the bacteria to appear as blue-black, square-ended cells with pink capsules.
Biochemical tests are often employed to confirm the presence of B. anthracis. These tests assess various metabolic properties, such as the ability to ferment specific sugars or produce characteristic enzymes. For instance, B. anthracis typically exhibits positive reactions for nitrate reduction, lecithinase activity, and gelatin hydrolysis. Furthermore, characteristics such as gamma phage susceptibility, catalase production, a lack of motility, a lack of hemolytic activity when cultured on blood agar, and susceptibility to penicillin are also detectable using biochemical tests (Martin et al., 2020). The needs for molecular diagnosis arise based on several reasons, including the closeness of B. anthracis to related species such as B. cereus both phenotypically and genetically, and also phenotypical association with others such as B. thuringiensis, B. mycoides, B. pseudomycoides, and B. weihenstephanensis (47). The similarities span across phenotypic, genetic, and plasmid characteristics (48). In addition to the species-level challenges, the diagnosis of B. anthracis is also affected by clinical presentations and the presence of the related bacteria in the environment, just as it is for B. anthracis. For example, the presence of spores in the environmental samples requires more detailed examination and characterization using advanced tools for differentiating between B. anthracis and other closely related species. This is vitally important because the closely related spores also possess similar environmental resistance characteristics against temperature, radiation, common disinfectants, and many other chemicals.
5.2 Molecular detection of Bacillus anthracis
Molecular methods have revolutionized the field of pathogen detection, including the identification of B. anthracis (49). These techniques rely on the detection of specific genes unique to the bacterium using targets such as DNA, antigens, or antibodies.
The techniques based on antigens–antibodies include the principles of several immunoassays that contribute to the identification and characterization of B. anthracis. These methods identify components of B. anthracis such as the glycoprotein BclA (present in the spores), the oligosaccharide epitopes of BclA, the extracellular antigen 1 EA1 (S-layer component) and protective antigen PA, the anthrax toxin component, and the poly-D-glutamic capsule (47, 50–53). The identification techniques based on antigens–antibodies include tools such as enzyme-linked immunosorbent assays (ELISAs), flow cytometric assays combined with fluorescein-labeled antibodies and FRET (Förster resonance energy transfer), the Luminex assay, magnetic particle fluorogenic immunoassays (MPFIAs), ABICAP immunofiltration, lateral flow assays, and biosensors (53–58). The existing knowledge base on anthrax has been built through the use of these tools to identify several cellular immunity mechanisms and quantify immune responses in response to anthrax infections. Even when disease outbreaks have not occurred, immunoassays can be used to identify the immunological components that demonstrate possible exposure to B. anthracis and prompt interventions, such as therapeutics and vaccinations, alongside biosecurity measures to prevent the exposure of naive populations to B. anthracis.
5.2.1 Polymerase chain reaction
Conventional PCR and real-time PCR utilize knowledge of DNA identification and amplification of genetic composite of B. anthracis, thus allowing its differentiation from related bacteria (59). PCR and RT-PCR (reverse transcription polymerase chain reaction) help to identify the virulent genes of B. anthracis serving as both genetic and chromosomal markers. These techniques have been demonstrated to have high specificity and sensitivity is identifying the virulent factors of B. anthracis encoded in the plasmids, such as pXO1 and pXO2. The factors pXO1 and pXO2 are key components that modulate the anthrax toxin (protective antigen, edema factor, and lethal factor) production located on pXO1 and genes coding the capsule located on pXO2 (Makino et al., 1993). Some of the genes, including Ba813, BA-5449, ORF vrrA, and rpoB have been successfully identified using PCR (60; 47, 61–63). Furthermore, the applications of DNA sequencing also contribute immensely toward the detection of B. anthracis using specific single-nucleotide polymorphisms (SNPs) and minor groove-binding (MGB) probes that are specific to the point mutation in the plcR gene of B. anthracis, among many others (Matero et al., 2005; 47, 53).
In addition to PCR, DNA amplification in isothermal conditions, such as loop-mediated isothermal amplification (LAMP), rolling circle amplification (RCA), recombinase polymerase amplification (RPA), strand displacement amplification (SDA), single-primer isothermal amplification (SPIA), and polymerase spiral reaction (PSR) helicase-dependent amplification (HDA), and isothermal and chimeric primer-initiated amplification of nucleic acids (ICAN), has been demonstrated (47). Several studies have also demonstrated the application of these techniques in the identification of genetic and chromosomal markers such as pag (pXO1), capB (pXO2), capC (pXO2), lef (pXO1), and Ba813, BA_5345, and sap. (53, 64–66). DNA-based identification techniques improve our understanding of anthrax and also the detection of it in materials present in the environment or live tissues, even if an outbreak, has not occurred. These tools allow us to predict disease outbreaks and tailor efforts toward establishing preventive and control measures that mitigate future occurrences of anthrax. These tools can also help in decision-making regarding instituting relevant policies to contain affected areas and animals and preventing the exposure of more susceptible animals and human populations to B. anthracis (53).
The application of immunoassays and DNA-based methods provides unique advantages in terms of diagnosis, but partly have time limitations. The development and application of rapid test kits have been helpful in the prompt and early detection of B. anthracis with high sensitivity and specificity. Some of the test kits include but are not limited to nucleic acid probes and genosensors, antibody probes and immunosensors, aptamers, and peptide–nucleic acid chimera probes. Although nucleic acid-based probes work in accordance with the principles mentioned above, they bind to DNA markers more quickly and easily, thus enabling prompt diagnosis. The nucleic acid probe and genosensor methods have been demonstrated in the identification of DNA markers such as lef (pXO1) and pagA. (47). They mostly act by developing specific probes that have the capability to identify the specific genetic composites of B. anthracis. Similarly, the antibody probes and immunosensors identify antibodies of B. anthracis by binding to the antigens of the bacterium. Furthermore, the aptamers and peptide–nucleic acid chimera probes utilize the principles of interactions between single-stranded DNA or RNA onto different targets such as microorganisms and toxins (47, 53). The use of spectrometry has become very useful in the identification of B. anthracis in recent years. Matrix-assisted laser desorption ionization time-of-flight (MALDI-TOF) mass spectrometry (MS) facilitates easy diagnosis and identification of B. anthracis from various samples such as the blood of live tissues, environmental materials, and clinical samples (67, 68; Zasada et al., 2013; 47, 53).
PCR, LAMP, RPA, and NGS techniques enable rapid, sensitive, and specific detection of the bacterium, thereby significantly reducing the time required for diagnosis, while also improving our understanding of B. anthracis diversity and evolution. Molecular methods continue to advance with the integration of portable and point-of-care devices, enhancing their applicability in various settings, including field surveillance, outbreak investigations, and biodefense. However, it is important to ensure that appropriate quality control measures are in place and access to trained personnel for the accurate interpretation of molecular results is provided.
5.3 Spectrometric methods
Matrix-assisted laser desorption ionization time-of-flight (MALDI-TOF) mass spectrometry uses a database of mass spectra of different microbes in specific media to conduct their identification (47). B. anthracis has been successfully identified using this technique by Pauker et al. (68) and Dybwad et al. (2013), but not without cases of false positives and false negatives. This approach is very fast, economical, and efficient; however, it needs the isolate to be in pure culture; hence, it cannot be used for clinical and environmental samples.
6 Future challenges
Bacillus anthracis poses a persistent threat to public health, animal populations, and national security. As we look to the future, several factors contribute to the ongoing concern surrounding B. anthracis. B. anthracis has been weaponized in the past and remains a potential bioweapon. Its ability to form highly resilient spores and the devastating impact of anthrax on human health make it an attractive option for bioterrorism (69).
The continuous monitoring of antimicrobial susceptibility patterns is essential, as B. anthracis may develop resistance to current treatment options. The emergence of drug-resistant strains could complicate disease management and limit effective therapeutic interventions. Bruce et al., 2021 identified 10 antimicrobial resistance genes (bla1, fosB, bla2, vmlR, mphL, cfrC, oxa-59, dfrG, tem-116, and bcll) in 356 genomes of B. anthracis from GenBank, which further corroborates the need for a more careful antimicrobial stewardship approach.
The persistence of B. anthracis spores in soil and animal remains presents a significant challenge (59). Environmental reservoirs allow the sporadic reemergence of anthrax, leading to sporadic outbreaks and potential zoonotic transmission, as spores can survive for more than 10 years in soil, which can trigger the reemergence of the disease many years after the death of the host (59, 70). Shifting climate patterns, including increased temperatures and extreme weather events, may influence the geographic distribution of B. anthracis. These changes could expand the areas conducive to anthrax outbreaks and introduce the disease to new regions.
7 Recommendations and conclusion
The prevention of anthrax has been proven achievable through vaccination against the anthrax toxin components of the disease. The anthrax vaccine approved by the US Food and Drug Administration (FDA) is approved for use in three groups of adults aged 18 years to 65 years who may be at risk of encountering anthrax because of their job. The vaccine can be administered in two different situations: routine occupational use (before possible exposure) and post-event emergency use (after possible exposure) (21, 71–73). The prevention and management of anthrax necessitate the implementation of a multifaceted approach encompassing hygienic measures, surveillance, the avoidance of contact with diseased animals or contaminated substances, educational initiatives, heightened awareness, and the use of antibiotics. Anthrax is a pathogenic bacterial infection attributed to the microorganism B. anthracis. While its primary impact is observed in animals, it is also capable of infecting human hosts.
In terms of hygiene, when engaging in activities involving animals or their derived products, it is imperative to adhere to appropriate hygiene and safety protocols. This entails the utilization of protective attire, such as clothing, gloves, and masks, during the manipulation of items that may be contaminated. When ingesting animal-derived items such as meat, it is imperative to ensure that they are cooked completely so that any potential spores of bacteria are eliminated. It is also advisable to refrain from consuming raw or undercooked meat and dairy products, particularly those of uncertain origin.
For surveillance, it is important to establish and deploy a comprehensive surveillance system to identify and track the occurrence of outbreaks in both animal and human populations. The rapid identification of a condition or issue is crucial for prompt intervention, as is encouraging healthcare providers and veterinarians to promptly report any suspected instances of anthrax to the appropriate local health authorities.
For the act of refraining from making connections or engaging in interpersonal interactions, it is advisable to refrain from any form of interaction with animals that are infected. It is important to avoid handling or having close proximity to animals that are unwell or deceased, particularly when there is suspicion of their having anthrax. It is also advisable to refrain from contacting soil or materials that may be contaminated and to exercise caution when working in regions where diseased animals have been present or when handling substances such as bone meal or animal hides that could potentially harbor anthrax bacteria.
Public awareness campaigns are important because they disseminate information to the general population, particularly in regions where anthrax is prevalent, regarding the potential hazards linked to the disease and effective preventive measures against it. It is imperative to ensure that healthcare practitioners possess comprehensive knowledge on the clinical manifestations of anthrax and the corresponding treatment guidelines.
It is important to note that anthrax is a very uncommon occurrence in numerous regions across the globe, and the appropriate preventive actions may differ depending on the local prevalence and associated risk factors. It is therefore advisable to seek information from local health authorities and specialists regarding region-specific measures for the prevention and control of anthrax. Moreover, it is imperative to adhere to the prescribed vaccination schedules for livestock in regions where anthrax poses a recognized threat to mitigate the occurrence of animal outbreaks, hence diminishing the potential for human exposure to the disease.
To mitigate the bioweapon threat posed by B. anthracis, it is crucial to implement comprehensive measures aimed at prevention and preparedness. It is also recommended that the security measures and regulations for handling and storing B. anthracis cultures and spores are enhanced. Strict control and oversight should be enforced to prevent unauthorized access and theft from laboratories or research facilities. In addition, implementing robust surveillance systems to monitor the acquisition, transport, and potential misuse of B. anthracis to enable the timely detection of suspicious activities and effective sharing of intelligence among relevant agencies is vital.
Additionally, it is important to provide comprehensive training to laboratory personnel on biosafety practices, including the proper handling, storage, and disposal of B. anthracis and when doing so, it is important to lay emphasis on the need to strictly adhere to biosafety protocols and conduct regular risk assessments. It is also recommended that the public are educated about anthrax, its transmission, and the signs and symptoms associated with it. Promoting awareness campaigns to encourage the reporting of suspicious activities and emphasize the importance of early detection and response would also be advisable.
Fostering international collaboration and information sharing to prevent the proliferation of B. anthracis bioweapons and strengthening diplomatic efforts and engaging in multilateral agreements to ensure global cooperation in biodefense is also crucial. However, implementing these recommendations requires a coordinated effort among governments, law enforcement agencies, public health authorities, and research institutions. By prioritizing prevention and preparedness measures, we can enhance our ability to mitigate the potential bioweapon threat posed by B. anthracis.
Despite significant advances made in the detection, prevention, and treatment of infections caused by it, B. anthracis remains a global concern. Vigilance in biodefense, continuous surveillance, and research into new therapeutic strategies are imperative to mitigate future threats posed by this pathogen. Collaborative efforts across disciplines, international borders, and public health agencies will also be vital for effective preparedness and response to B. anthracis-related risks in the coming years.
Author contributions
SO: Conceptualization, Writing – original draft, Writing – review & editing. MO: Conceptualization, Writing – original draft, Writing – review & editing. KF: Conceptualization, Writing – original draft, Writing – review & editing. ATA: Writing – original draft, Writing – review & editing. OCA: Writing – review & editing. AA: Writing – review & editing. BO: Writing – review & editing. AB: Writing – review & editing. ML: Writing – review & editing. OA: Writing – review & editing. EC: Writing – review & editing.
Funding
The author(s) declare that no financial support was received for the research, authorship, and/or publication of this article.
Acknowledgments
We acknowledge the authors of this review article for their efforts toward the conceptualizing, writing, and reviewing of this work.
Conflict of interest
The authors declare that the research was conducted in the absence of any commercial or financial relationships that could be construed as a potential conflict of interest.
Publisher’s note
All claims expressed in this article are solely those of the authors and do not necessarily represent those of their affiliated organizations, or those of the publisher, the editors and the reviewers. Any product that may be evaluated in this article, or claim that may be made by its manufacturer, is not guaranteed or endorsed by the publisher.
References
1. Koch R. The aetiology of anthrax based on the ontogeny of the anthrax bacillus. Beirt. Biol Pflanz (1877) 2:277–82.
2. Cote CK, Welkos SL. Anthrax toxins in context of bacillus anthracis spores and spore germination. Toxins (2015) 7(8):3167–78. doi: 10.3390/toxins7083167
3. Pittiglio C, Shadomy S, El Idrissi A, Soumare B, Lubroth J, Makonnen Y. Seasonality and ecological suitability modelling for anthrax (Bacillus anthracis) in western Africa. Animals: an Open Access J MDPI (2022) 12(9):1146. doi: 10.3390/ani12091146
4. Zink TK. Anthrax attacks: lessons learned on the 10th anniversary of the anthrax attacks. Disaster Med Public Health Preparedness (2011) 5(3):173–4. doi: 10.1001/dmp.2011.71
5. Pohanka M. Bacillus anthracis as a biological warfare agent: infection, diagnosis and countermeasures. Bratislavske lekarske listy (2020) 121(3):175–81. doi: 10.4149/BLL_2020_026
6. Carlson CJ, Getz WM, Kausrud KL, Cizauskas CA, Blackburn JK, Bustos Carrillo FA, et al. Spores and soil from six sides: interdisciplinarity and the environmental biology of anthrax (Bacillus anthracis). Biol Rev Cambridge Philos Soc (2018) 93(4):1813–31. doi: 10.1111/brv.12420
7. Pilo P, Frey J. Pathogenicity, population genetics and dissemination of Bacillus anthracis. Infect Genet Evol (2018) 64:115–25. doi: 10.1016/j.meegid.2018.06.024
8. Blackburn JK, Goodin DG. Differentiation of springtime vegetation indices associated with summer anthrax epizootics in west Texas, USA, deer. J wildl. Dis (2013) 49(3):699–703. doi: 10.7589/2012-10-253
9. Cossaboom CM, Khaiseb S, Haufiku B, Katjiuanjo P, Kannyinga A, Mbai K, et al. Anthrax epizootic in wildlife, bwabwata national park, Namibia 2017. Emerg. Infect Dis (2019) 25(5):947–50. doi: 10.3201/eid2505.180867
10. Ezhova E, Orlov D, Suhonen E, Kaverin D, Mahura A, Gennadinik V, et al. Climatic factors influencing the anthrax outbreak of 2016 in siberia, Russia. EcoHealth (2021) 18(2):217–28. doi: 10.1007/s10393-021-01549-5
11. Obanda V, Otieno VA, Kingori EM, Ndeereh D, Lwande OW, Chiyo PI. Identifying edaphic factors and normalized difference vegetation index metrics driving wildlife mortality from anthrax in Kenya’s wildlife areas. Front Ecol Evol (2021) 9:643334. doi: 10.3389/fevo.2021.643334
12. Makino S, Uchida I, Terakado N, Sasakawa C, Yoshikawa M. Molecular characterization and protein analysis of the cap region, which is essential for encapsulation in Bacillus anthracis. J Bacteriol (1989) 171(2):722–30. doi: 10.1128/jb.171.2.722-730.1989
13. Klee SR, Ozel M, Appel B, Boesch C, Ellerbrok H, Jacob D, et al. Characterization of Bacillus anthracis-like bacteria isolated from wild great apes from Cote d’Ivoire and Cameroon. J Bacteriol (2006) 188(15):5333–44. doi: 10.1128/JB.00303-06
14. Kolstø AB, Tourasse NJ, Økstad OA. What sets Bacillus anthracis apart from other Bacillus species? Annu Rev Microbiol (2009) 63:451–76. doi: 10.1146/annurev.micro.091208.073255
15. Walsh MG, de Smalen AW, Mor SM. Climatic influence on anthrax suitability in warming northern latitudes. Sci Rep (2018) 8(1):9269. doi: 10.1038/s41598-018-27604-w
16. Hugh-Jones M, Blackburn J. The ecology of Bacillus anthracis. Mol Aspects Med (2009) 30(6):356–67. doi: 10.1016/j.mam.2009.08.003
17. Abrami L, Brandi L, Moayeri M, Brown MJ, Krantz BA, Leppla SH, et al. Hijacking multivesicular bodies enables long-term and exosome-mediated long-distance action of anthrax toxin. Cell Rep (2013) 5(4):986–96. doi: 10.1016/j.celrep.2013.10.019
18. Liu JZ, Ali SR, Bier E, Nizet V. Innate immune interactions between bacillus anthracis and host neutrophils. Front Cell Infect Microbiol (2018) 8:2. doi: 10.3389/fcimb.2018.00002
19. Pantha B, Cross A, Lenhart S, Day J. Modeling the macrophage-anthrax spore interaction: Implications for early host-pathogen interactions. Math Biosci (2018) 305:18–28. doi: 10.1016/j.mbs.2018.08.010
20. Pan Z, Dumas EK, Lawrence C, Pate L, Longobardi S, Wang X, et al. Bacillus anthracis edema toxin inhibits efferocytosis in human macrophages and alters efferocytic receptor signaling. Int J Mol Sci (2019) 20(5):1167. doi: 10.3390/ijms20051167
21. Farcasanu M, Wang AG, Uchański T, Bailey LJ, Yue J, Chen Z, et al. Rapid discovery and characterization of synthetic neutralizing antibodies against anthrax edema toxin. Biochemistry (2019) 58(27):2996–3004. doi: 10.1021/acs.biochem.9b00184
22. Meselson M, Guillemin J, Hugh-Jones M, Langmuir A, Popova I, Shelokov A, et al. The Sverdlovsk anthrax outbreak of 1979. Sci (New York N.Y.) (1994) 266(5188):1202–8. doi: 10.1126/science.7973702
23. Goel AK. Anthrax: A disease of biowarfare and public health importance. World J Clin Cases (2015) 3(1):20–33. doi: 10.12998/wjcc.v3.i1.20
24. Blackburn JK, Skrypnyk A, Bagamian KH, Nikolich MP, Bezymennyi M, Skrypnyk V. Anthrax in a backyard domestic dog in Ukraine: a case report. Vector borne zoonotic Dis (Larchmont N.Y.) (2014) 14(8):615–7. doi: 10.1089/vbz.2013.1519
25. Doganay M, Dinc G, Kutmanova A, Baillie L. Human anthrax: update of the diagnosis and treatment. Diagnostics (Basel Switzerland) (2023) 13(6):1056. doi: 10.3390/diagnostics13061056
26. Nigeria Centre for Disease Control (NCDC). (2023). Available at: www.ncdc.gov.ng/news/491/confirmation-of-anthrax-outbreak-in-nigeria (Accessed August 30 2023).
27. Flemming HC, Wingender J. The biofilm matrix. Nat Rev Microbiol (2010) 8(9):623–33. doi: 10.1038/nrmicro2415
28. Petrova OE, Sauer K. Escaping the biofilm in more than one way: Desorption, detachment or dispersion. Curr Opin Microbiol (2016) 30:67–78. doi: 10.1016/j.mib.2016.01.004
29. Costerton JW, Lewandowski Z, Caldwell DE, Korber DR, Lappin-Scott HM. Microbial biofilms. Annu Rev Microbiol (1995) 49:711–45. doi: 10.1146/annurev.mi.49.100195.003431
30. Hall-Stoodley L, Costerton JW, Stoodley P. Bacterial biofilms: from the natural environment to infectious diseases. Nat Rev Microbiol (2004) 2(2):95–108. doi: 10.1038/nrmicro821
31. Lee K, Costerton JW, Ravel J, Auerbach RK, Wagner DM, Keim P, et al. Phenotypic and functional characterization of Bacillus anthracis biofilms. Microbiology (2007) 153(6):1693–701. doi: 10.1099/mic.0.2006/003376-0
32. Auger S, Ramarao N, Faille C, Fouet A, Aymerich S, Gohar M. Biofilm formation and cell surface properties among pathogenic and nonpathogenic strains of the Bacillus cereus group. Appl Environ Microbiol (2009) 75(20):6616–8. doi: 10.1128/AEM.00155-09
33. Schuch R, Fischetti VA. The secret life of the anthrax agent Bacillus anthracis: Bacteriophage-mediated ecological adaptations. PloS One (2009) 4(8). doi: 10.1371/journal.pone.0006532
34. Schuch R, Pelzek AJ, Kan S, Fischetti VA. Prevalence of Bacillus anthracis-like organisms and bacteriophages in the intestinal tract of the earthworm Eisenia fetida. Appl Environ Microbiol (2010) 76(7):2286–94. doi: 10.1128/AEM.02518-09
35. Arora G, Sajid A, Virmani R, Singhal A, Kumar CMS, Dhasmana N, et al. Ser/Thr protein kinase PrkC-mediated regulation of GroEL is critical for biofilm formation in Bacillus anthracis. NPJ Biofilms Microbiomes (2017) 3:7. doi: 10.1038/s41522-017-0015-4
36. Driks A. The Bacillus anthracis spore. Mol Aspects Med (2009) 30(6):368–73. doi: 10.1016/j.mam.2009.08.001
37. Turner WC, Kausrud KL, Krishnappa YS, Cromsigt JP, Ganz HH, Mapaure I, et al. Fatal attraction: vegetation responses to nutrient inputs attract herbivores to infectious anthrax carcass sites. Proc Biol Sci (2014) 281(1795):20141785. doi: 10.1098/rspb.2014.1785
38. World Health Organization (WHO). Anthrax in humans and animals (2008). Available at: https://www.who.int/europe/news-room/questions-and-answers/item/anthrax (Accessed October 30 2023).
39. Ganz HH, Turner WC, Brodie EL, Kusters M, Shi Y, Sibanda H, et al. Interactions between Bacillus anthracis and plants may promote anthrax transmission. PloS Negl Trop Dis (2014) 8(6):e2903. doi: 10.1371/journal.pntd.0002903
40. Hoberg EP, Brooks DR. Evolution in action: climate change, biodiversity dynamics and emerging infectious disease. Philos Trans R Soc London. Ser B Biol Sci (2015) 370(1665):20130553. doi: 10.1098/rstb.2013.0553
41. Whitmee S, Haines A, Beyrer C, Boltz F, Capon AG, de Souza Dias BF, et al. Safeguarding human health in the Anthropocene epoch: report of The Rockefeller Foundation-Lancet Commission on planetary health. Lancet (London England) (2015) 386(10007):1973–2028. doi: 10.1016/S0140-6736(15)60901-1
42. Stendel M, Christensen JH. Impact of global warming on permafrost conditions in a coupled GCM. Geophys Res Lett (2002) 29(13):10–1-10-4. doi: 10.1029/2001GL014345
43. Elvander M, Persson B, Sternberg Lewerin S. Historical cases of anthrax in Sweden 1916-1961. Transboundary Emerging Dis (2017) 64(3):892–8. doi: 10.1111/tbed.12456
44. Cizauskas CA, Turner WC, Wagner B, Küsters M, Vance RE, Getz WM. Gastrointestinal helminths may affect host susceptibility to anthrax through seasonal immune trade-offs. BMC Ecol (2014) 14:27. doi: 10.1186/s12898-014-0027-3
45. Dragon DC, Rennie RP. The ecology of anthrax spores: tough but not invincible. Can veterinary J = La Rev veterinaire Can (1995) 36(5):295–301.
46. Okutani A, Inoue S, Morikawa S. Complete genome sequences of penicillin-resistant bacillus anthracis strain PCr, isolated from bone powder. Microbiol Resource Announce (2019) 8(35):e00670–19. doi: 10.1128/MRA.00670-19
47. Zasada AA. Detection and identification of bacillus anthracis: from conventional to molecular microbiology methods. Microorganisms (2020) 8(1):125. doi: 10.3390/microorganisms8010125
48. Klee SR, Brzuszkiewicz EB, Nattermann H, Brüggemann H, Dupke S, Wollherr A, et al. The genome of a Bacillus isolate causing anthrax in chimpanzees combines chromosomal properties of B. cereus with B. anthracis virulence plasmids. PloS One (2010) 5(7):e10986. doi: 10.1371/journal.pone.0010986
49. Roonie A, Majumder S, Kingston JJ, Parida M. Molecular characterization of B. anthracis isolates from the anthrax outbreak among cattle in Karnataka, India. BMC Microbiol (2020) 20(1):232. doi: 10.1186/s12866-020-01917-1
50. Tamborrini M, Holzer M, Seeberger PH, Schürch N, Pluschke G. Anthrax spore detection by a luminex assay based on monoclonal antibodies that recognize anthrose-containing oligosaccharides. Clin Vaccine Immunology: CVI (2010) 17(9):1446–51. doi: 10.1128/CVI.00205-10
51. Mechaly A, Vitner E, Levy H, Weiss S, Bar-David E, Gur D, et al. Simultaneous immunodetection of anthrax, plague, and tularemia from blood cultures by use of multiplexed suspension arrays. J Clin Microbiol (2018) 56(4):e01479–17. doi: 10.1128/JCM.01479-17
52. Pillai SP, Prentice KW, Ramage JG, DePalma L, Sarwar J, Parameswaran N, et al. Rapid presumptive identification of bacillus anthracis isolates using the tetracore redLine alert™ Test. Health Secur (2019) 17(4):334–43. doi: 10.1089/hs.2019.0038
53. Braun P, Nguyen MD, Walter MC, Grass G. Ultrasensitive Detection of Bacillus anthracis by Real-Time PCR Targeting a Polymorphism in Multi-Copy 16S rRNA Genes and Their Transcripts. Int J Mol Sci (2021) 22(22):12224. doi: 10.3390/ijms222212224
54. Stopa PJ. The flow cytometry of Bacillus anthracis spores revisited. Cytometry (2000) 41(4):237–44. doi: 10.1002/1097-0320(20001201)41:4<237::aid-cyto1>3.0.co;2-3
55. Kuehn A, Kovác P, Saksena R, Bannert N, Klee SR, Ranisch H, et al. Development of antibodies against anthrose tetrasaccharide for specific detection of Bacillus anthracis spores. Clin Vaccine Immunology: CVI (2009) 16(12):1728–37. doi: 10.1128/CVI.00235-09
56. Kim J, Gedi V, Lee SC, Cho JH, Moon JY, Yoon MY. Advances in anthrax detection: overview of bioprobes and biosensors. Appl Biochem Biotechnol (2015) 176(4):957–77. doi: 10.1007/s12010-015-1625-z
57. Cohen N, Zahavy E, Zichel R, Fisher M. An internal standard approach for homogeneous TR-FRET immunoassays facilitates the detection of bacteria, biomarkers, and toxins in complex matrices. Anal. bioanal. Chem (2016) 408(19):5179–88. doi: 10.1007/s00216-016-9602-0
58. Kolton CB, Marston CK, Stoddard RA, Cossaboom C, Salzer JS, Kozel TR, et al. Detection of Bacillus anthracis in animal tissues using InBios active anthrax detect rapid test lateral flow immunoassay. Lett Appl Microbiol (2019) 68(6):480–4. doi: 10.1111/lam.13134
59. Barandongo ZR, Dolfi AC, Bruce SA, Rysava K, Huang YH, Joel H, et al. The persistence of time: the lifespan of Bacillus anthracis spores in environmental reservoirs. Res Microbiol (2023) 174(6):104029. doi: 10.1016/j.resmic.2023.104029
60. Jackson PJ, Walthers EA, Kalif AS, Richmond KL, Adair DM, Hill KK, et al. Characterization of the variable-number tandem repeats in vrrA from different Bacillus anthracis isolates. Appl Environ Microbiol (1997) 63(4):1400–5. doi: 10.1128/aem.63.4.1400-1405.1997
61. Ramisse V, Patra G, Vaissaire J, Mock M. The Ba813 chromosomal DNA sequence effectively traces the whole Bacillus anthracis community. J Appl Microbiol (1999) 87(2):224–8. doi: 10.1046/j.1365-2672.1999.00874.x
62. Zasada AA, Gierczynski R, Raddadi N, Daffonchio D, Jagielski M. Some Bacillus thuringiensis strains share rpoB nucleotide polymorphisms also present in Bacillus anthracis. J Clin Microbiol (2006) 44(4):1606–7. doi: 10.1128/JCM.44.4.1606-1607.2006
63. Irenge LM, Gala JL. Rapid detection methods for Bacillus anthracis in environmental samples: a review. Appl Microbiol Biotechnol (2012) 93(4):1411–22. doi: 10.1007/s00253-011-3845-7
64. Qiao YM, Guo YC, Zhang XE, Zhou YF, Zhang ZP, Wei HP, et al. Loop-mediated isothermal amplification for rapid detection of Bacillus anthracis spores. Biotechnol Lett (2007) 29(12):1939–46. doi: 10.1007/s10529-007-9472-9
65. Kurosaki Y, Sakuma T, Fukuma A, Fujinami Y, Kawamoto K, Kamo N, et al. A simple and sensitive method for detection of Bacillus anthracis by loop-mediated isothermal amplification. J Appl Microbiol (2009) 107(6):1947–56. doi: 10.1111/j.1365-2672.2009.04379.x
66. Euler M, Wang Y, Heidenreich D, Patel P, Strohmeier O, Hakenberg S, et al. Development of a panel of recombinase polymerase amplification assays for detection of biothreat agents. J Clin Microbiol (2013) 51(4):1110–7. doi: 10.1128/JCM.02704-12
67. Elhanany E, Barak R, Fisher M, Kobiler D, Altboum Z. Detection of specific Bacillus anthracis spore biomarkers by matrix-assisted laser desorption/ionization time-of-flight mass spectrometry. Rapid Commun mass spectrom.: RCM (2001) 15(22):2110–6. doi: 10.1002/rcm.491
68. Pauker VI, Thoma BR, Grass G, Bleichert P, Hanczaruk M, Zöller L, et al. Improved Discrimination of Bacillus anthracis from Closely Related Species in the Bacillus cereus Sensu Lato Group Based on Matrix-Assisted Laser Desorption Ionization-Time of Flight Mass Spectrometry. J Clin Microbiol (2018) 56(5):e01900–17. doi: 10.1128/JCM.01900-17
69. Lee VJ. Biowarfare pathogens. Is the research flavor different than that of clinically relevant pathogens? Annu Rep med. Chem (2004) 39:211–21. doi: 10.1016/S0065-7743(04)39017-2
70. Aborode AT, Ojo-Akosile T, Uwah EA, Ottoho E, Ogunleye SC, Kamaldeen AB, et al. The outbreak of anthrax in Nigeria: Re-enforcing one health. New Microbes New Infect (2023), 101189. doi: 10.1016/j.nmni.2023.101189
71. Zhou B, Carney C, Janda KD. Selection and characterization of human antibodies neutralizing Bacillus anthracis toxin. Bioorg Med Chem (2008) 16(4):1903–13. doi: 10.1016/j.bmc.2007.11.001
72. Glinert I, Bar-David E, Sittner A, Weiss S, Schlomovitz J, Ben-Shmuel A, et al. Revisiting the concept of targeting only bacillus anthracis toxins as a treatment for anthrax. Antimicrob. Agents Chemother (2016) 60(8):4878–85. doi: 10.1128/AAC.00546-16
73. Food and Drug Administration (FDA). (2018). Available at: www.fda.gov/vaccines-blood-biologics/vaccines/anthrax (Accessed October 30, 2023).
Keywords: bacillus anthracis, anthrax, transmission, zoonoses, bioweapon anthrax
Citation: Ogunleye SC, Olorunshola MM, Fasina KA, Aborode AT, Akinsulie OC, Amoo A, Olatoye BJ, Bakare A, Lawal MA, Adekanye O and Chinyere EC (2024) Anthrax outbreak: exploring its biological agents and public health implications. Front. Trop. Dis 4:1297896. doi: 10.3389/fitd.2023.1297896
Received: 20 September 2023; Accepted: 15 November 2023;
Published: 18 January 2024.
Edited by:
ThankGod Emmanuel Onyiche, University of Maiduguri, NigeriaReviewed by:
Tsepo Ramatla, North-West University, South AfricaSammy Ohene-Aboagye, Rush University, United States
Copyright © 2024 Ogunleye, Olorunshola, Fasina, Aborode, Akinsulie, Amoo, Olatoye, Bakare, Lawal, Adekanye and Chinyere. This is an open-access article distributed under the terms of the Creative Commons Attribution License (CC BY). The use, distribution or reproduction in other forums is permitted, provided the original author(s) and the copyright owner(s) are credited and that the original publication in this journal is cited, in accordance with accepted academic practice. No use, distribution or reproduction is permitted which does not comply with these terms.
*Correspondence: Seto C. Ogunleye, c2NvNzdAbXNzdGF0ZS5lZHU=