- 1Human Health Theme, International Centre of Insect Physiology and Ecology (icipe), Nairobi, Kenya
- 2MRC-University of Glasgow Centre for Virus Research, University of Glasgow, Glasgow, United Kingdom
Long-lasting insecticidal nets (LLINs) and indoor residual spraying (IRS) have resulted in a major decrease in malaria transmission. However, it has become apparent that malaria can be effectively transmitted despite high coverage of LLINs/IRS. Residual transmission can occur due to Plasmodium-carrying Anopheles mosquitoes that are insecticide resistant and have feeding and resting behavior that reduces their chance of encountering the currently deployed indoor malaria control tools. Residual malaria transmission is likely to be the most significant hurdle to achieving the goal of malaria eradication and research and development towards new tools and strategies that can control residual malaria transmission is therefore critical. One of the most promising strategies involves biological agents that are part of the mosquito microbiome and influence the ability of Anopheles to transmit Plasmodium. These differ from biological agents previously used for vector control in that their primary effect is on vectoral capacity rather than the longevity and fitness of Anopheles (which may or may not be affected). An example of this type of biological agent is Microsporidia MB, which was identified in field collected Anopheles arabiensis and caused complete inhibition of Plasmodium falciparum transmission without effecting the longevity and fitness of the host. Microsporidia MB belongs to a unique group of rapidly adapting and evolving intracellular parasites and symbionts called microsporidia. In this review we discuss the general biology of microsporidians and the inherent characteristics that make some of them particularly suitable for malaria control. We then discuss the research priorities for developing a transmission blocking strategy for the currently leading microsporidian candidate Microsporidia MB for malaria control.
Introduction
Malaria continues to be a major health issue across sub-Saharan Africa, with this region accounting for 93% of the global malaria deaths (1). The core preventive strategies for malaria control are the use of insecticide treated nets (ITNs), indoor residual spraying (IRS) and in some situations larval source management. As a result of improved coverage of these interventions, an estimated 170 million cases and 938,000 deaths were averted in 2020, compared to the estimated burden if case incidence and mortality rate had remained at the levels of 2000 (1). By 2020, 65% of households in sub-Saharan Africa had at least one ITN, increasing from about 5% in 2000. ITN use reduces child mortality and also survival to adulthood (2–4). IRS is another proven method to control malaria transmission (5, 6). However, its use reduced globally from 5.8% in 2010 to 2.6% in 2020 (1). The effectiveness of ITNs and IRS relies on several factors including susceptibility of mosquitoes to the class of insecticide used, adequate coverage rates, quality and timely implementation, and user acceptance or compliance. Some of the factors that limit the effectiveness of ITNs and IRS can be addressed but even full implementation of these core interventions cannot entirely halt malaria parasite transmission across all settings (7). Residual malaria transmission is the actual maintained inoculation of Plasmodium, either in mosquitoes or humans, despite a well-designed and executed vector control program and is of great concern for malaria elimination (Figure 1). As the core interventions include ITNs and IRS, the term residual malaria transmission is defined as all forms of malaria transmission that persist after full universal coverage with effective ITNs and/or IRS interventions has been achieved (8). Although it may vary according to location, the main mosquito-related characteristics of residual malaria transmission include shift in resting and biting place, biting preference, biting time and species composition (8–10). Some of the vector control methods that have been proposed to eliminate residual malaria transmission include, improved implementation of larval source management, attractive toxic sugar baits, zooprophylaxis, reducing mosquito entrance into houses (e.g. screened eave tubes), personal protection (repellents) and outdoor insecticide use (treated hammocks and sheets) (8–10). However, there is a need for novel and improved strategies that target outdoor mosquitoes, do not involve insecticides, are effective against lower densities of Anopheles and are scalable and sustainable. Many biological and genetic control technologies are theoretically suitable for this and are at various stages of design either to suppress, replace or modify Plasmodium-susceptible Anopheles populations (Figure 1) (11, 12). Biological malaria mosquito control agents include a diverse range from organisms such as viruses, bacteria, fungi and microsporidia, nematodes and fish (12, 13). Earlier studies investigating the potential of biological control agents focused mainly on the ability of these organisms to kill mosquitoes. However, over the last few decades a variety of naturally occurring biological agents, mostly microbial, have been identified in mosquitoes that are not overtly pathogenic. Many of these microbes can be expected to enhance their spread through host populations by contributing to keeping their host mosquitoes alive.
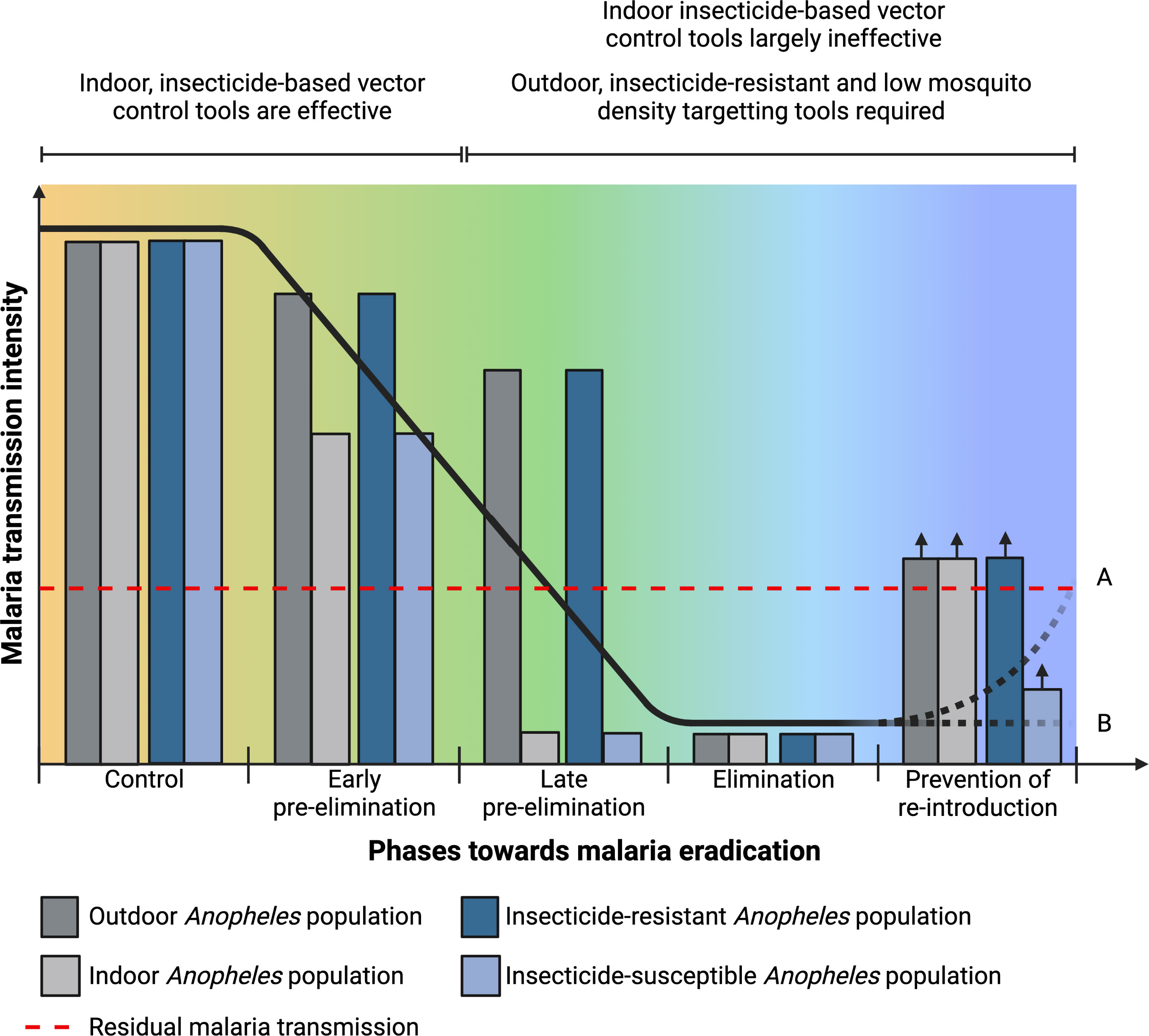
Figure 1 Residual malaria transmission and Plasmodium-susceptible Anopheles population in relation to the different epidemiological phases towards eradication and characteristics required in control tools for residual malaria transmission and during pre-elimination and elimination phases. Elimination phase may lead to (A) increase in malaria transmission intensity if only indoor insecticide-based tools are used or (B) maintained low transmission intensity if complementary tools that target outdoor and low densities of insecticide-resistant Anopheles population are used.
Anopheles mosquitoes host a diverse community of living microbes in their intestinal tract that form the intestinal microbiota. The Anopheles intestinal microbiota has been investigated for the capacity to block Plasmodium transmission. Studies generally point to Anopheles having a simple yet highly dynamic and variable bacterial microbiome (14–17). While there are clades that are consistently found, it is not evident that a ‘core’ bacterial community exists (18). Inoculation of bacterial strains isolated from Anopheles mosquitoes can result in mosquitoes becoming refractory to Plasmodium infection (19–21), however, these experiments are limited by the fact that inoculation is unlikely to recapitulate the natural infection process, since microbes inoculated from pure cultures are at concentrations significantly higher than they would be encountered under natural conditions. This artificial inoculation may elicit a response (immune or physiological) in the mosquito that is different from their effects under natural conditions (22, 23). There is, however, some recent evidence that an intestinal microbiome member of Anopheles can interfere with Plasmodium vivax transmission under more natural conditions (24). Members of the bacterial intestinal microbiota have also been genetically modified to induce Plasmodium refractoriness (24–26), which may maximize transmission blocking independent of high bacterial concentrations. In addition to the bacterial microbiota, the fungal microbiota has also been explored for transmission blocking potential, with studies predominately focusing on the yeast Wickerhamomyces (27) and Metarhizium, which has been modified to produce scorpine (an anti-microbial protein) and shown to reduced Plasmodium sporozoite intensity by 98% (28, 29). However, the application and use of transgenic microbes is challenged by low acceptance from the public, wild type strains outcompeting genetically modified strains, chances of horizontal gene transfer to other microbes and possibility of irreversible unintended outcomes (30). Therefore, despite a number Anopheles intestinal microbiome members having been associated with Plasmodium refractoriness, the potential routes towards the development of transmission blocking strategies are not very straightforward.
Specialized members of the mosquito microbiome, known as endosymbionts, have the capacity to enter the bodies and cells of their host mosquitoes. From the standpoint of transmission blocking strategies, endosymbionts are of significant interest. By virtue of their intracellular niche, these microbes can affect host biology with higher levels of precision. Generally, endosymbionts are host specific and have evolved strategies to be spread directly between hosts, often by mother to offspring transmission. By far the most comprehensively studied example is Wolbachia, an intracellular bacterial symbiont found in the majority of insect species. Wolbachia is transmitted vertically from parent to offspring and Wolbachia infection can reach a high prevalence in natural insect populations, often through the manipulation of host reproduction. One of the most common forms of Wolbachia-induced reproductive manipulation is cytoplasmic incompatibility, which occurs as a consequence of the modification of sperm in Wolbachia-infected males (31). As a result of this modification, the infected males are unable to form viable offspring when eggs of uninfected females are fertilized (32). This results in a selective advantage for Wolbachia-infected lineages and the potential for Wolbachia to spread through host populations. Natural Wolbachia infections are rare in Anopheles mosquitoes and had not been reported prior to the last decade. Trans-infection was established by artificial infection in Anopheles stephensi and Anopheles gambiae, with subsequent vertical transmission but this was linked to a high fitness cost, particularly in the presence of members of the microbiome (33, 34). Wolbachia infections have been reported in wild Anopheles gambiae populations (35–38), however, these natural infections are very low density and have been linked to contamination of samples in Anopheles gambiae (32, 39). Wolbachia infections associated with high density have recently been found in Anopheles species that are not primary vector species (40). Ongoing efforts to transfer these infections into Anopheles gambiae will be important for determining if Wolbachia can play a role in malaria transmission blocking.
Recent studies showed that a naturally occurring endosymbiont, Microsporidia MB, is found in all the primary malaria vectors in Africa (41–43). In addition, Microsporidia MB impairs the development of Plasmodium in Anopheles arabiensis. Anopheles arabiensis plays an important role in residual malaria transmission as an exophilic species that has proportionally increased in east Africa with the decrease in the more endophilic An. coluzzii and An. gambiae (42). Microsporidia MB is known to be transmitted vertically from mother to offspring and horizontally through mating, with no apparent fitness cost in the infected male or female mosquitoes (42, 43), characteristics that are associated with symbionts that can spread to high prevalence in insects (44). Studies on Microsporidia MB have led to renewed interest in microsporidia for vector control, mainly as a transmission blocking strategy suitable for the control of residual malaria transmission during the control as well as the pre-elimination phases, when mosquito populations will be low (Figure 1). Microsporidia are highly specialized organisms that have a well-developed ability to influence their host’s biology host from their niche inside the host cell. As obligate intracellular symbionts, microsporidians can have intimate and intricate interactions with their hosts at the cellular level (45), in a manner that is similar to other symbionts that occupy a cellular niche, such as Wolbachia. Also, like Wolbachia and other insect endosymbionts, Microsporidia MB is vertically transmitted (42), which can play an important role in sustaining the symbiont in the host insect population. Unlike many other endosymbiotic microbes, microsporidians form spores and often retain the capacity to be horizontally transmitted. Microsporidia MB has an efficient sexual horizontal transmission route (43), which could play an important role in its spread through populations. A high natural prevalence of Microsporidia MB has been observed in certain populations of An. arabiensis mosquitoes. This together with their ability to prevent transmission of malaria in An. arabiensis makes them promising candidates for malaria control. In this review we discuss the unique biology of microsporidia, the advantage that microsporidia have over other biological control agents and our outlook for research priorities in this field with the overall aim to highlight the potential of microsporidia as a sustainable transmission-blocking strategy for the control of residual malaria transmission.
Microsporidia: pathogens and endosymbionts
Microsporidia were first identified in 1857 by Carl Wilhelm von Nägeli as the cause of pébrine, a disease that ravaged the European silkworm industry (46, 47). Nägeli called the microsporidian Nosema bombycis (47). At that time several researchers worked on this disease, among them the most prominent was Louis Pasteur. Pasteur was able to determine that N. bombycis is transmitted vertically through egg and described methods for identifying, controlling and preventing of pébrine disease in silkworms (48). Soon after this microsporidia became well known as pathogens in many non-human hosts, particularly invertebrates and fish (47). Microsporidia can also be pathogens of humans, with the first case of human infection with microsporidia reported in 1985, and mainly associated with immune-compromised HIV-infected population (49). In invertebrates, Microsporidia are often transmitted from mother to offspring (maternal) which is associated with lower virulence and high host specificity. When maternal transmission is the dominant form of transmission, microsporidian can induce a variety of reproductive manipulations commonly associated with insect endosymbionts. For example, sex ratio distortion towards females has been reported in Dictyocoela, a microsporidian that infects Amphipod crustaceans (50). Overall, microsporidia forms a myriad of intimate associations with their hosts ranging from intracellular pathogen to endosymbiont (Box 1) (52).
Box 1. Relevant definitions and concepts
Parasitism is the association between two different organisms wherein one benefits at a high expense of the other (51)
Symbiosis is any permanent or semi-permanent interactions between two different organisms that may have no benefit or cost to either, or a low cost for one with benefit to the other (52).
Endosymbionts are symbionts that are present inside the body or cell of hosts. They can be obligate, in most cases providing nutrients otherwise not available to the host (53) or facultative, not ubiquitous but possibly still having important evolutionary consequences for the host (54).
Merogony is asexual reproduction whereby intracellular microbe replicates its own nucleus inside its host’s cell and then induces cell segmentation.
Sporogony is asexual reproduction by multiple fission of a zygote to produce spores.
Equilibrium prevalence is the maximum prevalence level a microbe can reach in nature at a given time. Prevalence depends on transmission modes (55) and environmental factors (56, 57). As environmental factors may change, this equilibrium is dynamic.
Taxonomy and phylogeny of microsporidia
The phylum microsporidia contains nearly 1300 described species distributed in 220 genera (58). This probably represents only a fraction of the real diversity of microsporidia. The position of the microsporidia lineage on the tree of life has undergone radical changes (59). When Nägeli first identified Nosema bombycis, he described it as a yeast-like fungus and included it in the Schizomycetes, which is very close to the currently accepted position of the microsporidia (46, 59). Following taxonomic work based on morphological characters, microsporidia were later relocated to a primitive amitochondrian group called Archezoa due to the perceived absence of cell organelles especially mitochondria. With the increase in molecular data, it became widely accepted that microsporidia are in fact close relatives to fungi. However, the exact phylogenetic position of microsporidia relative to fungi still remains a debate amongst taxonomists (59, 60). Currently, Microsporidia are considered as basal fungi and are included as part of the Opisthosporidia superphylum, consisting of Microsporidia, Cryptomycota and Aphelida phyla. Although they share the evolutionary origins of true fungi (Eumycota), these sister phyla separate themselves from true fungi through shared morphological features, including dual layered chitinous spore walls and electron-dense anchoring disks. Despite these conserved features, the separation of Microsporidia from the other phyla of the Opisthosporidia is defined by the presence of unique organelles, such as the polar tube, and significant reductions with essential metabolic pathways (60). Further studies on early branching microsporidia will help in establishing this (61).
There are five major clades in the phylogenetic tree of canonical microsporidia based on ssrRNA sequences. The five clades largely correspond to the hosts and habitats associated with members of the clade, with a number of exceptions. Clade I, II and V consists of microsporidia found in fresh water (Class Aquasporidia), clade III of marine microsporidia (Class Marinosporidia) and clade IV of terrestrial microsporidia (Class Terresporidia) (Figure 2) (62). Some exceptions to the association between clades and host habitats include species from marine environments being present in all clades. This classification, which is widely cited, has been criticized on the basis of ecological heterogeneity across major lineages (63) and is still debated (58, 64).
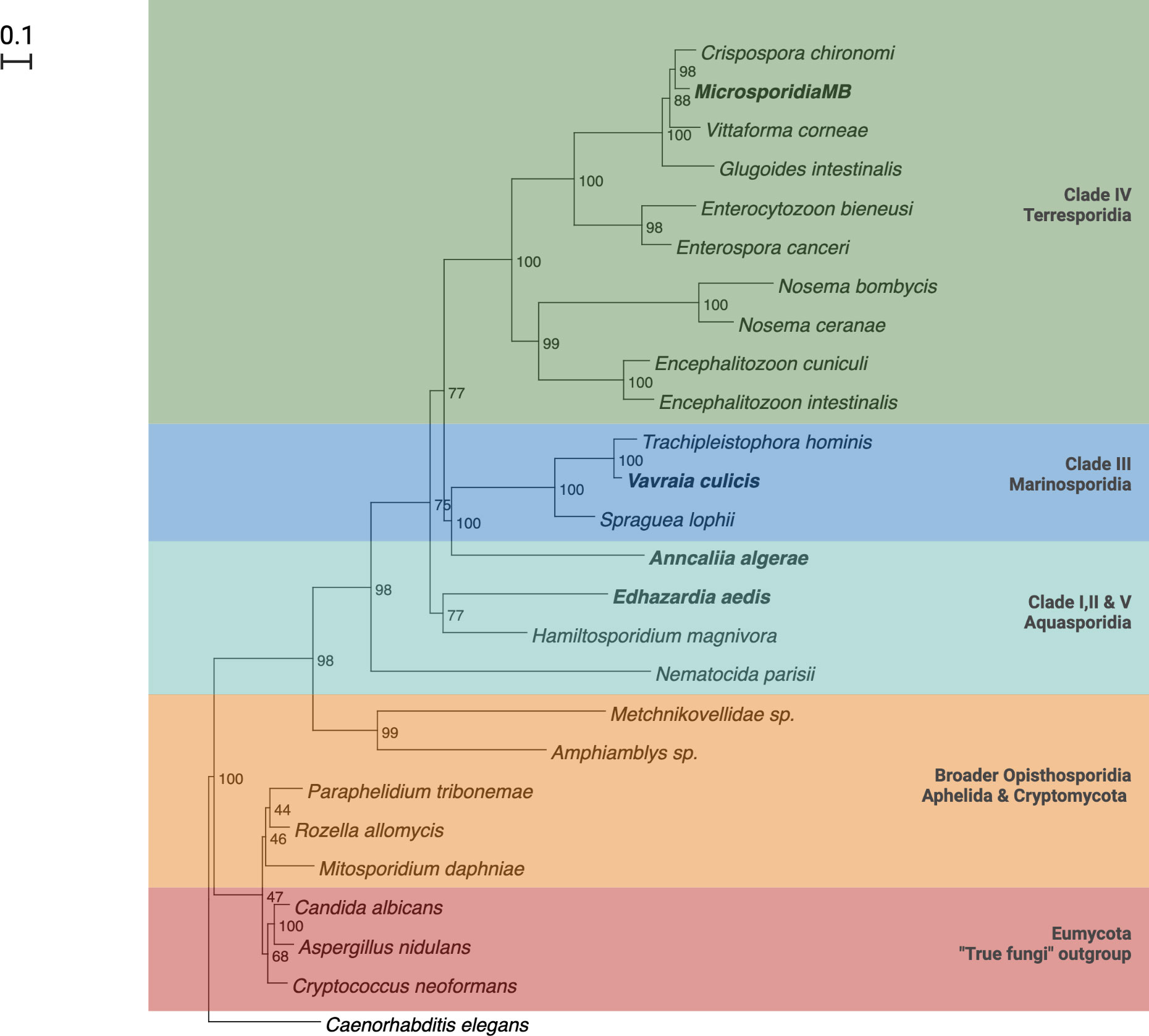
Figure 2 Phylogenetic tree of Microsporidia adapted from Herren et. al., (2020) and Vossbrinck and Vossbrinck (2005) (42, 62).
Microsporidia life cycles
Microsporidia are obligate intracellular eukaryotic parasites and can only survive outside host cells in the form of a spore. Spores are the mature, infectious stage of the life cycle and are often basis for morphological identification (Figure 3). Some species of microsporidia can produce different types of spores during their life cycle e.g. Edhazardia aedis, a parasite of Aedes aegypti produces four types of spores (65, 66). Spores sizes vary considerably from about 1 μm (human parasite Enterocytozoon bieneusi) in length to 40 μm (freshwater oligochaetes parasite Bacillidium filiferum) (67). The spore shape of most species is oval or pyriform, but rodlike, spherical, and other shapes are not unusual. The spore coat consists of two layers: an outer glycoprotein layer called exospore and inner chitinous layer called endospore. The plasma membrane lines the inside of endospore surrounding the sporoplasm. Sporoplasm consists of one or two nuclei, an anterior extrusion apparatus and a posterior vacuole. The extrusion apparatus consists of vesiculotubular polaroplast, lamellar polaroplast, the anchoring disk, manubrium and a polar tube that coils around the sporoplasm (68, 69). The polar tube is unique to microsporidia and infects the host cell during germination by injecting sporoplasm through the cell membrane and into the host cell or if a spore is phagocytosed by a host cell, polar tube pierces the phagocytic vacuole, delivering the sporoplasm into the host cell cytoplasm (68).
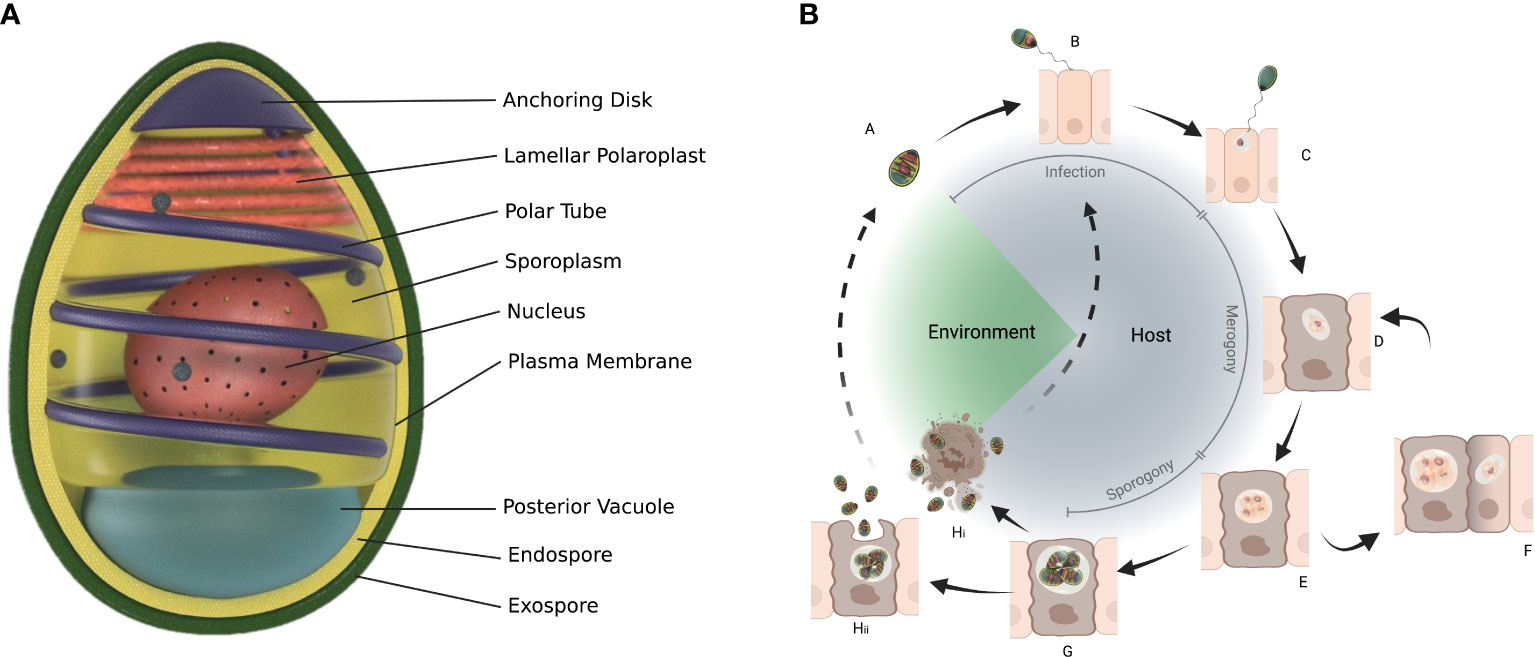
Figure 3 General structure of spore (A) and life cycle of microsporidians. The spore (A) infects a cell with its protruding polar tube (B) and injects the sporoplasm (C) into the cell. Within the cell microsporidia go through merogony (D-F) and sporogony (G) which can result in more than one type of spores (Hi, Hii). Structure of spore adapted from Wittner M, Weiss LM, ed. The Microsporidia and Microsporidiosis. Washington, DC: ASM Press; 1999 (65).
The general microsporidian life cycle starts with a spore infection followed by merogony (Box 1) and sporogony (Figure 3). Spores can be ingested, acquired through contact or mating, or in the case of vertical transmission can infect developing embryos or eggs. The spores germinate causing extrusion of the polar tube and the injection of the sporoplasm into the host cell or spores can be phagocytised by the host cell (70, 71). The sporoplasm develops into meronts in the host cell (72). This is the proliferative stage and depending on the species division can be by binary fission or multiple fission with the formation of multinucleate plasmodial forms (70). Sporogony (Box 1) starts with the thickening of the meront cell membranes to form sporonts. Sporonts after subsequent division, give rise to sporoblasts that develop into mature spores without additional multiplication (70). Production of different types of spores is indicative of adaptation by microsporidia to their host and may either be because of the different hosts through which the microsporidia completes its life cycle, or as an outcome of different life cycle paths or tissue colonization patterns occurring in the same host (73). Once sporogony is complete, the host cell ruptures, releasing mature spores into the environment and completing its life cycle. However, there are exceptions to this and exocytosis can occur with the spores coming out of the host cell covered with the host apical membrane (e.g. Nematocida parisii) or the spores simply push out (e.g. Enterospectra longa), both causing minimal damage to the host cell (74). As both merogony and sporogony result in multiplication, microsporidia can have high reproductive potential (69). In some case multiple hosts are involved in the lifecycle e.g. Amblyospora connecticus is a parasite of mosquito Ochlerotatus cantator mosquito and copepod Acanthocyclops vernalis (66, 75).
Microsporidia transmission
Microsporidia are well adapted to a diversity of transmission routes involving either or both horizontal and vertical modes (Figure 4). Horizontal transmission appears to be the most common mode of transmission of microsporidia. However, vertical transmission is probably under-reported because of the low virulence or symbiotic nature of vertically transmitted microsporidia which allows them to go undetected especially prior to the advent of molecular techniques for identifying infected hosts.
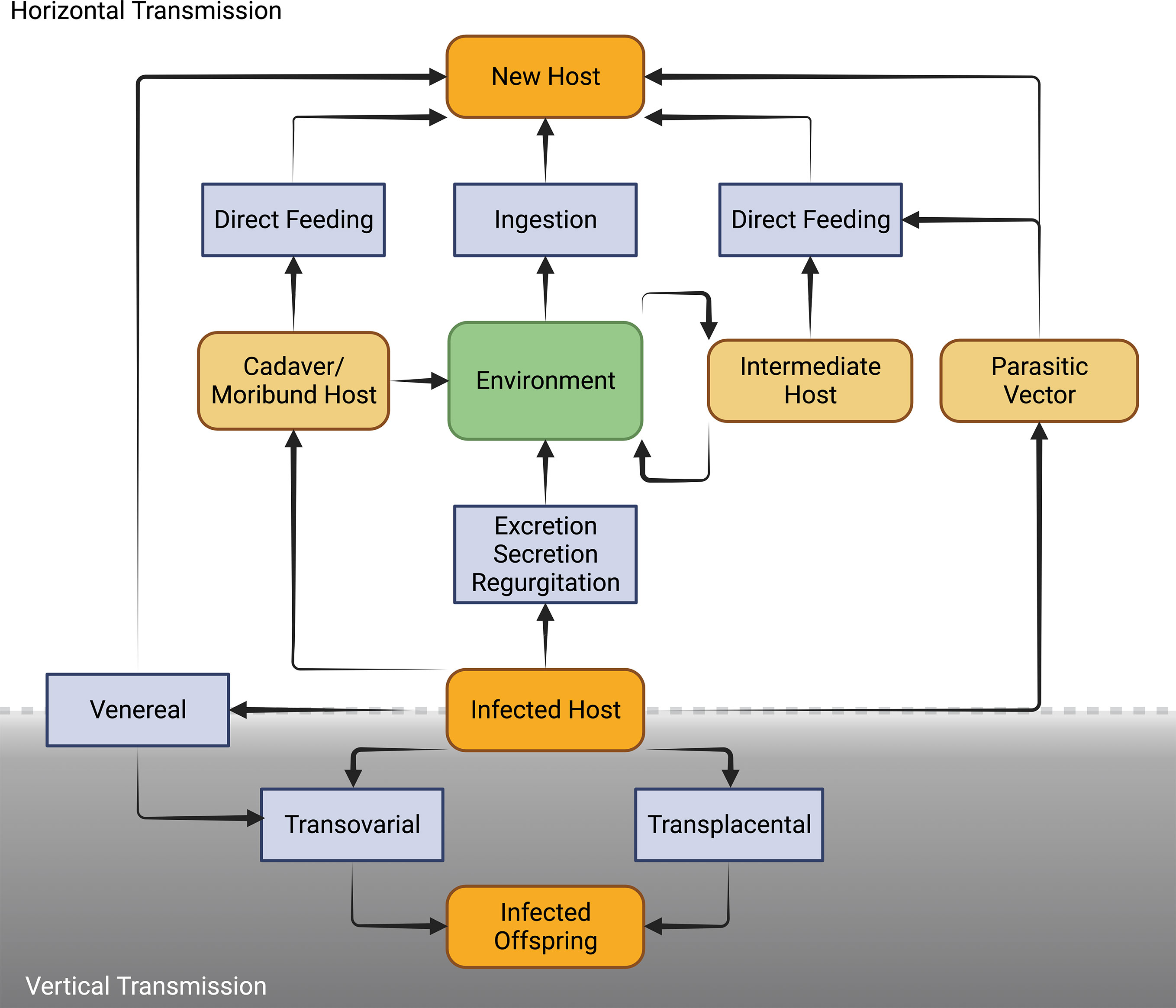
Figure 4 Schematic diagram of the diverse routes of horizontal and vertical mode of transmission in Microsporidia [Adapted from Becnel and Andreadis (76)].
Horizontal transmission is usually through the ingestion of environmental spores and sexual transmission as hosts mate but also has been shown in laboratory to occur through ovipositional activities of hymenopterous parasitoids (76). For microsporidians that rely entirely or predominately on horizontal transmission, infections are usually chronic and adversely affect host’s fecundity and longevity. Generally, microsporidia that are horizontally transmitted produce many spores and have higher virulence (Figure 5) (77). To achieve a high number of spores, in some cases there is intra-host transmission from the focal point of infection (often the intestine) to other nutrient-dense host tissues such as fat body. In some microsporidians, intra-host transmission is well-developed and characterized by production of more than one type of spore. “Early” spores have shorter polar tubes and thinner spore walls, likely to save time and material in their production. These spores infect other tissues in the same host. Another mechanism by which some species of microsporidia spread within the host is restructuring multicellular host tissues to form a syncytia (78). “Environmental” or “late” spores have thicker spore wall and longer polar tubes to survive outside the host and transmit infection to another host. While horizontal transmission via ingestion and inhalation is clearly effective, as the sole mode of transmission it requires a high population density of hosts (79).
Horizontal transmission through mating occurs when Microsporidia are transmitted between gonads of hosts of the opposite sex during copulation (80). This mode of transmission likely more efficient and allows the infected hosts with lower spore loads to still transmit to new hosts, however, it reduces the host range of the Microsporidia since sexual contact of hosts occurs only within a species, eventually leading to the evolution of host-specificity (79). For horizontal transmission through mating, the host’s mating behavior contributes substantially to the transmission rate, with transmission rate being higher in more promiscuous hosts. Overlapping host generations are required for the persistence of a strictly sexually transmitted microsporidian (44).
Vertical transmission occurs when a microsporidian infection is transferred from gonad tissue to the developing eggs. This mode of transmission limits the negative effects that the microsporidian can have on its host, since the reproductive output of the host becomes linked to its own transmission success (55). As the offspring only inherits cytoplasm from the egg, vertical transmission is predominately uniparental. For vertically transmitted microsporidians, non-transmitting male hosts are less important relative to their female counterparts as vehicles for transmission. In many endosymbionts, including Microsporidia, this has resulted in the evolution of sex-specific effects on hosts. If the microsporidian is also horizontally transmitted, it would be selected for higher rates of spore production and pathogenicity in males, in some cases killing them to release infectious spores into the environment (late male killing). In the case of strict maternal transmission, males can be selectively killed as embryos to prevent them competing with sibling females (early male killing), essentially increasing infected female host fitness at the expense of infected male fitness (81). Another reproductive manipulation caused by vertically transmitted microsporidians is feminization, whereby genetic male individuals are converted into a functional phenotypic female that is able to transmit the infection (82). Vertical transmission is largely maternal and through cytoplasm in Microsporidia, however there may be exceptions. Paternal transmission would be possible when Microsporidia are found in the semen e.g. Nosema apis and N. ceranae (83). Non-cytoplasmic infections of offspring have been shown for Encephalitozoons (transplacental) in mice (84). Formation of syncitia by Nematocida parisii to bridge between host tissues may offer another non-cytoplasmic mean for Microsporidia to infect host gametes or offspring (78). Exclusive vertical transmission is, however, considered an evolutionary end point that is irreversible because of the negative genetic effects (accumulation of mutations and gene loss) which a strict vertical transmission may have on the parasite (85). This is probably the reason for mixed patterns of horizontal and vertical transmission in microbes including Microsporidia (80).
Mixed patterns of horizontal or vertical transmission involve trade-offs between them. A higher rate of horizontal transmission e.g. a large number of spores would adversely affect the longevity and fecundity of the host (Figure 5). This in turn causes lower rate of vertical transmission. At low host densities, contact rates between host and pathogen may drop below the threshold necessary for persistence of the parasite. Persistence is more likely if the pathogen can be vertically transmitted and has low virulence allowing the host to survive till reproduction (79). Example of the consequences of these tradeoffs are found in male-killing Microsporidia such as Amblyospora sp., which kills male mosquitoes following infection through horizontal transmission of spores released after death of intermediary hosts (various copepode species) in the water. However, when spores infect female mosquitoes, the same parasite is largely avirulent and vertically transmitted to offspring (75). Ecological factors also influence the tradeoffs between horizontal and vertical transmission. In the context of a co-infection with two microsporidians Vavraia culicis and Edhazardia aedis, Aedes aegypti larval mortality increased with low food availability, thus, increasing horizontal transmission for V. culicis (86). Presence of intermediate host also influences the transmission pattern e.g., when copepods are present in breeding sites, Amblyospora sp. are mainly transmitted horizontally in Ochlerotatus cantator mosquito larvae. However, when these copepods are not available either because of not being at the stage that is susceptible to Microsporidia infection or in low numbers due to natural population oscillations, Amblyospora sp. are mainly transmitted vertically by the mosquitoes (75).
Microsporidia in mosquitoes
Microsporidia, to a large extent, are insect specialist parasites and symbionts; a 100 of the 220 described microsporidian genera have insects as their hosts. Entomogenous Microsporidia were the first to be studied in detail and form the base of our knowledge on Microsporidia biology in general (62, 76). Most Microsporidia that infect insects are found in Class Aquasporidia and Terresporidia (62). Dipterans serve as host to almost half of the entomogenous genera (76).
Microsporidia have been reported in all the medically important genera of mosquitoes i.e. Aedes, Culex and Anopheles mosquitoes (87–89). The host-parasite relationship of mosquitoes and microsporidia can be categorized into two types. The first type includes Microsporidia like Anncaliia algerae and Vavraia culicis that produce one type of spores that are transmitted horizontally, mainly by ingestion and complete their life-cycle in one mosquito filial generation. These Microsporidia are highly pathogenic and not host or tissue specific (66). These Microsporidia belong to Clade III in the phylogenetic tree and generally do not have co-evolutionary histories with their host, which renders them less mosquito-specific (Figure 2) (62, 90). The second type of Microsporidia includes Edhazardia aedis that produce multiple spore types for either horizontal or vertical transmission. They are known to complete their life cycle in two mosquitoes generations and may or may not require an intermediate host. These Microsporidia are host and tissue specific (66). They belong to Clade I in the phylogenetic tree and generally have longer co-evolutionary histories with hosts and as a result are very specific to their mosquito-host (Figure 2) (62, 66, 90).
Anncaliia algerae (syn. Nosema algerae, syn. Brachiola algerae), was reported in field Anopeheles gambiae mosquito in 1959 (88). In 1970, A. algerae was identified as a pathogen in colonized An. stephensi (91). Laboratory infection showed a reduction in fecundity and adult mortality which despite being slow (12 ± 1.7 days) was considered enough to effect malaria transmission (92, 93). Mass production of a biological control agent is a key step towards its utilization. Therefore, spore proliferation of A. algerae on a different host, corn earworm, Heliothis zea (Lepidoptera: Noctuidae), was encouraging (93). Vavraia culicis was originally isolated from field populations Culex pipiens in the Czech Republic. Studies indicated that this microsporidian had a relatively broad host range and could be transmitted by the ingestion of spores. Vavraia culicis was found to infect a range of mosquito species and have late-acting pathogenicity and, therefore, explored as a possible biopesticide (94).
One of the best studied examples of the second type of microsporidian is Edhazardia aedis, which infects Aedes aegypti. Edhazardia aedes spores can infect mosquito larvae via ingestion. After infecting the gut epithelial cells, secondary spores are released that result in the infection of additional tissue, especially the oenocytes (95). In adult females, an additional round of sporulation occurs leading to the infection of ovaries and vertical transmission. Vertically infected male larvae build up high infection loads in their fat body which ultimately leads to death and mass release of spores. Infected females generally survive and are able to continue the vertical component of the transmission cycle (96). Microsporidians with similar complex life cycles are also associated with Anopheles mosquitoes. Among them is the microsporidian species in the genera Parathelohania. Parathelohania spp. undergo vertical and horizontal transmission in several species of Anopheles mosquitoes and generally have an intermediate copepod host (97). Several of these mosquito-associated Microsporidia have been studied for their potential use as biological control agents. Biological control was largely investigated in several clade III microsporidians that are horizontally transmitted, have monomorphic spores and simple lifecycles in a single host (Figure 2) (58).
Recently, a novel microsporidian that was discovered in Anopheles arabiensis, Microsporidia MB. Microsporidia MB is in clade IV and therefore not closely related to any of the two types of mosquito associated microsporidians but have characteristics that makes it more suitable for malaria control (42). Microsporidia MB was shown to be transmitted vertically, bearing some similarities to mosquito associated microsporidians in clade I (42). However, in addition to vertical transmission, Microsporidia MB are also sexually transmitted (43). Both vertical and sexual transmission are associated with lower pathogenicity and the therefore the potential to maintain a higher equilibrium prevalence (44). Tissue specificity is also known for Microsporidia MB and correlates with the observed transmission routes, with Microsporidia MB being present in male and female gonads in An. arabiensis as well as the adult gut (42, 43).
Desirable characteristics of symbiotic Microsporidia as microbial malaria control agent
The potential role of microbes as disease transmission blockers has been widely investigated in recent years. This includes genetically modified and unmodified microbes that may be pathogenic or symbiotic. In general, highly pathogenic microbes transmit horizontally, microbes with moderate to low virulence tend towards mixed asexual-horizontal, sexual-horizontal and vertical transmission while microbes that are symbionts are transmitted with sexual-horizontal and vertical transmission (Figure 5) (79). From the standpoint of disease blocking, mixed sexual-horizontal and vertical transmission is likely to be the combination that enables feasible dissemination while maximizing equilibrium population prevalence and persistence. These transmission patterns need to be coupled with an ability to block the transmission of Plasmodium through the host mosquito.
The ability to naturally confer Anopheles resistance to Plasmodium infection gives Microsporidia MB an advantage over genetically modified microbes (68). Other advantages of a Microsporidia-based control strategy are transmission modes that favor low fitness cost, high host specificity and self-dissemination, environmental spores that are stable and can be used as inoculum and the intracellular niche that reduces competition with other microbes has renewed interest in Microsporidia.
Microsporidia, Anopheles and Plasmodium interaction
The interaction between Microsporidia and Plasmodium in mosquitoes was first reported in 1959 (88). Studies with A. algerae and another V. culicis showed that in addition to the moderate impact on Anopheles mortality and fecundity, there was also a priming effect that influenced the susceptibility of the mosquitoes to Plasmodium (89, 98, 99). This priming has been reported in An. stephensi, An. gambiae and Ae. aegypti. Adults that emerged from An. stephensi larvae exposed to A. algerae had 70% fewer oocysts of Plasmodium yoelli in the midgut after 14 days (99). Similar outcome was reported in An. gambiae exposed to V. culicis during the larval stage with a reduced likelihood (59% vs 82%) and intensity (8.9 vs 20.7 oocysts) of P. berghei (98).
Since both A. algerae and V. culicis are highly pathogenic, it is likely that Plasmodium protection is a consequence of activation of systemic immune response in the host or an over proliferation of Microsporidia potentially outcompeting Plasmodium for resources. From an applied standpoint these Microsporidia would be more useful for population control than transmission blocking. In contrast, Microsporidia MB showed a complete inhibition of Plasmodium transmission with little to no effect on the fitness of hosts, suggesting a more targeted Plasmodium protection mechanism. Possible mechanisms include priming of the mosquito immune system or changes in host metabolism that inturn influence vector capacity (42, 100). The Microsporidia MB Plasmodium transmission blocking effect occurs in the midgut prior to the formation of oocysts (42). The ability to naturally make Anopheles mosquitoes resistant to Plasmodium infection gives Microsporidia MB an advantage over genetically modified biopesticides (28).
Host and tissue specificity
Host and tissue specificity are related as both result from evolution and co-speciation with the original hosts. This specificity is typically restricted to a single host species or to a group of affiliated species (73, 101). Microsporidia are host specific to the extent that some studies consider the host an important taxonomic character (62, 76). Host specificity prevents non-target effects of an intervention and therefore a key requirement before introduction of the control agent in nature. Anncaliia algerae and V. culicis both infect multiple mosquito genera (102). Microsporidia MB to date has been identified in An. arabiensis, An. funestus, An. gambiae s.s and An. coluzzii (41–43). Microsporidia MB was not detected in Aedes, Culex, Culicoides, cyclops or Daphnia associated with habitats where Microsporidia MB infected An. arabiensis were found (43). However, a recent study investigating microbes associated with mosquitoes in south America found a close relative of Microsporidia MB in culex mosquitoes (103). Although this may indicate lack of an intermediate host and host specificity of Microsporidia MB, more studies are needed to confirm.
Tissue specificity or tropism is advantageous for parasites, in general, because it allows them to avoid the host’s immune response and increase transmission while avoiding over proliferation. Microsporidia are intracellular and in arthropods found mainly in gut, fat body and male and female reproductive tissues (104). Anncaliia sp. and Vavraia sp. infect a range of insect tissues including fat body, gut, malpighian tubules, muscle, nerve, salivary glands (102). Microsporidia MB was mainly detected in midgut and reproductive tissues (42). This contrasts with entomopathogenic fungi that grow in the hemocoel and invade almost all the tissues. Tissue specificity is, therefore, characteristic of symbionts to ensure their transmission while avoiding a high fitness and survival cost on the host (73). These characteristics also make microsporidians suitable for a sustainable control strategies (12, 94).
Microsporidia persistence and prevalence in nature
Microsporidia includes parasites and symbionts that can be vertically and/or horizontally transmitted. Each of these combination may lead to a different potential evolutionary outcome (55). Vertical transmission selects for less virulent strains of pathogens which in turn have low fitness cost for the host and in case of symbionts may also benefit the host (55, 79, 105). When a pathogen/symbiont is only transmitted vertically, they can evolve to parasitize only the male hosts or to not benefit the male host (106). However, this would not be the case if the pathogen or symbiont is also transmitted horizontally through mating. Horizontal transmission is also important for the introduction of the pathogen or symbiont into a susceptible population, however, vertical transmission becomes increasingly important as the parasite spreads in the host population and susceptible hosts are depleted (107). Therefore, theoretically a microsporidian that is symbiotic and is transmitted vertically and horizontally has the potential to reach high prevalence levels in nature under favorable environmental conditions.
Very few surveys have been carried out on natural prevalence of Anopheles microbiota and and those that do often focus on specific pathogens of interest. Service (1977) looked at the parasite and predator prevalence in pools, ponds and rice paddies after insecticide spray to highlight the non-target effects of insecticide in Kenya. This study can also be used to observe the persistence and natural buildup of parasites in Anopheles larvae after a population crash. A fungus Coelomomyces sp. was found in 15.9% and 2.1%, microsporidian (Parathelohania sp.) were found in 8.8% and 6.7% and mermithid nematodes in 0.5% and 6.9% of the larvae in the rice paddies and ponds respectively (108). This study indicates that microsporidians maintain some of the highest prevalence of mosquito pathogens in certain mosquito populations in Kenya.
Microsporidia MB prevalence has been found to be on average 6-8% in blood-fed An. arabiensis in Kenya, however seasonal fluctuations resulted in prevalence up to 23% (42). A microsporidian pathogen of grasshoppers, Paranosema locustae, is one of the few microsporidians that has been successfully commercialized to control rangeland grasshoppers (109). P. locustae is horizontally transmitted and causes a chronic low virulence infection associated with reduced feeding, development, and fecundity, increased mortality rates and a behavioral shift from gregarious form to the less damaging solitary form. P. locustae is native to North America but was introduced to Argentina in the late seventies-early eighties and mid-nineties. A study found that over 20 years after any further introductions, grasshoppers were still infected at prevalences ranging from 10-50% in sites in the Pampas and Patagonia regions of Argentina and that sites with P. locustae had not experienced grasshopper outbreaks (110). Another microsporidian that has demonstrated potential as self-sustaining biopesticide is Nosema pyrausta, which infects the European corn borer, Ostrinia nubilalis. Nosema pyrausta causes a chronic, generally nonlethal, debilitation and has efficient vertical transmission. N. pyrausta was not widely implemented due to the widespread adoption of transgenic corn expressing insecticidal proteins of Bacillus thuringiensis which resulted in effective control of the European corn borer. In addition, the lack of in vitro mass spore production methods limited the development and implementation of N. pyrausta as a biopesticide. These examples illustrating the capacity of certain microsporidians to remain at a prevalence up to 50% for more than 20 years after dissemination is indicative that they could provide more sustainability and economical control (104, 110).
Current research gap in the development of Microsporidia-based malaria control strategy
Microsporidia MB has been shown to completely impair the transmission of Plasmodium without effecting mosquito survival. This together with the ability to transmit both horizontally and vertically makes Microsporidia MB a promising candidate as a resistance-proof and self-sustainable malaria control tool (44). However, our knowledge of microsporidia biology is not up to par with other biological control agents such as entomopathogenic fungi and Wolbachia. Fortunately, the technological advancement in research over the last few decades provides an unprecedented opportunity to understand the biology, life cycle, strain diversity, genetics, transmission routes and the underlaying mechanisms that impair Plasmodium development (Figure 6). Also, there is a lot of knowledge that can be borrowed from the “laboratory to field” development of genetically modified mosquitoes and Wolbachia-infected mosquitoes as mosquito control strategies (Figure 7) (111, 112). This will contribute to the potential for ‘fast-tracking’ the development of Microsporidia MB-based control strategy for malaria transmission.
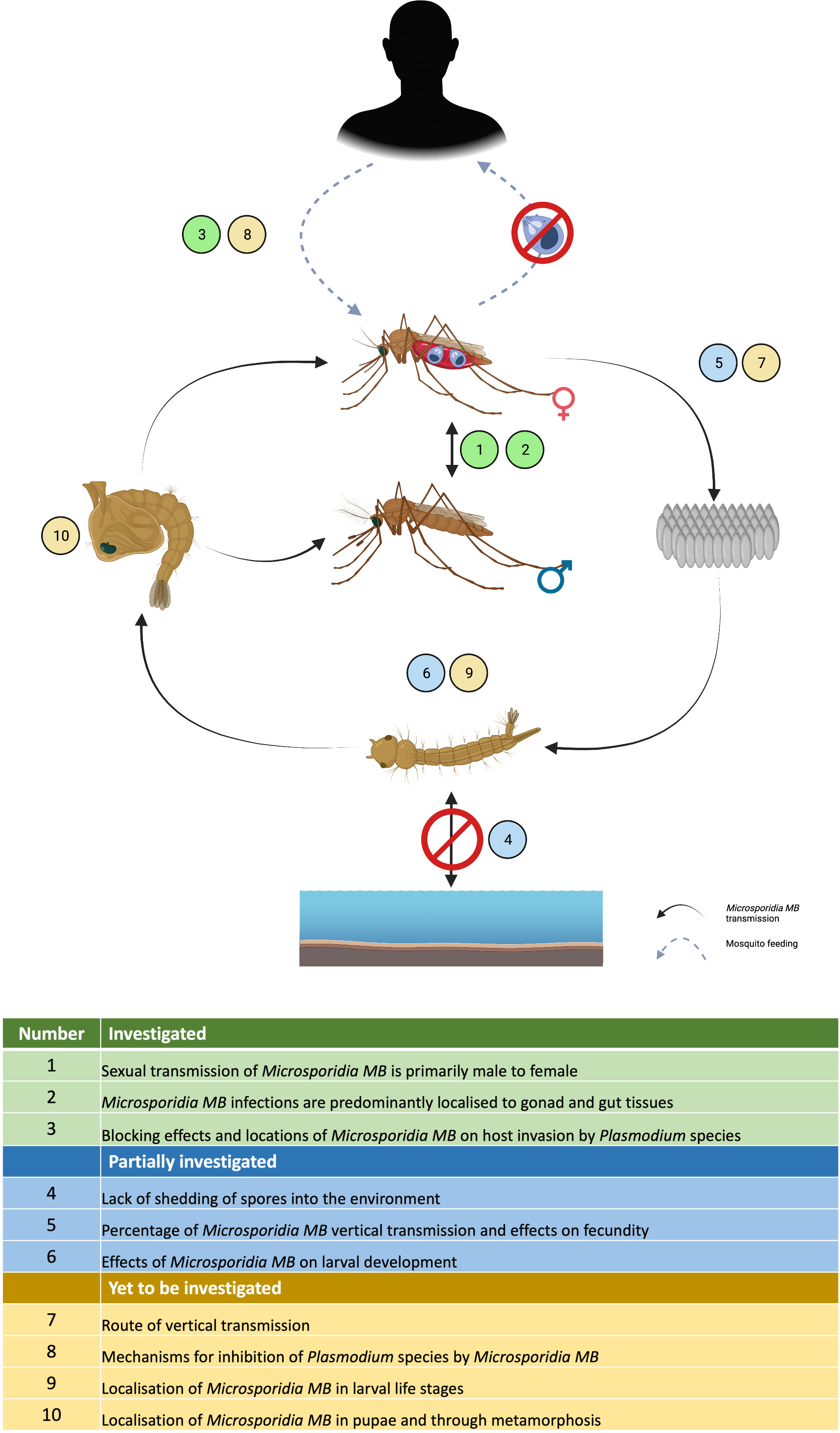
Figure 6 Current status of Microsporidia MB research highlighting the areas that have been investigated, partially investigated or yet to be investigated in the laboratory.
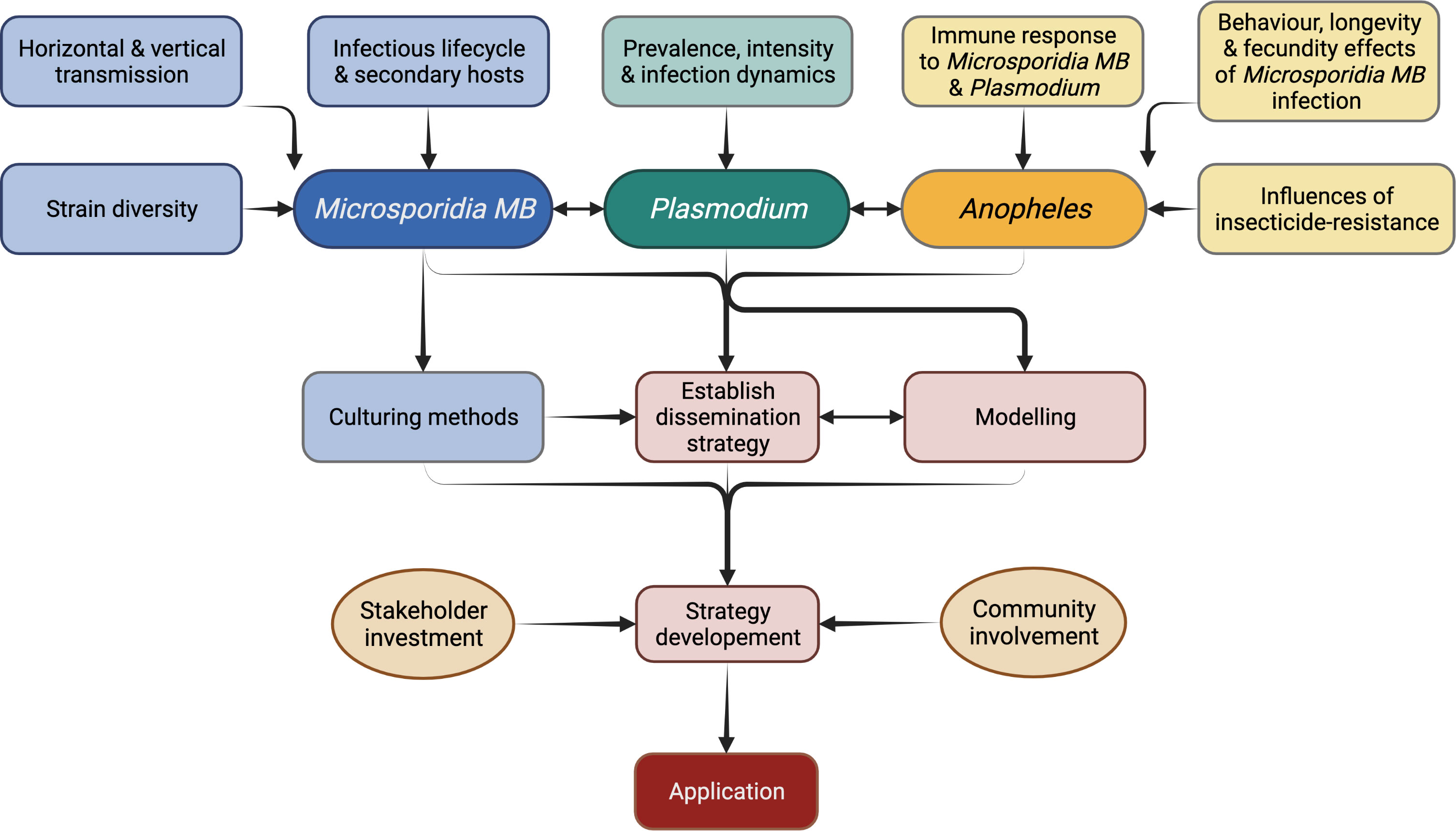
Figure 7 Research priorities for the application of Microsporidia MB-based malaria control strategy.
Better understanding of transmission and life cycle of Microsporidia MB
Vertical transmission and horizontal transmission through mating has been shown for Microsporidia MB but the exact route of transmission and whether additional transmission routes exist still needs to be determined (Figure 6). For example, Microsporidia MB transmission from mother to offspring may be transovarial (inside the egg) or transovum (on the egg surface). There is a large range in transmission efficiency, suggesting that vertical transmission may be influenced by the mother’s infection intensity and age. The finding that Microsporidia MB density is high in the mosquito intestine (43) suggests there may be more routes of horizontal transmission apart from mating (Figure 6). Intestinal infections could be linked to an environmental transmission route e.g. by ingesting spores from the water or faeces of an infected larvae (79). Understanding these routes will also improve our understanding of the life cycle of Microsporidia MB. Microscopic techniques, utilized to diagnose microsporidiosis can be adapted to understand the infection routes and dynamics of Microsporidia MB infection in Anopheles mosquitoes (Figure 6) (113).
Further investigation will be required to determine if Microsporidia MB has alternate hosts. Cyclops and Daphnia collected from the field sites that had approx. 10% Microsporidia MB prevalence in Anopheles, were found to be uninfected (43). Also, phylogenetically, Microsporidia MB belongs to clade IV that has not co-evolved with mosquitoes. Both suggest a simple life cycle without an intermediate host (42, 43, 62, 66). However, Microsporidia MB is closely related to a non-biting midge microsporidia Crispospora chironomid which is also found in clade IV (114). This indicates a certain extent of co-evolution and, therefore, the transmission and life cycle of Microsporidia MB will need to be studied further.
Spore culture, infection methods and persistence of spores
Currently, experimentation relies solely on the progeny of field collected Microsporidia MB-infected mosquitoes. This is a tedious process, involving field collection of blood-fed females, forced oviposition, screening for Microsporidia MB-infection, and rearing of the progeny. This work can be significantly reduced by propagation of spore cultures. Proliferative stages of Microsporidia spores are intracellular which makes it difficult to culture them. The spores of A. algerae were successfully produced on a non-host corn earworm, however, for Microsporidia MB the preference would be to identify in vivo culture techniques (93, 115, 116). Cell cultures have been used to propagate Wolbachia (117) and mammalian-infecting microsporidians and it should be possible to adapt these for Microsporidia MB (115, 116). This will involve research on methods for extraction of spores from the host tissue, purification of spores and storage conditions for spores before use for cell culture, host-infection or dispersal (118). Cell cultures will also allow study of life cycle and molecular studies including improved genome sequencing (116). Spores obtained from cultures will be key in carrying out standardised infections of mosquitoes for experiments. This would involve determining suitable infection methods such as oral, microinjections or mechanical inoculation of the genitalia. Spores could also be used to test the persistence under various temperature and humidity regimes and, if needed, select for strains that are more persistent in nature and the optimum storage conditions (76). Apart from experimentation, spore production is essential for Microsporidia MB-based malaria control which may involve spore dispersal into the environment to infect wild Anopheles populations or to infect and rear Microsporidia MB-infected mosquitoes for release (Figure 7).
Microsporidia MB strains and Anopheles background
Many strains of Microsporidia MB may be circulating in nature given that microsporidians are a rapidly evolving group. Strains can vary in transmission modes, virulence, and host resulting in a range of outcomes on host range, fecundity, longevity and immunity (55, 79, 94). Therefore, it is essential to identify the Microsporidia MB strains that are most suitable for malaria transmission blocking in mosquitoes with Plasmodium intensity within the natural range. A precursor to this will be the assembly of a complete/near-complete draft genome of the Microsporidia MB.
Host background may influence the prevalence and intensity of Microsporidia MB and, therefore, the transmission blocking phenotype. An. gambiae complex (An. gambiae s.s. and An. coluzzii) mosquitoes homozygous for the 2La+ allele were found to be more susceptible to Plasmodium but not to M. anisopliae (119). Gene mapping of Microsporidia-infected and uninfected Anopheles can reveal genotypes that are more susceptible to Microsporidia MB. This may lead to identification of Anopheles populations best suited to Microsporidia MB-based malaria control interventions.
The proportion of insecticide resistant Anopheles mosquitoes will increase over time due to the use of insecticide-based vector control tool. Insecticide resistance has been shown to be influenced by gut microbiota in Anopheles as well as other insects with certain bacteria protecting the mosquito (120, 121). In the context of host background, it is therefore important to also look at the prevalence of Microsporidia MB in insecticide resistant and susceptible populations. This would identify any antagonism of a Microsporidia MB-based strategy with current control strategies. Irrespective of the insecticide resistance status, mosquito microbiome interact and can effect microbial transmission and host fitness e.g. Asaia prevents Wolbachia transmission and increases blood-feeding induced mortality in Anopheles (33). These interactions are therefore important to investigate for Microsporidia MB.
Standardised semi-field tests and operational scalability
Standardised semi-field testing needs to be carried out to determine the spreading potential of Microsporidia MB in Anopheles population in close to natural conditions (122). Data obtained from these tests will provide an insight into scalability and used in theoretical models to make informed decisions while developing the Microsporidia MB- based control strategy.
Depending on the outcome of ongoing research, the control strategy may involve dispersal of environmental spores, Microsporidia MB positive male and/or female mosquitoes or both. The operational aspects of larval source management can be used as an analogy to understand the advantages and challenges of spore dispersal. Spore dispersal would be suitable for places that have few, fixed and findable breeding sites (123). It will target exophilic, insecticide-resistant and multiple species of Anopheles collectively. In general, biocontrol efforts with microsporidians need small amounts of infective spores introduced at relatively large temporal intervals which is an advantage, logistically (124). However, if this is not the case frequent dispersal of Microsporidia MB spores may be required to increase its prevalence in nature. Rainfall may affect the efficacy of the intervention by producing new and temporary breeding sites as well as flushing out the Microsporidia spores from the treated breeding sites (125, 126). Spore dispersal will therefore need to be timed accordingly. The most common and productive breeding sites are vegetated, as a result the spores will need to be either formulated or applied so that the spores enter and spread across the water surface and are not lost on the vegetation. This may require formulating the spores to ensure their uniform spread in vegetated breeding sites e.g. with surface films (127). Focal point spore infection, by attracting ovipositing Anopheles to breeding site with semiochemicals, may eliminate the need to treat majority of the breeding sites. Targeting adults, for example using attractants and spores added to sugar baits, is also a possible dissemination approach.
Release of Microsporidia MB positive males or both sexes involves mass production of Anopheles mosquitoes. Much work has been done to determine the conditions suitable for the production of large number of mosquitoes that are not inferior to the natural population in terms of fitness or longevity (128–130). However, it will be essential to test this with Microsporidia MB-infected mosquitoes following the established guidelines for mass rearing of Anopheles mosquitoes (131). This strategy unlike the spore dispersal will be species-specific and in locations where two or more malaria vectors exist will require the mass production and release of all the vector species. Release of only males may be more acceptable in this regard given they are non-biting, but efficiency of vertical transmission in the field following mating transfer from males to females must be determined as this will be a critical factor (43). However, male only or male-biased releases involve a step for sex separation for which there is currently no established method (132). Sexing pupae based on size is not suitable for An. arabiensis and development of a genetic sexing strain will add a layer of regulatory requirements to an otherwise natural control strategy (133). Addition of toxicants e.g. ivermectin or malathion to blood meals to selectively kill the females would probably be the most suitable method to develop selecting Microsporidia MB positive males for release, although this will preclude the release of newly eclosed males (132).
Community sensitization and stakeholder involvement is important for any intervention but especially for release of Anopheles mosquitoes (Figure 7). In 1975, irradiated males were planned for release to suppress populations of Culex and Ae. aegypti in India, but the media and negative public opinion resulted in failure of the program (133, 134). Over the past few years successful releases of Wolbachia-infected mosquitoes have been carried out with careful community consultation and there is wide acceptance for these programs (135, 136). However, creating awareness on the released mosquitoes being unable to transmit malaria, and acceptance from the community and stakeholders remains essential for each release (133, 137).
The Microsporidia MB release programs would initially involve community engagements, mass rearing (and/or spore production in case of Micropsoridia MB based strategy) and release of mosquitoes (Figure 7). Post-release surveillance will be required to monitor the prevalence rates of Microsporidia MB positive Anopheles mosquitoes and inform decision-making on whether further top-up releases are required (133). Cost estimates for use of Micropsoridia MB in urban and rural settings with either spore dispersal or mosquito release, as was carried out for Wolbachia Ae. aegypti releases, will inform the development of the control strategy (138).
Conclusion
Identification of the high transmission blocking potential of Microsporidia MB has resulted in a renewed interest in microsporidians for malaria control, now as a transmission blocking strategy rather than for population suppression. Microsporidians are a unique clade of highly specialized parasites and symbionts which have evolved a variety of strategies to ensure transmission; as a result they have inherent characteristics, comparable to Wolbachia, that make them attractive for developing malaria control approaches. Microsporidia MB has considerable potential to be a sustainable strategy for control of residual malaria, as well as pre-elimination phase of malaria control provided that the major research gaps are addressed.
Author contributions
TB conceived the concept, carried out the literature review and drafted the article. RP contributed to the accuracy of the review and figures. JH provided guidance in the concept and writing of the article. All authors contributed to the article and approved the submitted version.
Funding
The authors gratefully acknowledge the financial support for this research by the following organizations and agencies Open Philanthropy (SYMBIOVECTOR Track A) and the Bill and Melinda Gates Foundation (INV0225840). The International Centre of Insect Physiology and Ecology (icipe) receives support from: the Swedish International Development Cooperation Agency (Sida); the Swiss Agency for Development and Cooperation (SDC); the Federal Democratic Republic of Ethiopia; and the Government of the Republic of Kenya. The views expressed herein do not necessarily reflect the official opinion of the donors.
Acknowledgments
We are grateful to Steve Sinkins for providing overall guidance on the operational aspects in this review. All figures were created with BioRender.com. We acknowledge the support of the SymbioVector team at icipe-Human health theme, Kenya.
Conflict of interest
The authors declare that the research was conducted in the absence of any commercial or financial relationships that could be construed as a potential conflict of interest.
Publisher’s note
All claims expressed in this article are solely those of the authors and do not necessarily represent those of their affiliated organizations, or those of the publisher, the editors and the reviewers. Any product that may be evaluated in this article, or claim that may be made by its manufacturer, is not guaranteed or endorsed by the publisher.
References
2. Fink G, Mrema S, Abdulla S, Kachur SP, Khatib R, Lengeler C, et al. Mosquito net use in early childhood and survival to adulthood in Tanzania. N Engl J Med (2022) 386:428–36. doi: 10.1056/nejmoa2112524
3. Pryce J, Richardson M, Lengeler C. Insecticide-treated nets for preventing malaria. Cochrane Database Syst Rev (2018) 11(11):CD000363. doi: 10.1002/14651858.CD000363.pub3
4. Nevill CG, Some ES, Mung’ala VO, Mutemi W, New L, Marsh K, et al. Insecticide-treated bednets reduce mortality and severe morbidity from malaria among children on the Kenyan coast. Trop Med Int Heal (1996) 1:139–46. doi: 10.1111/j.1365-3156.1996.tb00019.x
5. WHO. Indoor residual spraying: Use of indoor residual spraying for scaling up global malaria control and elimination: WHO position statement. Geneva: World Health Organization. (2006).
6. Wagman JM, Varela K, Zulliger R, Saifodine A, Muthoni R, Magesa S, et al. Reduced exposure to malaria vectors following indoor residual spraying of pirimiphos-methyl in a high-burden district of rural Mozambique with high ownership of long-lasting insecticidal nets: entomological surveillance results from a cluster-randomized trial. Malar J (2021) 20:1–17. doi: 10.1186/s12936-021-03583-8
7. WHO. Control of residual malaria parasite transmission: guidance note Geneva: World Health Organization. (2014) p. 1–5.
8. Killeen GF. Characterizing, controlling and eliminating residual malaria transmission. Malar J (2014) 13:1–22. doi: 10.1186/1475-2875-13-330
9. Okumu F, Finda M. Key characteristics of residual malaria transmission in two districts in south-eastern Tanzania - implications for improved control. J Infect Dis (2021) 223:S143–54. doi: 10.1093/infdis/jiaa653
10. Carnevale P, Manguin S. Review of issues on residual malaria transmission. J Infect Dis (2021) 223:S61–80. doi: 10.1093/infdis/jiab084
11. Wang GH, Gamez S, Raban RR, Marshall JM, Alphey L, Li M, et al. Combating mosquito-borne diseases using genetic control technologies. Nat Commun (2021) 12:1–12. doi: 10.1038/s41467-021-24654-z
12. Kamareddine L. The biological control of the malaria vector. Toxins (Basel) (2012) 4:748–67. doi: 10.3390/toxins4090748
13. Bukhari T, Takken W, Koenraadt CJM. Biological tools for control of larval stages of malaria vectors - a review. Biocontrol Sci Technol (2013) 23:987–1023. doi: 10.1080/09583157.2013.810706
14. Ngo CT, Romano-Bertrand S, Manguin S, Jumas-Bilak E. Diversity of the bacterial microbiota of anopheles mosquitoes from binh phuoc province, Vietnam. Front Microbiol (2016) 7:2095. doi: 10.3389/fmicb.2016.02095
15. Akorli J, Gendrin M, Pels NAP, Yeboah-Manu D, Christophides GK, Wilson MD. Seasonality and locality affect the diversity of anopheles gambiae and anopheles coluzzii midgut microbiota from Ghana. PLoS One (2016) 11:e0157529. doi: 10.1371/journal.pone.0157529
16. Bascuñán P, Niño-Garcia JP, Galeano-Castañeda Y, Serre D, Correa MM. Factors shaping the gut bacterial community assembly in two main Colombian malaria vectors. Microbiome (2018) 6:148. doi: 10.1186/s40168-018-0528-y
17. Hegde S, Khanipov K, Albayrak L, Golovko G, Pimenova M, Saldaña MA, et al. Microbiome interaction networks and community structure from laboratory-reared and field-collected aedes aegypti, aedes albopictus, and culex quinquefasciatus mosquito vectors. Front Microbiol (2018) 9:2160. doi: 10.3389/fmicb.2018.02160
18. Gendrin M, Christophides GK. “The anopheles mosquito microbiota and their impact on pathogen transmission”. In: Manguin S, editor. Anopheles mosquitoes - new insights into malaria vectors. London: InTech (2013). doi: 10.5772/3392
19. Pumpuni CB, Beier MS, Nataro JP, Guers LD, Davis JR. Plasmodium falciparum: Inhibition of sporogonic development in anopheles stephensi by gram-negative bacteria. Exp Parasitol (1993) 77:195–9. doi: 10.1006/expr.1993.1076
20. Gonzalez-Ceron L, Santillan F, Rodriguez MH, Mendez D, Hernandez-Avila JE. Bacteria in midguts of field-collected anopheles albimanus block plasmodium vivax sporogonic development. J Med Entomol (2003) 40:371–4. doi: 10.1603/0022-2585-40.3.371
21. Dong Y, Manfredini F, Dimopoulos G. Implication of the mosquito midgut microbiota in the defense against malaria parasites. PLoS Pathog (2009) 5:1–10. doi: 10.1371/journal.ppat.1000423
22. Akhouayri IG, Habtewold T, Christophides GK. Melanotic pathology and vertical transmission of the gut commensal elizabethkingia meningoseptica in the major malaria vector anopheles gambiae. PLoS One (2013) 8:1–12. doi: 10.1371/journal.pone.0077619
23. Bahia AC, Dong Y, Blumberg BJ, Mlambo G, Tripathi A, Ben Marzouk-Hidalgo OJ, et al. Exploring anopheles gut bacteria for plasmodium blocking activity. Environ Microbiol (2014) 16:2980–94. doi: 10.1111/1462-2920.12381
24. Gao H, Bai L, Jiang Y, Huang W, Wang L, Li S, et al. A natural symbiotic bacterium drives mosquito refractoriness to plasmodium infection via secretion of an antimalarial lipase. Nat Microbiol (2021) 6:806–17. doi: 10.1038/s41564-021-00899-8
25. Gao H, Cui C, Wang L, Jacobs-Lorena M, Wang S. Mosquito microbiota and implications for disease control. Trends Parasitol (2020) 36:98–111. doi: 10.1016/J.PT.2019.12.001
26. Capone A, Ricci I, Damiani C, Mosca M, Rossi P, Scuppa P, et al. Interactions between asaia, plasmodium and anopheles: New insights into mosquito symbiosis and implications in malaria symbiotic control. Parasites Vectors (2013) 6:182. doi: 10.1186/1756-3305-6-182
27. Favia G, Ricci I, Damiani C, Raddadi N, Crotti E, Marzorati M, et al. Bacteria of the genus asaia stably associate with anopheles stephensi, an Asian malarial mosquito vector. Proc Natl Acad Sci U.S.A. (2007) 104:9047–51. doi: 10.1073/pnas.0610451104
28. Fang W, Vega-Rodríguez J, Ghosh AK, Jacobs-Lorena M, Kang A, Leger RJ St. Development of a transgenic fungi that kill human malaria parasite in mosquitoes. Science (2011) 331:1074–7. doi: 10.1126/science.1199115
29. Heinig RL, Thomas MB. Interactions between a fungal entomopathogen and malaria parasites within a mosquito vector. Malar J (2015) 14:1–10. doi: 10.1186/s12936-014-0526-x
30. Karalis DT, Karalis T, Karalis S, Kleisiari AS. Genetically modified products, perspectives and challenges. Cureus (2020) 12:e7306. doi: 10.7759/cureus.7306
31. Werren JH, Baldo L, Clark ME. Wolbachia: Master manipulators of invertebrate biology. Nat Rev Microbiol (2008) 6:741–51. doi: 10.1038/nrmicro1969
32. Gomes FM, Barillas-Mury C. Infection of anopheline mosquitoes with wolbachia: Implications for malaria control. PLoS Pathog (2018) 14:e1007333. doi: 10.1371/journal.ppat.1007333
33. Hughes GL, Dodson BL, Johnson RM, Murdock CC, Tsujimoto H, Suzuki Y, et al. Native microbiome impedes vertical transmission of wolbachia in anopheles mosquitoes. Proc Natl Acad Sci U.S.A. (2014) 111:12498–503. doi: 10.1073/pnas.1408888111
34. Bian G, Joshi D, Dong Y, Lu P, Zhou G, Pan X, et al. Wolbachia invades anopheles stephensi populations and induces refractoriness to plasmodium infection. Science (2013) 340:748–51. doi: 10.1126/science.1236192
35. Walker T, Quek S, Jeffries CL, Bandibabone J, Dhokiya V, Bamou R, et al. Stable high-density and maternally inherited wolbachia infections in anopheles moucheti and anopheles demeilloni mosquitoes. Curr Biol (2021) 31:2310–2320.e5. doi: 10.1016/j.cub.2021.03.056
36. Shaw WR, Marcenac P, Childs LM, Buckee CO, Baldini F, Sawadogo SP, et al. Wolbachia infections in natural anopheles populations affect egg laying and negatively correlate with plasmodium development. Nat Commun (2016) 7:1–7. doi: 10.1038/ncomms11772
37. Jeffries CL, Lawrence GG, Golovko G, Kristan M, Orsborne J, Spence K, et al. Novel wolbachia strains in anopheles malaria vectors from sub-Saharan Africa. Wellcome Open Res (2018) 3:338434. doi: 10.12688/wellcomeopenres.14765.2
38. Baldini F, Segata N, Pompon J, Marcenac P, Robert Shaw W, Dabiré RK, et al. Evidence of natural wolbachia infections in field populations of anopheles gambiae. Nat Commun (2014) 5:1–7. doi: 10.1038/ncomms4985
39. Chrostek E, Gerth M. Is anopheles gambiae a natural host of wolbachia? MBio (2019) 10:1–10. doi: 10.1128/mBio.00784-19
40. Walker T, Quek S, Jeffries CL, Bandibabone J, Dhokiya V, Bamou R, et al. Genomic and microscopic evidence of stable high density and maternally inherited wolbachia infections in anopheles mosquitoes. bioRxiv (2020). doi: 10.1101/2020.10.29.357400
41. Akorli J, Akorli EA, Tetteh SNA, Amlalo GK, Opoku M, Pwalia R, et al. Microsporidia MB is found predominantly associated with anopheles gambiae s.s and anopheles coluzzii in Ghana. Sci Rep (2021) 11:1–5. doi: 10.1038/s41598-021-98268-2
42. Herren JK, Mbaisi L, Mararo E, Oundo JW, Makhulu EE, Butungi H, et al. A microsporidian blocks plasmodium falciparum transmission in anopheles arabiensis mosquitoes. Nat Commun (2020) 11:799445. doi: 10.1101/799445
43. Nattoh G, Maina T, Makhulu EE, Mbaisi L, Mararo E, Otieno FG, et al. Horizontal transmission of the symbiont microsporidia MB in anopheles arabiensis. Front Microbiol (2021) 12:647183. doi: 10.3389/fmicb.2021.647183
44. Knell RJ, Webberley KM. Sexually transmitted diseases of insects: distribution, evolution, ecology and host behaviour. Biol Rev Camb Philos Soc (2004) 79:557–81. doi: 10.1017/s1464793103006365
45. Weidner E, Overstreet R. Microsporidia have a peculiar outer membrane with exterior cytoplasmic proteins. Acta Parasitol (2022) 67:49–54. doi: 10.1007/s11686-021-00446-9
46. Nageli C. Uber die neue krankheit der seidenraupe und verwandte organismen. Bot Zeit (1857) 15:760.
47. Franzen C. Microsporidia : A review of 150 years of research. Parasitology (2008) 2:1–34. doi: doi 10.2174/1874421400802010001
49. Desportes I, Charpentier Y, Galian A, Bernard F, Cochand-Priollet B, Lavergne A, et al. Occurrence of a new microsporidan: Enterocytozoon bieneusi n. g., n. sp., in the enterocytes of a human patient with AIDS. J Protozool (1985) 32:250–4. doi: 10.1111/J.1550-7408.1985.TB03046.X
50. Quiles A, Wattier RA, Bacela-Spychalska K, Grabowski M, Rigaud T. Dictyocoela microsporidia diversity and co-diversification with their host, a gammarid species complex (Crustacea, amphipoda) with an old history of divergence and high endemic diversity. BMC Evol Biol (2020) 20:149. doi: 10.1186/s12862-020-01719-z
51. Olano JP, Weller PF, Guerrant RL, Walker DH. “Principles of parasitism: Host-parasite interactions.,”. In: Tropical infectious diseases: Principles, pathogens and practice. Elsevier (2011). p. 1–7. doi: 10.1016/B978-0-7020-3935-5.00001-X
53. Douglas AE. Nutritional interactions in insect-microbial symbioses: aphids and their symbiotic bacteria buchnera. Annu Rev Entomol (1998) 43:17–37. doi: 10.1146/annurev.ento.43.1.17
54. Heyworth ER, Ferrari J. A facultative endosymbiont in aphids can provide diverse ecological benefits. J Evol Biol (2015) 28:1753–60. doi: 10.1111/jeb.12705
55. Lipsitch M, Siller S, Novak MA. The evolution of virulence in pathogens with vertical and horizontal transmission. Int J Org Evol (1996) 50:1729–41. doi: 10.1525/california/9780520280540.003.0006
56. Hien NT, Anh DD, Le NH, Yen NT, Phong TV, Nam VS, et al. Environmental factors influence the local establishment of wolbachia in aedes aegypti mosquitoes in two small communities in central Vietnam. Gates Open Res (2021) 5:147. doi: 10.12688/gatesopenres.13347.1
57. Alonso AC, Stein M, Matías Hisgen C, Micieli MV. Abiotic factors affecting the prevalence of wolbachia (Rickettsiaceae) in immature aedes albopictus (Skuse) (Culicidae). J Invertebr Pathol (2022) 189:107730. doi: 10.1016/J.JIP.2022.107730
58. Park E, Poulin R. Revisiting the phylogeny of microsporidia. Int J Parasitol (2021) 51:855–64. doi: 10.1016/j.ijpara.2021.02.005
59. Corradi N, Keeling PJ. Microsporidia: A journey through radical taxonomical revisions. Fungal Biol Rev (2009) 23:1–8. doi: 10.1016/j.fbr.2009.05.001
60. Bass D, Czech L, Williams BAP, Berney C, Dunthorn M, Mahé F, et al. Clarifying the relationships between microsporidia and cryptomycota. J Eukaryot Microbiol (2018) 65:773–82. doi: 10.1111/jeu.12519
61. Galindo LJ, Torruella G, Moreira D, Timpano H, Paskerova G, Smirnov A, et al. Evolutionary genomics of metchnikovella incurvata (Metchnikovellidae): An early branching microsporidium. Genome Biol Evol (2018) 10:2736–48. doi: 10.1093/gbe/evy205
62. Vossbrinck CR, Debrunner-Vossbrinck BA. Molecular phylogeny of the microsporidia: ecological, ultrastructural and taxonomic considerations. Folia Parasitol (Praha) (2005) 52:131–42. doi: 10.14411/fp.2005.017
63. Williams BAP, Hamilton KM, Jones MD, Bass D. Group-specific environmental sequencing reveals high levels of ecological heterogeneity across the microsporidian radiation. Environ Microbiol Rep (2018) 10:328–36. doi: 10.1111/1758-2229.12642
64. Tokarev YS, Huang WF, Solter LF, Malysh JM, Becnel JJ, Vossbrinck CR. A formal redefinition of the genera nosema and vairimorpha (Microsporidia: Nosematidae) and reassignment of species based on molecular phylogenetics. J Invertebr Pathol (2020) 169:107279. doi: 10.1016/J.JIP.2019.107279
65. Vávra J, Larsson JIR. The microsporidia and microsporidiosis. Wittner M, Weiss LM eds. Weiss The microsporidia and microsporidiosis (1999), 7–84 p. doi: 10.1128/9781555818227.ch2
66. Becnel JJ, White SE, Shapiro AM. Review of microsporidia-mosquito relationships: From the simple to the complex. Folia Parasitol (Praha) (2005) 52:41–50. doi: 10.14411/fp.2005.006
67. Vávra J, Ronny Larsson JI. “Structure of microsporidia.,” microsporidia: Pathogens of opportunity. Chichester, UK: John Wiley & Sons, Inc (2014) p. 1–70. doi: 10.1002/9781118395264.ch1
68. Didier ES, Weiss LM. Overview of microsporidia and microsporidiosis. Protistology (2008) 5:243–55.
69. Weiss LM, Schwartz DA. Microsporidiosis. Guerrant RL, Walker DH, Weller PF eds. Tropical infectious diseases: Principles, pathogens and practice (Third Edition) Elsevier (2011). 714–21 p. doi: 10.1016/B978-0-7020-3935-5.00102-6
70. Cali A, Takvorian PM. “Developmental morphology and life cycles of the microsporidia.,”. In: Wittner M, Weiss LM, editors. The microsporidia and microsporidiosis. Washington, DC, USA: ASM Press (2014). p. 85–128. doi: 10.1128/9781555818227.ch3
71. Franzen C. Microsporidia: How can they invade other cells? Trends Parasitol (2004) 20:275–9. doi: 10.1016/j.pt.2004.04.009
72. Han B, Takvorian PM, Weiss LM. Invasion of host cells by microsporidia. Front Microbiol (2020) 11:172. doi: 10.3389/fmicb.2020.00172
73. Vávra J, Lukeš J. “Microsporidia and ‘The art of living together,.’”. In: Rollinson D, editor. Advances in parasitology. Academic Press (2013). p. 253–319. doi: 10.1016/B978-0-12-407706-5.00004-6
74. Tamim El Jarkass H, Reinke AW. The ins and outs of host-microsporidia interactions during invasion, proliferation and exit. Cell Microbiol (2020) 22:e13247. doi: 10.1111/cmi.13247
75. Andreadis TG. Evolutionary strategies and adaptations for survival between mosquito-parasitic microsporidia and their intermediate copepod hosts: a comparative examination of amblyospora connecticus and hyalinocysta chapmani (Microsporidia: Amblyosporidae. Folia Parasitol (Praha) (2005) 52:23–35. doi: 10.14411/fp.2005.004
76. Becnel JJ, Andreadis TG. “Microsporidia in insects.,” the microsporidia and microsporidiosis. Washington, DC, USA: ASM Press (2014) p. 447–501. doi: 10.1128/9781555818227.ch14
77. Gandon S. Evolution of multihost parasites. Evolution (2004) 58:455–69. doi: 10.1111/j.0014-3820.2004.tb01669.x
78. Balla KM, Luallen RJ, Bakowski MA, Troemel ER. Cell-to-cell spread of microsporidia causes caenorhabditis elegans organs to form syncytia. Nat Microbiol (2016) 1:139–48. doi: 10.1038/nmicrobiol.2016.144.Cell-to-cell
79. Antonovics J, Wilson AJ, Forbes MR, Hauffe HC, Kallio ER, Leggett HC, et al. The evolution of transmission mode. Philos Trans R Soc Lond B Biol Sci (2017) 372:7–11. doi: 10.1098/rstb.2016.0083
80. Agnew P, Becnel JJ, Ebert D, Michalakis Y. “Symbiosis of microsporidia and insects.,”. In: Bourtzis K, Miller TA, editors. Insect symbiosis. CRC Press (2003). p. 145–63. doi: 10.1201/9780203009918
81. Werren JH, Neill SLO. “The evolution of heritable symbionts.,”. In: O’Neill SL, Hoffmann AA, Werren JH, editors. Influential passengers:inherited microorganisms and arthropod reproduction. Oxford University Press (1997). p. 1–41. Available at: http://www.ncbi.nlm.nih.gov/16386933.
82. Cormier A, Chebbi MA, Giraud I, Wattier R, Teixeira M, Gilbert C, et al. Comparative genomics of strictly vertically transmitted, feminizing microsporidia endosymbionts of amphipod crustaceans. Genome Biol Evol (2021) 13:1–13. doi: 10.1093/gbe/evaa245
83. Roberts KE, Evison SEF, Baer B, Hughes WOH. The cost of promiscuity: sexual transmission of nosema microsporidian parasites in polyandrous honey bees. Sci Rep (2015) 5:10982. doi: 10.1038/srep10982
84. Kotková M, Sak B, Hlásková L, Květoňová D, Kváč M. Evidence of transplacental transmission of encephalitozoon cuniculi genotype II in murine model. Exp Parasitol (2018) 193:51–7. doi: 10.1016/j.exppara.2018.09.001
85. Sachs JL, Skophammer RG, Regus JU. Evolutionary transitions in bacterial symbiosis. Proc Natl Acad Sci USA (2011) 108 Suppl:10800–7. doi: 10.1073/pnas.1100304108
86. Duncan AB, Agnew P, Noel V, Michalakis Y. The consequences of co-infections for parasite transmission in the mosquito aedes aegypti. J Anim Ecol (2015) 84:498–508. doi: 10.1111/1365-2656.12302
87. Gajanana A, Tewari SC, Reuben R, Rajagopalan PK. Partial suppression of malaria parasites in aedes aegypti and anopheles stephensi doubly infected with nosema algerae and plasmodium. Indian J Med Res (1979) 70:417–23.
88. Fox RM, Weiser J. A microsporidian parasite of anopheles gambiae in Liberia. J Parasitol (1959) 45:21–30. doi: 10.2307/3274782
89. Hulls R. The adverse effects of a microsporidan on sporogony and infectivity of plasmodium berghei. Trans R Soc Trop Med Hyg (1971) 65:421–2. doi: 10.1016/0035-9203(71)90120-9
90. Bjørnson S, Oi D. “Microsporidia biological control agents and pathogens of beneficial insects.,”. In: Weiss LM, Becnel JJ, editors. Microsporidia. Chichester, UK: John Wiley & Sons, Inc (2014). p. 635–70. doi: 10.1002/9781118395264.ch25
91. Vavra J, Undeen AH. Nosema algerae n. sp. (Cnidospora, microsporida) a pathogen in a laboratory colony of anopheles stephensi liston (Diptera, culicidae). J Protozool (1970) 17:240–9. doi: 10.1111/j.1550-7408.1970.tb02365.x
92. Haq N, Reisen WK, Aslamkhan M. The effects of nosema algerae on the horizontal life table attributes of anopheles stephensi under laboratory conditions. J Invertebr Pathol (1981) 37:236–42. doi: 10.1016/0022-2011(81)90081-1
93. Undeen AH, Alger NE. The effect of the microsporidan, nosema algerae, on anopheles stephensi. J Invertebr Pathol (1975) 25:19–24. doi: 10.1016/0022-2011(75)90281-5
94. Lorenz LM, Koella JC. The microsporidian parasite vavraia culicis as a potential late life-acting control agent of malaria. Evol Appl (2011) 4:783–90. doi: 10.1111/j.1752-4571.2011.00199.x
95. Andreadis TG. Host range tests with edhazardia aedis (Microsporida: Culicosporidae) against northern nearctic mosquitoes. J Invertebr Pathol (1994) 64:46–51. doi: 10.1006/jipa.1994.1067
96. Becnel JJ, Garcia JJ, Johnson MA. Edhazardia aedis (Microspora: Culicosporidae) effects on the reproductive capacity of aedes aegypti (Diptera: Culicidae). J Med Entomol (1995) 32:549–53. doi: 10.1093/jmedent/32.4.549
97. Omrani SM, Moosavi SF, Farrokhi E. Parathelohania iranica sp. nov. (Microsporidia: Amblyosporidae) infecting malaria mosquito anopheles superpictus (Diptera: Culicidae): Ultrastructure and molecular characterization. J Invertebr Pathol (2017) 146:1–6. doi: 10.1016/j.jip.2017.03.011
98. Bargielowski I, Koella JC. A possible mechanism for the suppression of plasmodium berghei development in the mosquito anopheles gambiae by the microsporidian vavraia culicis. PLoS One (2009) 4:1–5. doi: 10.1371/journal.pone.0004676
99. Schenker W, Maier WA, Seitz HM. The effects of nosema algerae on the development of plasmodium yoelii nigeriensis in anopheles stephensi. Parasitol Res (1992) 78:56–9. doi: 10.1007/BF00936182
100. Belda Cuesta E, Coulibaly B, Bukhari T, Eiglmeier K, Kone R, Coulibaly MB, et al. Comprehensive ecological and geographic characterization of eukaryotic and prokaryotic microbiomes in African anopheles. Front Microbiol (2021) 12:10. doi: 10.3389/fmicb.2021.635772
101. Agnew P, Bedhomme S, Haussy C, Michalakis Y. Age and size at maturity of the mosquito culex pipiens infected by the microsporidian parasite vavraia culicis. Proc R Soc B Biol Sci (1999) 266:947–52. doi: 10.1098/rspb.1999.0728
102. Andreadis TG. Microsporidian parasites of mosquitoes. J Am Mosq Control Assoc (2007) 23:3–29. doi: 10.2987/8756-971x(2007)23[3:mpom]2.0.co;2
103. Vivero-Gomez RJ, Castañeda-Monsalve VA, Atencia MC, Hoyos-Lopez R, Hurst GD, Cadavid-Restrepo G, et al. Molecular phylogeny of heritable symbionts and microbiota diversity analysis in phlebotominae sand flies and culex nigripalpus from Colombia. PLoS Negl Trop Dis (2021) 15:e0009942. doi: 10.1371/journal.pntd.0009942
104. Perlmutter JI, Bordenstein SR. Microorganisms in the reproductive tissues of arthropods. Nat Rev Microbiol (2020) 18:97–111. doi: 10.1038/s41579-019-0309-z
105. Lively CM, Clay K, Wade MJ, Fuqua C. Competitive co-existence of vertically and horizontally transmitted parasites. Evol Ecol Res (2005) 7:1183–90.
106. Hurst GDD, Frost CL. Reproductive parasitism: maternally inherited symbionts in a biparental world. Cold Spring Harb Perspect Biol (2015) 7:1–20. doi: 10.1101/cshperspect.a017699
107. Lipsitch M, Nowak MA, Ebert D, May RM. The population dynamics of vertically and horizontally transmitted parasites. Proc R Soc B Biol Sci (1995) 260:321–7. doi: 10.1098/rspb.1995.0099
108. Service MW. Mortalities of the immature stages of species b of the anopheles gambiae complex in Kenya: Comparison between rice fields and temporary pools, identification of predators, and effects of insecticidal spraying. J Med Entomol (1977) 13:535–45. doi: 10.1093/jmedent/13.4-5.535
109. Dakhel WH, Jaronski ST, Schell S. Control of pest grasshoppers in north America. Insects (2020) 11:1–18. doi: 10.3390/insects11090566
110. Lange CE, Mariottini Y, Plischuk S, Cigliano MM. Naturalized, newly-associated microsporidium continues causing epizootics and expanding its host range. Protistology (2020) 14:32–7. doi: 10.21685/1680-0826-2020-14-1-4
111. Nguyen TH, Nguyen H, Nguyen TY, Vu SN, Tran ND, Le TN, et al. Field evaluation of the establishment potential of wmelpop wolbachia in Australia and Vietnam for dengue control. Parasites Vectors (2015) 8:1–14. doi: 10.1186/s13071-015-1174-x
112. Schairer CE, Najera J, James AA, Akbari OS, Bloss CS. Oxitec and MosquitoMate in the united states: lessons for the future of gene drive mosquito control. Pathog Glob Health (2021) 115:365–76. doi: 10.1080/20477724.2021.1919378
113. Garcia LS. Laboratory identification of the microsporidia. J Clin Microbiol (2002) 40:1892–901. doi: 10.1128/JCM.40.6.1892-1901.2002
114. Tokarev YS, Voronin VN, Seliverstova EV, Pavlova OA, Issi IV. Life cycle, ultrastructure, and molecular phylogeny of crispospora chironomi g.n. sp.n. (Microsporidia: Terresporidia), a parasite of chironomus plumosus l. (Diptera: Chironomidae). Parasitol Res (2010) 107:1381–9. doi: 10.1007/s00436-010-2012-x
115. Visvesvara GS. In vitro cultivation of microsporidia of clinical importance. Clin Microbiol Rev (2002) 15:401–13. doi: 10.1128/CMR.15.3.401-413.2002
116. Lallo MA, Vidoto Da Costa LF, Alvares-Saraiva AM, Rocha PRD, Spadacci-Morena DD, Konno FT de C, et al. Culture and propagation of microsporidia of veterinary interest. J Vet Med Sci (2016) 78:171–6. doi: 10.1292/jvms.15-0401
117. Fallon AM. Growth and maintenance of wolbachia in insect cell lines. Insects (2021) 12:706. doi: 10.3390/insects12080706
118. Solter LF, Becnel JJ, Vávra J. “Research methods for entomopathogenic microsporidia and other protists”. In: Manual of techniques in invertebrate pathology. Elsevier Ltd (2012). p. 329–71. doi: 10.1016/B978-0-12-386899-2.00011-7
119. Riehle MM, Bukhari T, Gneme A, Guelbeogo WM, Coulibaly B, Fofana A, et al. The anopheles gambiae 2La chromosome inversion is associated with susceptibility to plasmodium falciparum in Africa. Elife (2017) 6:1–24. doi: 10.7554/eLife.25813
120. Barnard K, Jeanrenaud ACSN, Brooke BD, Oliver SV. The contribution of gut bacteria to insecticide resistance and the life histories of the major malaria vector anopheles arabiensis (Diptera: Culicidae). Sci Rep (2019) 9:9117. doi: 10.1038/s41598-019-45499-z
121. Xia X, Sun B, Gurr GM, Vasseur L, Xue M, You M. Gut microbiota mediate insecticide resistance in the diamondback moth, plutella xylostella (L.). Front Microbiol (2018) 9:25. doi: 10.3389/fmicb.2018.00025
122. Hammond A, Pollegioni P, Persampieri T, North A, Minuz R, Trusso A, et al. Gene-drive suppression of mosquito populations in large cages as a bridge between lab and field. Nat Commun (2021) 12:1–9. doi: 10.1038/s41467-021-24790-6
123. WHO Larval source management: a supplementary malaria vector control measure: an operational manual. Geneva: World Health Organization (2013).
124. Jaronski ST. “Mass production of entomopathogenic fungi: State of the art.,”. In: Juan A, Morales-Ramos M, Guadalupe R, editors. Mass production of beneficial organisms: Invertebrates and entomopathogens. Academic Press (2014). p. 357–413. DIS-I, Organisms MP of B, 2014, 357-413 P, 9780123914538 I. doi: 10.1016/B978-0-12-391453-8.00011-X
125. Harbison JE, Layden JE, Xamplas C, Zazra D, Henry M, Ruiz MOH. Observed loss and ineffectiveness of mosquito larvicides applied to catch basins in the northern suburbs of Chicago IL, 2014. Environ Health Insights (2015) 9:1–5. doi: 10.4137/EHI.S24311
126. Fillinger U, Kannady K, William G, Vanek MJ, Dongus S, Nyika D, et al. A tool box for operational mosquito larval control: preliminary results and early lessons from the urban malaria control programme in dar es salaam, Tanzania. Malar J (2008) 7:20. doi: 10.1186/1475-2875-7-20
127. Bukhari T, Takken W, Githeko AK, Koenraadt CJM. Efficacy of aquatain, a monomolecular film, for the control of malaria vectors in rice paddies. PLoS One (2011) 6:1–13. doi: 10.1371/journal.pone.0021713
128. Mamai W, Lobb LN, Bimbilé Somda NS, Maiga H, Yamada H, Lees RS, et al. Optimization of mass-rearing methods for anopheles arabiensis larval stages: Effects of rearing water temperature and larval density on mosquito life-history traits. J Econ Entomol (2018) 111:2383–90. doi: 10.1093/jee/toy213
129. Carvalho DO, Nimmo D, Naish N, McKemey AR, Gray P, Wilke ABB, et al. Mass production of genetically modified aedes aegypti for field releases in Brazil. J Vis Exp (2014) 183:e3579. doi: 10.3791/3579
130. Soma DD, Maïga H, Mamai W, Bimbile-Somda NS, Venter N, Ali AB, et al. Does mosquito mass-rearing produce an inferior mosquito? Malar J (2017) 16:357. doi: 10.1186/s12936-017-2012-8
131. IAEA. Guidelines for standardised mass rearing of anopheles mosquitoes. Vienna: : Joint FAO/IAEA Division of Nuclear Techniques in Food and Agriculture (2017).
132. Mashatola T, Ndo C, Koekemoer LL, Dandalo LC, Wood OR, Malakoane L, et al. A review on the progress of sex-separation techniques for sterile insect technique applications against anopheles arabiensis. Parasites Vectors (2018) 11:137–71. doi: 10.1186/s13071-018-3219-4
133. Ritchie SA, Staunton KM. Reflections from an old Queenslander : can rear and release strategies be the next great era of vector control? Proc R Soc B Biol Sci (2019) 286:1–8. doi: 10.1098/rspb.2019.0973
135. Pinto SB, Riback TIS, Sylvestre G, Costa G, Peixoto J, Dias FBS, et al. Effectiveness of wolbachia-infected mosquito deployments in reducing the incidence of dengue and other aedes-borne diseases in niterói, Brazil: A quasi-experimental study. PLoS Negl Trop Dis (2021) 15:e0009556. doi: 10.1371/journal.pntd.0009556
136. Nazni WA, Hoffmann AA, NoorAfizah A, Cheong YL, Mancini MV, Golding N, et al. Establishment of wolbachia strain wAlbB in Malaysian populations of aedes aegypti for dengue control. Curr Biol (2019) 29:4241–4248.e5. doi: 10.1016/j.cub.2019.11.007
137. Ernst KC, Haenchen S, Dickinson K, Doyle MS, Walker K, Monaghan AJ, et al. Awareness and support of release of genetically modified “sterile” mosquitoes, key West, Florida, USA. Emerg Infect Dis (2015) 21:320–4. doi: 10.3201/eid2102.141035
Keywords: Microsporidia MB, malaria, transmission-blocking, microbiome, residual
Citation: Bukhari T, Pevsner R and Herren JK (2022) Microsporidia: a promising vector control tool for residual malaria transmission. Front. Trop. Dis 3:957109. doi: 10.3389/fitd.2022.957109
Received: 30 May 2022; Accepted: 05 July 2022;
Published: 28 July 2022.
Edited by:
Souleymane Doucoure, Institut de recherche pour le développement, SenegalReviewed by:
Jewelna Akorli, Noguchi Memorial Institute for Medical Research, GhanaFelipe Arley Costa Pessoa, Instituto Leônidas & Maria Deane (ILMD/Fiocruz Amazônia), Brazil
Copyright © 2022 Bukhari, Pevsner and Herren. This is an open-access article distributed under the terms of the Creative Commons Attribution License (CC BY). The use, distribution or reproduction in other forums is permitted, provided the original author(s) and the copyright owner(s) are credited and that the original publication in this journal is cited, in accordance with accepted academic practice. No use, distribution or reproduction is permitted which does not comply with these terms.
*Correspondence: Tullu Bukhari, dGJ1a2hhcmlAaWNpcGUub3Jn