- 1Medical Biology/Public Health, Institut de Recherche en Sciences de la Santé (IRSS), Bobo-Dioulasso, Burkina Faso
- 2Institut des Sciences et Techniques (INSTech), Bobo Dioulasso, Burkina Faso
- 3Medical Biology/Public Health, Laboratoire de Recherche et d’Enseignement en Santé et Biotechnologie Animale (LARESBA), Université Nazi Boni, Bobo-Dioulasso, Burkina Faso
- 4Institut Pasteur de la Guyane, Microbiota of Insect Vectors Group, Cayenne, French Guiana
- 5Institut Pasteur, Université Paris Cité, Parasites and Insect Vectors Department, Paris, France
Insect-borne diseases are responsible for important burdens on health worldwide particularly in Africa. Malaria alone causes close to half a million deaths every year, mostly in developing, tropical and subtropical countries, with 94% of the global deaths in 2019 occurring in the WHO African region. With several decades, vector control measures have been fundamental to fight against malaria. Considering the spread of resistance to insecticides in mosquitoes and to drugs in parasites, the need for novel strategies to inhibit the transmission of the disease is pressing. In recent years, several studies have focused on the interaction of malaria parasites, bacteria and their insect vectors. Their findings suggested that the microbiota of mosquitoes could be used to block Plasmodium transmission. A strategy, termed paratransgenesis, aims to interfere with the development of malaria parasites within their vectors through genetically-modified microbes, which produce antimalarial effectors inside the insect host. Here we review the progress of the paratransgenesis approach. We provide a historical perspective and then focus on the choice of microbial strains and on genetic engineering strategies. We finally describe the different steps from laboratory design to field implementation to fight against malaria.
Introduction
Insect-Borne Diseases (IBD) are responsible for significant human morbidity and mortality in the world. Most of the pathogens are transmitted by arthropods vectors through blood transmission (1). Some of these pathogens, which cause devastating infectious diseases such as malaria, dengue, filariasis, yellow fever, as well as zoonotic arboviruses, such as West Nile Virus (WNV), Saint Louis Encephalitis Virus (SLEV) and Eastern Equine Encephalitis Virus (EEE), are transmitted by mosquitoes (2). After the scaling up of vector control interventions using insecticides, efforts are now limited by the development of defense mechanisms by which insects can resist to toxic substances (3). We are also witnessing a spread of some vector-borne diseases due to the geographic expansion of mosquitoes which can adapt to different environments (4). Among these diseases, malaria remains a severe burden in developing countries causing 602,000 deaths in 2020 (5). The fight against this mosquito-borne disease using chemicals generated problems of resistance in parasites and insects, so the development of additional control measures is imperative to reduce the transmission. One of the approaches under intense study to fight against IBD, referred to as paratransgenesis, relies on the genetic modification of a microbiota strain to express and deliver anti-pathogen effector molecules to reduce vectorial transmission. In this approach, genetically-engineered microbiota strains would be used to stop the development of causative agent of the disease and spread in natural mosquito populations to interfere with pathogen transmission (6). However, current challenges remain before any implementation of this strategy. This review provides an overview on historical and current work on paratransgenesis strategy to limit the burden of vector-borne diseases, with a special focus on malaria transmission. After providing a historical perspective, we focus on different requirements to consider when developing a paratransgenic approach, dealing with choice of an appropriate microbe and with the design of the paratransgenesis strategy. Finally, we discuss about the required steps on the way from laboratory to implementation.
Historical Perspective
Paratransgenesis was investigated for the first time in the gut of the kissing bug Rhodnius prolixus, an insect belonging to the Reduviidae family and Triatomidae sub-family that transmits Chagas disease (7). The strategy consisted in manipulating the symbiotic bacterium Rhodococcus rhodnii to express the antimicrobial peptide Cecropin A in order to stop the development of Trypanasoma cruzi in the vector without any detected fitness cost for the host (8). The transmission and the stability of the transformed symbiont without antibiotic selection were also shown (9). These findings stimulated interest in the role of gut symbionts to reduce vector competence by genetic manipulation. The potential of paratransgenesis was subsequently tested in tsetse flies, vectors of sleeping sickness, to reduce the transmission of African animal trypanosomiasis. The common symbiotic bacterium Sodalis, colonizing the midgut, hemolymph and salivary glands of different species of these vectors (Glossina morsitans, Glossina p. palpalis, Glossina austeni, Glossina brevipalpis) was genetically modified to produce green fluorescent protein (GFP) and then inoculated in female flies. This resulted in the spread of the fluorescent bacterium in a cage population by vertical and trans-stadial transmission (10, 11). Subsequent studies identified antimicrobial peptide genes (attacin, cecropin, diptericin) in tsetse flies (12–14) which can efficiently reduce vector competence, i.e. permissiveness of the vector to the transmitted parasite. Focusing on Anopheles mosquitoes, vectors of malaria, Bisi and Lampe engineered Escherichia coli and Pantoea agglomerans to express and secrete three anti-Plasmodium effectors using plasmids carrying secretion signals for the target molecules (15). Going further, Wang et al. engineered P. agglomerans to produce molecules that decreased by up to 98% the development of Plasmodium falciparum and Plasmodium berghei in the mosquito gut (16). To this aim, they used two types of strategies, the production of exogenous antimicrobial peptides which kill or suppress parasites and of peptides that block key interactions between the malaria parasites and mosquitoes after binding to parasites or to epithelia. Since colonization of P. agglomerans in the mosquito may be a limiting factor, they subsequently focused on Serratia AS1, which efficiently colonizes mosquitoes and can be engineered to strongly inhibit P. falciparum (17). Current research focuses on methods to improve paratransgenesis efficiency, including a better control of transgene expression and ethical suitability of paratransgenic candidates (18, 19).
Requirements for Efficient Paratransgenesis
Choosing the Right Microbe
An Efficient Colonizer in Field Mosquitoes
Several basic requirements for an effective implementation of paratransgenesis are described in Figure 1. The microbe chosen to deliver anti-parasite molecules should compete with other microbial species to survive in the mosquito midgut and in the environment. Since the first studies of the microbiota in the mosquito midgut over 50 years ago (20), research on mosquito microbiota composition has undergone dramatic development (21). A substantial bacterial growth is observed in the adult mosquito midgut after a blood meal (22), but bacterial diversity is also reduced at this stage (23). Moreover, the efficiency of transmission from mother to offspring, which varies extensively between microbes, needs to be assessed as it will have much consequences on the way a strain can be used.
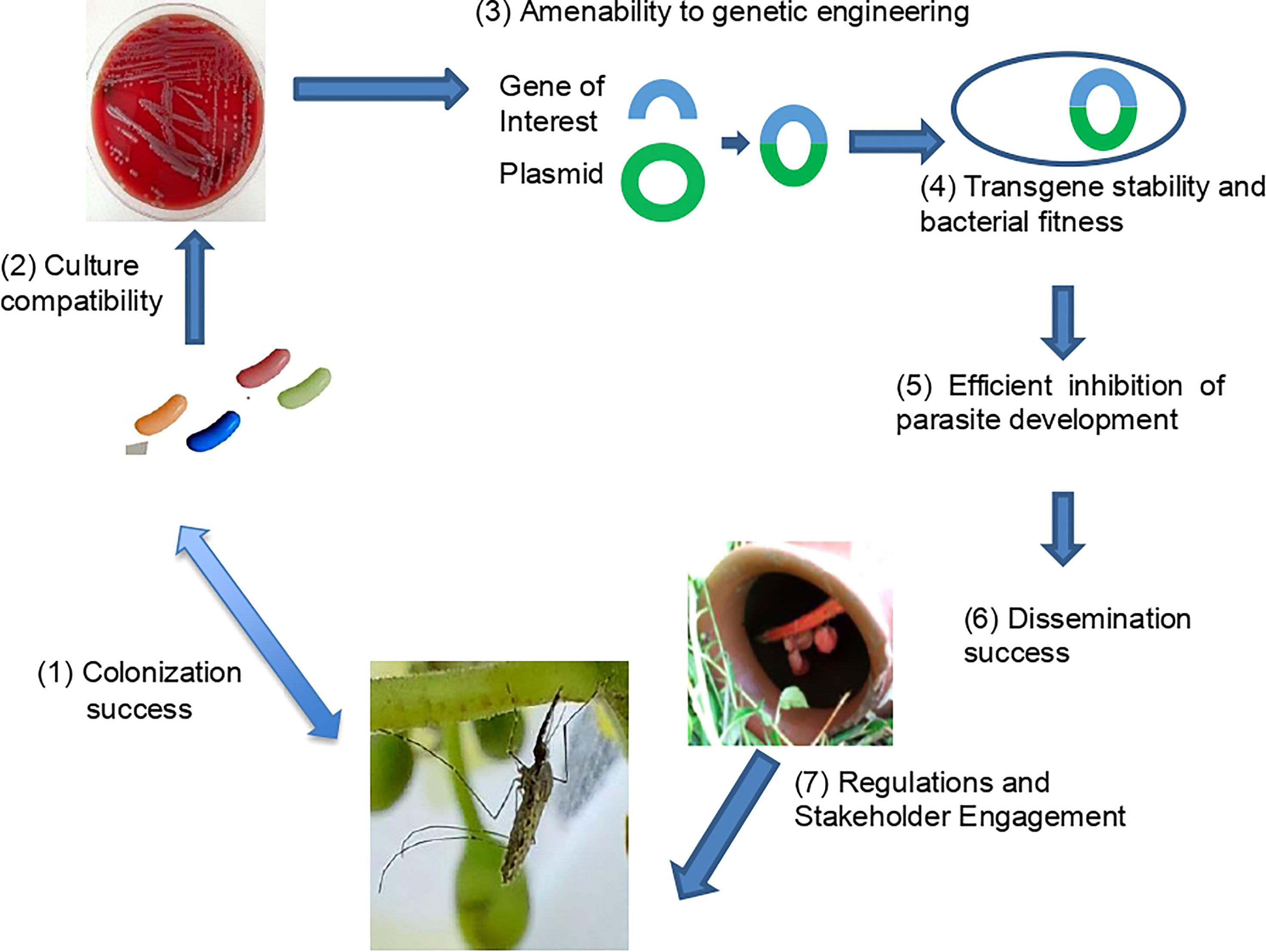
Figure 1 Seven requirements for a successful paratransgenic malaria control strategy (1). the microbial strain should be efficient at colonizing the targeted malaria vector(s). (2) The strain should be easily culturable. (3) The strain needs to be transformed with plasmids containing genes encoding effector molecules that block development or pathogen transmission (4) and remains stable in mosquito without fitness cost for the microbial strain. (5) The engineered bacterium should efficiently block parasites within the mosquito host. (6) The best approach to efficiently and safely disseminate the paratransgenic microbe should be defined. (7) Long-term interactions with local stakeholders (authorities, population) is essential to ensure the acceptability of the approach.
Amongst bacteria frequently found in wild populations of mosquitoes, Straif et al. found that P. agglomerans was the dominant bacterium in the gut of different mosquito species in Mali and Kenya (24). This bacterial species has also been commonly associated to Anopheles stephensi, Anopheles gambiae and Anopheles albimanus (25). Serratia has been reported in a number of studies with a wide geographic distribution (26, 27). A novel strain of Serratia sp. (AS1) has been proposed as a paratransgenic candidate by Wang et al. (17) due to its efficient horizontal and vertical transmission. When introducing 5% of Serratia-colonized mosquitoes in a cage, they observed that the next generation is fully colonized. Serratia sp. AS1 colonizes the gut, hemolymph, ovaries and male accessory glands of mosquitoes without affecting their fitness. It can disseminate rapidly in a mosquito cage population through vertical, horizontal and trans-stadial transmission. This genus is ubiquitous and notably commonly found in diverse mosquito species (17). Asaia is another bacterial genus that is found frequently in field mosquitoes and able to colonize several tissues in different species of malaria vectors such as An. stephensi and An. gambiae (28). This bacterium colonizes the larval gut, salivary glands and reproductive organs of laboratory-reared An. stephensi and field An. gambiae (29–31). It disseminates in mosquito populations via transmission from male to female, from larvae to adult and from female to larval progeny (29, 31, 32).
Compatibility to Genetic Engineering
Another important point is the amenability of the strain to genetic engineering. Hence, the first proof-of-principle for such symbiotic control has been provided using E. coli (33). Recombinant E. coli was first shown to significantly reduce P. berghei infection into Anopheles mosquitoes when expressing a single chain immunotoxin transgene. Six years later, E. coli was transformed to display SM1 (Salivary gland and Midgut peptide 1) and phospholipase-A(2) (PLA2), two anti-Plasmodium effector molecules, in its outer membrane (34). In an attempt to transfer such an approach to bacteria that naturally colonize mosquitoes, some studies then focused on P. agglomerans, which offers transgenesis possibility (15, 16). These studies were highly successful but Pantoea was found to have a limited colonization ability in mosquitoes. Serratia and Asaia were also found to be amenable to genetic manipulation leading to production of antimicrobial peptides (19, 25, 29).
Some yeasts and fungi can also be genetically engineered and/or may combine an entomopathogenic effect with an antiplasmodial impact. For instance, Metarhizium anisopliae an entomopathogenic fungus is known as killer of adult An. gambiae mosquitoes (35). It can be modified to express SM1 and scorpine, which were found to inhibit the development of Plasmodium reducing the sporozoite load (i.e., infection in the salivary glands) by 71-90% (36).
Natural Impact on Plasmodium
Several non-engineered strains have been found to naturally block malaria transmission. Starting with such an antimalarial strain can be seen as an advantage as it may increase the efficiency of transmission blocking and have a natural long-lasting impact in case the transgene is lost. Enterobacter EspZ, isolated in wild mosquitoes in Zambia, interferes with parasite development before midgut epithelium invasion. It renders mosquitoes refractory to P. falciparum infection by generating Reactive Oxygen Species (ROS) (37). Some strains of Serratia have also been found to naturally inhibit Plasmodium in mosquitoes (38). Serratia marcescens Y1 and Serratia ureilytica isolated from Anopheles sinensis interfere in the gut immunity to block the development of P. berghei, P. vivax and/or P. falciparum (26, 39). Chromobacterium sp. Panama isolated in the midgut of Ae. aegypti was also found to have a natural anti-Plasmodium activity in An. gambiae by secreting romidepsin, a histone diacetylase inhibitor (40). The bacterium is reported to activate the immune response as well as to reduce the larval and adult stages survivorship (41). The endosymbiont Wolbachia has been detected in several mosquito species in Africa, in a minority of individuals (42, 43). In An. gambiae and An. coluzzii, it has been found to negatively impact the late stages of Plasmodium development (44, 45).
Considering non-bacterial candidates, the yeast Wickerhamomyces anomalus stably colonizes the An. stephensi midgut reproductive organs. Some of its strains reduce the transmission of rodent malaria by inhibiting the P. berghei development (46). As it is recognized as a safe biocontrol agent, it has been proposed as an interesting “symbiotic-control tool” against transmission of malaria (47). Also related to fungi, Microsporidia MB naturally impairs P. falciparum development in An. gambiae s.l., yet this fungus is not currently amenable to culturing and genetic engineering (48).
Safe to the Environment
The implementation of paratransgenesis strategies requires to control the spread of the engineered microbiota strain specifically in the targeted natural mosquito populations or to ensure that a potential spread to the environment would not cause any damage (Table 1). This may be achieved using vertically-transmitted endosymbionts to prevent colonization of non-target species and spread to the environment. For instance, Wolbachia is a vertically-transmitted bacterium, transferred within the egg from mother to offspring. This obligate intracellular bacteria is detected in 40 to 69% terrestrial arthropods species including insects, mites and isopods (64–66). It is notably found in some mosquitoes, including Culex quinquefasciatus and Aedes albopictus (67) and used in Aedes aegypti to inhibit transmission of arthropod-borne viruses, notably dengue, as it naturally reduces vector competence (68). The release of Wolbachia-infected mosquitoes has already been shown efficient to colonize mosquito populations with this bacterium and to efficiently limit dengue in several countries (69). Hence, its potential in Anopheles is also investigated with much interest to decrease the malaria burden. It is found in Anopheles, but at a lower rate than in some Aedes and Culex species (42, 43) and with less efficient transmission over generations due to competition with Asaia (70, 71). As it has a natural negative impact on Plasmodium and is not yet amenable to culturing and/or genetic engineering, its use in Anopheles may first be developed as a natural blocker of transmission rather than as a paratransgenic tool. A putative plasmid encompassing 14 genes was however identified recently in Wolbachia in Culex mosquitoes, which may open additional possibilities for its genetic manipulation (72).
Paratransgenesis candidates may also be chosen because they are already ubiquitous in the environment, hence unlikely to cause any disturbance if used as paratransgenic transmission-blockers. For instance, P. agglomerans is found in nature, particular in surface of plants and flowers (73, 74), suggesting that its spread in natural mosquito populations may be envisaged using sugar baits (discussed below). It was described as a bacterium associated to wild and laboratory-reared mosquito species (22, 24, 34).
The impact of bacterial candidates on transmission of other pathogens should also be investigated. Notably, several Serratia species have been found to increase vector competence of Ae. aegypti to dengue and other arboviruses, notably via production of an enzyme which disturbs the gut mucus (75, 76). Thus, any Serratia paratransgenesis candidate will need to be specifically checked for such activity.
Strategies of Genetic Engineering
Transgene
As parasite numbers drastically decrease in the early stages of infection in the mosquito gut lumen (77), paratransgenesis may be used to bolster this reduction to reach complete elimination. To this aim, one of the important requirements is to identify and produce efficient effector molecules to block parasite development or kill it; doing so without impairing vector fitness or impairing its microbiota and surrounding organisms would avoid any selective pressure in these organisms (25, 78). Several antiplasmodial transgenes have been described and categorized to different classes according to their mechanism of action by Wang and Jacobs-Lorena (25).
Firstly, Scorpine, Gambicin, Hemolytic C-Type Lectin CEL-III, angiotensin II, magainins, synthetic anti-parasitic lytic peptides Shiva 1, Shiva 3 and gomesin have been mentioned to have lysis activities on P. falciparum and P. berghei (25). The use of paratransgenic antimicrobial peptides may however affect the mosquito microbiota as well as the paratransgenic bacterium itself in addition to Plasmodium. This may lead to indirect effects, such as a reduced microbiota load or the loss of the transgene, and thus interfere with the efficiency of the approach.
Secondly, some effector peptides block parasite development by inhibiting ookinetes or sporozoites bind to midgut or salivary gland epithelium surfaces. For example, EPIP (Plasmodium Enolase-Plasminogen interaction peptide) inhibits midgut invasion by preventing plasminogen binding to the ookinete surface (79). In 2012, Wang et al. used a combination of the antimicrobial peptide scorpine and of a peptide composed of 4 EPIPs to efficiently inhibit ookinete development in the mosquito gut (16). Anti-sporozoite or anti-ookinete single-chain monoclonal antibodies scFvs (Single Chain Variable Fragment) were also found to inhibit Plasmodium development in the mosquito, especially when combined with antimicrobial peptides (80, 81) suggesting that such transgenes may also be of interest for paratransgenesis purposes. Other proposed peptides include the 12-amino acid peptide SM1, which binds to putative receptors on the luminal surface of the mosquito midgut and basal surface of the salivary gland epithelia, blocking ookinete and sporozoite invasion (82), and phospholipase A2 (PLA2) that inhibits ookinete invasion, possibly by modifying the properties of the midgut epithelial membrane. E. coli has been engineered to inhibit the malaria parasite development by displaying SM1 and PLA2 on the bacterial surface, leading to a significant yet modest inhibition of P. berghei development (34).
Thirdly, manipulation of mosquito immunity may be an alternative strategy. Blood meal-induced expression of Akt, a key signaling component in the insulin signaling pathway, in transgenic mosquitoes renders the mosquito refractory to Plasmodium infection. Over expression of the IMD pathway-mediated transcription factor Rel2 renders the mosquito resistant to Plasmodium infection (83). Similarly, one can imagine the use of such microbes which would induce a basal immune response without too much cost for their own fitness. However, this kind of strategy has not yet been developed, as far as we know.
Expression of the Transgene
When considering transgene expression, several issues need to be addressed. First, recombinant proteins may remain blocked into the bacterial cell, preventing them to reach their target. To address this, Wang et al. used the Type-1 secretion system HasA in Serratia, which allows the secretion of their unfolded transgenic polypeptide through both membranes in one step. Grogan et al. recently identified novel secretion signals in Asaia sp (84). Bacteria carrying two of these signals allowed more efficient excretion than the initial paratransgenesis strain. Even though the increased release of scorpine comes with a cost, reflected by a reduction in bacterial growth and colonization potential, strains carrying these signals have higher antiplasmodial activity against P. berghei than the initial strain. Secondly, the transgene needs to be expressed at a high level to reach efficiency. To this aim, Wang et al. cloned five effector genes, two of them present in several copies, as a single construct of in Serratia, which are secreted together and inhibit ookinete development in the mosquito (17).Thirdly, some transgenes are antimicrobials hence it is necessary to deal with potential fitness costs for the microbe. Shane et al. addressed this question by producing an Asaia strain expressing antiplasmodial effectors specifically after the blood meal in order to increase the fitness of their paratransgenic bacterium and thus to promote dissemination throughout large population of field mosquitoes (19).
Dealing With Transgene Stability
The stable introduction of an anti-parasitic gene at a sufficient frequency and efficacy into a population of vector mosquitoes remains challenging. The development of inducible transgenes contributes to address this problem by reducing fitness costs for bacteria (19). However, no transgene has yet been found to be stable in paratransgenic candidates. On the contrary, Huang et al. found that Serratia AS1, which efficiently spreads in Anopheles and inhibit P. falciparum in mosquitoes after genetic manipulation, loses the plasmid carrying the exogenous gene in three mosquito generations. They also did not detect any plasmid horizontal transfer from Serratia to other bacteria in the mosquito gut (18). They pointed out that this lack of stability may be an advantage in terms of safety: using such transient transgenes, which are lost after one to two months, would allow to reduce the burden of a seasonal outbreak without spreading transgenes in the long-term in a vector population, which would reduce parasite resistance selection risk or other non-foreseen problematics. Such an approach based on an unstable transgene may get authorities approval in a shorter term. This instability, together with the reported semi-isolation of mosquito populations from neighboring villages in terms of microbiota composition (85), may provide arguments to progress in investigating the feasibility of paratransgenesis in the field while reducing the risk of out-of-control transgene spread.
Thus, two alternative strategies with different advantages can be considered regarding transgene stability. On one hand, implementation of stable paratransgenesis may be performed once and reduce disease transmission for years, similar to the current use of non-engineered Wolbachia to limit dengue transmission (57, 68, 71, 86, 87). This approach proves to be cost effective but requires a good control of the risks to the environment, it is thus restricted so far to non-genetically-engineered biocontrol agents. Alternatively, an unstable paratransgenesis approach, where the microbe and/or the transgene are lost after some generations, may be used to reduce environmental risks (18). In this case, implementation campaigns will be regularly required.
Avoiding Development of Antimicrobial Resistance
Prior to release genetically-modified bacteria to the environment, it is important to consider antibiotic resistance issues. Antibiotic-resistance elements are known to be efficiently spread across bacterial species, which has disastrous consequences for human health. When considering paratransgenesis, it is essential not to introduce any antibiotic-resistance marker used for genetic engineering and to avoid resistance to antimicrobial peptides.
First, plasmids and/or chromosomes need to be cleared from any antibiotic-resistance gene used for genetic engineering. To this aim, counter selection methods have been set up to kill bacteria under some specific nutritive conditions if they carry an adjacent gene to the resistance marker (88).
Secondly, the antiparasitic activity should be designed in a way to avoid the rise of microbial resistance. Considering resistance in Plasmodium, the combination of several antimicrobial peptides with inhibitors of parasite binding to the gut, as developed in Serratia, increases efficiency while also reducing the risk of developing resistance (17). Considering resistance in a wider microbial community due to the pressure of wide-spectrum antimicrobial peptides, the latter are thought to be safer than empirical antibiotics, with a low tendency to select for resistance (89). As they are thought to be key to deal with antibiotic resistance issues in the future, the resistance that they select for is under active study (90). Where resistance may still develop, it will be important to know about resistance mechanisms and their potential for horizontal transfer prior to any use in paratransgenesis. Inducible expression of the transgene in specific conditions, e.g. specifically in the blood-fed mosquito gut, may also reduce the selective pressure on the microbiota. Alternatively, the identification of any antimicrobial peptide with a narrow spectrum, active on parasites without harming the mosquito microbiota, would be interesting.
From the Laboratory to Implementation
Unlike vector control tools, the goal of paratransgenesis in malaria control is not to kill the mosquito, but to transform it in an ineffective vector. Similar to population replacement strategies, the ecological niche remains occupied by the same mosquito species, yet vectorial capacity is reduced. Hence, this type of approach is thought to have a reduced risk of ecological disturbance due to the spread of an initially low-abundant competitor species, compared approaches removing a mosquito population and thus leaving an empty niche. Population replacement strategies notably include the release of Wolbachia-carrying Ae. aegypti to inhibit dengue transmission (69, 91), or of genetically-engineering mosquitoes with reduced vector competence (92).
Prior to implementation, vector control using paratransgenesis requires in depth ecological studies. This involves different steps in contained environments, starting with as small and large cages in the laboratory, following up with semi-field trials and finally with field trials. Some recent studies focused on the ability of some paratransgenesis candidate strains to colonize mosquito populations. Mancini et al. used large cages simulating near-natural ecosystem conditions for an effective paratransgenesis set-up. Upon introduction of transgenic Asaia in large cages via colonized males or soaked cotton pads, they observed an efficient spread of transgenic bacteria in populations of An. gambiae or An. stephensi with both introduction methods, indicating a promising perspective to open field trials (32). It would be interesting to perform the same test with other paratransgenesis candidates to have a wider picture of their diffusion and transmission through mosquito populations. Moreover, before field trials, the efficacy and safety of transgenes and paratransgenic mosquito life-history traits (lifespan, mobility, mating success) would need to be tested in large outdoor settings such as ‘Malaria Spheres’ in order to expose mosquitoes to normal environmental conditions. A recent study indicated that the spread of engineered refractoriness to Plasmodium infection in a vector population depends on the dispersal and mating behavior of the introduced engineered specimen (93). In Burkina Faso, the recent semi-field study of Nignan et al. showed that colonized mosquitoes were more likely to mate together than with their field counterparts (94). As mating success is known to be affected by microbiota composition in laboratory conditions (95), such cross-mating assessment in semi-field settings will also be required regarding candidate paratransgenic approaches. Modifications in microbiota composition in the surrounding mosquito population will also need to be tested in semi-field conditions before environmental trials, to assess colonization success of the paratransgenic microbe and other secondary impacts on mosquito microbiota.
In such set ups, Lovett et al. showed a successful dissemination of wild-type Metarhizium pingshaense fungi in An. gambiae and An. coluzzii mosquitoes in Burkina Faso (96). Such outdoor experiments allow to take into account highly variable and uncontrollable parameters which mosquitoes will face in the field, including temperature and relative humidity fluctuations that influence mosquito physiology, metabolism and survival (97). Highly variable factors including seasonality, diet, genetic identity, blood source, larval breeding site are also known to affect mosquito microbiota composition independently to mosquito species (98, 99). How such factors may affect the efficiency of a paratransgenesis approach has not yet been specifically assessed.
Going to field conditions, release of modified bacteria has not yet been performed but a recent study used cotton baits soaked with sugar, fruit cocktail or honey and placed in clay jars or in window entry traps around villages where malaria is prevalent to evaluate sugar-bait set ups for prospective bacterial release (100). Window entry traps were found to attract more mosquitoes but the proportion of sugar fed mosquitoes was higher in clay jars, and honey was the best attractant. Prior to any field study involving a paratransgenesis candidate, the spread of genetically engineered constructs will require to ensure the acceptability of the chosen approach with the population. As this method may lead to some modification in the environment, interactions with local stakeholders will be required to balance such changes with the benefit for human health and the cost effectiveness of the approach. Guidance on such public engagement practices has been published, emphasizing that specific funding should be allocated to public engagement, which should start from early stages of the project and be active over many years, throughout the project. Such long-term interaction with mutual respect between different stakeholders and experts in diverse topics such as biology, history or politics, aims to promote honesty and transparency, und thus to reduce the risk of distrust (101, 102). Such experience is being built in countries including Burkina Faso, Mali and Uganda, where the first field trials of gene drive are expected in the next 5-10 years through the Target malaria project (103). This project identified key stakeholders, who will similarly need to be involved when considering the implementation of any other novel transmission-blocking approach such as paratransgenesis (103, 104).
Conclusion
This work reviewed recent progress in the development of paratransgenesis, a promising proposed strategy to reduce malaria transmission. We reviewed the arguments to consider when selecting paratransgenesis candidates and the genetic manipulation strategies under development. Vast efforts have also been spent to investigate bacterial spread in laboratory and semi-field mosquito populations. The stable introduction of modified microbes in field vector populations, the effect of antibiotics during mass-drug administration campaigns on transgenic bacteria in mosquitoes in endemic area and the risk of antimicrobial resistance selection against introduced transgenes are still questions to address before effective implementation of this approach. Despite these concerns, the approach presents advantages including a simple and fast genetic manipulation and potential for easy and low-cost introduction into mosquito populations. Moreover, the spread of the genetically-engineered bacteria within wild mosquito populations will not face the genetic reproduction barriers observed with genetically-modified mosquitoes. Regarding the regulatory and ethical requirements, accurate guidelines for field application on release of modified bacteria in the environment will need to be discussed in close collaboration with local authorities and representatives of the population. Altogether, paratransgenesis appears as an approach of interest for malaria control, compatible with other current and future control methods, from the use of insecticides to transgenic mosquitoes or transmission-blocking strategies.
Author Contributions
Conceptualization, AF, MG, and RSY; Methodology, AF and M.G; Writing – Original Draft, AF and MG; Writing – Review and Editing, AF, EB, and MG; Supervision, MG, GO, RSY, and J-BO. All authors contributed to the article and approved the submitted version.
Funding
This work was supported by the Developing Excellence in Leadership and Genetic Training for Malaria Elimination in Sub-Saharan Africa (PhD studentship number 041 to AF), by the French Government’s Investissement d’Avenir program, Laboratoire d’Excellence “Integrative Biology of Emerging Infectious Diseases” (grant no. ANR-10-LABX-62-IBEID) and by ANR JCJC MosMi (grant no. ANR-18-CE15-0007) to MG.
Conflict of Interest
The authors declare that the research was conducted in the absence of any commercial or financial relationships that could be construed as a potential conflict of interest.
Publisher’s Note
All claims expressed in this article are solely those of the authors and do not necessarily represent those of their affiliated organizations, or those of the publisher, the editors and the reviewers. Any product that may be evaluated in this article, or claim that may be made by its manufacturer, is not guaranteed or endorsed by the publisher.
Acknowledgments
We thank Abdoulaye Djimde for support, Ottavia Romoli for critical reading of the manuscript and Hilde de Reuse for insightful discussion.
References
1. Institute of Medicine. Vector-Borne Diseases. Washington, D.C: National Academies Press (2008). Available at: http://www.nap.edu/catalog/11950.
2. Wilke ABB, Marrelli MT. Paratransgenesis: A Promising New Strategy for Mosquito Vector Control. Parasit Vectors (2015) 8(1):342. doi: 10.1186/s13071-015-0959-2
3. Dreistadt SH, Dahlsten DL. Report on Reports: Pesticide Resistance: Strategies and Tactics for Management. Environ Sci Policy Sustain Dev (1987) 29(3):25–7. doi: 10.1080/00139157.1987.9928872
4. Fonseca DM, Smith JL, Wilkerson RC, Fleischer RC. Pathways of Expansion and Multiple Introductions Illustrated by Large Genetic Differentiation Among Worldwide Populations of the Southern House Mosquito. Am J Trop Med Hyg (2006) 74(2):284–9. doi: 10.4269/ajtmh.2006.74.284
5. WHO. World Malaria Report 2021 (2021). Available at: https://www.who.int/india/health-topics/malaria/summary-of-world-malaria-report-2021.
6. Riehle MA, Jacobs-Lorena M. Using Bacteria to Express and Display Anti-Parasite Molecules in Mosquitoes: Current and Future Strategies. Insect Biochem Mol Biol (2005) 35(7):699–707. doi: 10.1016/j.ibmb.2005.02.008
7. Beard CB, Cordon-Rosales C, Durvasula RV. Bacterial Symbionts of the Triatominae and Their Potential Use in Control of Chagas Disease Transmission. Annu Rev Entomol (2002) 47(1):123–41. doi: 10.1146/annurev.ento.47.091201.145144
8. Durvasula RV, Gumbs A, Panackal A, Kruglov O, Aksoy S, Merrifield RB, et al. Prevention of Insect-Borne Disease: An Approach Using Transgenic Symbiotic Bacteria. Proc Natl Acad Sci USA (1997) 94(7):3274–8. doi: 10.1073/pnas.94.7.3274
9. Durvasula RV, Gumbs A, Panackal A, Kruglov O, Taneja J, Kang AS, et al. Expression of a Functional Antibody Fragment in the Gut of Rhodnius Prolixus via Transgenic Bacterial Symbiont Rhodococcus Rhodnii. Med Vet Entomol (1999) 13(2):115–9. doi: 10.1046/j.1365-2915.1999.00175.x
10. Weiss BL, Mouchotte R, Rio RVM, Wu Y, Wu Z, Heddi A, et al. Interspecific Transfer of Bacterial Endosymbionts Between Tsetse Fly Species: Infection Establishment and Effect on Host Fitness. Appl Environ Microbiol (2006) 72(11):7013–21. doi: 10.1128/AEM.01507-06
11. Aksoy S, Weiss B, Attardo G. Paratransgenesis Applied for Control of Tsetse Transmitted Sleeping Sickness. In: Transgenesis and the Management of Vector-Borne Disease. New York, NY: Springer New York (2008) 35–48. doi: 10.1007/978-0-387-78225-6_3
12. Hu Y, Aksoy S. An Antimicrobial Peptide With Trypanocidal Activity Characterized From Glossina Morsitans Morsitans. Insect Biochem Mol Biol (2005) 35(2):105–15. doi: 10.1016/j.ibmb.2004.10.007
13. Hu C, Aksoy S. Innate Immune Responses Regulate Trypanosome Parasite Infection of the Tsetse Fly Glossina Morsitans Morsitans. Mol Microbiol (2006) 60(5):1194–204. doi: 10.1111/j.1365-2958.2006.05180.x
14. Hao Z, Kasumba I, Lehane MJ, Gibson WC, Kwon J, Aksoy S. Tsetse Immune Responses and Trypanosome Transmission: Implications for the Development of Tsetse-Based Strategies to Reduce Trypanosomiasis. Proc Natl Acad Sci (2001) 98(22):12648–53. doi: 10.1073/pnas.221363798
15. Bisi DC, Lampe DJ. Secretion of Anti-Plasmodium Effector Proteins From a Natural Pantoea Agglomerans Isolate by Using PelB and HlyA Secretion Signals. Appl Environ Microbiol (2011) 77(13):4669–75. doi: 10.1128/AEM.00514-11
16. Wang S, Ghosh AK, Bongio N, Stebbings KA, Lampe DJ, Jacobs-Lorena M. Fighting Malaria With Engineered Symbiotic Bacteria From Vector Mosquitoes. Proc Natl Acad Sci (2012) 109(31):12734–9. doi: 10.1073/pnas.1204158109
17. Wang S, Dos-Santos ALA, Huang W, Liu KC, Oshaghi MA, Wei G, et al. Driving Mosquito Refractoriness to Plasmodium Falciparum With Engineered Symbiotic Bacteria. Science (80- ) (2017) 357(6358):1399–402. doi: 10.1126/science.aan5478
18. Huang W, Wang S, Jacobs-Lorena M. Self-Limiting Paratransgenesis. PloS Negl Trop Dis (2020) 14(8):e0008542. doi: 10.1371/journal.pntd.0008542. Ribeiro JMC, editor.
19. Shane JL, Grogan CL, Cwalina C, Lampe DJ. Blood Meal-Induced Inhibition of Vector-Borne Disease by Transgenic Microbiota. Nat Commun (2018) 9(1):4127. doi: 10.1038/s41467-018-06580-9
20. Jadin J, Vincke IH, Dunjic A, Delville JP, Wery M, Bafort J, et al. [Role of Pseudomonas in the Sporogenesis of the Hematozoon of Malaria in the Mosquito]. Bull Soc Pathol Exot Filiales (1966) 59(4):514–25.
21. Minard G, Mavingui P, Moro CV. Diversity and Function of Bacterial Microbiota in the Mosquito Holobiont. Parasit Vectors (2013) 6(1):1–12. doi: 10.1186/1756-3305-6-146
22. Pumpuni CB, Demaio J, Kent M, Davis JR, Beier JC. Bacterial Population Dynamics in Three Anopheline Species: The Impact on Plasmodium Sporogonic Development. Am J Trop Med Hyg (1996) 54(2):214–8. doi: 10.4269/ajtmh.1996.54.214
23. Wang Y, Gilbreath TM, Kukutla P, Yan G, Xu J. Dynamic Gut Microbiome Across Life History of the Malaria Mosquito Anopheles Gambiae in Kenya. PloS One (2011) 6(9):e24767. doi: 10.1371/journal.pone.0024767. Leulier F, editor.
24. Straif SC, Mbogo CNM, Toure AM, Walker ED, Kaufman M, Toure YT, et al. Midgut Bacteria in Anopheles Gambiae and An. Funestus (Diptera: Culicidae) From Kenya and Mali. J Med Entomol (1998) 35(3):222–6. doi: 10.1093/jmedent/35.3.222
25. Wang S, Jacobs-Lorena M. Paratransgenesis Applications. In: Arthropod Vector: Controller of Disease Transmission, London, United Kingdom: Academic Press Is an imprint of Elsevier (2017) 1:219–34. doi: 10.1016/B978-0-12-805350-8.00013-1
26. Gao H, Bai L, Jiang Y, Huang W, Wang L, Li S, et al. A Natural Symbiotic Bacterium Drives Mosquito Refractoriness to Plasmodium Infection via Secretion of an Antimalarial Lipase. Nat Microbiol (2021) 6(6):806–17. doi: 10.1038/s41564-021-00899-8
27. Gendrin M, Christophides GK. The Anopheles Mosquito Microbiota and Their Impact on Pathogen Transmission. In: Anopheles Mosquitoes - New Insights Into Malaria Vectors. London, United Kingdom: InTech (2013). Available at: http://www.intechopen.com/books/anopheles-mosquitoes-new-insights-into-malaria-vectors/the-anopheles-mosquito-microbiota-and-their-impact-on-pathogen-transmission.
28. Damiani C, Ricci I, Crotti E, Rossi P, Rizzi A, Scuppa P, et al. Mosquito-Bacteria Symbiosis: The Case of Anopheles Gambiae and Asaia. Microb Ecol (2010) 60(3):644–54. doi: 10.1007/s00248-010-9704-8
29. Favia G, Ricci I, Damiani C, Raddadi N, Crotti E, Marzorati M, et al. Bacteria of the Genus Asaia Stably Associate With Anopheles Stephensi, an Asian Malarial Mosquito Vector. Proc Natl Acad Sci (2007) 104(21):9047–51. doi: 10.1073/pnas.0610451104
30. Damiani C, Ricci I, Crotti E, Rossi P, Rizzi A, Scuppa P, et al. Paternal Transmission of Symbiotic Bacteria in Malaria Vectors. Curr Biol (2008) 18(23):R1087–8. doi: 10.1016/j.cub.2008.10.040
31. Favia G, Ricci I, Marzorati M, Negri I, Alma A, Sacchi L, et al. Bacteria of the Genus Asaia: A Potential Paratransgenic Weapon Against Malaria. In: Transgenesis and the Management of Vector-Borne Disease. New York, NY: Springer New York (2008). p. 49–59. doi: 10.1007/978-0-387-78225-6_4
32. Mancini MV, Spaccapelo R, Damiani C, Accoti A, Tallarita M, Petraglia E, et al. Paratransgenesis to Control Malaria Vectors: A Semi-Field Pilot Study. Parasit Vectors (2016) 9(1):1–9. doi: 10.1186/s13071-016-1427-3
33. Yoshida S, Ioka D, Matsuoka H, Endo H, Ishii A. Bacteria Expressing Single-Chain Immunotoxin Inhibit Malaria Parasite Development in Mosquitoes. Mol Biochem Parasitol (2001) 113(1):89–96. doi: 10.1016/S0166-6851(00)00387-X
34. Riehle MA, Moreira CK, Lampe D, Lauzon C, Jacobs-Lorena M. Using Bacteria to Express and Display Anti-Plasmodium Molecules in the Mosquito Midgut. Int J Parasitol (2007) 37(6):595–603. doi: 10.1016/j.ijpara.2006.12.002
35. Scholte E-J, Ng’habi K, Kihonda J, Takken W, Paaijmans K, Abdulla S, et al. An Entomopathogenic Fungus for Control of Adult African Malaria Mosquitoes. Science (80- ) (2005) 308(5728):1641–2. doi: 10.1126/science.1108639
36. Fang W, Vega-Rodríguez J, Ghosh AK, Jacobs-Lorena M, Kang A, St. Leger RJ. Development of Transgenic Fungi That Kill Human Malaria Parasites in Mosquitoes. Science (80- ) (2011) 331(6020):1074–7. doi: 10.1126/science.1199115
37. Cirimotich CM, Dong Y, Clayton AM, Sandiford SL, Souza-Neto JA, Mulenga M, et al. Natural Microbe-Mediated Refractoriness to Plasmodium Infection in Anopheles Gambiae. Science (80- ) (2011) 332(6031):855–8. doi: 10.1126/science.1201618
38. Bando H, Okado K, Guelbeogo WM, Badolo A, Aonuma H, Nelson B, et al. Intra-Specific Diversity of Serratia Marcescens in Anopheles Mosquito Midgut Defines Plasmodium Transmission Capacity. Sci Rep (2013) 3(1):1641. doi: 10.1038/srep01641
39. Bai L, Wang L, Vega-Rodríguez J, Wang G, Wang S. A Gut Symbiotic Bacterium Serratia Marcescens Renders Mosquito Resistance to Plasmodium Infection Through Activation of Mosquito Immune Responses. Front Microbiol (2019) 10:1580/full(JULY). doi: 10.3389/fmicb.2019.01580/full
40. Saraiva RG, Huitt-roehl CR, Tripathi A, Cheng Y, Bosch J, Townsend CA, et al. Chromobacterium spp. Mediate Their Anti-Plasmodium Activity Through Secretion of the Histone Deacetylase Inhibitor Romidepsin. Sci Rep (2018). 8:6176 doi: 10.1038/s41598-018-24296-0
41. Ramirez JL, Short SM, Bahia AC, Saraiva RG, Dong Y, Kang S, et al. Chromobacterium Csp_P Reduces Malaria and Dengue Infection in Vector Mosquitoes and Has Entomopathogenic and In Vitro Anti-Pathogen Activities. PloS Pathog (2014) 10(10):e1004398. doi: 10.1371/journal.ppat.1004398. Levashina E, editor.
42. Jeffries CL, Rogers ME, Walker T. Establishment of a Method for Lutzomyia Longipalpis Sand Fly Embryo Microinjection: The First Step Towards Potential Novel Control Strategies for Leishmaniasis. Wellcome Open Res (2018) 3(0):55. doi: 10.12688/wellcomeopenres.14555.1
43. Baldini F, Segata N, Pompon J, Marcenac P, Robert Shaw W, Dabiré RK, et al. Evidence of Natural Wolbachia Infections in Field Populations of Anopheles Gambiae. Nat Commun (2014) 5(1):3985. doi: 10.1038/ncomms4985
44. Gomes FM, Hixson BL, Tyner MDW, Ramirez JL, Canepa GE, Alves e Silva TL, et al. Effect of Naturally Occurring Wolbachia in Anopheles Gambiae s.L. Mosquitoes From Mali on Plasmodium Falciparum Malaria Transmission. Proc Natl Acad Sci USA (2017) 114(47):12566–71. doi: 10.1073/pnas.1716181114
45. Shaw WR, Marcenac P, Childs LM, Buckee CO, Baldini F, Sawadogo SP, et al. Wolbachia Infections in Natural Anopheles Populations Affect Egg Laying and Negatively Correlate With Plasmodium Development. Nat Commun (2016) 7(May):11772. doi: 10.1038/ncomms11772
46. Cappelli A, Valzano M, Cecarini V, Bozic J, Rossi P, Mensah P, et al. Killer Yeasts Exert Anti-Plasmodial Activities Against the Malaria Parasite Plasmodium Berghei in the Vector Mosquito Anopheles Stephensi and in Mice. Parasit Vectors (2019) 12(1):329. doi: 10.1186/s13071-019-3587-4
47. Cappelli A, Favia G, Ricci I. Wickerhamomyces Anomalus in Mosquitoes: A Promising Yeast-Based Tool for the “Symbiotic Control” of Mosquito-Borne Diseases. Front Microbiol (2021) 11:621605/full(January). doi: 10.3389/fmicb.2020.621605/full
48. Herren JK, Mbaisi L, Mararo E, Makhulu EE, Mobegi VA, Butungi H, et al. A Microsporidian Impairs Plasmodium falciparum Transmission in Anopheles arabiensis Mosquitoes. Nat Commun (2020) 11(1):2187. doi: 10.1038/s41467-020-16121-y
49. Yoshida S, Shimada Y, Kondoh D, Kouzuma Y, Ghosh AK, Jacobs-Lorena M, et al. Hemolytic C-Type Lectin CEL-III From Sea Cucumber Expressed in Transgenic Mosquitoes Impairs Malaria Parasite Development. PloS Pathog (2007) 3(12):e192. Vernick K, editor. doi: 10.1371/journal.ppat.0030192
50. Favia G, Ricci I, Scuppa P, Damiani C, Rossi P, Capone A, et al. Facing Malaria Parasite With Mosquito Symbionts. In: Malaria Parasites. London, United Kingdom: InTech (2012). Available at: http://www.intechopen.com/books/malaria-parasites/facing-malaria-parasite-with-mosquito-symbionts-.
51. Ricci I, Valzano M, Ulissi U, Epis S, Cappelli A, Favia G. Symbiotic Control of Mosquito Borne Disease. Pathog Glob Health (2012) 106(7):380–5. doi: 10.1179/2047773212Y.0000000051
52. Bahia AC, Dong Y, Blumberg BJ, Mlambo G, Tripathi A, BenMarzouk-Hidalgo OJ, et al. Exploring Anopheles Gut Bacteria for Plasmodium Blocking Activity. Environ Microbiol (2014) 16(9):2980–94. doi: 10.1111/1462-2920.12381
53. Stathopoulos S, Neafsey DE, Lawniczak MKN, Muskavitch MAT, Christophides GK. Genetic Dissection of Anopheles gambiae Gut Epithelial Responses to Serratia Marcescens. PloS Pathog (2014) 10(3):e1003897. doi: 10.1371/journal.ppat.1003897. Schneider DS, editor.
54. Ragvendran C, Natarajan D. Serratia marcescens (Enterobacteriaceae): An Alternate Biocontrol Agent for Mosquito Vectors Aedes aegypti and Culex quinquefasciatus (Diptera: Culicidae). PTB Reports (2017) 3(1):14-20.doi: 10.5530/PTB.2017.3.3
55. Heu K, Romoli O, Schönbeck JC, Ajenoe R, Epelboin Y, Kircher V, et al. The Effect of Secondary Metabolites Produced by Serratia marcescens on Aedes aegypti and Its Microbiota. Front Microb (2021) 12(July):1–13. doi: 10.3389/fmicb.2021.645701
56. Bian G, Joshi D, Dong Y, Lu P, Zhou G, Pan X, et al. Wolbachia Invades Anopheles stephensi Populations and Induces Refractoriness to Plasmodium Infection. Science (2013) 340(6133):748–51. doi: 10.1126/science.1236192
57. Hughes GL, Koga R, Xue P, Fukatsu T RJ, Hughes GL, Koga R, et al. Wolbachia Infections Are Virulent and Inhibit the Human Malaria Parasite Plasmodium Falciparum in Anopheles Gambiae. PloS Pathog (2011) 7(pat.1002043):e1002043. Schneider DS, editor. doi: 10.1371/journal.ppat.1002043
58. Cappelli A, Ulissi U, Valzano M, Damiani C, Epis S, Gabrielli MG, et al. A Wickerhamomyces anomalus Killer Strain in the Malaria Vector Anopheles stephensi. PloS One (2014) 9(5):e95988. doi: 10.1371/journal.pone.0095988. Moreira LA, editor.
59. Ricci I, Mosca M, Valzano M, Damiani C, Scuppa P, Rossi P, et al. Different Mosquito Species Host Wickerhamomyces Anomalus (Pichia anomala): Perspectives on Vector-Borne Diseases Symbiotic Control. Antonie Van Leeuwenhoek (2011) 99(1):43–50. doi: 10.1007/s10482-010-9532-3
60. Garza-Hernández JA, Rodríguez-Pérez MA, Salazar MI, Russell TL, Adeleke MA, de Luna-Santillana E de J, et al. Vectorial Capacity of Aedes aegypti for Dengue Virus Type 2 Is Reduced With Co-Infection of Metarhizium anisopliae. PloS Negl Trop Dis (2013) 7(3):e2013. doi: 10.1371/journal.pntd.0002013. Turell MJ, editor.
61. Blanford S, Jenkins NE, Read AF, Thomas MB. Evaluating the Lethal and Pre-Lethal Effects of a Range of Fungi Against Adult Anopheles stephensi Mosquitoes. Malar J (2012) 11(1):365. doi: 10.1186/1475-2875-11-365
62. Dong Y, Morton JC, Ramirez JL, Souza-Neto JA, Dimopoulos G. The Entomopathogenic Fungus Beauveria bassiana Activate Toll and JAK-STAT Pathway-Controlled Effector Genes and Anti-Dengue Activity in Aedes aegypti. Insect Biochem Mol Biol (2012) 42(2):126–32. doi: 10.1016/j.ibmb.2011.11.005
63. Blanford S, Chan BHK, Jenkins N, Sim D, Turner RJ, Read AF, et al. Fungal Pathogen Reduces Potential for Malaria Transmission. Science (80- ) (2005) 308(5728):1638–41. doi: 10.1126/science.1108423
64. Serbus LR, Casper-Lindley C, Landmann F, Sullivan W. The Genetics and Cell Biology of Wolbachia -Host Interactions. Annu Rev Genet (2008) 42(1):683–707. doi: 10.1146/annurev.genet.41.110306.130354
65. Townson H. Wolbachia as a Potential Tool for Suppressing Filarial Transmission. Ann Trop Med Parasitol (2002) 96 Suppl 2(8):S117–27. doi: 10.1179/000349802125002464
66. Ferri E, Bain O, Barbuto M, Martin C, Lo N, Uni S, et al. New Insights Into the Evolution of Wolbachia Infections in Filarial Nematodes Inferred From a Large Range of Screened Species. PloS One (2011) 6(6):e20843. doi: 10.1371/journal.pone.0020843. Rénia L, editor.
67. Atyame CM, Pasteur N, Dumas E, Tortosa P, Tantely ML, Pocquet N, et al. Cytoplasmic Incompatibility as a Means of Controlling Culex Pipiens Quinquefasciatus Mosquito in the Islands of the South-Western Indian Ocean. PloS Negl Trop Dis (2011) 5(12):e1440. doi: 10.1371/journal.pntd.0001440. Dinglasan RR, editor.
68. Moreira LA, Iturbe-Ormaetxe I, Jeffery JA, Lu G, Pyke AT, Hedges LM, et al. A Wolbachia Symbiont in Aedes aegypti Limits Infection With Dengue, Chikungunya, and Plasmodium. Cell (2009) 139(7):1268–78. doi: 10.1016/j.cell.2009.11.042
69. Utarini A, Indriani C, Ahmad RA, Tantowijoyo W, Arguni E, Ansari MR, et al. Efficacy of Wolbachia-Infected Mosquito Deployments for the Control of Dengue. N Engl J Med (2021) 384(23):2177–86. doi: 10.1056/NEJMoa2030243
70. Rossi P, Ricci I, Cappelli A, Damiani C, Ulissi U, Mancini MV, et al. Mutual Exclusion of Asaia and Wolbachia in the Reproductive Organs of Mosquito Vectors. Parasit Vectors (2015) 8(1):1–10. doi: 10.1186/s13071-015-0888-0
71. Hughes GL, Dodson BL, Johnson RM, Murdock CC, Tsujimoto H, Suzuki Y, et al. Native Microbiome Impedes Vertical Transmission of Wolbachia in Anopheles Mosquitoes. Proc Natl Acad Sci (2014) 111(34):12498–503. doi: 10.1073/pnas.1408888111
72. Reveillaud J, Bordenstein SR, Cruaud C, Shaiber A, Esen ÖC, Weill M, et al. The Wolbachia Mobilome in Culex Pipiens Includes a Putative Plasmid. Nat Commun (2019) 10(1):1051. doi: 10.1038/s41467-019-08973-w
73. Andrews JH, Harris RF. The Ecology and Biogeography of Microorganisms on Plant Surfaces. Annu Rev Phytopathol (2000) 38:145–80. doi: 10.1146/annurev.phyto.38.1.145
74. Pusey PL. Crab Apple Blossoms as a Model System for Fire Blight Biocontrol Research. Acta Hortic (1996) 411):289–94. doi: 10.17660/ActaHortic.1996.411.57
75. Wu P, Sun P, Nie K, Zhu Y, Shi M, Xiao C, et al. A Gut Commensal Bacterium Promotes Mosquito Permissiveness to Arboviruses. Cell Host Microbe (2019) 25(1):101–112.e5. doi: 10.1016/j.chom.2018.11.004
76. Apte-Deshpande A, Paingankar M, Gokhale MD, Deobagkar DN. Serratia Odorifera a Midgut Inhabitant of Aedes aegypti Mosquito Enhances Its Susceptibility to Dengue-2 Virus. PloS One (2012) 7(7):e40401. doi: 10.1371/journal.pone.0040401. Vasilakis N, editor.
77. Abraham EG, Jacobs-Lorena M. Mosquito Midgut Barriers to Malaria Parasite Development. Insect Biochem Mol Biol (2004) 34(7):667–71. doi: 10.1016/j.ibmb.2004.03.019
78. Jacobs-Lorena M. Interrupting Malaria Transmission by Genetic Manipulation of Anopheline Mosquitoes. J Vector Borne Dis (2003) 40(3–4):73–7.
79. Ghosh AK, Coppens I, Gardsvoll H, Ploug M, Jacobs-Lorena M. Plasmodium Ookinetes Coopt Mammalian Plasminogen to Invade the Mosquito Midgut. Proc Natl Acad Sci (2011) 108(41):17153–8. doi: 10.1073/pnas.1103657108
80. Isaacs AT, Li F, Jasinskiene N, Chen X, Nirmala X, Marinotti O, et al. Engineered Resistance to Plasmodium falciparum Development in Transgenic Anopheles stephensi. PloS Pathog (2011) 7(4):e1002017. doi: 10.1371/journal.ppat.1002017. Besansky NJ, editor.
81. de Lara Capurro M, Coleman J, Beerntsen BT, Myles KM, Olson KE, Rocha E, et al. Virus-Expressed, Recombinant Single-Chain Antibody Blocks Sporozoite Infection of Salivary Glands in Plasmodium gallinaceum-Infected Aedes aegypti. Am J Trop Med Hyg (2000) 62(4):427–33. doi: 10.4269/ajtmh.2000.62.427
82. Ghosh AK, Ribolla PEM, Jacobs-Lorena M. Targeting Plasmodium Ligands on Mosquito Salivary Glands and Midgut With a Phage Display Peptide Library. Proc Natl Acad Sci (2001) 98(23):13278–81. doi: 10.1073/pnas.241491198
83. Corby-Harris V, Drexler A, Watkins de Jong L, Antonova Y, Pakpour N, Ziegler R, et al. Activation of Akt Signaling Reduces the Prevalence and Intensity of Malaria Parasite Infection and Lifespan in Anopheles Stephensi Mosquitoes. PloS Pathog (2010) 6(7):e1001003. doi: 10.1371/journal.ppat.1001003. Vernick KD, editor.
84. Grogan C, Bennett M, Moore S, Lampe D. Novel Asaia bogorensis Signal Sequences for Plasmodium Inhibition in Anopheles stephensi. Front Microbiol (2021) 12:633667/full. doi: 10.3389/fmicb.2021.633667/full
85. Buck M, Nilsson LKJ, Brunius C, Dabiré RK, Hopkins R, Terenius O. Bacterial Associations Reveal Spatial Population Dynamics in Anopheles gambiae Mosquitoes. Sci Rep (2016) 6(September 2015):1–9. doi: 10.1038/srep22806
86. Silva JBL, Magalhães Alves D, Bottino-Rojas V, Pereira TN, Sorgine MHF, Caragata EP, et al. Wolbachia and Dengue Virus Infection in the Mosquito Aedes Fluviatilis (Diptera: Culicidae). PloS One (2017) 12(7):e0181678. doi: 10.1371/journal.pone.0181678. Bourtzis K, editor.
87. Walker T, Johnson PH, Moreira LA, Iturbe-Ormaetxe I, Frentiu FD, McMeniman CJ, et al. The Wmel Wolbachia Strain Blocks Dengue and Invades Caged Aedes Aegypti Populations. Nature (2011) 476(7361):450–3. doi: 10.1038/nature10355
88. Thomason LC, Sawitzke JA, Li X, Costantino N, Court DL. Recombineering: Genetic Engineering in Bacteria Using Homologous Recombination. Curr Protoc Mol Biol (2014) 2014:1–39. doi: 10.1002/0471142727.mb0116s106
89. Mwangi J, Hao X, Lai R, Zhang ZY. Antimicrobial Peptides: New Hope in the War Against Multidrug Resistance. Zool Res (2019) 40(6):488–505. doi: 10.24272/j.issn.2095-8137.2019.062
90. Joo HS, Fu CI, Otto M. Bacterial Strategies of Resistance to Antimicrobial Peptides. Philos Trans R Soc B Biol Sci (2016) 371(1695):20150292. doi: 10.1098/rstb.2015.0292
91. Nazni WA, Hoffmann AA, NoorAfizah A, Cheong YL, Mancini MV, Golding N, et al. Establishment of Wolbachia Strain Walbb in Malaysian Populations of Aedes aegypti for Dengue Control. Curr Biol (2019) 29(24):4241–4248.e5. doi: 10.1016/j.cub.2019.11.007
92. Adolfi A, Gantz VM, Jasinskiene N, Lee H-F, Hwang K, Terradas G, et al. Efficient Population Modification Gene-Drive Rescue System in the Malaria Mosquito Anopheles stephensi. Nat Commun (2020) 11(1):5553. doi: 10.1038/s41467-020-19426-0
93. Okanda FM, Dao A, Njiru BN, Arija J, Akelo HA, Touré Y, et al. Behavioural Determinants of Gene Flow in Malaria Vector Populations : Anopheles Gambiae Males Select Large Females as Mates. Malaria J (2002) 7:1–7. doi: 10.1186/1475-2875-1-1
94. Nignan C, Poda BS, Sawadogo SP, Maïga H, Dabiré KR, Gnankine O, et al. Local Adaptation and Colonization are Potential Factors Affecting Sexual Competitiveness and Mating Choice in Anopheles Coluzzii Populations. Sci Rep (2022) 12:1–12. doi: 10.1038/s41598-021-04704-8
95. Pike A, Dong Y, Dizaji NB, Gacita A, Mongodin EF, Dimopoulos G. To Spread in a Population. Science (2017) 357(6358):1396–9. doi: 10.1126/science.aak9691
96. Lovett B, Bilgo E, Millogo SA, Ouattarra AK, Sare I, Gnambani EJ, et al. Transgenic Metarhizium Rapidly Kills Mosquitoes in a Malaria-Endemic Region of Burkina Faso. Science (2019) 364(6443):894–7. doi: 10.1126/science.aaw8737
97. Aboagye-Antwi F, Tripet F. Effects of Larval Growth Condition and Water Availability on Desiccation Resistance and Its Physiological Basis in Adult Anopheles gambiae Sensu Stricto. Malar J (2010) 9(1):225. doi: 10.1186/1475-2875-9-225
98. Romoli O, Gendrin M. The Tripartite Interactions Between the Mosquito, Its Microbiota and Plasmodium. Parasit Vectors (2018) 11(1):200. doi: 10.1186/s13071-018-2784-x
99. Tripet F, Aboagye-Antwi F, Hurd H. Ecological Immunology of Mosquito–Malaria Interactions. Trends Parasitol (2008) 24(5):219–27. doi: 10.1016/j.pt.2008.02.008
100. Bilgo E, Lovett B, Bayili K, Millogo AS, Saré I, Dabiré RK, et al. Transgenic Metarhizium pingshaense Synergistically Ameliorates Pyrethroid-Resistance in Wild-Caught, Malaria-Vector Mosquitoes. PloS One (2018) 13(9):e0203529. doi: 10.1371/journal.pone.0203529. Favia G, editor.
101. Cisnetto V, Barlow J. The Development of Complex and Controversial Innovations. Genetically Modified Mosquitoes for Malaria Eradication. Res Policy (2020) 49(3):103917. doi: 10.1016/j.respol.2019.103917
102. Thizy D, Emerson C, Gibbs J, Hartley S, Kapiriri L, Lavery J, et al. Guidance on Stakeholder Engagement Practices to Inform the Development of Area-Wide Vector Control Methods. PloS Negl Trop Dis (2019) 13(4):e0007286. doi: 10.1371/journal.pntd.0007286. Matovu E, editor.
103. Hartley S, Smith RDJ, Kokotovich A, Opesen C, Habtewold T, Ledingham K, et al. Ugandan Stakeholder Hopes and Concerns About Gene Drive Mosquitoes for Malaria Control: New Directions for Gene Drive Risk Governance. Malar J (2021) 20:1–13. doi: 10.1186/s12936-021-03682-6
Keywords: mosquitoes, bacteria, Plasmodium, paratransgenesis strategy, malaria transmission
Citation: Fofana A, Yerbanga RS, Bilgo E, Ouedraogo GA, Gendrin M and Ouedraogo J-B (2022) The Strategy of Paratransgenesis for the Control of Malaria Transmission. Front. Trop. Dis 3:867104. doi: 10.3389/fitd.2022.867104
Received: 31 January 2022; Accepted: 12 April 2022;
Published: 29 June 2022.
Edited by:
Marta Ferreira Maia, University of Oxford, United KingdomReviewed by:
Yara M. Traub-Csekö, Oswaldo Cruz Foundation (Fiocruz), BrazilPaolo Gabrieli, University of Milan, Italy
Copyright © 2022 Fofana, Yerbanga, Bilgo, Ouedraogo, Gendrin and Ouedraogo. This is an open-access article distributed under the terms of the Creative Commons Attribution License (CC BY). The use, distribution or reproduction in other forums is permitted, provided the original author(s) and the copyright owner(s) are credited and that the original publication in this journal is cited, in accordance with accepted academic practice. No use, distribution or reproduction is permitted which does not comply with these terms.
*Correspondence: Aminata Fofana, YW1pbmF0YWZvZmFuYTkyMEBnbWFpbC5jb20=
†These authors have contributed equally to this work