- 1National Research Center for Protozoan Diseases, Obihiro University of Agriculture and Veterinary Medicine, Hokkaido, Japan
- 2Department of Tropical Medicine, The Jikei University School of Medicine, Tokyo, Japan
- 3Center for Medical Entomology, The Jikei University School of Medicine, Tokyo, Japan
- 4Division of Virology, Department of Microbiology and Immunology, Institute of Medical Science, University of Tokyo, Tokyo, Japan
- 5Division of Medical Zoology, Department of Infection and Immunity, Jichi Medical University, Tochigi, Japan
Live microbe vaccines are designed to elicit strong cellular and antibody responses without developing the symptoms of the disease, and these are effective in preventing infectious diseases. A flying vaccinator (also known as a flying syringe) is a conceptual, genetically engineered hematophagous insect that is used to deliver vaccines such as an antigen from a parasite produced in mosquito saliva; bites from such insects may elicit antibody production by immunizing the host with an antigen through blood-feeding. In addition to a simple vaccine antigen, a flying vaccinator may potentially load a live attenuated microbe with an appropriate mechanism for sustaining its constitutive proliferation in the insect. In this study, a recombinant vesicular stomatitis virus (VSV) lacking the glycoprotein gene (VSV-G) was used to produce replication-restricted VSV (rrVSV) containing GFP. Transgenic Anopheles stephensi mosquitoes, in which the salivary glands expressed a VSV-G gene driven by an aapp salivary gland-specific promoter, were generated and injected intraperitoneally with rrVSV. The injected rrVSV entered the cells of the salivary gland and stimulated endogenous production of progeny rrVSV particles, as seen in rrVSV-infected Drosophila melanogaster expressing VSV-G. These data suggested the possibility of developing a valuable tool for delivering genetically attenuated virus vaccines via mosquito saliva, although efficient replication-restricted virus production is required.
Introduction
Using genetic transformation to hematophagous insects such as mosquitoes have advanced substantially in the past two decades. The establishment of broadly applicable genetic transformation systems has expanded the opportunity to understand gene functions in diverse insect species (1). It has recently become possible to introduce foreign genes into medically important insect vectors, including the major human malaria vectors (Anopheles mosquitoes) and dengue/zika vectors (Aedes mosquitoes) (2–6). Stable germline transformation of blood-sucking mosquitoes is becoming increasingly important for applications, including controlling pathogen transmission and managing insect populations in the field (7). Using tissue-specific expression systems, anti-pathogen gene products are introduced to be expressed. These agents can affect the pathogen’s viability, proliferation, or differentiation in the gut, salivary glands, or hemolymph of mosquitoes.
The first generation of mosquitoes refractory to disease transmission was reported with transgenic mosquitoes expressing a peptide, SM1, which binds to the midgut and salivary glands of Anopheles stephensi. The number of oocysts and sporozoites of Plasmodium berghei, a rodent malaria parasite species, was reduced in SM1-expressing female mosquitoes (8). Following this example, transgenic Aedes aegypti mosquitoes highly resistant to dengue-2 virus (DENV2) were produced. These transgenic mosquitoes enable a DENV2-specific inverted-repeat RNA to be expressed specifically in the midgut after blood-sucking. The artificially produced RNA partially formed a double-stranded RNA with the DENV2 genome, initiating RNA interference to reduce viral load (9). Genetic transformation has even granted mosquitoes multiple, different features that are not possessed naturally, such as boosted innate immunity (10), defects in flying capability (11), male sterility (12), hyper-hemolysis of sucked blood (13), and harboring an anti-pathogen antibody (14).
A flying vaccinator, alternatively known as a flying syringe, is a genetically engineered blood-feeding insect to deliver vaccine agents by replacing its natural populations. This strategy was first reported for a malaria mosquito strain carrying a vaccine antigen against leishmaniasis and malaria (15–17). The concept of a flying vaccinator relies on an indispensable feature of hematophagy; namely, it involves injecting saliva that inhibits host responses such as blood-clotting and vascular constriction to ensure smooth blood-sucking (18). Mosquito saliva contains over 20 proteins that are injected, such as an anticoagulant protein supporting the hematophagous process, and it has been proposed that by genetic transformation, exogenous proteins could be transferred from mosquitoes to an animal through blood-sucking when these introduced proteins are expressed in the salivary glands. For example, a gene encoding an antigen protein that triggers an immune response in humans could be integrated into the mosquito genome, resulting in the addition of the foreign protein into the mixture of saliva proteins.
It has been reported previously in several studies that hematophagous mosquitoes are a tractable tool as a means to deliver protective vaccines. A genetically modified mosquito strain of An. stephensi secreting the SP15 antigen as a component of saliva was developed. SP15 is a candidate vaccine against leishmaniasis, a parasitic disease spread by sand flies that can cause skin sores and organ damage, and repeated bites by the mosquitoes induced the development of anti-SP15 antibodies in mice (15). A transgenic mosquito strain expressing a partial circumsporozoite protein (CSP) from a rodent malaria parasite (Plasmodium berghei) in its saliva was also developed to raise an antibody against CSP in mice by blood-feeding (19). In addition, an alternative concept of a flying vaccinator that drives a live-attenuated pathogen itself was examined (20). Instead of applying the genetic transformation to mosquitoes, genetically engineered human malaria parasites (Plasmodium falciparum) lacking two essential, pre-erythrocytic stage-expressed genes (P52 and P36) were produced to create a vaccine that protects against malaria infection. Direct injection of P52/P36-deficient sporozoites into the human body, which is normally carried into human blood vessels by mosquito bites, produced an antibody that was specific to the Plasmodium parasite (20), suggesting a possibility that a mosquito infected with these attenuated malaria parasites may be capable of vaccinating a human via blood-sucking.
In this study, we developed a transgenic mosquito capable of continuously producing a genetically attenuated, live virus, which was achieved by engineering a replication-restricted virus. The replication-restricted virus did not carry the genetic material to produce additional viral envelope proteins required for virus cell entry. Vesicular stomatitis virus (VSV) lacking an envelope glycoprotein (VSV-G) was employed as a model of a replication-restricted virus (referred to as rrVSV). A transgenic An. stephensi mosquito strain, in which the salivary glands expressed VSV-G protein, was developed to permit replication and production of rrVSV only in the salivary glands. Our findings here may expand the range of applications of flying vaccinators to delivering live attenuated agents.
Materials and Methods
Ethics Statement
This study was carried out according to the recommendations in the Guide for the Laboratory Animals of the Obihiro University of Agriculture and Veterinary Medicine. The protocol was approved by the Committee on the Animal Experiments of Obihiro University of Agriculture and Veterinary Medicine (Permit Number: 21–41 and 21–42). All experiments using mice were performed under anesthesia, and all efforts were made to minimize suffering following the Guidelines for Animal Experimentation of the Japanese Association for Laboratory Animal Science and the Fundamental Guidelines for Proper Conduct of Animal Experiment and Related Activities in Academic Research Institutions under the jurisdiction of the Ministry of Education, Culture, Sports, Science and Technology, Japan.
Insect Maintenance
D. melanogaster was raised on a standard fly medium at 25°C. w1118 flies were used as a wild-type line. The fly lines da-GAL4, actin-GAL4, and UAS-GFP (S65T) were obtained from the Bloomington Stock Center. The pxn-GAL4 line was provided from Micheal J. Galko. A wild-type strain of laboratory-reared An. stephensi was used throughout this study (a gift from Dr. Yasuo Chinzei). Adult females and males were kept together in mesh nylon cages (30 cm × 30 cm × 30 cm) in the following conditions: 27°C; 80% relative humidity; and 12 h:12 h = L:D photoperiod. These mosquitoes had constant access to a 10% sucrose solution on filter paper. Eggs laid on wet filter papers were transferred to water trays. Larvae were fed Koi food (Hikari; Kyorin Corporation). Four- to 10-day-old females were used in all experiments.
Transformation Vector
For the transformation of fruit fly, a cDNA fragment for the open reading frame of VSV-G [described in the previous report (21)] was inserted into a pUAST vector (pUAST-VSV-G). The mosquito transformation plasmid pBac[3xP3-EGFPafm] and the helper plasmid phsp-pBac were kindly provided by Dr. Marcelo Jacobs-Lorena (8). A 1683 bp fragment of the aapp promoter sequence (22), a 402 bp fragment of the antryp1 terminator sequence (23), and a 1536 bp fragment of the open reading frame of VSV-G were inserted into the pBac[3xP3-EGFPafm] to generate pBac-aapp-VSV-G for mosquito transformation (Figure 4B).
Germline Transformation of D. melanogaster
The ectopic expression of VSV-G glycoprotein in the fly was achieved using the GAL4/UAS system. The UAS‐VSV-G transgenic fly was generated by general P element‐mediated transformation, with a slight modification (24). Briefly, the pUAST-VSV-G plasmid was injected into w1118 embryos with a helper plasmid expressing transposase to produce transgenic flies. At least two independently transformed lines were obtained for the transgenic construct.
Germline Transformation of An. stephensi
Germline transformation of An. stephensi mosquito was performed as described previously with some modifications (8). Briefly, embryos were microinjected with an aliquot of the solution containing two plasmids, pBac-aapp-VSV-G (200 ng/µL) and phsp-pBac (50 ng/µL) using quartz capillaries. Each EGFP-expressing adult mosquito (G0) from the injected embryos was mated with 5–10 wild-type, opposite-sex mosquitoes. The progeny larvae (G1) were screened for expressing GFP fluorescence (Figure 4C). GFP-positive individuals were then crossed with a wild-type to establish the transgenic line.
Viral Infection to Insects
The rrVSV (VSVΔG*-G) is a recombinant VSV derived from a full-length cDNA clone of the VSV genome (Indiana serotype), in which the coding region of GFP replaced the coding region of the glycoprotein. The rrVSV was initially produced by reverse genetics as described previously (25). Either an adult fly (2 days old) or a female mosquito (7–8 days old) was injected with a 65 nL aliquot of the solution containing rrVSV (7.8 × 104 ffu/insect) using an IM-300 microinjector (Narishige) as reported previously (13). Injected insects were kept at 27°C with 80% relative humidity for mosquitoes and 29°C for fruit fly during each experiment.
Immunoblotting
The whole body of a fly was lysed in an SDS sample buffer. All samples were separated by 10% SDS-PAGE and subjected to immunoblotting described previously (24). The following antibodies were used for immunoblotting in this study: mouse anti-VSV-G monoclonal antibody (1:2000, Sigma), mouse anti-β-tubulin monoclonal antibody (1:1000, Chemicon), rabbit anti-GFP antibody (1:1000, MBL), anti-mouse IgG-HRP antibody (1:2000, Promega), and anti-rabbit IgG-HRP antibody (1:1000, Transduction). The signals were visualized using Immobilon Western Chemiluminescent HRP Substrate (Millipore). Immunoblotting with an anti-beta-tubulin antibody was used as a loading control.
Immunohistochemistry
Either tissues or cells were dissected from control or infected insects. Immunostaining of insect tissues or cells was carried out as reported previously with some modifications (26). The following antibodies were used for immunostaining: mouse anti-VSV-G monoclonal antibody (1:1000, Sigma), rabbit anti-GFP antibody (1:1000, MBL), goat anti-mouse IgG-Alexa 488 antibody (1:100, Invitrogen), goat anti-mouse IgG-Alexa 568 antibody (1:100, Invitrogen), and goat anti-rabbit IgG-Alexa 488 antibody (1:100, Invitrogen). TO-PRO-3 (Invitrogen) and propidium iodide (PI) are used for nuclear staining. All fluorescent signals were examined using a TCS SP5 confocal microscope (Leica).
Titration of rrVSV
Infected insects were dissected to isolate tissues at each indicated time point. The saliva of mosquitoes was collected as described previously (27). The whole body, salivary glands, carcass parts, and saliva were homogenized or mixed in 1× PBS. After centrifugation, the supernatants were filtered (0.45 µm) to collect the solution containing rrVSV particles. A diluted series of the virus solutions were inoculated into Drosophila S2 cells (1 × 105 cells/well). Infected S2 cells were maintained in standard conditions (27°C in Drosophila Schneider’s medium [GIBCO] supplemented with 10% fetal bovine serum, 5 mg/mL peptone, 100 U/mL penicillin, and 100 µL/mL streptomycin). After incubation for 12–16 hours, the number of GFP-expressing cells was counted using a fluorescence microscope.
Statistical Analysis
Student’s t-test was performed for analyzing the titer of rrVSV (ffu assay). All results are indicated in the appropriate figure legends. Results are represented as mean ± S.D.
Results
Replication-Restricted Vesicular Stomatitis Virus System
To develop a flying vaccinator capable of infecting a bitten host with a live attenuated virus, we adopted a replication-restricted virus to secrete an antigen in a transgenic hematophagous insect. VSV, a prototypic enveloped virus that has been used frequently to investigate virus entry, replication, and assembly, exhibits broad host range and robust replication properties in a wide variety of mammalian and insect cells, including mosquitoes (28). A recombinant rrVSV, which contains the green fluorescent protein (GFP) gene instead of a receptor-binding glycoprotein gene (VSV-G), which is indispensable for viral entry into cells, can bud from host cells only as “disabled” virus particles lacking VSV-G (Figure 1). When a glycoprotein or glycoprotein complex from a heterologous virus is expressed transiently in host cells, rrVSV carrying the heterologous glycoprotein is released with high efficiency (21, 25, 29, 30), which has been used as a vaccine vector because of its replication competency (31–33). rrVSV produced from cells expressing VSV-G appears to be a proper virus particle except for the lack of the VSV-G gene (Figure 1). VSV-G-incorporated rrVSV is a single-round infectious particle capable of invading a naïve cell only once. Thus, in this study, VSV-G-expressing hematophagous insects are expected to be a scaffold for the replication of rrVSV, which are unable to produce their glycoprotein, and to be a live syringe for a single round of rrVSV infection into a host animal.
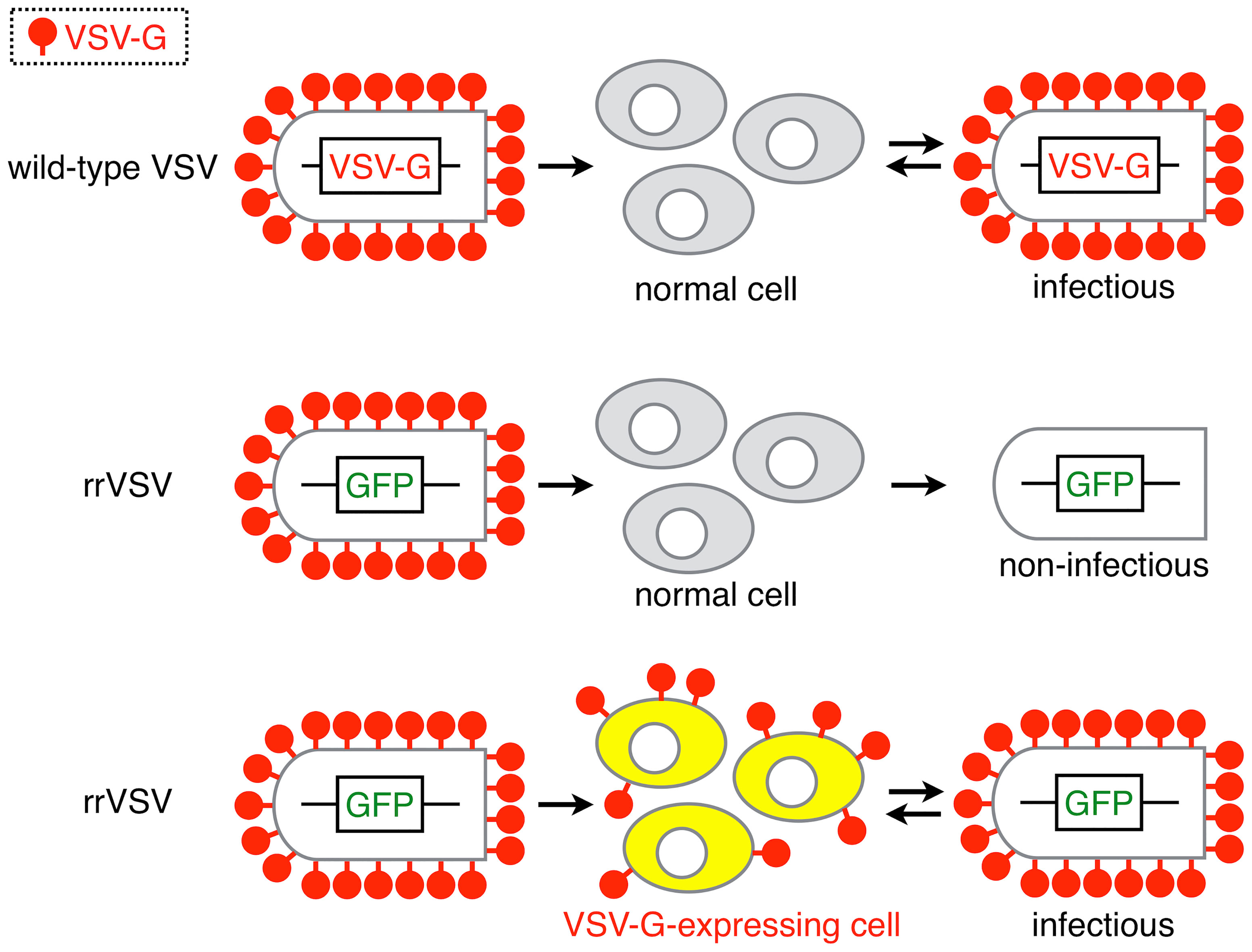
Figure 1 Replication-restricted vesicular stomatitis virus. The restriction of VSV replication was achieved using the following procedure. A wild-type VSV particle, a prototypic enveloped virus with a receptor-binding glycoprotein gene (VSV-G) that encodes an essential protein for viral entry into cells, infects a host cell and shows complete viral properties such as entry, replication, and assembly to produce fully infectious virus particles. A virus particle of the replication-restricted VSV (rrVSV), in which the GFP gene replaces the VSV-G gene, infects host cells. When VSV-G is artificially expressed in host cells, newly generated viral particles incorporate the glycoprotein into the virion, and the resulting progenies are identical to the original rrVSV. The rrVSV, however, cannot produce any progeny viruses because of the lack of VSV-G; the viral particles lose their ability to enter another cell.
Propagation of rrVSV in the Fruit Fly
To examine whether insects harboring VSV-G can drive rrVSV replication inside their body, we first introduced the fruit fly (D. melanogaster), a non-blood sucking insect that has frequently been used for transgenesis (Figure 2A). A fruit fly injected with fully infectious wild-type VSV directly into the abdomen continued to carry more virus particles for 10 days than virus particles initially administered, indicating that fruit fly may possess cellular components sufficient to support VSV replication (Figure 2B). A transgenic fly strain to overexpress VSV-G was established (UAS-VSV-G), and expression of the glycoprotein in combination with a fly strain expressing GAL4 in whole bodies (da-GAL4) or hemocytes (insect blood cells) (pxn-GAL4) was confirmed (Figures 2C, D). VSV-G-expressing adult transgenic flies were subjected to direct injection of rrVSV into the abdomen, and dense accumulation patches of fluorescent spots of GFP were observed in various parts of the body at 5 days post-infection (dpi), whereas no visible signals were detected in wild-type flies (Figure 3A, a, b). The clustered GFP-positive cells seen in VSV-G transgenic flies suggested that a number of newly generated rrVSV reentered into neighboring cells iteratively to accumulate GFP proteins (Figure 3A, c-f). According to these findings, increased rrVSV burden was observed in VSV-G transgenic flies, whereas the original rrVSV particles that were infected into wild-type flies rapidly disappeared at least at 2 dpi (Figures 3B, C), indicating that the rrVSV system worked in vivo and could apply to other insect species.
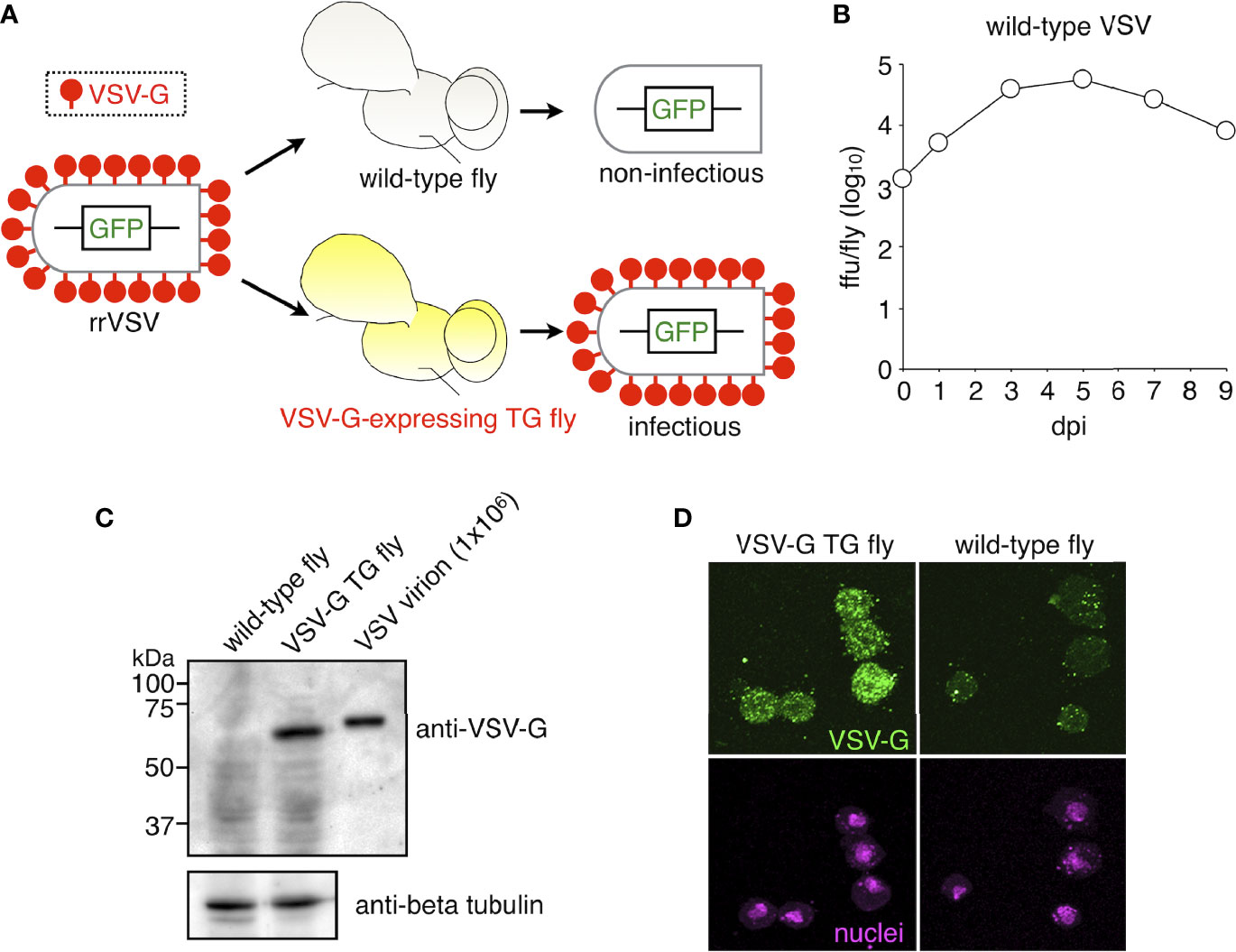
Figure 2 Generation of transgenic fruit flies expressing VSV-G. (A) Scheme for the production of rrVSV in transgenic flies, which can elicit the expression of VSV-G glycoprotein using the GAL4/UAS system. (B) The viral burden of intact VSV in wild-type fruit flies. Five flies were injected with wild-type VSV (7.8 × 104 ffu/fly) and homogenized to measure infectious viral particles (ffu) at each time point (days post-infection [dpi]). (C) Expression of VSV-G in a transgenic fruit fly. UAS-VSV-G flies were crossed to driver da-GAL4 flies, and the progeny obtained (VSV-G TG flies (da>VSV-G)) were homogenized to examine the expression of VSV-G protein by immunoblotting. Wild-type flies (w1118) were used as negative control, intact VSV virion (1 × 106 ffu) as a positive control, and an anti-beta tubulin antibody to detect beta-tubulin as the loading control. (D) VSV-G expression was visualized by immunohistochemistry using an anti-VSV-G antibody with hemocytes (blood cells) from VSV-G transgenic flies. UAS-VSV-G flies were crossed with a driver flies pxn-GAL4, expressing a target protein in hemocytes. The progeny VSV-G TG flies (pxn>VSV-G) were examined for VSV-G expression compared with wild-type flies (w1118). Green, VSV-G (anti-VSV-G); magenta, nuclei (PI). See Supplementary Table 1 for data of experiment, exact sample sizes, and p values.
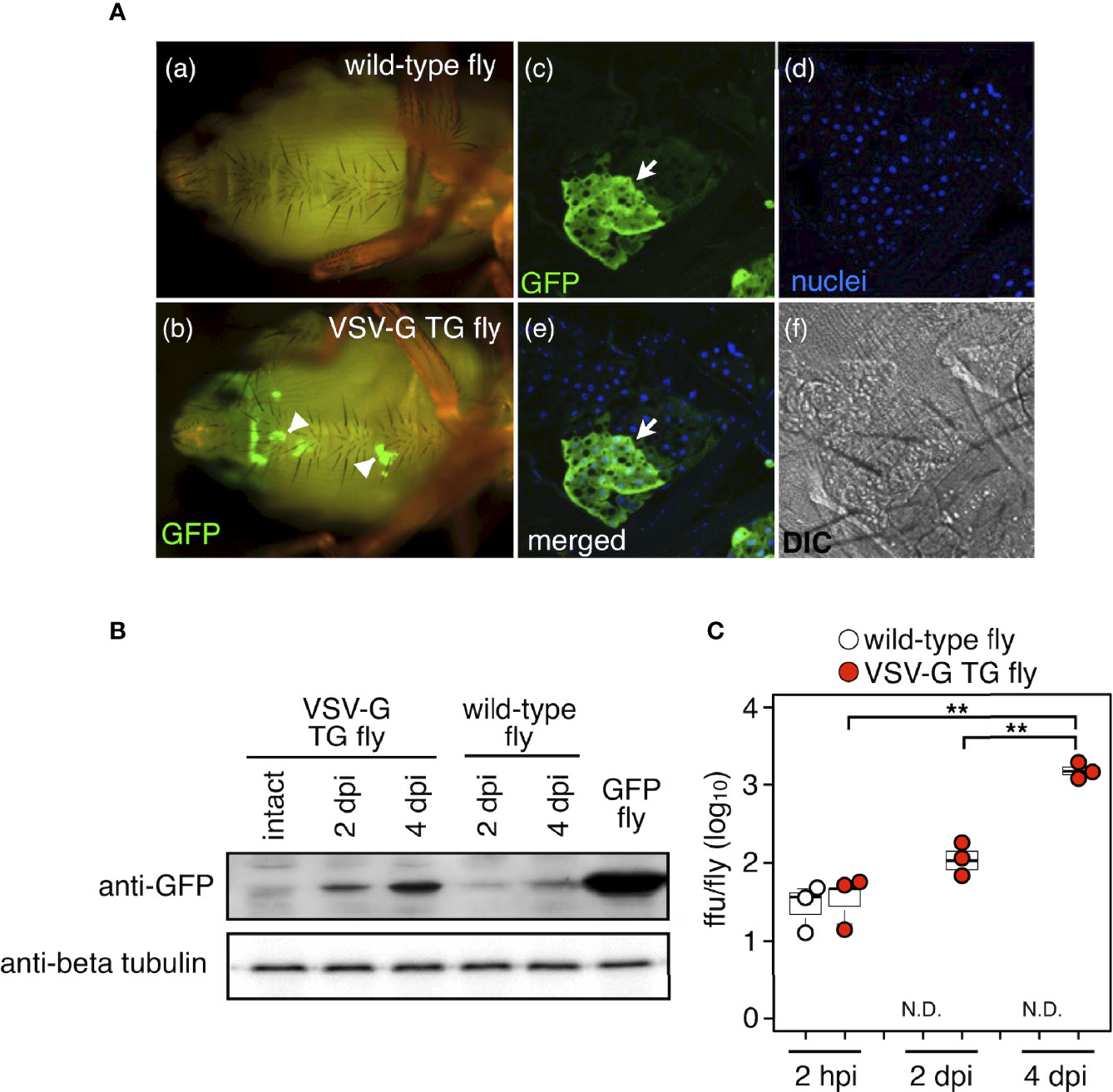
Figure 3 Propagation of replication-restricted VSV in fruit flies. (A) The occurrence of rrVSV-harboring cells in VSV-G transgenic flies. rrVSV (7.8 × 104 ffu/fly) was injected into VSV-G TG flies (da>VSV-G) and wild-type flies (w1118). Several dense accumulation patches of GFP (arrowhead) were detected in the abdomens of VSV-G TG flies at 5 days post-infection (dpi) by immunohistochemistry using an anti-GFP antibody. High magnification images of a cluster of GFP-positive cells (arrow) are shown in (c-f). Green, GFP; blue, nuclei (TO-PRO-3). (B) VSV-G TG flies (da>VSV-G) and wild-type flies (w1118) were injected with rrVSV as in (A) and homogenized at each time point to examine GFP protein levels. GFP flies (act>GFP) were used as a positive control of immunoblotting and an anti-beta tubulin antibody to detect beta-tubulin as the loading control. (C) Viral loads in the whole body of VSV-G transgenic fruit flies. The rrVSV-injected flies (three flies/group) were homogenized at 2 hours post-infection, 2 dpi, and 4 dpi. The average number of viral particles (ffu) was calculated from 3 groups at each time point using a fluorescence-forming assay with cultured insect cells. **p < 0.01, N.D., not detected. See Supplementary Table 1 for data of experiment, exact sample sizes, and p values.
Generation of Transgenic Mosquitoes With the Salivary Gland-Specific Expression of VSV-G
We then performed germline transformation of mosquito embryos to elicit stable expression of VSV-G. A cDNA fragment (termed aapp-VSV-G), consisting of the VSV-G envelope protein-coding gene driven by the salivary gland-specific aapp promoter (22), was inserted into a piggyBac transposon vector, and the plasmid fabricated was used for transforming the germline of the mosquito An. stephensi (Figures 4A, B). In addition to the availability of methods inducing transgenesis, an Anopheline species was employed in this study because these mosquitoes are normally unable to transmit arbovirus and seemed to be more appropriate to examine the concept of viral, attenuated vaccinating via mosquito blood-sucking. Of 2635 embryos injected, 67 G0 transient transformants (with partial GFP fluorescence observed in tissues) were obtained. Crossing the individual G0 transformant with wild-type mosquitoes, we established 8 transgenic lines (aapp-VSV-G transgenic mosquito) with stable GFP expression (Figure 4C). Immunofluorescence assays on salivary glands identified that one of the transgenic lines expressed VSV-G as a protein in both lateral lobes and the medial lobe (Figure 4D), and this line was then used for further experiments. These mosquito lines transformed with the aapp-VSV-G construct seemed to have similar fitness parameters (such as survival, fecundity, and fertility) as those of wild-type mosquitoes because these lines were maintained well in an insectarium.
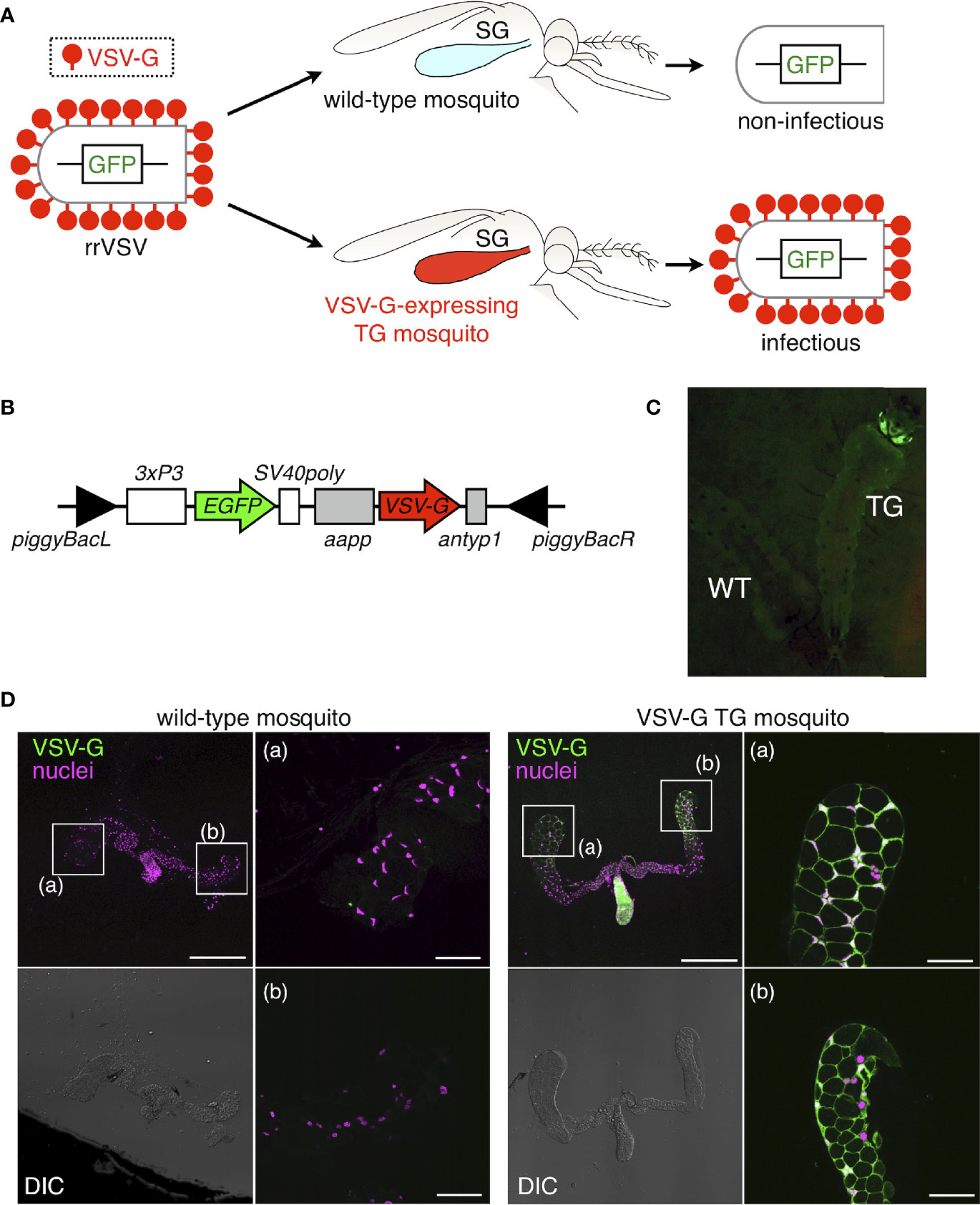
Figure 4 Generation of transgenic mosquitoes expressing VSV-G in salivary glands. (A) Scheme of producing rrVSV in genetically modified mosquitoes with forced expression of VSV-G glycoprotein only in salivary glands. (B) Schematic diagram of the piggyBac-based transformation vector (pBac-aapp-VSV-G) integrated into the An. stephensi germline. The EGFP gene cassette consists of the D. melanogaster Pax6 eye-specific promoter (3xP3), fluorescent selectable marker (EGFP, green), and SV40 terminator sequence (SV40poly). The VSV-G gene cassette consists of the An. stephensi aapp promoter (aapp), VSV-G gene (VSV-G, red), and An. stephensi trypsin terminator sequence (antyp1). The left (piggyBacL) and right (piggyBacR) arms of the piggyBac transposon are indicated by black triangles. (C) Ectopic expression of 3xP3-EGFP selectable marker in compound eyes of aapp-VSV-G transgenic mosquitoes (TG, right) compared with wild-type (WT, left). (D) VSV-G expression was visualized by immunohistochemistry using an anti-VSV-G antibody with salivary glands of aapp-VSV-G transgenic mosquitoes. High magnification images of lateral lobes are presented in (a, b). Scale bar=50 µm. Green, VSV-G (anti-VSV-G); magenta, nuclei (PI).
In Vivo Persistence of rrVSV in Aapp-VSV-G Transgenic Mosquitoes
To investigate whether forced expression of VSV-G in mosquitoes could enable rrVSV to replicate autonomously, we performed infection of rrVSV in a female, aapp-VSV-G transgenic mosquitoes by direct injection of rrVSV particles into the mosquito abdomen, in which hemocoel constantly circulates. Accumulated GFP fluorescence was observed in cells of the salivary gland of rrVSV-infected aapp-VSV-G mosquitoes (Figure 5A). The naïve, non-infected transgenic mosquitoes only showed expression of marker GFP in a peripheral part of the salivary gland, presumably because of leaky activity of the 3×P3 promoter (Figure 5A). The detectable amount of GFP fluorescence that originated from the gene-cassette being carried in rrVSV suggested that virus re-entry may occur in cells expressing VSV-G, as observed in VSV-G transgenic Drosophila (Figure 3A). Because no structural changes at both the tissue and cellular levels were observed in rrVSV-infected salivary glands (Figure 5A), massive replication of rrVSV may not cause any damage, such as apoptosis, in these cells. To examine whether the aapp-VSV-G transgenic mosquito could produce progeny rrVSV that were infective, we dissected mosquitoes to detect viral particles in salivary glands and carcasses (other parts of the mosquito body except for the salivary gland) (Figure 5B). At 3 days after injection of rrVSV into mosquitoes, wild-type salivary glands contained a small amount of rrVSV [73 ± 18.75 ffu (focus forming unit)].
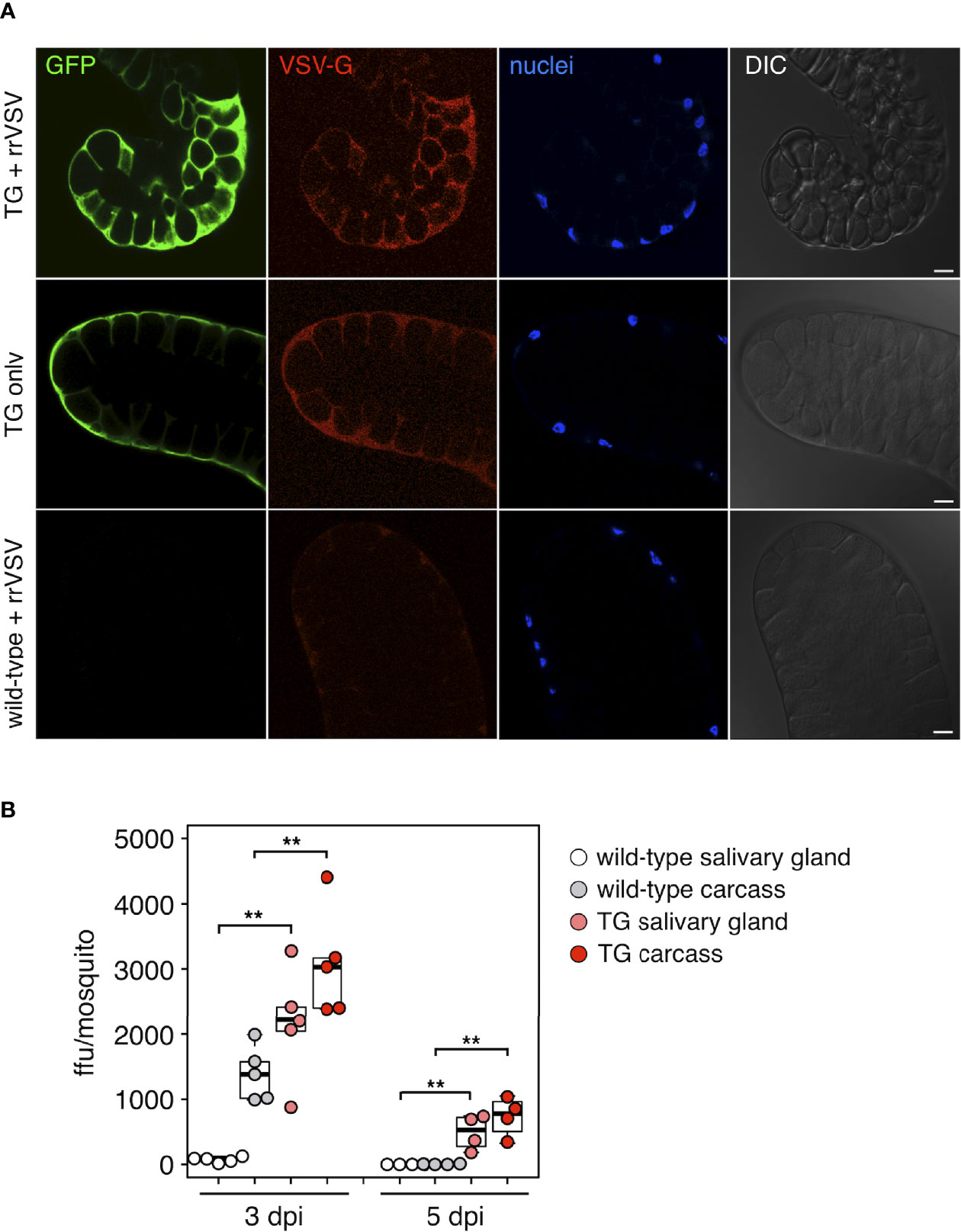
Figure 5 In vivo replication of rrVSV in transgenic mosquitoes expressing VSV-G. (A) Identification of rrVSV in salivary glands of aapp-VSV-G transgenic mosquitoes. Each mosquito was injected with 7.8 × 104 ffu of rrVSV. rrVSV was detected at 5 days after injection using an immunofluorescence assay with an anti-GFP antibody. GFP-positive cells (green) indicate rrVSV-infected salivary gland cells expressing VSV-G. Scale bar=20 µm. Green, GFP; red, VSV-G (anti-VSV-G); blue, nuclei (TO-PRO-3). (B) Viral loads in the salivary gland and the carcass of aapp-VSV-G transgenic mosquitoes. The rrVSV-injected mosquitoes (five mosquitoes/group) shown in (A) were dissected at 3 days and 5 days post-infection (dpi). The average number of viral particles (ffu) was calculated from 3-4 groups at each time point using a fluorescence-forming assay with cultured insect cells. **p < 0.01, N.D., not detected. See Supplementary Table 1 for data of experiment, exact sample sizes, and p values.
On the other hand, salivary glands from aapp-VSV-G mosquitoes were associated with a 29.5-fold increase in virus titer (2156 ± 387.28 ffu; p<0.01). The carcasses of transgenic mosquitoes also showed more rrVSV (3078 ± 368.21 ffu) than wild-type mosquitoes (1391 ± 186.4 ffu; p<0.01), probably because a part of the newly produced rrVSV in salivary glands could be released into the hemocoel and circulate in the body cavity. In wild-type mosquitoes, the injected rrVSV almost entirely disappeared at 5 dpi, whereas detectable amounts of rrVSV were still observed in both salivary glands (497.5 ± 134 ffu) and the carcass (733.75 ± 155.3 ffu) of aapp-VSV-G mosquitoes (Figure 5B). Finally, we attempted to detect rrVSV in mosquito saliva, collected at 3 and 5 dpi as excretion from the proboscis (mouthpart) of infected aapp-VSV-G mosquitoes. No infectious virus particles were observed in the saliva secreted from transgenic salivary glands, indicating that the low efficiency of rrVSV production could be improved for acquiring immunogenicity of the vaccine in mosquito-bitten hosts.
Discussion
Arboviruses transmitted by blood-sucking bugs have been the cause of a range of devastating diseases in recent decades, such as re-emerged West Nile, chikungunya, dengue, and zika, which generate public severe health emergencies. No vaccines targeting these pathogens are available yet, mainly because of different subtypes and strain variations of these viruses (34). In this study, a new platform for producing a live attenuated virus vaccine was developed in a blood-sucking insect species, Anopheles mosquitoes. Successive propagation of attenuated VSV (rrVSV), which lacked the glycoprotein gene in its viral genome, was confirmed in mosquitoes ectopically expressing the glycoprotein using a transgenic method. The present study is the first investigation where a genetically attenuated virus has been loaded into mosquitoes under the idea of a flying vaccinator.
Flying vaccinators can be distinguished conceptually into three types. The first is a mosquito inoculated with a live attenuated (or weakened) arbovirus vaccine strain, which is currently available in clinical practice, such as a less virulent virus of yellow fever (17D). The 17D strain of yellow fever virus is almost identical to intact virus except for mutations in its genome, and it can be injected into humans after virus propagation in the mosquito body and subsequently biting a human. Although the live attenuated virus is a promising tool to elicit a strong and protective immune response, this version of a flying vaccinator may not be a completely secure method because of a possible risk of virus reversion to a virulent strain during serial passage in the cells/tissues of mosquitoes (35). The second is a mosquito ectopically expressing an antigenic protein from the pathogen in its salivary glands to deliver the exogenous protein into the host via injected saliva. For this, a specific protein encoded by the pathogen genome and exposed as an antigen on the pathogen surface must be selected to provoke a response from the human immune system that can provide future protection against the target pathogen. Protein immunization often requires booster injections with adjuvants because of the poor immunogenicity of proteins and an inability to stimulate cellular immune responses (36). Indeed, a mosquito strain capable of injecting saliva with Plasmodium CSP as an antigen needed approximately 1,500 bites per mouse (a mouse was bitten by 100 transgenic mosquitoes, which was repeated 20 times in four months) to raise sufficient amounts of antibodies to disturb malaria parasite invasion into host hepatocytes (19). As reported in this study, the third approach is a mosquito harboring genetically engineered, live attenuated virus, in which a part of the genome or genes is artificially disrupted. The virus particles delivered via mosquito blood-sucking behave in the manner of single-round infectious agents, providing for the safety of recombinant proteins and the efficiency of live attenuated virus in vaccination. Although the genetically attenuated viruses are generally disabled to replicate in normal cells, this limitation can be overcome through compensating by introducing the viral gene component into the mosquito genome using transgenic methods, as shown practically in this report.
Remarkable advances in making genetically engineered viruses have broadened the opportunities to develop potent vaccines against pathogenic arboviruses. The ultimate form of a genetically attenuated virus lacking any viral genomic material, called a virus-like particle (VLP), has only repetitive nanostructures that mimic the original virus structures, further increasing safety (36). Trials in the production of genetically attenuated viruses, including VLPs, have been carried out for members of major arbovirus families such as the Flaviviridae (dengue, West Nile, Japanese encephalitis) and Togaviridae (chikungunya) (37). These compromised viruses can still trigger both humoral and cellular immune responses in hosts, and recent findings raise an intriguing possibility that a flying vaccinator may enhance the immunogenicity of the attenuated virus by using mosquito saliva. Hematophagous arthropod saliva facilitates the transmission of pathogens, including arboviruses (34). A set of saliva proteins secreted from the salivary glands of the Aedes mosquito enhanced the viral replication and pathogenesis of flavivirus such as dengue and West Nile virus in infected hosts (38–40). Either salivary gland extract or the saliva itself of mosquito can locally inhibit eliciting the anti-viral Th1 immune response at the intradermal site of viral inoculation after a mosquito bite, allowing the virus to enter the host and replicate more efficiently (41–44). The blood-sucking of VSV-infected Aedes mosquito (Aedes toriseriatus) can induce antibodies specific to VSV in mice sera more efficiently than virus injection, suggesting that mosquito saliva stimulated the enhancement of VSV infection (45). Salivary gland homogenate dampened interferon-mediated anti-viral effects and stimulated virus propagation in vitro (46). Although the possibility of immuno-modulatory effects of mosquito saliva on increasing the immunogenicity of the genetically attenuated virus remains to be examined, it seems to be worth considering such an “adjuvant” function of flying vaccinators when loaded with live pathogenic materials.
It could be argued why the titer of rrVSV was not so high in the transgenic Anopheles mosquitoes as shown in this study, despite the observation that rrVSV replicated to produce significant numbers of viral particles in both salivary glands and the carcass (Figure 5B). Among insect species in the field, VSV is frequently identified in Aedes mosquitoes, and VSV increases in Ae. aegypti by 3,000–30,000 times in 1 week after intrathoracic injection of the virus (47). Salivary glands of Ae. aegypti continue to produce substantial amounts of VSV even at 9 days post-infection, which results in virus transmission into mice (28). It is well known that Aedes species are more efficient transmitters of arboviruses than Anopheles mosquitos, based on previous observations such as the viral load of dengue virus being significantly lower in Anopheles albimanus than in Ae. aegypti (48). It was also suggested that arbovirus demands cellular processes, particularly proper post-translational modifications, which are essential in correct arboviral glycoprotein folding to yield high-quality virus particles (36). Given the possibility that most Anopheles species may be poor at multiplying such viruses, arboviral vector mosquitoes such as Aedes albopictus and Culex tritaeniorhynchus, which are responsible for the spread of viruses causing dengue, zika, and encephalitides, could be considered as appropriate platforms of flying vaccinators and be utilized in further experiments.
In conclusion, a novel class of flying vaccinators for the genetically attenuated virus was developed experimentally in this study. Further examination regarding the efficient production of the replication-restricted virus remains to be performed. Confirming and improving the possible ability of the transgenic Anopheles mosquitoes to inject an adequate amount of rrVSV into vertebrate hosts to raise immune response is another challenge. At least, this method may make it possible to perform intensive studies using mosquitoes carrying human hazardous arbovirus, even in counties where highly secure containment systems are mandatory. Our findings in this study could also be applicable to Aedes and Culex mosquitoes, which are significant vectors for other viruses such as dengue, zika, West Nile fever, and Japanese encephalitis.
Data Availability Statement
The original contributions presented in the study are included in the article/Supplementary Material. Further inquiries can be directed to the corresponding author.
Ethics Statement
The animal study was reviewed and approved by the Committee on the Animal Experiments of Obihiro University of Agriculture and Veterinary Medicine.
Author Contributions
NS, CK, and HK designed the experiments. NS and CK carried out the experiments and analyzed the data. KT, MS, and YK contributed to the experiments using replication-restricted viruses. HA, DY, SY, and HM contributed to making the transgenic mosquito strain. HA and SF contributed to data analysis and discussions. ES analyzed the data and made the figures. HK and NS wrote the manuscript. HK supervised the study. All authors contributed to the article and approved the submitted version.
Funding
This work was supported by JSPS KAKENHI Grant Number 20688013 (HK), the Funding Program for Next Generation World-Leading Researchers (NEXT Program) (LS002) (HK), and the International Collaborative Research Program for Tackling the NTDs Challenges in African countries from Japan Agency for Medical Research and Development, AMED (JP17jm0510002h0003) (HK). The funders had no role in study design, data collection, analysis, decision to publish, or manuscript preparation.
Conflict of Interest
The authors declare that the research was conducted in the absence of any commercial or financial relationships that could be construed as a potential conflict of interest.
Publisher’s Note
All claims expressed in this article are solely those of the authors and do not necessarily represent those of their affiliated organizations, or those of the publisher, the editors and the reviewers. Any product that may be evaluated in this article, or claim that may be made by its manufacturer, is not guaranteed or endorsed by the publisher.
Acknowledgments
We thank all members of the Kanuka laboratory for their enthusiastic participation. We are grateful to Y. Furukawa for assistance with mosquito rearing, M. Ote, C. Sakuma, T. Sakurai, and E. Saiki for critical reading of the manuscript, and M. Jacobs-Lorena for supplying the plasmid.
Supplementary Material
The Supplementary Material for this article can be found online at: https://www.frontiersin.org/articles/10.3389/fitd.2022.850111/full#supplementary-material
Supplementary Table 1 | Statistical reporting for results related to Figures 2, 3 and 5. Detailed results from all figures are provided.
References
1. Wimmer EA. Applications of Insect Transgenesis. Nat Rev Genet (2003) 4:225–32. doi: 10.1038/nrg1021
2. Catteruccia F, Nolan T, Loukeris TG, Blass C, Savakis C, Kafatos FC, et al. Stable Germline Transformation of the Malaria Mosquito Anopheles Stephensi. Nature (2000) 405:959–62. doi: 10.1038/35016096
3. Grossman GL, Rafferty CS, Clayton JR, Stevens TK, Mukabayire O, Benedict MQ. Germline Transformation of the Malaria Vector, Anopheles Gambiae, With the Piggybac Transposable Element. Insect Mol Biol (2001) 10:597–604. doi: 10.1046/j.0962-1075.2001.00299.x
4. Nolan T, Bower TM, Brown AE, Crisanti A, Catteruccia F. Piggybac-Mediated Germline Transformation of the Malaria Mosquito Anopheles Stephensi Using the Red Fluorescent Protein dsRED as a Selectable Marker. J Biol Chem (2002) 277:8759–62. doi: 10.1074/jbc.C100766200
5. Perera OP, Harrell RA II, Handler AM. Germ-Line Transformation of the South American Malaria Vector, Anopheles Albimanus, With a Piggybac/EGFP Transposon Vector is Routine and Highly Efficient. Insect Mol Biol (2002) 11:291–7. doi: 10.1046/j.1365-2583.2002.00336.x
6. Lobo NF, Hua-Van A, Li X, Nolen BM, Fraser MJ Jr. Germ Line Transformation of the Yellow Fever Mosquito, Aedes Aegypti, Mediated by Transpositional Insertion of a Piggybac Vector. Insect Mol Biol (2002) 11:133–9. doi: 10.1046/j.1365-2583.2002.00317.x
7. O’Brochta DA, Handler AM. Perspectives on the State of Insect Transgenics. Adv Exp Med Biol (2008) 627:1–18. doi: 10.1007/978-0-387-78225-6_1
8. Ito J, Ghosh A, Moreira LA, Wimmer EA, Jacobs-Lorena M. Transgenic Anopheline Mosquitoes Impaired in Transmission of a Malaria Parasite. Nature (2002) 417:452–5. doi: 10.1038/417452a
9. Franz AW, Sanchez-Vargas I, Adelman ZN, Blair CD, Beaty BJ, James AA, et al. Engineering RNA Interference-Based Resistance to Dengue Virus Type 2 in Genetically Modified Aedes Aegypti. Proc Natl Acad Sci USA (2006) 103:4198–203. doi: 10.1073/pnas.0600479103
10. Antonova Y, Alvarez KS, Kim YJ, Kokoza V, Raikhel A. The Role of NF-kappaB Factor REL2 in the Aedes Aegypti Immune Response. Insect Biochem Mol Biol (2009) 39:303–14. doi: 10.1016/j.ibmb.2009.01.007
11. Fu G, Lees RS, Nimmo D, Aw D, Jin L, Gray P, et al. Female-Specific Flightless Phenotype for Mosquito Control. Proc Natl Acad Sci USA (2010) 107:4550–4. doi: 10.1073/pnas.1000251107
12. Thomas DD, Donnelly CA, Wood RJ, Alphey LS. Insect Population Control Using a Dominant, Repressible, Lethal Genetic System. Science (2000) 287:2474–6. doi: 10.1126/science.287.5462.2474
13. Yoshida S, Shimada Y, Kondoh D, Kouzuma Y, Ghosh AK, Jacobs-Lorena M, et al. Hemolytic C-Type Lectin CEL-III From Sea Cucumber Expressed in Transgenic Mosquitoes Impairs Malaria Parasite Development. PloS Pathog (2007) 3:e192. doi: 10.1371/journal.ppat.0030192
14. Sumitani M, Kasashima K, Yamamoto DS, Yagi K, Yuda M, Matsuoka H, et al. Reduction of Malaria Transmission by Transgenic Mosquitoes Expressing an Antisporozoite Antibody in Their Salivary Glands. Insect Mol Biol (2013) 22:41–51. doi: 10.1111/j.1365-2583.2012.01168.x
15. Yamamoto DS, Nagumo H, Yoshida S. Flying Vaccinator; a Transgenic Mosquito Delivers a Leishmania Vaccine via Blood Feeding. Insect Mol Biol (2010) 19:391–8. doi: 10.1111/j.1365-2583.2010.01000.x
16. Matsuoka H, Ikezawa T, Hirai M. Production of a Transgenic Mosquito Expressing Circumsporozoite Protein, a Malarial Protein, in the Salivary Gland of Anopheles Stephensi (Diptera: Culicidae). Acta Med Okayama (2010) 64:233–41. doi: 10.18926/AMO/40131
17. Matsuoka H, Sano G, Hattori R, Tomita H, Yamamoto DS, Hirai M. One Injection of DsRed Followed by Bites From Transgenic Mosquitoes Producing DsRed in the Saliva Elicits a High Titer of Antibody in Mice. Trop Med Health (2012) 40:47–52. doi: 10.2149/tmh.2011-10
18. Crampton JM, Stowell SL, Karras M, Sinden RE. Model Systems to Evaluate the Use of Transgenic Haematophagous Insects to Deliver Protective Vaccines. Parassitologia (1999) 41:473–7.
19. Yamamoto DS, Sumitani M, Nagumo H, Yoshida S, Matsuoka H. Induction of Antisporozoite Antibodies by Biting of Transgenic Anopheles Stephensi Delivering Malarial Antigen via Blood Feeding. Insect Mol Biol (2012) 21:223–3. doi: 10.1111/j.1365-2583.2011.01128.x
20. Spring M, Murphy J, Nielsen R, Dowler M, Bennett JW, Zarling S, et al. First-In-Human Evaluation of Genetically Attenuated Plasmodium Falciparum Sporozoites Administered by Bite of Anopheles Mosquitoes to Adult Volunteers. Vaccine (2013) 31:4975–83. doi: 10.1016/j.vaccine.2013.08.007
21. Whitt MA. Generation of VSV Pseudotypes Using Recombinant ΔG-VSV for Studies on Virus Entry, Identification of Entry Inhibitors, and Immune Responses to Vaccines. J Virol Methods (2010) 169:365–74. doi: 10.1016/j.jviromet.2010.08.006
22. Yoshida S, Watanabe H. Robust Salivary Gland-Specific Transgene Expression in Anopheles Stephensi Mosquito. Insect Mol Biol (2006) 15:403–10. doi: 10.1111/j.1365-2583.2006.00645.x
23. Skavdis G, Sidén-Kiamos I, Müller HM, Crisanti A, Louis C. Conserved Function of Anopheles Gambiae Midgut-Specific Promoters in the Fruitfly. EMBO J (1996) 15:344–50. doi: 10.1002/j.1460-2075.1996.tb00364.x
24. Kanuka H, Kuranaga E, Takemoto K, Hiratou T, Okano H, Miura M. Drosophila Caspase Transduces Shaggy/GSK-3beta Kinase Activity in Neural Precursor Development. EMBO J (2005) 24:3793–806. doi: 10.1038/sj.emboj.7600822
25. Takada A, Robison C, Goto H, Sanchez A, Murti KG, Whitt MA, et al. A System for Functional Analysis of Ebola Virus Glycoprotein. Proc Natl Acad Sci USA (1997) 94:14764–9. doi: 10.1073/pnas.94.26.14764
26. Shinzawa N, Nelson B, Aonuma H, Okado K, Fukumoto S, Miura M, et al. P38 MAPK-Dependent Phagocytic Encapsulation Confers Infection Tolerance in Drosophila. Cell Host Microbe (2009) 6:244–52. doi: 10.1016/j.chom.2009.07.010
27. Anderson SL, Richards SL, Smartt CT. A Simple Method for Determining Arbovirus Transmission in Mosquitoes. J Am Mosq. Control. Assoc (2010) 26:108–11. doi: 10.2987/09-5935.1
28. Liu IK, Zee YC. The Pathogenesis of Vesicular Stomatitis Virus, Serotype Indiana, in Aedes Aegypti Mosquitoes. I. Intrathoracic Injection. Am J Trop Med Hyg (1976) 25:177–85. doi: 10.4269/ajtmh.1976.25.177
29. Tatsuo H, Ono N, Tanaka K, Yanagi Y. SLAM (CDw150) is a Cellular Receptor for Measles Virus. Nature (2000) 406:893–7. doi: 10.1038/35022579
30. Matsuura Y, Tani H, Suzuki K, Kimura-Someya T, Suzuki R, Aizaki H, et al. Characterization of Pseudotype VSV Possessing HCV Envelope Proteins. Virology (2001) 286:263–75. doi: 10.1006/viro.2001.0971
31. Publicover J, Ramsburg E, Rose JK. A Single-Cycle Vaccine Vector Based on Vesicular Stomatitis Virus can Induce Immune Responses Comparable to Those Generated by a Replication-Competent Vector. J Virol (2005) 79:13231–8. doi: 10.1128/JVI.79.21.13231-13238.2005
32. Lee BH, Yoshimatsu K, Araki K, Okumura M, Nakamura I, Arikawa J. A Pseudotype Vesicular Stomatitis Virus Containing Hantaan Virus Envelope Glycoproteins G1 and G2 as an Alternative to Hantavirus Vaccine in Mice. Vaccine (2006) 24:2928–34. doi: 10.1016/j.vaccine.2005.12.040
33. Kapadia SU, Simon ID, Rose JK. SARS Vaccine Based on a Replication-Defective Recombinant Vesicular Stomatitis Virus is More Potent Than One Based on a Replication-Competent Vector. Virology (2008) 376:165–72. doi: 10.1016/j.virol.2008.03.002
34. Manning JE, Morens DM, Kamhawi S, Valenzuela JG, Memoli M. Mosquito Saliva: The Hope for a Universal Arbovirus Vaccine? J Infect Dis.(2018) 218:7–15 doi: 10.1093/infdis/jiy179
35. Beck AS, Barrett AD. Current Status and Future Prospects of Yellow Fever Vaccines. Expert Rev Vaccines (2015) 14:1479–92. doi: 10.1586/14760584.2015.1083430
36. Fuenmayor J, Gòdia F, Cervera L. Production of Virus-Like Particles for Vaccines. N Biotechnol (2017) 39:174–80. doi: 10.1016/j.nbt.2017.07.010
37. Pijlman GP. Enveloped Virus-Like Particles as Vaccines Against Pathogenic Arboviruses. Biotechnol J (2015) 10:659–70. doi: 10.1002/biot.201400427
38. Pingen M, Bryden SR, Pondeville E, Schnettler E, Kohl A, Merits A, et al. Host Inflammatory Response to Mosquito Bites Enhances the Severity of Arbovirus Infection. Immunity (2016) 44:1455–69. doi: 10.1016/j.immuni.2016.06.002
39. Styer LM, Lim PY, Louie KL, Albright RG, Kramer LD, Bernard KA. Mosquito Saliva Causes Enhancement of West Nile Virus Infection in Mice. J Virol (2011) 85:1517–27. doi: 10.1128/JVI.01112-10
40. Conway MJ, Watson AM, Colpitts TM, Dragovic SM, Li Z, Wang P, et al. Mosquito Saliva Serine Protease Enhances Dissemination of Dengue Virus Into the Mammalian Host. J Virol (2014) 88:164–75. doi: 10.1128/JVI.02235-13
41. McCracken MK, Christofferson RC, Grasperge BJ, Calvo E, Chisenhall DM, Mores CN. Aedes Aegypti Salivary Protein “Aegyptin” Co-Inoculation Modulates Dengue Virus Infection in the Vertebrate Host. Virology (2014) 468-470:133–9. doi: 10.1016/j.virol.2014.07.019
42. Schneider BS, Soong L, Coffey LL, Stevenson HL, McGee CE, Higgs S. Aedes Aegypti Saliva Alters Leukocyte Recruitment and Cytokine Signaling by Antigen-Presenting Cells During West Nile Virus Infection. PloS One (2010) 5:e11704. doi: 10.1371/journal.pone.0011704
43. Thangamani S, Higgs S, Ziegler S, Vanlandingham D, Tesh R, Wikel S, et al. Host Immune Response to Mosquito-Transmitted Chikungunya Virus Differs From That Elicited by Needle Inoculated Virus. PloS One (2010) 5:e12137. doi: 10.1371/journal.pone.0012137
44. Cox J, Mota J, Sukupolvi-Petty S, Diamond MS, Rico-Hesse R. Mosquito Bite Delivery of Dengue Virus Enhances Immunogenicity and Pathogenesis in Humanized Mice. J Virol (2012) 86:7637–49. doi: 10.1128/JVI.00534-12
45. Limesand KH, Higgs S, Pearson LD, Beaty BJ. Potentiation of Vesicular Stomatitis New Jersey Virus Infection in Mice by Mosquito Saliva. Parasite Immunol (2000) 22:461–7. doi: 10.1046/j.1365-3024.2000.00326.x
46. Limesand KH, Higgs S, Pearson LD, Beaty BJJ. Effect of Mosquito Salivary Gland Treatment on Vesicular Stomatitis New Jersey Virus Replication and Interferon Alpha/Beta Expression In Vitro. J Med Entomol (2003) 40:199–205. doi: 10.1603/0022-2585-40.2.199
47. Bergold GH, Suárez OM, Munz K. Multiplication in and Transmission by Aedes Aegypti of Vesicular Stomatitis Virus. J Invertebr. Pathol (1968) 11:406–28. doi: 10.1016/0022-2011(68)90190-0
Keywords: transgenic mosquito, virus, vaccine, infectious diseases, blood-sucking
Citation: Shinzawa N, Kashima C, Aonuma H, Takahashi K, Shimojima M, Fukumoto S, Saiki E, Yamamoto DS, Yoshida S, Matsuoka H, Kawaoka Y and Kanuka H (2022) Generation of Transgenic Mosquitoes Harboring a Replication-Restricted Virus. Front. Trop. Dis 3:850111. doi: 10.3389/fitd.2022.850111
Received: 07 January 2022; Accepted: 07 March 2022;
Published: 19 May 2022.
Edited by:
Eric Ochomo, Kenya Medical Research Institute (KEMRI), KenyaReviewed by:
Haidong Wang, Wageningen University and Research, NetherlandsDenis Voronin, National Institute of Allergy and Infectious Diseases (NIH), United States
Maggy T. Sikulu-Lord, The University of Queensland, Australia
Copyright © 2022 Shinzawa, Kashima, Aonuma, Takahashi, Shimojima, Fukumoto, Saiki, Yamamoto, Yoshida, Matsuoka, Kawaoka and Kanuka. This is an open-access article distributed under the terms of the Creative Commons Attribution License (CC BY). The use, distribution or reproduction in other forums is permitted, provided the original author(s) and the copyright owner(s) are credited and that the original publication in this journal is cited, in accordance with accepted academic practice. No use, distribution or reproduction is permitted which does not comply with these terms.
*Correspondence: Hirotaka Kanuka, a2FudWthQGppa2VpLmFjLmpw
†These authors have contributed equally to this work