- 1Laboratório de Bioquímica de Artrópodes Hematófagos, Instituto de Bioquímica Médica Leopoldo de Meis, Universidade Federal do Rio de Janeiro, Rio de Janeiro, Brazil
- 2Laboratório de Bioquímica de Insetos e Parasitos, Instituto de Biofísica Carlos Chagas Filho, Universidade Federal do Rio de Janeiro, Rio de Janeiro, Brazil
- 3Laboratório de Biologia Molecular de Parasitas e Vetores, Instituto Oswaldo Cruz, Fiocruz, Rio de Janeiro, Brazil
- 4Laboratório de Entomologia Médica, Instituto René Rachou, Oswaldo Cruz Foundation (FIOCRUZ), Belo Horizonte, Brazil
Sexual dimorphism in immune function is prevalent across different species, where males trade their ability to fight pathogens for a practical reproductive function while females favor an extended lifespan. In insects, these differences in immune function reflect an evolutionary life strategy, where females have a presumably more robust immune system than insect males. Here, we evaluate immune functioning in four male and female insect vectors, Aedes aegypti (Diptera, Culicidae), Anopheles aquasalis (Diptera, Culicidae), Lutzomyia longipalpis (Diptera, Psychodidae) and Rhodnius prolixus (Hemiptera, Reduviidae). We show evidence that challenges the concept of immune sexual dimorphism in three of these insect vectors. In the three Diptera species, A. aegypti, A. aquasalis and L. longipalpis that transmit arboviruses, Plasmodium spp. (Haemospororida, Plasmodiidae) and Leishmania spp. (Trypanosomatida, Trypanosomatidae), respectively, unchallenged adult males express higher levels of immune-related genes than adult females and immature developmental stages. The main components of the Toll, IMD, and Jak/STAT pathways and antimicrobial effectors are highly expressed in whole-body males. Additionally, males present lower midgut basal microbiota levels than females. In A. aegypti mosquitoes, the differences in immune gene expression and microbiota levels are established in adult mosquitoes but are not present at the recently emerged adults and pupal stage. Antibiotic treatment does not affect the consistently higher expression of immune genes in males, except defensin, which is reduced significantly after microbiota depletion and restored after re-introduction. Our data suggest that Diptera males have a basal state of activation of the immune system and that activation of a more robust response through systemic immune challenge acutely compromises their survival. The ones who survive clear the infection entirely. Females follow a different strategy where a moderate immune reaction render higher tolerance to infection and survival. In contrast, hematophagous adult males of the Hemiptera vector R. prolixus, which transmits Trypanosoma cruzi, present no differences in immune activation compared to females, suggesting that diet differences between males and females may influence immune sexual dimorphism. These findings expand our understanding of the biology of insect vectors of human pathogens, which can help to direct the development of new strategies to limit vector populations.
Introduction
The immune system evolved for millions of years being polished by natural selection and allowing the coexistence of various organisms. Insects are one of the most successful and abundant animal groups on Earth, partly because of their efficient immune responses that allow them to survive infections. Insects’ immune responses include: (a) epithelial barriers (cuticle, trachea, gut) and clotting; (b) humoral pathway activation including Toll, Immune deficiency (IMD), and Janus Kinase and Signal Transducer and Activator of Transcription (JAK-STAT) pathways with the production of regulators and effectors by the fat body and the midgut epithelia; and (c) hemocyte-dependent cellular reactions, including melanization, coagulation and encapsulation (1). Insects lack antibody-based adaptive immunity, but they mount potent innate immune responses to fight invading organisms [reviewed by (2)].
Aedes aegypti is a well-studied vector of arboviruses such as Dengue, Zika, Chikungunya and Yellow Fever. Mosquito classical pathways Toll, IMD, JAK/STAT and RNA interference have been described as critical to limit invading pathogens [reviewed by (3)] Bacterial and fungal infections trigger the expression of antimicrobial peptides, among the main humoral defense molecules, along with the phenoloxidase activation and complement-like cascades. Activation of Toll and IMD pathways also impact cellular mechanisms of defense, by increasing the lipid droplet contents in the midgut epithelia (4). The microbiota in Aedes aegypti has been pointed out as one of the apices of a tripartite interaction with the mosquito immune system and the infectious agent. The proliferation of the microbiota after a blood meal triggers the expression of antimicrobial peptides and other Toll, IMD and Jak-STAT components, which in turn limit viral replication in the gut impacting vectorial capacity (5, 6).
Anopheles aquasalis is a vector of Plasmodium parasites. Previous studies showed that the JAK-STAT immune pathway (7), GATA Serpent transcription factor (8) and autophagy (9) are activated after A. aquasalis infection with P. vivax. However, only a handful of genes related to immune responses were detected in high throughput expression experiments during early stages of A. aquasalis infection with P. vivax. Bacteria responsive protein 2, Fibronectin, Alpha-2-macroglobulin receptor-associated protein, Ficolin genes and LRR-containing protein were superexpressed in A. aquasalis after infection with P. vivax (9, 10).
The sand fly Lutzomyia longipalpis is the main vector of visceral leishmaniasis in America. Initial L. longipalpis transcriptomic studies reported changes in the expression of several immune-related genes in insects challenged with Leishmania, including components of the Toll, IMD and JNK pathways as well as oxidative stress-related molecules (11, 12). This was followed by the demonstration, for the first time in this vector, of the implication of a specific immune pathway on the successful infection of the insect with Leishmania. Silencing of Caspar, the negative regulator of the IMD pathway, led to a decreased Leishmania infection prevalence and population size (13). Later studies demonstrated the presence of active Toll and IMD responses in even a L. longipalpis embryonic cell line (LL5), in response to various stimuli (14). More recent studies indicated a role for TGF-beta in the control of vector infection by Leishmania (15), and a complex interplay among L. longipalpis AMPs, resident microbiota and Leishmania (16).
Rhodnius prolixus is an important vector of the parasite Trypanosoma cruzi, the etiological agent of Chagas Disease. The immune response of this insect to Trypanosoma spp. involves a hemocyte cellular defense through phagocytosis and nodule formation and a humoral defense with production of eicosanoids, ecdysone, antimicrobial peptides, free radicals and activation of prophenoloxidase [reviewed by (17, 18)].
Across animal kingdom, usually females have longer lifespans and immunity plays a central role in this outcome. Although females are more immunocompetent than males (e.g. (19, 20), they have a higher incidence of inflammatory and autoimmune diseases (e.g. 21). Several factors such as genetic backgrounds in sex chromosomes, anatomic niches, sex hormones and behavior can directly affect immune reactions against pathogens and the development of diseases (22). Ultimately, superior female immunocompetence could reflect a trade-off between immunity and other fitness-related traits that are combined, or independent of, sexual selection (23–28).
The main goal of this study was to evaluate the differences in immune response of the male and female insect vectors A. aegypti, A. aquasalis, L. longipalpis and R. prolixus. To that, we evaluated the expression of immune pathways genes and effectors in different stages of males and females’ life cycle and, in the case of A. aegypti, after challenge with the endosymbiotic bacterium Enterobacter cloacae also evaluating the survival rate and bacterial loads after the challenge. A. aegypti, A. aquasalis, L. longipalpis and R. prolixus were chosen as models due to their importance on arboviruses, Plasmodium, Leishmania and T. cruzi transmission in the Americas. Understanding vector immune responses can be useful for developing new control strategies to interrupt pathogen life cycle or to compromise the vector’s fitness and to successfully block transmission.
Material and Methods
Insect Sampling
A. aegypti (Red Eye strain) were raised in an insectary at the Federal University of Rio de Janeiro, Brazil, according to Barletta and collaborators (4), A. aquasalis in an insectary at René Rachou Institute, Brazil, as described in Bahia and collaborators (10), and L. longipalpis in an insectary at Oswaldo Cruz Foundation, Brazil, according to Lawyer and collaborators (29). R. prolixus were raised in an insectary at the Federal University of Rio de Janeiro as described in Dias and collaborators (30). All insect species were reared at 28°C, 80% humidity and with a 12-hour day/night cycle. Larvae of A. aegypti and A. aquasalis were fed with dog chow and of L. longipalpis with a mixture of rabbit chow and feces. Adults were maintained in cages and fed a solution of 10% sucrose ad libitum. One hour (recently) emerged (before mating)- and four- to seven-day-old Diptera adult insects were used in the experiments. Pupae were separated by sex according to the protocol according to Fay and collaborators (31). R. prolixus unfed male and female adults were raised in an insectary at the Federal University of Rio de Janeiro, Brazil and used for this study.
When necessary, A. aegypti were placed in sanitized cages (cups, filter paper and nets) and 10% sugar solution containing antibiotics (10 μg ml–1 penicillin/streptomycin and 15 μg ml–1 gentamicin; Invitrogen, GIBCO, BRL) (32) in sterile cotton were used to deplete the midgut bacteria.
Bacteria Challenge and A. aegypti Survival
For the survival experiments, E. cloacae from a culture at ~ 1 OD600 (109 bacteria per ml) was washed with PBS and resuspended to a final concentration of 108 bacteria per μl. The bacteria were injected (in 69nL) in A. aegypti male and female thoraxes using a nano-injector Nanoject III (Drummond). Sucrose solution (10%) was provided just after the procedure. After the challenge, mosquitoes were monitored for survival daily. Three independent experiments were performed. Survival percentages represent the mean survival percentage for all three biological replicates. Statistical significance was determined by Kaplan–Meier survival analysis with GRAPHPAD PRISM9 software (GraphPad Software, La Jolla, CA, USA), and P-values were determined by the Wilcoxon test as described in Corby-Harris and colleagues (33). Two days after injection, individual A. aegypti males and females were homogenized in PBS and spread on LB agar plates in serial dilutions. Plates were placed at 37°C overnight to allow E.cloacae growth. This experiment was performed three times, and the bacteria of five to six male and female adult individual mosquitoes were quantified in each experimental group (PBS and bacteria-injected) based on CFU.
Bacterial Culture Growth
To quantify the midgut cultivable bacteria from A. aegypti mosquitoes, insects were surface sterilized with 1% Sodium Hypochlorite for 1 minute, then 70% Ethanol for another minute and lastly in PBS to remove most of the chemicals. The midgut was dissected under aseptic conditions. Pools of 5 midguts were homogenized in Luria-Bertani (LB) medium, serially diluted, and plated on LB agar. Plates were allowed to grow overnight at 28°C and the number of colony forming units (CFU) was counted.
Microbiota Depletion and Microbiota Introduction
To deplete the microbiota from 4 – 7 day old A. aegypti mosquitoes, fresh antibiotic mixture (100 I.U./mL Penicillin, 100 μg/mL Streptomycin) was offered daily in the sugar cotton for 3 days.
To evaluate the effect of the establishment of the microbiota, recently emerged A. aegypti mosquitoes (up to 1 hour post emergence) were kept on sugar containing fresh antibiotic mixture (100 I.U./mL Penicillin, 100 μg/mL Streptomycin) or fed a sugar meal containing a microbiota mixture from our own colony. To isolate that mixture, four-day old A. aegypti were surface sterilized as previously described and the midgut was dissected, homogenized in LB medium and placed in a shaker overnight at 250 rpm at 28°C. The next day bacteria mixture was washed with PBS and then frozen at -80°C. Stocks were then growing overnight in LB medium at 28°C and then mixed with the sugar solution (10% sucrose). Sugar cotton was changed daily with a fresh microbiota solution.
RNA Extraction, cDNA Synthesis and qPCR Analysis
The RNA from A. aegypti, A. aquasalis and L. longipalpis 4-7 days old unchallenged males and females, A. aegypti sexed pupae, recently emerged adults and unfed adult males and females R. prolixus was extracted using the TRIzol™ Reagent (whole body) (Invitrogen, Carlsbad, CA, USA) according to Manufacturer’s protocol. Briefly, pools of 5 insects were placed in TRIzol, and homogenized with a motorized pestle. Two hundred microliters of chloroform were added (1/5 of TRIzol volume) to 1mL of TRIzol for the aqueous phase separation. Total RNA was precipitated with 2-propanol (1:1) added to the aqueous phase and resuspended in 30μl of nuclease free water. RNA concentration was measured at 260nm using a Nanodrop (ThermoFisher Scientific, Waltham, MA, USA). One microgram of total RNA was treated with Turbo DNase (Ambion Life Technologies, Grand Island, NY, USA) to remove genomic DNA contamination. Then, we proceed to the complementary DNA (cDNA) synthesis using one of the following kits: High-Capacity cDNA Reverse Transcription Kit (Applied Biosystems, Waltham, MA, USA) and the MMLV Reverse Transcriptase 1st-Strand cDNA Synthesis Kit (Promega, Madison, WI, USA) following the manufacturer’s instructions. Gene expression was assessed by quantitative PCR (qPCR) using the resulting cDNA as a template. We used the Sybr Green Master Mix Kit (Applied Biosystems, Life Technologies, Grand Island, NY, USA) with target specific primers and the assay ran on a 7500 Real-Time System (Applied Biosystems, Waltham, MA, USA) in a 15μl reaction. We used 40 reaction cycles of 95°C for 10 min, 95°C for 15 s and 60°C for 1 min. Relative quantitation results were normalized using the constitutive ribosomal protein 49 (Rp49) gene of A. aegypti, A. aquasalis (34) and L. longipalpis (35) and elongation factor 1 to R. prolixus (36). Constitutive gene expression of Rp49 or elongation factor 1 were used as an internal standard and analyzed using the ΔΔCt method (37, 38). Statistical differences of fold change values between the conditions were determined using either unpaired t-test or paired t-test in case of paired samples (GraphPad, San Diego, CA, USA). Each independent experiment was done with three biological replicates (three pools of 5 insects) for each condition tested, and each one was analyzed by qPCR in duplicates (technical replicates). At least three independent experiments were performed. Primer sequences used for A. aegypti, A. aquasalis, L. longipalpis and R. prolixus experiments are listed in Table S1 and in Barletta and collaborators (4); Bahia and collaborators (8, 10); Telleria and collaborators (16, 39), Tinoco-Nunes and colleagues (14); Berni and collaborators (40) Majerowicz and colleagues (36), Vieira and collaborators (41). The 16S rRNA gene was used to quantify bacterial community abundance (42).
Results
A. aegypti Males Presented Higher Transcriptional Levels of Immune-Related Genes and Reduced Bacterial Microbiota Than Females
To investigate differences in A. aegypti basal immune activation we evaluated the expression of immune genes in 4–7-day old unchallenged adult males and females. A higher transcriptional level of immune-related genes was observed in males in comparison to adult females (Figure 1). Toll pathway genes, like the downstream adaptor Myd88, the inhibitor cactus and the serine protease inhibitor gene serpin were significantly higher in males than females. Although the transcription factor REL1 did not present a significant difference between individuals, there was a tendency of higher expression in males (Figures 1A–D) For IMD pathway, the transcription factor REL2, the protein IMD and the antimicrobial peptide defensin were also higher in males than females, the latest being 35-fold higher in males (Figures 1E–G). Components of the JAK-STAT pathway, such as transcription factor STAT and complement-like molecule TEP were more than 3 times higher expressed in males (Figures 1H, I).
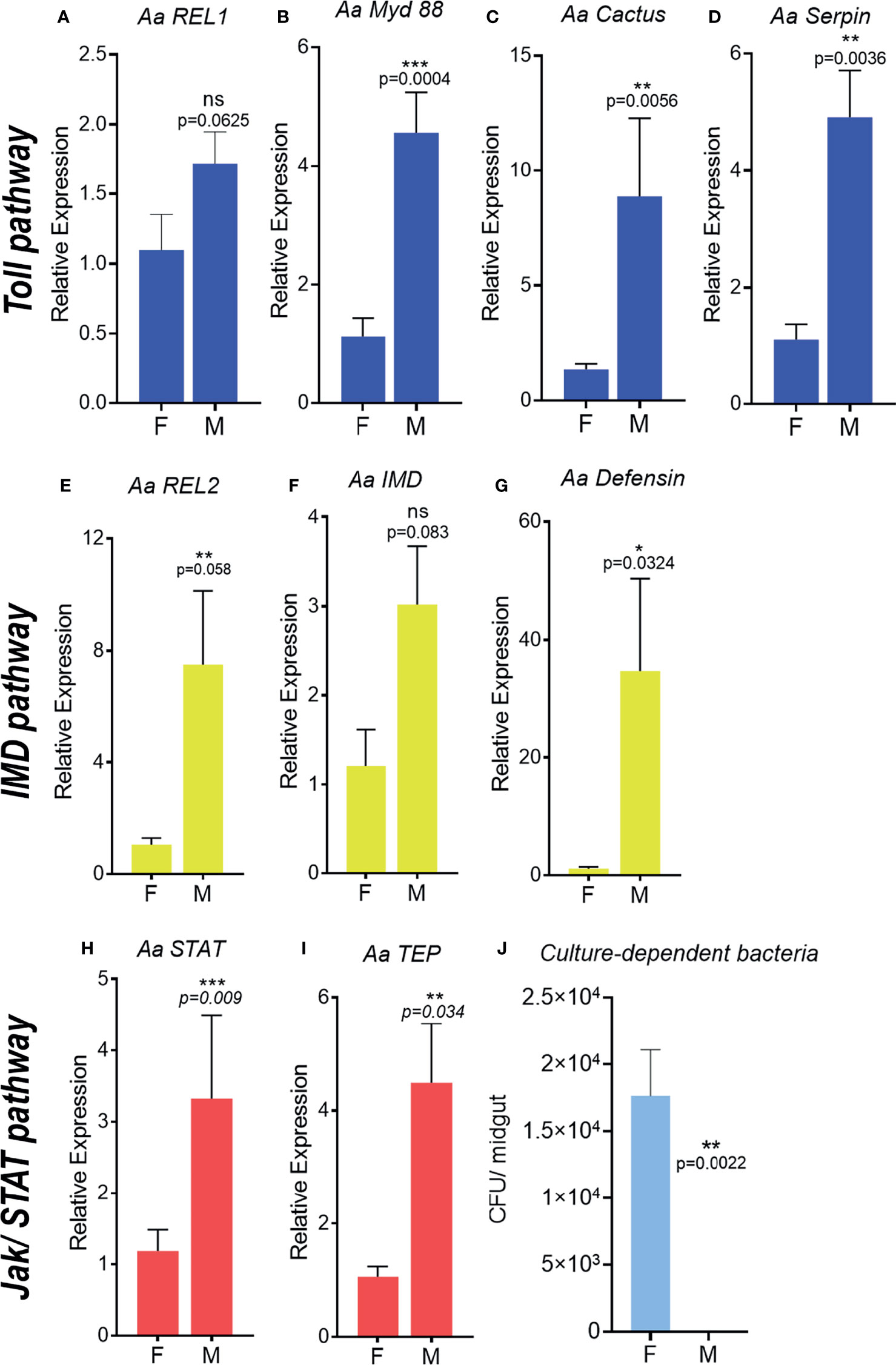
Figure 1 Immune pathway activation and microbiota quantification in unchallenged adult male and female Aedes aegypti. Relative expression of genes related to the Toll pathway in adults, REL1, (A), MyD 88 (B), Cactus (C), Serpin (D). Relative expression of genes related to the IMD pathway in adults, REL2 (E), IMD (F), Defensin (G). Relative expression of genes related to the JAK/STAT pathway in adults, STAT (H), TEP (I). Number of cultivable bacteria in the adult’s intestinal tract (J). F, female; M, male; CFU, colony forming units. ns, non-significant P < 0.05, *P ≤ 0.05, **P ≤ 0.01, ***P ≤ 0.001.
To investigate the effect of immune-related genes upregulation in A. aegypti males on the microbiota, we quantified the cultivable microbiota from the intestinal tract of unchallenged males and females. The unchallenged A. aegypti males presented no detectable levels of cultivable bacteria in its intestinal tract (Figure 1J and Figure S1B). However, unchallenged females’ intestinal tract harbor approximately 1.7 x 104cultivable bacteria (Figure 1J and Figure S1A), which was comparable to previous description of sugar-fed A. aegypti females (43).
Basal State of Immune Activation Is Not Present in Male Pupae and Recently Emerged A. aegypti Adults
To determine the onset of immune-related genes upregulation in A.aegypti males, expression levels of selected genes from sexed pupae and recently emerged male and female adults were evaluated. We did not observe differences in expression of the Toll (REL1, Myd88, Cactus, Serpin) (Figures 2A–D, 3A–E) and IMD pathway related genes (REL2, IMD and defensin) (Figures 2E–G, 3E–G) in neither pupae or recently emerged adult males and females. For Jak-STAT pathway, only transcription factor STAT was significantly upregulated in male pupae (Figure 2H), but not in recently emerged males (Figure 3H). No differences in TEP expression were observed in pupae and recently emerged adults (Figures 2I, 3I).
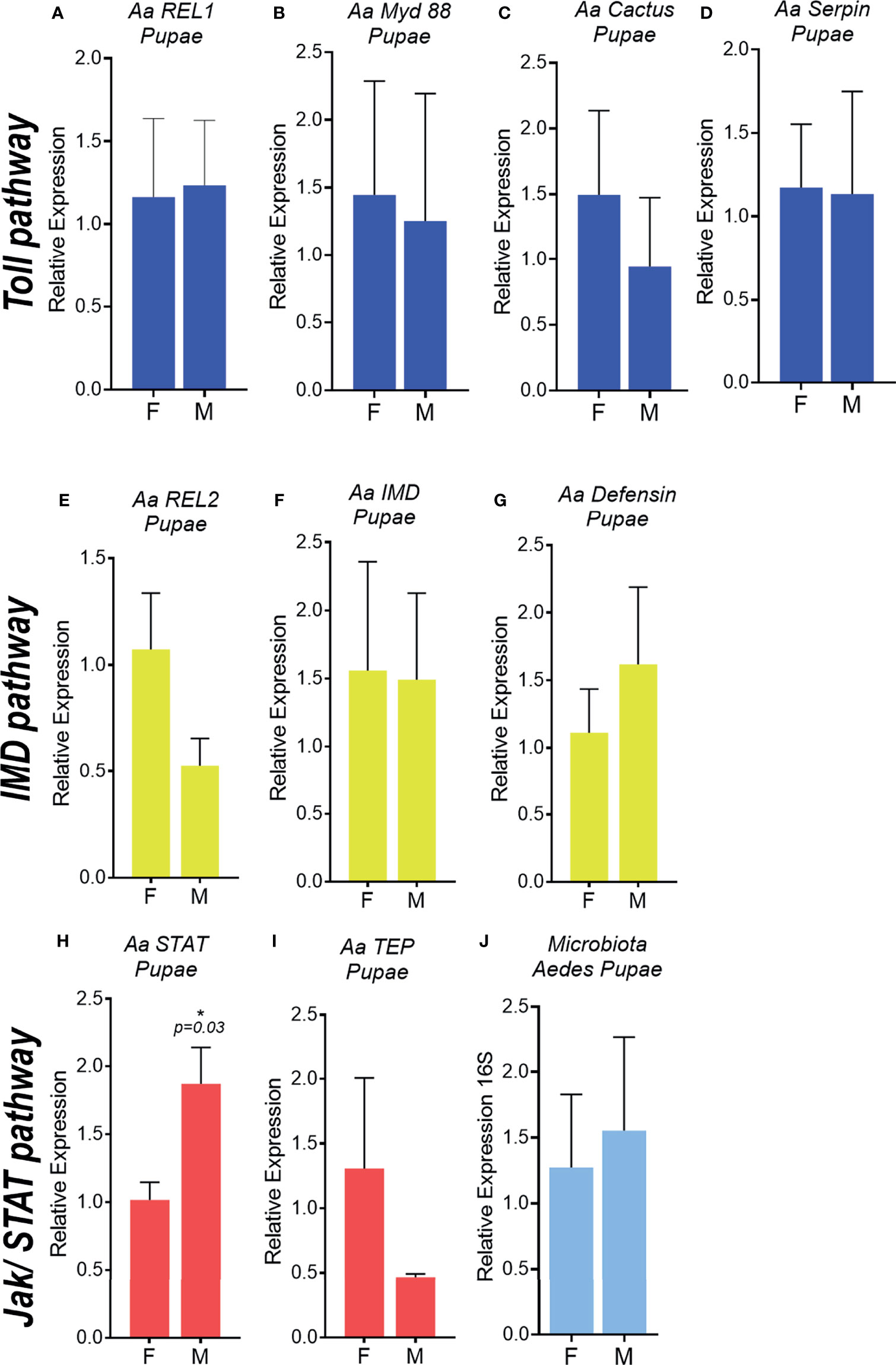
Figure 2 Immune pathway activation and microbiota quantification in male and female Aedes aegypti pupae. Relative expression of genes related to the Toll pathway in pupae, REL1 (A), MyD 88 (B), Cactus (C), Serpin (D). Relative expression of genes related to the IMD pathway in pupae, REL2 (E), IMD (F), Defensin (G). Relative expression of genes related to the JAK/STAT pathway in pupae, STAT (H), TEP (I). 16S rDNA relative expression in pupae (J). F, female; M, male. *P ≤ 0.05.
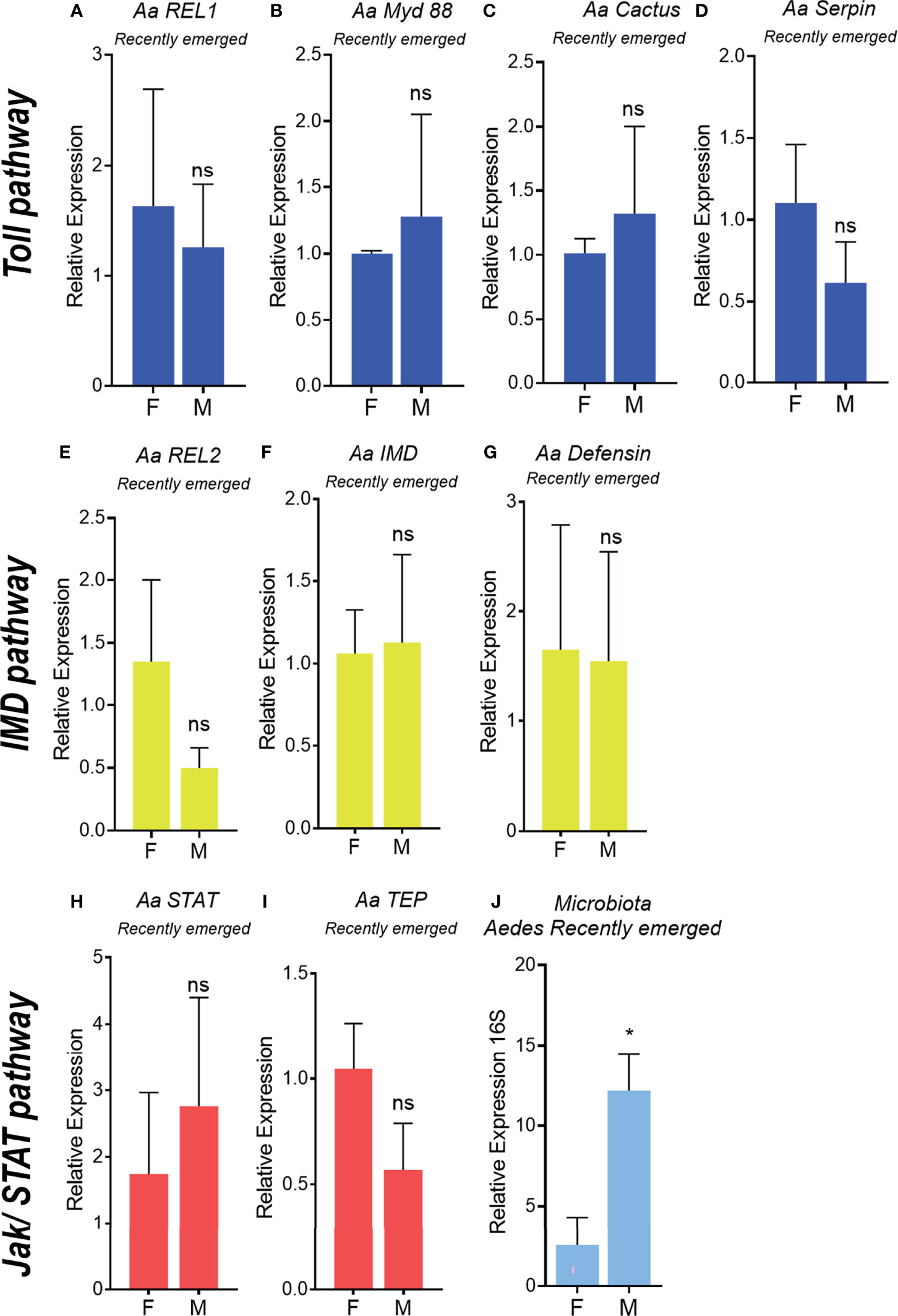
Figure 3 Immune pathway activation and microbiota quantification in recently emerged Aedes aegypti adults. Relative expression of genes related to the Toll pathway in recently emerged males and females, REL1 (A), MyD 88 (B), Cactus (C), Serpin (D). Relative expression of genes related to the IMD pathway in recently emerged males and females, REL2 (E), IMD (F), Defensin (G). Relative expression of genes related to the JAK/STAT pathway in recently emerged males and females, STAT (H), TEP (I). 16S rDNA relative expression in recently emerged adults (J). F, female; M, male. ns, non-significant P < 0.05, *P ≤ 0.05.
A. aegypti-Sexed Pupae Did Not Present Differences in Microbiota, but Recently Emerged Males Had Higher Levels of Microbiota Than Females
To evaluate the differences in microbiota levels in pupae and recently emerged females and males, the total bacteria from the A. aegypti intestinal tract in these insect groups was evaluated through 16Sr DNA expression. No differences were observed in total bacterial loads in A. aegypti male and female pupae (Figure 2J), but a 12-fold increase in the microbiota was found in recently emerged males compared with females (Figure 3J).
Antibiotic Treatment Did Not Affect Basal Immune Activation of A. aegypti Unchallenged Males
To test if bacterial microbiota influences immune-related gene expression, A. aegypti unchallenged males and females were treated with antibiotics for 3 days prior to expression analysis. The profile of expression of immune genes between males and females remains the same after antibiotics treatment, where males presented a significant (or a tendency to) higher expression than females (Figures 4A–F). Immune-related gene expression did not change between antibiotic-treated and untreated females (Figures 4A–D). However, the depletion of males’ microbiota significantly decreased Cactus expression but no other Toll pathway component or any other gene from the remaining pathways (Figure 4).
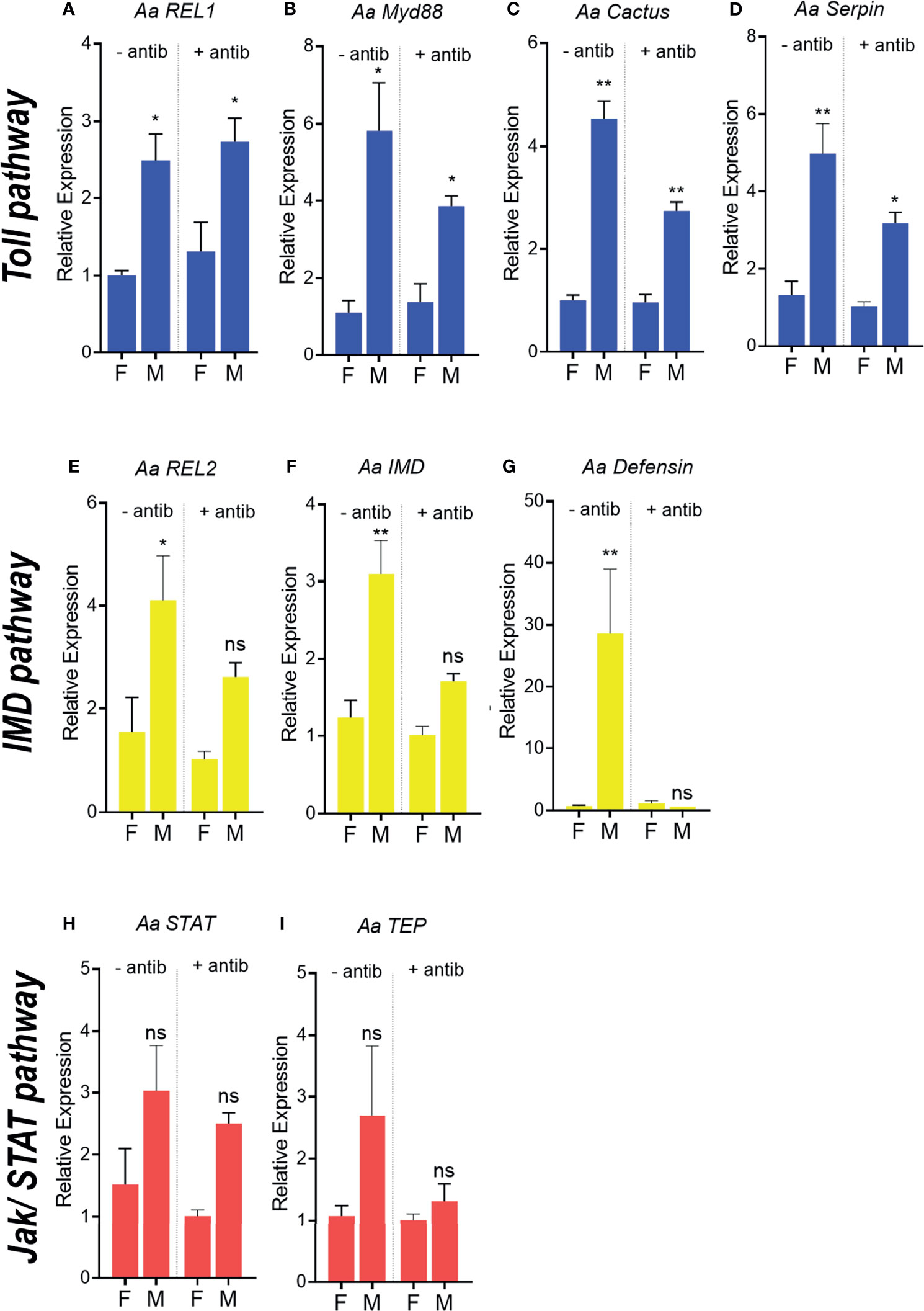
Figure 4 Antibiotic treatment and expression of immune-related genes in Aedes aegypti females and males. Relative expression of genes related to the Toll pathway in recently emerged males and females, REL1 (A), MyD 88 (B), Cactus (C), Serpin (D). Relative expression of genes related to the IMD pathway in adults, REL2 (E), IMD (F), Defensin (G). Relative expression of genes related to the JAK/STAT pathway in adults, STAT (H), TEP (I). - antib - without antibiotic treatment, + antibiotic - antibiotic treatment. ns, non-significant P < 0.05, *P ≤ 0.05, **P ≤ 0.01.
Introduction of the Microbiota in Recently Emerged A. aegypti Did Not Alter Sexual Dimorphism of Immune Gene Expression
To evaluate if microbiota establishment could play a role in determining sexual dimorphism in systemic immunity, recently emerged mosquitoes were either kept for 4 days in antibiotic solution or treated with a sugar meal containing a mixture of bacteria from the microbiota. Recently emerged males treated with antibiotics since emergence presented the same expression pattern as males that had microbiota introduced in the sugar meal. Toll pathway components were high in males of both groups in relation to females (Figures 5A–D). The same effect was observed for IMD and Jak/STAT markers (Figures 5E–I). Male mosquito’s microbiota depletes and recently emerged ones, before microbiota establishment had a similar expression pattern, with high levels of immune related genes in comparison to their respective female groups (Figures 4, 5). This confirms that immune sexual dimorphism is not dependent on the presence or the establishment of the microbiota in the midgut.
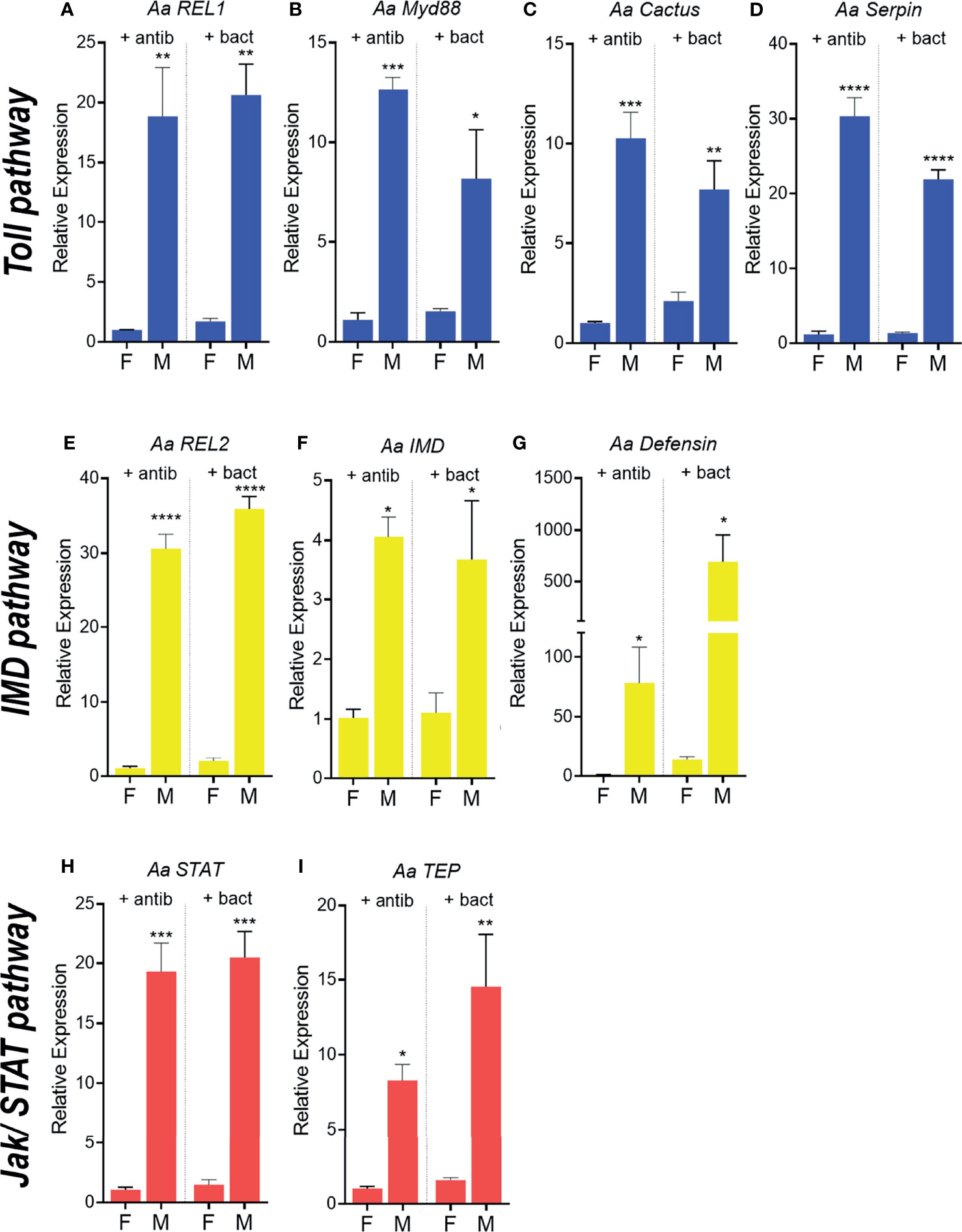
Figure 5 Expression of immune-related genes in Aedes aegypti females and males treated with antibiotics and supplemented with microbiota. Relative expression of genes related to the Toll pathway in females and males treated with antibiotic since emergence compared with insects or treated with antibiotic and supplemented with the microbiota, REL1 (A), MyD 88 (B), Cactus (C), Serpin (D). Relative expression of genes related to the IMD pathway in adults, REL2 (E), IMD (F), Defensin (G). Relative expression of genes related to the JAK/STAT pathway in adults, STAT (H), TEP (I). - antib - without antibiotic treatment, + antibiotic - antibiotic treatment. *P ≤ 0.05, **P ≤ 0.01, ***P ≤ 0.001, ****P ≤ 0.0001.
Male mosquitoes kept on antibiotics for four days since emergence already expressed high levels of immune genes in comparison to females submitted to the same treatment.
A. aegypti Males Clear Bacteria Levels in the Hemolymph After Challenge, Albeit With No Impact on Their Survival Rates in Comparison to Females
To functionally analyze and compare the ability of A. aegypti males and females to fight infections, we injected mosquitoes intrathoracically with approximately 105 E. cloacae. After the challenge, we quantified the bacteria load and evaluated insect survival. Males had a drastic drop in survival 2 days post challenge, reaching 45.6%, while females at the same day had 78% survival (Figure 6A). However, 3 days post challenge, females presented a sharp decrease in survival reaching 34.7%, while male survival was stable (Figure 6A). Bacterial levels present in the body of females and males, 2 days post bacterial challenge were quantified. Interestingly, 2 days post challenge, bacteria load present on the body of challenged males were comparable to unchallenged mosquitoes, demonstrating that surviving males were able to clear the infection (Figures 6B, C). Nevertheless, challenged females had high levels of bacteria 2 days post bacterial injection, indicating that females can tolerate high levels of bacteria enabling their survival (Figures 6B, C).
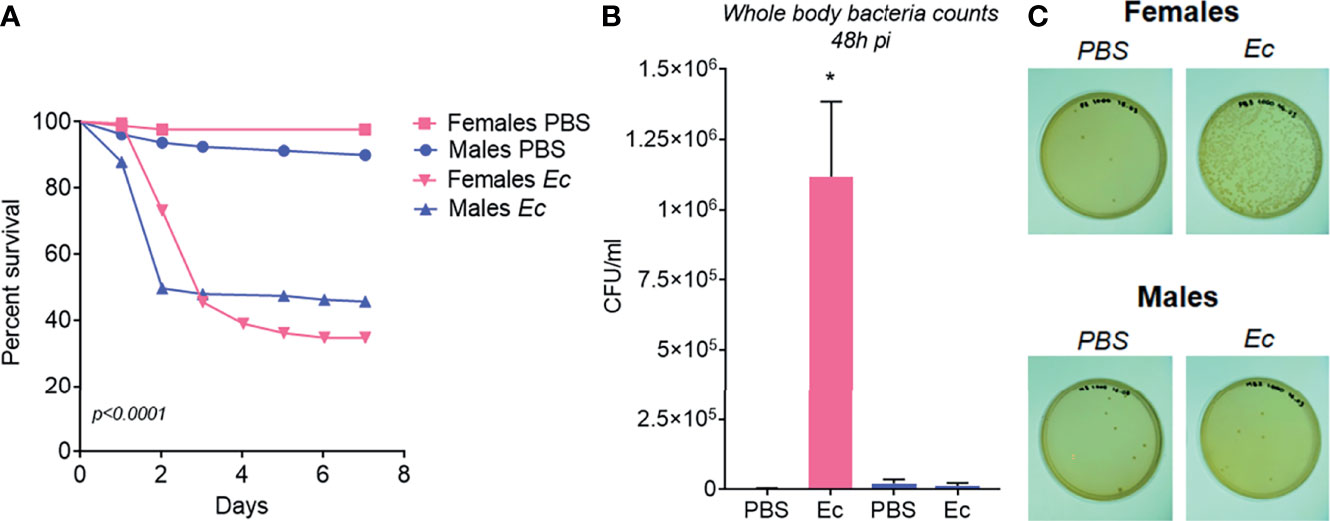
Figure 6 Functional experiments to access Aedes aegypti females and males immune response. (A) Survival rate from 1-7 days after females and males being injected with PBS or approximately 105 Enterobacter cloacae bacteria. (B) Bacteria counting from females and males two days after injection with PBS or E. cloacae. (C) Agar Plates with bacteria collected from females and males two days after injection with PBS or E. cloacae. *P ≤ 0.05.
A. aquasalis and L. longipalpis, Also Presented High Expression of Immune Genes and Reduced Levels of Bacterial Microbiota in Males Compared to Females
To understand if sex related differences in immune activation observed in A. aegypti adults also occurred in other vectors of human pathogens, we evaluated the expression of immune genes in A. aquasalis and L. longipalpis. A. aquasalis and L. longipalpis do not have their genome published and A. aquasalis is a poorly studied mosquito. This fact restricts the possibilities of analyzing expression of a comprehensive list of immune related genes, as was tested in A. aegypti.
In A. aquasalis males we observed a significantly higher expression of immune-related genes, nitric oxide synthase, fibronectin, ficolin and IAP (Figures 7A–D), as compared to females. Other immune-related genes, such as the transcription factor CLIP and GATA-Serpent, also have a tendency for higher expression in males than females albeit are not statistically different (Figures 7E, F). In L. longipalpis antimicrobial peptides, attacin, cecropin, lysozyme and defensin, (Figures 8A–D) had a higher basal transcription in unchallenged males compared to females. The same pattern was observed in genes from signaling cascades, Spatzle, Rel and STAT (Figures 8E–G).
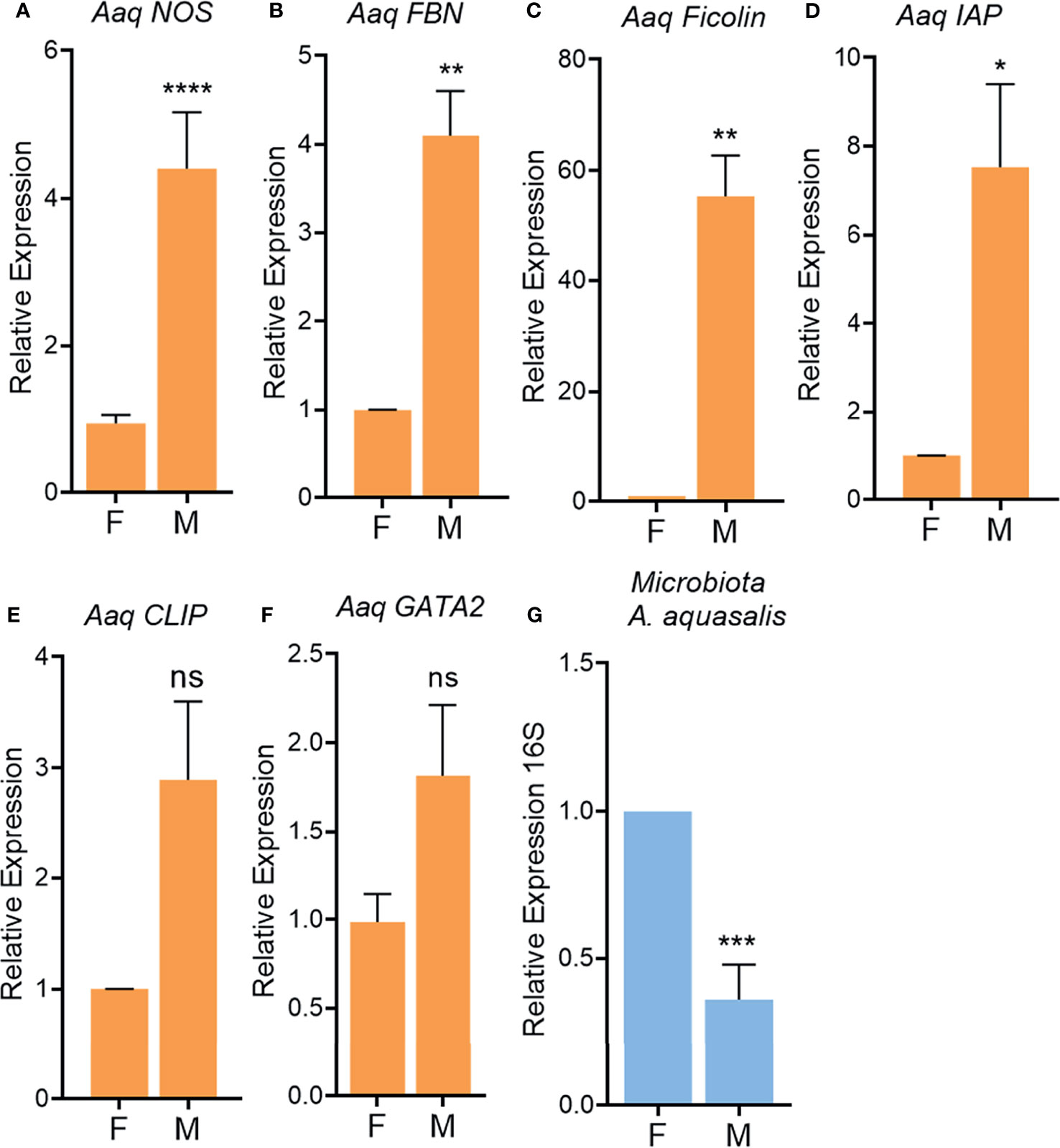
Figure 7 Immune-related gene activation in unchallenged male and female adult Anopheles aquasalis. Relative expression of the genes NOS (A), Fibronectin (FBN, B), Ficolin (C), IAP (D), CLIP (E), GATA (F) in adults. 16S rDNA relative expression in adults (G). F, female; M, male. ns, non-significant P > 0.05, *P ≤ 0.05, **P ≤ 0.01, ***P ≤ 0.001, ****P ≤ 0.0001.
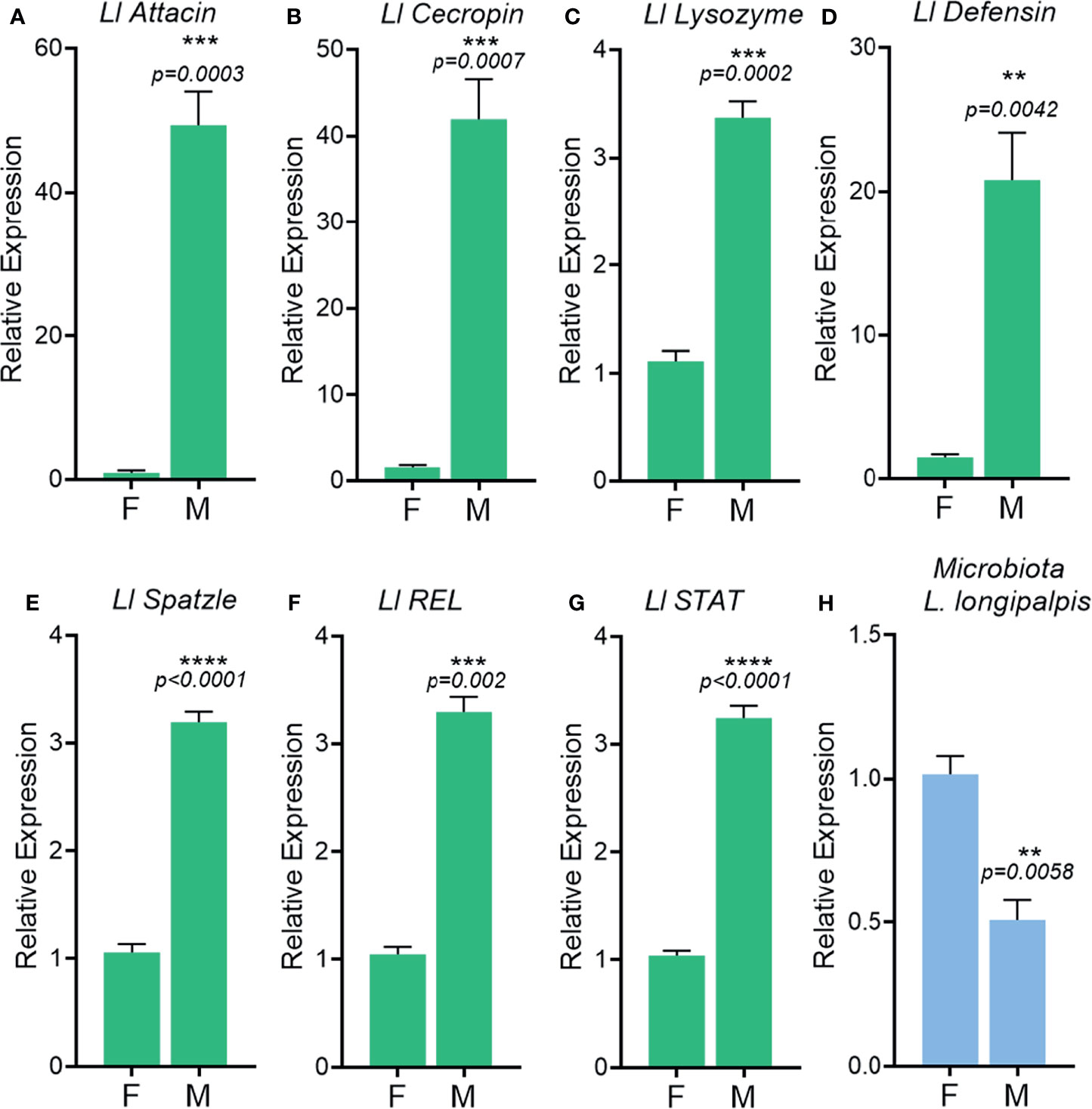
Figure 8 Immune-related gene activation in unchallenged male and female adult Lutzomyia longipalpis. Relative expression of the genes Attacin (A), Cecropin (B), Lysozyme (C), Defensin (D), Spatzle (E), REL (F), STAT (G) in adults. 16S rDNA relative expression in adults (H). F, female; M, male. **P ≤ 0.01, ***P ≤ 0.001, ****P ≤ 0.0001.
To understand if the higher expression of immune-related genes in A. aquasalis and L. longipalpis males would influence gut microbiota, bacteria from the intestinal tract of males and females were evaluated. Unchallenged A. aquasalis and L. longipalpis males presented significantly lower levels of total bacteria in its intestinal tract when compared to females (Figures 7G, 8H, respectively).
The results of immune-related genes expression and bacteria quantification in A. aquasalis and L. longipalpis were along the lines with the A. aegypti findings, where males had a higher basal immune activation and presented low levels of bacteria when compared to females (Figures 1A–J, 7A–G, 8A–H).
R. prolixus Females Presented High Expression of Immune Genes and Levels of Microbiota Compared to Males
To test if evolutionary differences in feeding behavior would influence immune sexual dimorphism, we compared the expression of immune genes between males and females of another human pathogen vector, R. prolixus, where both sexes are hematophagous. Surprisingly, males showed a lower expression of the immune-related genes tested (Dorsal, Cactus, Lysozyme B, Defensin A) as compared to females (Figures 9A–D). Defensin C was not significantly different between males and females (Figure 9E). Microbiota loads as well as the levels of expression of immune-related gene, were higher in females in comparison to males (Figure 9F).
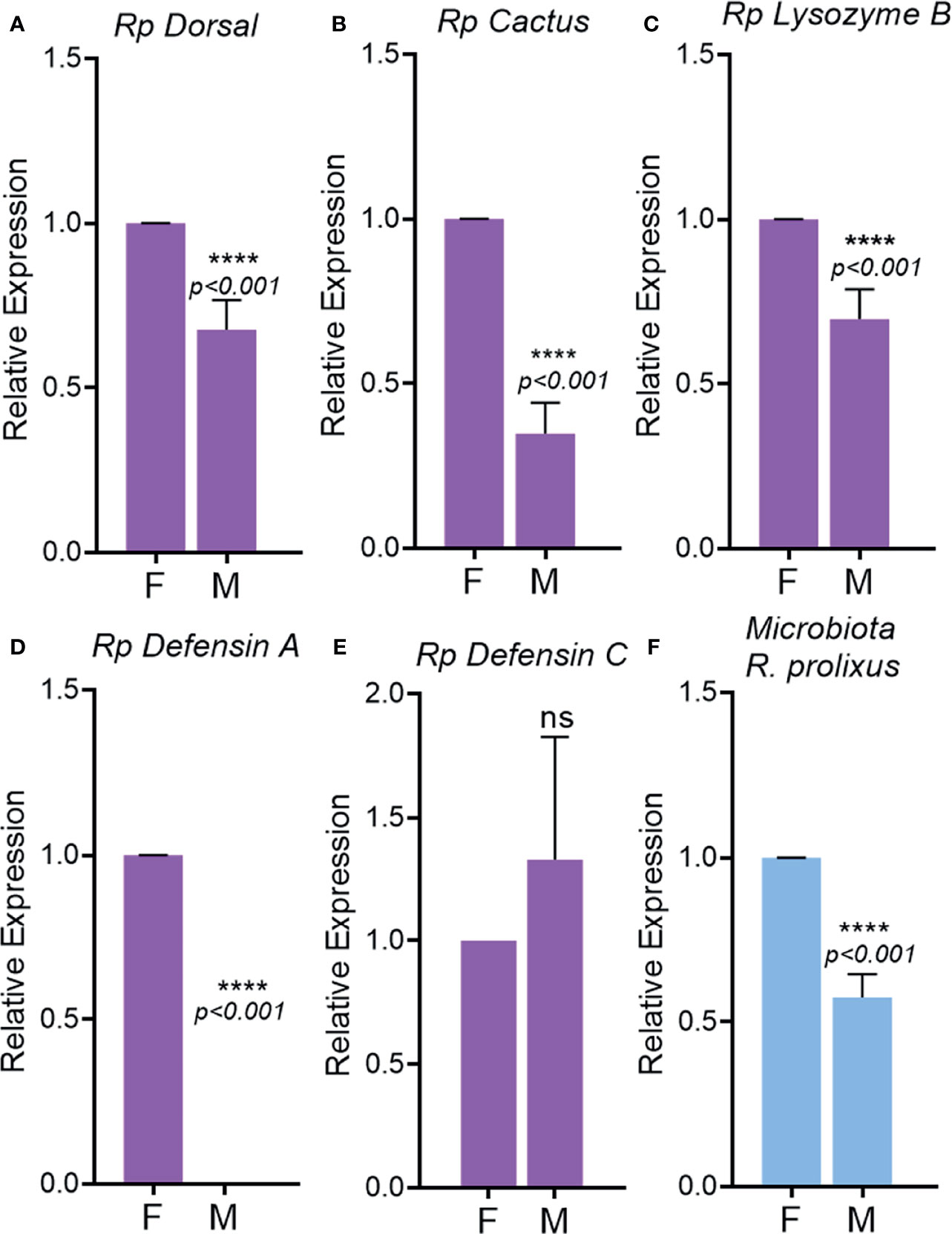
Figure 9 Immune-related gene activation in unchallenged males and females adults of Rhodnius prolixus. Relative expression of the genes Dorsal (A), Cactus (B), Lysozyme B (C), Defensin A (D), Defensin E (E). 16S rDNA relative expression in adults (F). F, female; M, male. ns, non-significant P > 0.05, ****P ≤ 0.0001.
Discussion
In animals, it is proposed that differences in immune system response between sexes causes variation in prevalence and intensity of infection, with females typically presenting greater immunity and more incidence of autoimmune diseases. In Drosophila (Diptera, Drosophilidae), dimorphism in infection outcome is also present, albeit the reported directions are difficult to generalize because are often condition- and pathogen-dependent. In general, the mechanisms related to sex differences in immunity are largely unresolved due to a lack of truly comparative studies (44). Here, we present a cross-species study where unchallenged adult males have higher transcriptional levels of immune-related genes than adult females while showing lower midgut basal microbiota. In contrast, the Hemiptera vector R. prolixus shows a different pattern of gene expression that will be further discussed.
In A. aegypti mosquitoes, the differences in immune gene expression and microbiota levels were not observed at the pupal stage and immediately after mosquito emergence. However, divergences are observed later in development even under antibiotic pressure, indicating that sexual dimorphism in A. aegypti is not dependent on the presence or establishment of the microbiota. One could speculate that timely-regulated hormonal action or epigenetic factors could triggers those differences in expression between males and females.
Interestingly, recently emerged male virgin mosquitoes did not show immune differences but present a 12-fold higher microbiota in comparison to females. Also, it is known that mating can modify the existing microbiota amount and composition (45).The high number of bacteria in the recently emerged males may triggers high activation observed later in male mosquitoes (46).
Besides load, bacterial composition plays important role in immune activation (47, 48) in mosquitoes and could also interfere in sexual dimorphism. Previous reports indicate no statistical difference in microbiota diversity in Aedes koreicus, both males and females are dominated by the phylum Proteobacteria, mainly, represented by the genus Asaia, Pseudomonas and Flavobacterium (49). However, in 2021 Diaz et al. demonstrated that overall, the most common bacteria for A. aegypti across male tissues was Asaia, with a relative abundance in the gut of 65.87%. In females, mating status influenced microbiota composition in the gut. In virgin females, Asaia and Serratia were the most abundant genus while in sugar fed mated females Pseudomonas were the most abundant (45). The gut microbiota from virgin males presented a poor diversity in comparison to females, regardless the mating or physiological status, like blood ingestion (45). That could point to a possible role of Asaia in maintaining the hyper-immune status in males, as females have a more complex and diverse microbiota composition.
In our experiments, depletion of a well-established microbiota upon antibiotic treatment in 4-7day old mosquitoes reduces the gene expression of IMD pathway components in males. The expression of Defensin is highly reduced in males after antibiotic treatment and, the same pattern is observed for the other components of the IMD pathway. In A. aegypti the IMD pathway controls microbiota levels in the midgut (50), which in turn regulates IMD components expression, like Defensin (51). Nonetheless, when antibiotics are given soon after emergence preventing the acquisition and establishment of the regular microbiota, IMD components expression is not affected. Although antibiotic treatment greatly reduced levels of the microbiota, the effects of antibiotic administration immediately post-emergence can put a qualitative selective pressure on which bacterial species can colonize the midgut. Studies on Aedes albopictus showed that antibiotic pressure in recently emerged adults rather than bacteria exclusion can cause dysbiosis (52).
Interestingly, A. aegypti males systemically challenged with E. cloacae had a sharp drop in survival, but the surviving males completely cleared the infection. This suggests that although male initial mortality was greater, they did not succumb due to infection, but might have died from an exacerbated immune reaction (53). On the other hand, females presented the opposite scenario, they endured the initial challenge having high levels of bacteria, which could indicate tolerance to the infection [i.e. (54)]. Besides that, evidence in literature support the fact that sexual dimorphism in A. aegypti can also significantly affect Dengue virus transmission in the field. In this way, it has been reported, that Vertical transmission of Dengue virus serotype 2 and 3 are significantly lower in the male progeny in comparison to females. Female infection rate reached 4.46%, while males presented a 0.56% infection rate (55).
A. aquasalis and L. longipalpis presented similar results to A. aegypti related to expression of immune genes and bacteria loads in males and females. Here we have shown that the expression of five genes involved in the insect immune response (ficolin, fibronectin, GATA factor, nitric oxide synthase and IAP-5) was higher in A. aquasalis unchallenged males than females. This result agrees with our previous studies that also show high expression of immune related genes in males compared to females (fibrinogen, bacteria responsive protein, cecropin, serpin, STAT, PIAS, catalase, SOD3a and SOD3B) (7, 10, 56). As mentioned before, various pathways and immune related molecules have been identified in L. longipalpis. Among these, the modulation of immunity related genes, including AMPs, was seen in relation to different challenges. In a recent publication, Telleria and collaborators (16) compared the expression of AMPs in male and female L. longipalpis at one and two days after sugar feeding. From the six AMPs studied, four had a striking higher expression in males at 2 days post feeding, while the other two showed a more discrete increase in expression. In parallel, the microbiota decreased significantly in males at day two, in relation to females. In the present study these results were corroborated, and we saw the higher expression in males of various AMPs and the activating ligand Spatzle (Toll pathway), STAT (Jak-STAT pathway) and Rel (IMD pathway). Accordingly, microbiota was also less abundant in males 48 hours after sugar feeding. Sexual dimorphism in immune-related gene expression might be explained by the feeding diet. Males feed exclusively on sugar rich saps; thus, they may have adapted to a stronger antibacterial immune response to control the growth of carbohydrate-related bacteria in their gut. The intersection of environmental variables such as diet and immune responses is likely to dictate immunocompetence outcomes.
The results from Diptera insects all together indicate that males are more prepared for eventual microorganism challenges. This might be an evolutionary strategy adopted by males to keep healthy during their short lifespan since they live less than females (57). Alternatively, males could express higher levels of effector genes, such as antimicrobial peptides, to transfer them to females during mating, as seen in Drosophila with andropin (58), found in accessory glands or ejaculatory ducts, and transfer them within the seminal fluid (59). Also, drosomycin is detected in Drosophila ejaculatory structures and is likely to be present in the ejaculate (60).
In Diptera, immune activation of unchallenged males could also be explained by the hypothesis of the susceptible male proposed by Hamilton and Zuk (61). According to that theory animals would choose mates that were genetically resistant to parasites and diseases, which could influence features of health and vigor. In that study, for birds, parasitic diseases can impact on plumage, vigor, and urine, which would decrease their chances of being chosen at courtship (61). Therefore, it is possible that Diptera males that are more resistant to infection and therefore display a more robust expression of immune-related genes were evolutionary selected.
In mammals’ hormones are believed to be partly responsible for the differences in immune activation between sexes (62). Presence of high concentrations of insects hormones, such as ecdysone and 20-OH-ecdysone, can act as an androgenic and estrogens sex steroid, respectively (63).
As opposed to vertebrates, arthropods lack the negative feedback system between the immune system and expression of sexual features and behavior, driven by testosterone/testosterone-like. For that reason, infections have a higher cost for male vertebrates but not as much for male invertebrates (64).
According to the Bateman’s principle and immunity, selection to prolong the period of egg production favors improved immune function in females while males allocate less towards immunity due to increased resources investment in traits critical to sexual selection (65). In Drosophila melanogaster, the Toll pathway has a central role in determining sexual dimorphism in response to Beauveria bassiana fungi (66).
The three Diptera vectors analyzed in this study have differences in feeding habits between sexes, where males feed on sugar solutions (nectar and plant sap) and females on sugar solutions and blood. In contrast, the Hemiptera R. prolixus male and female adults, which share a restricted hematophagous diet and are capable of modulating AMP gene expression upon ingestion of bacteria (67), presented an opposite trend in immune competence between sexes. The R. prolixus immune activation is more prominent in unfed females than males. These findings alert us that the differences in nutrient uptake (acquisition hypothesis) by Fanson and colleagues (65) between male and female Diptera may be the central point in the distinct immune competence of males. Diet changes, like food restriction, can also contribute to the emergence of stronger immune responses in males. In crickets under food restriction, females invest more in reproduction and breeding, which compromises their immune responses and they become more susceptible to infections (68). In Drosophila equality in immunity was observed when food availability and success in mating were present (69).
D. melanogaster males have higher expression of many Toll-regulated genes (44), which is less pronounced for the IMD pathway genes. The expression of some antimicrobial peptides (AMPs) requires a functional Toll pathway. The loss of function of this pathway affects males more strongly than females in response to challenge (70). Dimorphisms are found in IMD and Jak/STAT pathways with some components higher in females than males, with some exceptions. The antimicrobial peptide Diptericin, that is induced by the IMD pathway (71), has higher expression in unchallenged males than females; the Jak/STAT components, TurandotA, TurandotC, and TurandotM are male biased in response to infection (72).
Fly drosofila, certo? mutants for several different components of the Toll pathway completely ablate the differences in gene expression between males and females. In D. melanogaster, females present lower resistance to the bacterium Providencia rettgeri and the Toll pathway plays a key role in the sexual dimorphism in resistance to infection (72). The same is not observed in IMD mutants, where the differences persist. In contrast to this, we observed the same expression pattern in male and female adult from insect vectors for almost all genes derived from the Toll, IMD and Jak-STAT immune pathways.
Using whole body insects. gives a general idea of the expression pattern of the immune genes, we lose the differences that are tissue-specific between sexes. However, we know that insects are capable to activate first a local and later a systemic immune response based on interorgan molecular communications (73). Our data suggest that male immune genes are transcriptionally more active and maybe help A. aegypti males to clear bacterial infections more effectively, but further experiments should be performed to identify tissue-specific differences.
Based on our results and previous work, we can observe a lack of universal or even taxonomic sex differences in immunocompetence. We believe that the life-history strategies between the sexes within a single species may be decisive in directing the dimorphism of the immune system. Here we show that the feeding habit can influence the differences in the humoral immune responses between sexes in insect vectors. Furthermore, dimorphic features may be present not only on systemic humoral immunity, but also occur on epithelial and cellular immune responses, and differ between distinct immune compartments. More work is also needed to understand the interaction of the dimorphisms involved with microbiome sex differences that likely interact with dietary choices.
Data Availability Statement
The original contributions presented in the study are included in the article/Supplementary Material. Further inquiries can be directed to the corresponding authors.
Author Contributions
Experiments were designed by ABB, ACB, AP, PO, PP, YT-C, and MS; carried out by ABB, ACB, AP, EB, DG, VB-R, and MK; and analyzed by ABB, ACB, AP, EB, DG, VB-R, and MK. ABB, ACB, YT-C, and MS wrote the paper. All authors contributed to the article and approved the submitted version.
Funding
This work was supported by HHMI, Pronex, CNPq, Faperj and INCT-EM.
Conflict of Interest
The authors declare that the research was conducted in the absence of any commercial or financial relationships that could be construed as a potential conflict of interest.
Publisher’s Note
All claims expressed in this article are solely those of the authors and do not necessarily represent those of their affiliated organizations, or those of the publisher, the editors and the reviewers. Any product that may be evaluated in this article, or claim that may be made by its manufacturer, is not guaranteed or endorsed by the publisher.
Supplementary Material
The Supplementary Material for this article can be found online at: https://www.frontiersin.org/articles/10.3389/fitd.2022.847109/full#supplementary-material
Supplementary Figure 1 | Cultivable microbiota of the intestinal tract of Aedes aegypti females (A) and males (B) adults on agar plates.
Supplementary Figure 2 | Cultivable microbiota of the intestinal tract of Aedes aegypti females and males. (A, B) Females treated with PBS 1x. (C, D) Females treated with antibiotics (Ab). E and F - Males treated with PBS 1x. (C, D) Males treated with antibiotics (Ab).
Supplementary Table 1 | Primers used for immune-related gene expression analysis and bacteria quantification in Anopheles aquasalis, Rhodnius prolixus and Lutzomyia longipalpis.
References
1. Lemaitre B, Hoffmann J. The Host Defense of Drosophila Melanogaster. Annu Rev Immunol (2007) 25:697–743. doi: 10.1146/annurev.immunol.25.022106.141615
2. Cooper D, Eleftherianos I. Memory and Specificity in the Insect Immune System: Current Perspectives and Future Challenges. Front Immunol (2017). doi: 10.3389/fimmu.2017.00539
3. Samuel GH, Adelman ZN, Myles KM. Antiviral Immunity and Virus-Mediated Antagonism in Disease Vector Mosquitoes. Trends Microbiol (2018) 26:447–61. doi: 10.1016/j.tim.2017.12.005
4. Barletta ABF, Alves LR, Nascimento Silva MCL, Sim S, Dimopoulos G, Liechocki S, et al. Emerging Role of Lipid Droplets in Aedes Aegypti Immune Response Against Bacteria and Dengue Virus. Sci Rep (2016) 6:19928. doi: 10.1038/srep19928
5. Barletta ABF, Trisnadi N, Ramirez JL, Barillas-Mury C. Mosquito Midgut Prostaglandin Release Establishes Systemic Immune Priming. iScience (2019) 19:54–62. doi: 10.1016/j.isci.2019.07.012
6. Cansado-Utrilla C, Zhao SY, McCall PJ, Coon KL, Hughes GL. The Microbiome and Mosquito Vectorial Capacity: Rich Potential for Discovery and Translation. Microbiome (2021) 9:111. doi: 10.1186/s40168-021-01073-2
7. Bahia AC, Kubota MS, Tempone AJ, Araújo HRC, Guedes BAM, Orfanó AS, et al. The JAK-STAT Pathway Controls Plasmodium Vivax Load in Early Stages of Anopheles Aquasalis Infection. PloS Neglect Trop Dis (2011) 5(11):e1317. doi: 10.1371/journal.pntd.0001317
8. Bahia AC, Kubota MS, Souza-Neto JA, Koerich LB, Barletta AB, Araújo HRC, et al. An Anopheles Aquasalis GATA Factor Serpent is Required for Immunity Against Plasmodium and Bacteria. PloS Neglect Trop Dis (2018) 12:1–16. doi: 10.1371/journal.pntd.0006785
9. Santana RAG, Oliveira MC, Cabral I, Junior RCAS, de Sousa DRT, Ferreira L, et al. Anopheles Aquasalis Transcriptome Reveals Autophagic Responses to Plasmodium Vivax Midgut Invasion. Parasites Vectors (2019) 12:261. doi: 10.1186/s13071-019-3506-8
10. Bahia AC, Kubota MS, Tempone AJ, Pinheiro WD, Tadei WP, Secundino NFC, et al. Anopheles Aquasalis Infected by Plasmodium Vivax Displays Unique Gene Expression Profiles When Compared to Other Malaria Vectors and Plasmodia. PloS One (2010) 5(3):e9795. doi: 10.1371/journal.pone.0009795
11. Dillon RJ, Ivens AC, Churcher C, Holroyd N, Quail MA, Rogers ME, et al. Analysis of ESTs From Lutzomyia Longipalpis Sand Flies and Their Contribution Toward Understanding the Insect–Parasite Relationship. Genomics (2006) 88:831–40. doi: 10.1016/j.ygeno.2006.06.011
12. Pitaluga AN, Beteille V, Lobo AR, Ortigão-Farias JR, Dávila AMR, Souza AA, et al. EST Sequencing of Blood-Fed and Leishmania-Infected Midgut of Lutzomyia Longipalpis, the Principal Visceral Leishmaniasis Vector in the Americas. Mol Genet Genomics (2009) 282:307–17. doi: 10.1007/s00438-009-0466-2
13. Telleria EL, Sant’Anna MRV, Ortigão-Farias JR, Pitaluga AN, Dillon VM, Bates PA, et al. Caspar-Like Gene Depletion Reduces Leishmania Infection in Sand Fly Host Lutzomyia Longipalpis. J Biol Chem (2012) 287:12985–93. doi: 10.1074/jbc.M111.331561
14. Tinoco-Nunes B, Telleria EL, da Silva-Neves M, Marques C, Azevedo-Brito DA, Pitaluga AN, et al. The Sandfly Lutzomyia Longipalpis LL5 Embryonic Cell Line has Active Toll and Imd Pathways and Shows Immune Responses to Bacteria, Yeast and Leishmania. Parasites Vectors (2016) 9:222. doi: 10.1186/s13071-016-1507-4
15. Di-Blasi T, Telleria EL, Marques C, Couto R, de M, Silva-Neves M, et al. Lutzomyia Longipalpis TGF-β Has a Role in Leishmania Infantum Chagasi Survival in the Vector. Front Cell Infect Microbiol (2019) 9:71. doi: 10.3389/fcimb.2019.00071
16. Telleria EL, Tinoco-Nunes B, Leštinová T, de Avellar LM, Tempone AJ, Pitaluga AN, et al. Lutzomyia Longipalpis Antimicrobial Peptides: Differential Expression During Development and Potential Involvement in Vector Interaction With Microbiota and Leishmania. Microorganisms (2021) 9:1271. doi: 10.3390/microorganisms9061271
17. Azambuja P, Garcia ES, Waniek PJ, Vieira CS, Figueiredo MB, Gonzalez MS, et al. Rhodnius Prolixus: From Physiology by Wigglesworth to Recent Studies of Immune System Modulation by Trypanosoma Cruzi and Trypanosoma Rangeli. J Insect Physiol (2017) 97:45–65. doi: 10.1016/j.jinsphys.2016.11.006
18. Carmona-Peña SP, Contreras-Garduño J, Castro DP, Manjarrez J, Vázquez-Chagoyán JC. The Innate Immune Response of Triatomines Against Trypanosoma Cruzi and Trypanosoma Rangeli With an Unresolved Question: Do Triatomines Have Immune Memory? Acta Tropica (2021) 224:106108. doi: 10.1016/j.actatropica.2021.106108
19. Klein SL, Flanagan KL. Sex Differences in Immune Responses. Nat Rev Immunol (2016) 16:626–38. doi: 10.1038/nri.2016.90
20. Vincent CM, Dionne MS. Disparate Regulation of IMD Signaling Drives Sex Differences in Infection Pathology in Drosophila Melanogaster. Proc Natl Acad Sci (2021) 118:e2026554118. doi: 10.1073/pnas.2026554118
21. Fairweather D, Frisancho-Kiss S, Rose NR. Sex Differences in Autoimmune Disease From a Pathological Perspective. Am J Pathol (2008) 173(3):600–9. doi: 10.2353/ajpath.2008.071008
22. vom Steeg LG, Klein SL. SeXX Matters in Infectious Disease Pathogenesis. PloS Pathog (2016) 12(2):e1005374. doi: 10.1371/journal.ppat.1005374
23. Zuk M. Reproductive Strategies and Disease Susceptibility: An Evolutionary Viewpoint. Parasitol Today (1990) 6(7):231–3. doi: 10.1016/0169-4758(90)90202-F
24. Sheldon BC, Verhulst S. Ecological Immunology: Costly Parasite Defences and Trade-Offs in Evolutionary Ecology. Trends Ecol Evol (1996) 11:317–21. doi: 10.1016/0169-5347(96)10039-2
25. Zuk M, Stoehr AM. Immune Defense and Host Life History. Am Nat (2002) 160(4):S9–S22. doi: 10.1086/342131
26. Nunn CL, Lindenfors P, Pursall ER, Rolff J. On Sexual Dimorphism in Immune Function. Philos Trans R Soc B: Biol Sci (2009) 364(1513):61–9. doi: 10.1098/rstb.2008.0148
27. Restif O, Amos W. The Evolution of Sex-Specific Immune Defences. Proc R Soc B: Biol Sci (2010) 277:2247–55. doi: 10.1098/rspb.2010.0188
28. Bacelar FS, White A, Boots M. Life History and Mating Systems Select for Male Biased Parasitism Mediated Through Natural Selection and Ecological Feedbacks. J Theor Biol (2011) 269:131–7. doi: 10.1016/j.jtbi.2010.10.004
29. Lawyer P, Killick-Kendrick M, Rowland T, Rowton E, Volf P. Laboratory Colonization and Mass Rearing of Phlebotomine Sand Flies (Diptera, Psychodidae). Parasite (2017) 24:42. doi: 10.1051/parasite/2017041
30. Dias F, de A, Guerra B, Vieira LR, Perdomo HD, Gandara ACP, et al. Monitoring of the Parasite Load in the Digestive Tract of Rhodnius Prolixus by Combined qPCR Analysis and Imaging Techniques Provides New Insights Into the Trypanosome Life Cycle. PloS Neglect Trop Dis (2015) 9(10):e0004186. doi: 10.1371/journal.pntd.0004186
31. Fay RW, HB M. A Mechanical Device for Separating the Developmental Stages, Sexes and Species of Mosquitoes. 144–147: Mosq News (1959).
32. Dong Y, Manfredini F, Dimopoulos G. Implication of the Mosquito Midgut Microbiota in the Defense Against Malaria Parasites. PloS Pathog (2009) 5(5):e1000423. doi: 10.1371/journal.ppat.1000423
33. Corby-Harris V, Drexler A, Watkins de Jong L, Antonova Y, Pakpour N, Ziegler R, et al. Activation of Akt Signaling Reduces the Prevalence and Intensity of Malaria Parasite Infection and Lifespan in Anopheles Stephensi Mosquitoes. PloS Pathog (2010) 6:e1001003. doi: 10.1371/journal.ppat.1001003
34. Gentile C, Lima JBP, Peixoto AA. Isolation of a Fragment Homologous to the Rp49 Constitutive Gene of Drosophila in the Neotropical Malaria Vector Anopheles Aquasalis (Diptera: Culicidae). Memorias do Instituto Oswaldo Cruz (2005) 100(6):545–7. doi: 10.1590/S0074-02762005000600008
35. Meireles-Filho AC, Amoretty PR, Souza NA, Kyriacou CP, Peixoto AA. Rhythmic Expression of the Cycle Gene in a Hematophagous Insect Vector. BMC Mol Biol (2006) 7:38. doi: 10.1186/1471-2199-7-38
36. Majerowicz D, Alves-Bezerra M, Logullo R, Fonseca-de-Souza AL, Meyer-Fernandes JR, Braz GRC, et al. Looking for Reference Gensolation of a Fragment Homologous to the Rp49 Conin Rhodnius Prolixus (Hemiptera: Reduviidae). Insect Mol Biol (2011) 20:713–22. doi: 10.1111/j.1365-2583.2011.01101.x
37. Livak KJ, Schmittgen TD. Analysis of Relative Gene Expression Data Using Real-Time Quantitative PCR and the 2–ΔΔct Method. Methods (2001) 25:402–8. doi: 10.1006/meth.2001.1262
38. Pfaffl MW. A New Mathematical Model for Relative Quantification in Real-Time RT-PCR. Nucleic Acids Res (2001) 29:45e–45. doi: 10.1093/nar/29.9.e45
39. Telleria EL, Azevedo-Brito DA, Kykalová B, Tinoco-Nunes B, Pitaluga AN, Volf P, et al. Leishmania Infantum Infection Modulates the Jak-STAT Pathway in Lutzomyia Longipalpis LL5 Embryonic Cells and Adult Females, and Affects Parasite Growth in the Sand Fly. Front Trop Dis (2021) 2:747820. doi: 10.3389/fitd.2021.747820
40. Berni M, Fontenele MR, Tobias-Santos V, Caceres-Rodrigues A, Mury FB, Vionette-do-Amaral R, et al. Toll Signals Regulate Dorsal-Ventral Patterning and Anterior-Posterior Placement of the Embryo in the Hemipteran Rhodnius Prolixus. EvoDevo (2014) 5:38. doi: 10.1186/2041-9139-5-38
41. Vieira CS, Mattos DP, Waniek PJ, Santangelo JM, Figueiredo MB, Gumiel M, et al. Rhodnius Prolixus Interaction With Trypanosoma Rangeli: Modulation of the Immune System and Microbiota Population. Parasites Vectors (2015) 8:135. doi: 10.1186/s13071-015-0736-2
42. Lane D. 16s/23s rRNA Sequencing. In: Nucleic Acid Techniques in Bacterial Systematics. In: Nucleic Acid Technique in Bacterial Systematics. New York:John Wiley and Sons (1991), 115–75.
43. Oliveira JHM, Gonçalves RLS, Lara FA, Dias FA, Gandara ACP, Menna-Barreto RFS, et al. Blood Meal-Derived Heme Decreases ROS Levels in the Midgut of Aedes Aegypti and Allows Proliferation of Intestinal Microbiota. PloS Pathog (2011) 7(3):e1001320. doi: 10.1371/journal.ppat.1001320
44. Belmonte RL, Corbally MK, Duneau DF, Regan JC. Sexual Dimorphisms in Innate Immunity and Responses to Infection in Drosophila Melanogaster. Front Immunol (2020) 10:3075. doi: 10.3389/fimmu.2019.03075
45. Díaz S, Camargo C, Avila FW. Characterization of the Reproductive Tract Bacterial Microbiota of Virgin, Mated, and Blood-Fed Aedes Aegypti and Aedes Albopictus Females. Parasites Vectors (2021) 14:592. doi: 10.1186/s13071-021-05093-7
46. Kulkarni A, Pandey A, Trainor P, Carlisle S, Chhilar JS, Yu W, et al. Trained Immunity in Anopheles Gambiae: Antibacterial Immunity Is Enhanced by Priming via Sugar Meal Supplemented With a Single Gut Symbiotic Bacterial Strain. Front Microbiol (2021) 12:649213. doi: 10.3389/fmicb.2021.649213
47. Ramirez JL, Souza-Neto J, Torres Cosme R, Rovira J, Ortiz A, Pascale JM, et al. Reciprocal Tripartite Interactions Between the Aedes Aegypti Midgut Microbiota, Innate Immune System and Dengue Virus Influences Vector Competence. PloS Neglect Trop Dis (2012) 6:e1561. doi: 10.1371/journal.pntd.0001561
48. Sharma P, Rani J, Chauhan C, Kumari S, Tevatiya S, das De T, et al. Altered Gut Microbiota and Immunity Defines Plasmodium Vivax Survival in Anopheles Stephensi. Front Immunol (2020) 11:609. doi: 10.3389/fimmu.2020.00609
49. Alfano N, Tagliapietra V, Rosso F, Manica M, Arnoldi D, Pindo M, et al. Changes in Microbiota Across Developmental Stages of Aedes Koreicus, an Invasive Mosquito Vector in Europe: Indications for Microbiota-Based Control Strategies. Front Microbiol (2019) 10:2832. doi: 10.3389/fmicb.2019.02832
50. Barletta ABF, Nascimento-Silva MCL, Talyuli OAC, Oliveira JHM, Pereira LOR, Oliveira PL, et al. Microbiota Activates IMD Pathway and Limits Sindbis Infection in Aedes Aegypti. Parasites Vectors (2017) 10:103. doi: 10.1186/s13071-017-2040-9
51. Zhang R, Zhu Y, Pang X, Xiao X, Zhang R, Cheng G. Regulation of Antimicrobial Peptides in Aedes Aegypti Aag2 Cells. Front Cell Infect Microbiol (2017) 7:22. doi: 10.3389/fcimb.2017.00022
52. Guégan M, Minard G, Tran F-H, Tran Van V, Dubost A, Valiente Moro C. Short-Term Impacts of Anthropogenic Stressors on Aedes Albopictus Mosquito Vector Microbiota. FEMS Microbiol Ecol (2018) 94:12. doi: 10.1093/femsec/fiy188
53. González-González A, Wayne ML. Immunopathology and Immune Homeostasis During Viral Infection in Insects. Adv Virus Res (2020) 107:285–314. doi: 10.1016/bs.aivir.2020.06.001.
54. Oliveira JH, Bahia AC, Vale PF. How are Arbovirus Vectors Able to Tolerate Infection? Dev Comp Immunol (2020) 103:103514. doi: 10.1016/j.dci.2019.103514
55. Danis-Lozano R, Díaz-González EE, Malo-García IR, Rodríguez MH, Ramos-Castañeda J, Juárez-Palma L, et al. Vertical Transmission of Dengue Virus in Aedes Aegypti and its Role in the Epidemiological Persistence of Dengue in Central and Southern Mexico. Trop Med Int Health (2019) 24:1311–9. doi: 10.1111/tmi.13306
56. Bahia AC, Oliveira JHM, Kubota MS, Araújo HRC, Lima JBP, Ríos-Velásquez CM, et al. The Role of Reactive Oxygen Species in Anopheles Aquasalis Response to Plasmodium Vivax Infection. PloS One (2013) 8:1–10. doi: 10.1371/journal.pone.0057014
57. Briegel H, Kaiser C. Life-Span of Mosquitoes (Culicidae, Diptera) Under Laboratory Conditions. Gerontology (1973) 19:240–9. doi: 10.1159/000211976
58. Samakovlis C, Kylsten P, Kimbrell DA, Engström A, Hultmark D. The Andropin Gene and its Product, a Male-Specific Antibacterial Peptide in Drosophila Melanogaster. EMBO J (1991) 10:163–9. doi: 10.1002/j.1460-2075.1991.tb07932.x
59. Lung O, Kuo L, Wolfner MF. Drosophila Males Transfer Antibacterial Proteins From Their Accessory Gland and Ejaculatory Duct to Their Mates. J Insect Physiol (2001) 47:617–22. doi: 10.1016/S0022-1910(00)00151-7
60. Ferrandon D. A Drosomycin-GFP Reporter Transgene Reveals a Local Immune Response in Drosophila That is Not Dependent on the Toll Pathway. EMBO J (1998) 17:1217–27. doi: 10.1093/emboj/17.5.1217
61. Hamilton WD, Zuk M. Heritable True Fitness and Bright Birds: A Role for Parasites? Science (1982) 218:384–7. doi: 10.1126/science.7123238
62. Kelly CD, Stoehr AM, Nunn C, Smyth KN, Prokop ZM. Sexual Dimorphism in Immunity Across Animals: A Meta-Analysis. Ecol Lett (2018) 21(12):1885–94. doi: 10.1111/ele.13164
63. De Loof A, Huybrechts R. Insects Do Not Have Sex Hormones”: A Myth? Gen Comp Endocrinol (1998) 111:245–60. doi: 10.1006/gcen.1998.7101
64. Sheridan LAD, Poulin R, Ward DF, Zuk M. Sex Differences in Parasitic Infections Among Arthropod Hosts: Is There a Male Bias? Oikos (2000) 88:327–34. doi: 10.1034/j.1600-0706.2000.880211.x
65. Fanson BG, Fanson KV, Taylor PW. Sex Differences in Insect Immune Function: A Consequence of Diet Choice? Evol Ecol (2013) 27:937–47. doi: 10.1007/s10682-013-9638-y
66. Shahrestani P, Chambers M, Vandenberg J, Garcia K, Malaret G, Chowdhury P, et al. Sexual Dimorphism in Drosophila Melanogaster Survival of Beauveria Bassiana Infection Depends on Core Immune Signaling. Sci Rep (2018) 8:12501. doi: 10.1038/s41598-018-30527-1
67. Vieira CS, Waniek PJ, Mattos DP, Castro DP, Mello CB, Ratcliffe NA, et al. Humoral Responses in Rhodnius Prolixus: Bacterial Feeding Induces Differential Patterns of Antibacterial Activity and Enhances mRNA Levels of Antimicrobial Peptides in the Midgut. Parasites Vectors (2014) 7:232. doi: 10.1186/1756-3305-7-232
68. Zuk M, Simmons LW, Rotenberry JT, Stoehr AM. Sex Differences in Immunity in Two Species of Field Crickets. Can J Zool (2004) 82:627–34. doi: 10.1139/z04-032
69. McKean KA, Nunney L. Bateman’s PRINCIPLE AND IMMUNITY: PHENOTYPICALLY PLASTIC REPRODUCTIVE STRATEGIES PREDICT CHANGES IN IMMUNOLOGICAL SEX DIFFERENCES. Evolution (2005) 59(7):1510–7. doi: 10.1554/04-657
70. Chowdhury M, Li CF, He Z, Lu Y, Liu XS, Wang YF, et al. Toll Family Members Bind Multiple Spätzle Proteins and Activate Antimicrobial Peptide Gene Expression in Drosophila. J Biol Chem (2019) 294(26):10181. doi: 10.1074/jbc.RA118.006804
71. Regan JC, Khericha M, Dobson AJ, Bolukbasi E, Rattanavirotkul N, Partridge L. Sex Difference in Pathology of the Ageing Gut Mediates the Greater Response of Female Lifespan to Dietary Restriction. eLife (2016) 5:e10956. doi: 10.7554/eLife.10956
72. Duneau DF, Kondolf HC, Im JH, Ortiz GA, Chow C, Fox MA, et al. The Toll Pathway Underlies Host Sexual Dimorphism in Resistance to Both Gram-Negative and Gram-Positive Bacteria in Mated Drosophila. BMC Biol (2017) 15(1):124. doi: 10.1186/s12915-017-0466-3
Keywords: immunity, sexual dimorphism, insect vectors, mosquitoes, sandflies, kissing bugs
Citation: Barletta Ferreira AB, Bahia AC, Pitaluga AN, Barros E, Gama dos Santos D, Bottino-Rojas V, Kubota MS, Oliveira PL, Pimenta PFP, Traub-Csekö YM and Sorgine MHF (2022) Sexual Dimorphism in Immune Responses and Infection Resistance in Aedes aegypti and Other Hematophagous Insect Vectors. Front. Trop. Dis 3:847109. doi: 10.3389/fitd.2022.847109
Received: 01 January 2022; Accepted: 15 February 2022;
Published: 18 March 2022.
Edited by:
Paul O. Mireji, Kenya Agricultural and Livestock Research Organization, KenyaReviewed by:
Rajnikant Dixit, National Institute of Malaria Research (ICMR), IndiaFelipe Arley Costa Pessoa, Instituto Leônidas & Maria Deane (ILMD) (FIOCRUZ), Brazil
Copyright © 2022 Barletta Ferreira, Bahia, Pitaluga, Barros, Gama dos Santos, Bottino-Rojas, Kubota, Oliveira, Pimenta, Traub-Csekö and Sorgine. This is an open-access article distributed under the terms of the Creative Commons Attribution License (CC BY). The use, distribution or reproduction in other forums is permitted, provided the original author(s) and the copyright owner(s) are credited and that the original publication in this journal is cited, in accordance with accepted academic practice. No use, distribution or reproduction is permitted which does not comply with these terms.
*Correspondence: Ana Beatriz Barletta Ferreira, anabeatriz.barlettaferreira@nih.gov; Ana Cristina Bahia, anabahia@biof.ufrj.br; Yara Maria Traub-Csekö, ytraub@ioc.fiocruz.br; Marcos Henrique Ferreira Sorgine, sorgine@bioqmed.ufrj.br
†Present address: Vanessa Bottino-Rojas, Department of Microbiology and Molecular Genetics, University of California, Irvine, Irvine, CA, United States
Ana Beatriz Barletta Ferreira, Laboratory of Malaria and Vector Research, National Institute of Allergy and Infectious Diseases, National Institutes of Health, Rockville, MD, United States
‡These authors have contributed equally to this work