- 1Laboratório de Biologia Molecular de Parasitas e Vetores, Instituto Oswaldo Cruz, Oswaldo Cruz Foundation (FIOCRUZ), Rio de Janeiro, Brazil
- 2Charles University, Faculty of Science, Department of Parasitology, Prague, Czechia
Phlebotomine sand flies (Diptera, Psychodidae) belonging to the Lutzomyia genus transmit zoonoses in the New World. Lutzomyia longipalpis is the main vector of Leishmania infantum, which is the causative agent of visceral leishmaniasis in Brazil. To identify key molecular aspects involved in the interaction between vector and pathogens and contribute to developing disease transmission controls, we investigated the sand fly innate immunity mediated by the Janus kinase/signal transducer and activator of transcription (Jak-STAT) pathway in response to L. infantum infection. We used two study models: L. longipalpis LL5 embryonic cells co-cultured with L. infantum and sand fly females artificially infected with the parasite. We used qPCR to follow the L. longipalpis gene expression of molecules involved in the Jak-STAT pathway. Also, we modulated the Jak-STAT mediated immune response to understand its role in Leishmania parasite infection. For that, we used RNAi to silence the pathway regulators, protein inhibitor of activated STATs (PIAS) in LL5 cells, and STAT in adult females. In addition, the pathway suppression effect on parasite development within the vector was assessed by light microscopy in late-phase infection. The silencing of the repressor PIAS in LL5 cells led to a moderate increase in a protein tyrosine phosphatase 61F (PTP61F) expression. It suggests a compensatory regulation between these two repressors. L. infantum co-culture with LL5 cells upregulated repressors PIAS, suppressor of cytokine signaling (SOCS), and PTP61F. It also downmodulated virus-induced RNA-1 (VIR-1), a pathway effector, indicating that the parasite could repress the Jak-STAT pathway in LL5 cells. In Leishmania-infected L. longipalpis females, STAT and the antimicrobial peptide attacin were downregulated on the third day post-infection, suggesting a correlation that favors the parasite survival at the end of blood digestion in the sand fly. The antibiotic treatment of infected females showed that the reduction of gut bacteria had little effect on the Jak-STAT pathway regulation. STAT gene silencing mediated by RNAi reduced the expression of inducible nitric oxide synthase (iNOS) and favored Leishmania growth in sand flies on the first day post-infection. These results indicate that STAT participated in the iNOS regulation with subsequent effect on parasite survival.
1 Introduction
Phlebotomine sand flies (Diptera, Psychodidae) are vectors of a group of parasitic diseases classified as cutaneous or visceral leishmaniases, which are transmitted by the bite of an infected female sand fly. These diseases are caused by protozoan parasites belonging to the Leishmania genus, with over 20 species identified as infective to humans and endemic to 98 countries and territories distributed from temperate to tropical regions of the globe (1). Lutzomyia is the most important genus in the American continent due to its wide distribution and diversity of species (reviewed in 2). This genus includes Lutzomyia longipalpis, the main vector of visceral leishmaniasis in Brazil, caused by Leishmania infantum (syn. L. chagasi) (reviewed in 3).
To prevent the spread of vector-borne diseases, strategies such as genetic manipulation of the vector or paratransgenesis, as well as transmission-blocking vaccines, became promising alternatives to reduce vector competence in transmitting pathogens [reviewed in (4, 5)]. We are interested in understanding how the L. longipalpis immune system responds to parasitic challenges, therefore contributing to developing novel molecular-based tools to control the Leishmania cycle in the insect.
We have previously identified L. longipalpis components of the sand fly innate immunity regulated by the Toll and immune deficiency (IMD) pathways that ultimately produce antimicrobial peptides (AMPs) such as attacin, cecropin, and defensins (6–8). Both pathways were active in L. longipalpis LL5 embryonic cells (6, 9). In addition, the activation of the IMD pathway in the female sand fly can reduce the parasite survival in the insect, evidencing the involvement of the insect immunity in parasite control (8). Nevertheless, innate immunity is multi-faceted. Besides AMPs expression mediated by the Toll and IMD pathways, the Janus kinase/signal transducer and activator of transcription (Jak-STAT) pathway regulate the expression of cytokines involved in cell growth, differentiation, and apoptosis. It also regulates other effector molecules that play important roles in the immune response (reviewed in 10–12). Briefly, the pathway is activated by the unpaired (Upd) family of ligands that bind to the transmembrane receptor domeless (Dome), which is followed by the recruitment and transphosphorylation of associated Jak tyrosine kinase Hopscotch (Hop). These events result in the phosphorylation and dimerization of the transcription factor STAT, which is then translocated to the cell nucleus for target genes transcription. This cascade is regulated at different levels by repressor molecules, including the suppressors of cytokine signaling (SOCS) family that bind to Dome receptor and inhibit STAT recruitment. Also, protein tyrosine phosphatases (PTPs) dephosphorylate STAT preventing its translocation to the cell nucleus. In addition, the protein inhibitor of activated STATs (PIAS) inactivates STAT through direct binding [reviewed in (10–12)]. Graphical representations of the Jak-STAT pathway in insects can be found in several reviews (10, 13, 14).
Among STAT-targeted genes, the AMP attacin and the virus-induced RNA-1 (VIR-1) are effector molecules associated with the Jak-STAT pathway in Drosophila (12, 15). Nevertheless, attacins in Drosophila are mostly regulated by the IMD pathway (16). Attacins are primarily active against Gram-negative bacteria (17) but also have antiparasitic activity (18). VIR-1 is induced by a viral infection such as the Drosophila C virus (DCV) (19). In addition, the Jak-STAT pathway can regulate the inducible nitric oxide synthase (iNOS) (reviewed in 20, 21), with a consequent increase of nitric oxide production and deleterious effect on microorganisms (reviewed in 22). Interestingly, upon Plasmodium vivax infection, STAT expression was increased in the malaria vector Anopheles aquasalis, and the silencing of STAT caused the increase in oocysts numbers in the vector (23). Also, the increased nitric oxide levels in Anopheles stephensi hemolymph are important to control the development of Plasmodium berghei (24). These findings indicate the potential role of the Jak-STAT pathway and its downstream effects in balancing parasite infection.
Since the Jak-STAT pathway regulates the expression of molecules involved in immune response, we investigated the gene expression of Jak-STAT-related molecules in L. longipalpis LL5 embryonic cells and adult females challenged by L. infantum. Because the gut microbiota can influence the immune response, we used the strategy of depleting the commensal gut bacteria with antibiotics to investigate its impact on the expression of Jak-STAT-related genes in Leishmania-infected females. In addition, to study the role of the L. longipalpis STAT, a putative pathway transcription factor, we suppressed its expression by using RNAi-mediated gene silencing. We followed the outcome in sand fly Jak-STAT-related gene expression, bacterial and parasite detection, and parasite development in the vector’s gut.
2 Methods
2.1 Jak-STAT Related Gene Sequences
Amino acid sequences of the Jak-STAT pathway-related molecules were selected from available databases of Drosophila melanogaster (25), Anopheles gambiae, Aedes aegypti mosquitoes (26), and NCBI (27). They were used as a query on tblastn and blastp search against the L. longipalpis database available in VectorBase portal (26). The query sequences and their corresponding similarity rates with the top L. longipalpis blastp hits are shown in Supplementary Tables 1–7. A selection of L. longipalpis nucleotide sequence hits with the highest identity rates with mosquitoes sequences were retrieved and used in blastx search against the NCBI database (27) to assess their similarity to previously characterized sequences.
In addition, L. longipalpis translated sequences were analyzed using the InterPro Classification of Protein Families 81.0 tool (28) to search for signature domains. Similarities of L. longipalpis amino acid sequences with other insects were assessed by MUSCLE multiple sequence alignment (29) built-in Geneious 7.1.9 software (Biomatters, New Zealand). Cladograms were created using MEGA-X software (30), with the Maximum Likelihood method with a bootstrap of 100 replicates to model evolutionary rate differences among sites. Substitution models were defined for each gene using the MEGA-X tool according to the lowest Bayesian Information Criterion (BIC) score. For PIAS, Le and Gascuel’s model was used with discrete Gamma distribution, considering part of sites are evolutionarily invariable (LG+G+I). For SOCS, PTP61F, iNOS, and DUOX, we used Le and Gascuel’s model with discrete Gamma distribution (LG+G). For STAT, we used Jones-Taylor-Thornton’s model with discrete Gamma distribution (JTT+G). Finally, for VIR-1, we used Whelan and Goldman’s model with discrete Gamma distribution (WAG+G).
Because the L. longipalpis genes identification was based on similarity levels with previously characterized genes in other insect model species, we highlight that their sequences correspond to putative genes.
For relative expression studies, gene-specific oligonucleotides were designed using Primer3 online tool (31) on the open reading frame sequence for further gene expression analysis (Table 1).
2.2 Cell Cultures
L. longipalpis embryonic LL5 cells were grown at 29°C in L-15 medium (Sigma, USA) supplemented with 10% fetal bovine serum (Laborclin, Brazil), 10% tryptose phosphate broth, and 1% antibiotics (penicillin 100 U/mL and streptomycin 100 mg/mL, Sigma). For experimental procedures, LL5 cells were seeded in 24 well flat-bottom plates, and after overnight growth, a new supplemented L-15 medium was added and used for subsequent assays.
L. infantum (MHOM/BR/1974/PP75) was cultured in M199 medium (Sigma), adjusted to pH 7.4, and supplemented with 10% fetal bovine serum. For experimental procedures, parasites were harvested at the exponential growth phase and washed with PBS.
2.3 L. longipalpis Rearing and Treatment With Antibiotics
L. longipalpis females were obtained from colonized sand flies originally collected in Jacobina, BA, Brazil, and kept at temperatures between 24-28 and 70-80% relative humidity following standard insectary conditions (39). Adult insects were fed on 50-70% sucrose ad libitum. Females were blood-fed on anesthetized hamsters or mice once a week for colony maintenance. For bacterial depletion, sand flies were separated and kept with sucrose solution containing a mixture of antibiotics at a final concentration of 100 U/mL penicillin-streptomycin and 10 μg/mL gentamicin immediately after emergence from pupae until needed.
2.4 Double-Stranded RNA Synthesis
DNA templates were amplified from L. longipalpis cDNA obtained from insects kept under colony conditions for dsRNA in vitro synthesis.
PIAS template was amplified by PCR in two rounds. In the first round, gene-specific primers coupled to an adapter sequence on the 5′ end (dsPIAS-F and dsPIAS-R, Table 1) were used to amplify the partial coding sequence of the target gene. This first round product was subsequently used as a template in a second PCR containing primers with the adapter and T7 promoter sequences (T7+adaptor, Table 1). On both rounds, PCR conditions were: 95°C for 3 min; 35 cycles of 95°C for 30 sec, 57°C for 45 sec, and 72°C for 45 sec; followed by 72°C for 7 min.
The putative STAT template was amplified by PCR using gene-specific primers directly coupled to T7 promoter sequence (dsSTAT-F and dsSTAT-R, Table 1) in a touchdown PCR as follows: 95°C for 3 min; 16 cycles of 95°C for 45 sec, 68 to 50°C (progressively decreasing 1°C per cycle) for 45 sec, and 72°C for 45 sec; 26 cycles of 95°C for 45 sec, 50°C for 45 sec, and 72°C for 45 sec; 72°C for 3 min.
Up to 2 μg of these templates were used in a dsRNA synthesis reaction using MEGAscript RNAi kit (Invitrogen, USA) following the manufacturer’s instructions.
2.5 L. infantum Challenge and PIAS Gene Silencing in LL5
2.5.1 PIAS Silencing in LL5 Cells
For PIAS silencing, LL5 cells were transfected with a mixture of transfection agents containing 0.25 mL DharmaFECT (Thermo Fisher Scientific, USA), 23.25 mL DCCM medium, and 1.5 mL of PIAS dsRNA for a final concentration of 30 nM. As a control, cells were transfected with a non-related luciferase dsRNA (6). LL5 cells were maintained in this mixture for 16 h and then incubated for 12 h, 24 h, and 48 h at 30°C, before being resuspended in TRIzol reagent (Invitrogen).
2.5.2 L. infantum Challenge in LL5 Cells
For the Leishmania challenge, parasites were seeded to L-15 medium and added to LL5 cells at a microbe/cell ratio of 10 to 1. Non-challenged LL5 cells were used as control. Samples were collected at 24 h, 48 h, and 72 h post-challenge from three independent experiments by discharging the supernatant medium, washing the cells twice with PBS, adding 1 mL of TRIzol, and storing at -80°C for future RNA extraction.
2.6 STAT Gene Silencing and Artificial Feeding in L. longipalpis Females
2.6.1 STAT Gene Silencing in L. longipalpis Females
For the putative STAT gene silencing, STAT dsRNA was lyophilized and resuspended in nuclease-free H2O to 4.5 μg/μL final concentration. Females were microinjected intrathoracically with 32.2 nL of dsRNA using Nanoject II microinjector (Drummond, USA) (40). A non-related LacZ dsRNA was injected in negative control groups (41). For assays involving Leishmania infection, sand flies were artificially infected as described above on the following day after dsRNA microinjection and collected on subsequent days according to experimental design. For controlling gene silencing efficiency, injected flies were kept under colony conditions fed on sucrose and collected on three successive days post dsRNA microinjection.
2.6.2 Artificial Feeding in L. longipalpis Females
For artificial infection, females (3 to 6 days after emerging from pupae) were fed through chick skin membrane on inactivated New Zealand rabbit blood seeded with L. infantum (106 parasites/mL of blood), and control groups were fed on blood. Fully engorged females were separated and collected at 24 h, 48 h, 72 h, and 144 h post-feeding for RNA extraction and at 144 h for microscopy analysis. All samples were collected in pools of 10 whole body sand flies, or 15 dissected guts and corresponding carcasses (insect thorax and abdomen without gut) depending on experimental design.
2.7 RNA Extraction, cDNA Synthesis, and Gene Expression Analysis
According to each experimental design, samples were collected at different time points post challenges for total RNA extraction using TRIzol. Extracted RNA was incubated with RNase-free DNase I (Thermo Scientific) at 1 U/μg of total RNA for removing possible traces of DNA. Up to 1 μg of total RNA was used in reverse transcriptase reactions to produce cDNA using SuperScript III Reverse Transcriptase (Invitrogen). Protocols were followed according to each manufacturer’s instructions.
Gene expression was assessed by qPCR using cDNA templates, gene-specific primers (Table 1), and SYBR Green PCR Master Mix in a 7500 Real-Time PCR System (Applied Biosystems, USA) following manufacturer’s standard cycling conditions. The gene expression was calculated relative to a ribosomal protein (RP49) reference gene and expressed in fold change values in comparison to a control group (6) following the ΔΔCT method (42).
2.8 Leishmania Development in Sand Fly Guts
Sand flies were examined at 144 h post-infection by light microscopy for parasite load and localization. Guts were dissected in saline solution (NaCl 0.9%), covered with a thin glass slide, and examined under a 40x magnification objective lens. Parasite loads were estimated and classified as light (below 100 parasites), moderate (between 100 and 1000 parasites), or heavy infection (above 1000 parasites) (43). In addition, the localization of parasites in the gut (abdominal or thoracic gut, cardia, and colonized stomodeal valve) was recorded to evaluate the progress of Leishmania infection, following previously published methods (44).
2.9 Statistical Analysis
We used ordinary two-way ANOVA with Sidak’s correction for multiple comparisons test using GraphPad Prism software (version 6.07) (GraphPad Software Inc., USA) to test significant differences between experimental and control groups across various time points. The Sidak’s correction for multiple comparisons compute confidence intervals and significance based on adjusted P values. This method was applied to gene expression results obtained by qPCR and in infection estimation and localization results obtained by light microscopy observation.
3 Results
3.1 Gene Identification
We selected key molecules involved in the Jak-STAT pathway, such as regulators (repressors and transcription factor) and downstream effector molecules. We identified sequences similar to repressors PIAS, PTP61F, and SOCS, transcription factor STAT, and downstream related such as VIR-1 in the L. longipalpis transcript database available from VectorBase (26). Other related downstream genes such as attacin (6), iNOS (35), and DUOX (9) were identified in previous studies.
There was one PIAS ortholog (LLOJ002593) in the L. longipalpis database (Supplementary Table 1). The identification of signature domains in the L. longipalpis amino acid sequence (in silico predicted) showed that the sequence coding for the main pathway repressor contains the N-terminal PINIT (IPR023321) and Zinc finger (IPR004181) domains of the E3 SUMO-protein ligase PIAS1/PIAS3 family (Supplementary Figure 1A). The phylogenetic analysis showed that L. longipalpis PIAS sequence is distantly related to Bractrocera, Drosophila, Musca, and Stomoxys fly species and to Aedes and Anopheles mosquito species (Supplementary Figure 1B).
In the L. longipalpis database, there were three SOCS-like sequences (Supplementary Table 2). The sequence with the highest similarity with mosquitoes’ sequences (LLOJ002175) was selected for our future analyses. This L. longipalpis SOCS-like amino acid sequence contains the sarcoma homology 2 (SH2) (IPR000980) and SOCS box (IPR001496) domains (Supplementary Figure 2A). This sequence is closely related to Nyssomyia neivai and D. melanogaster SOCS16D sequences and form a cluster with A. aegypti and A. gambiae SOCS7. Another putative L. longipalpis SOCS sequences form clusters with D. melanogaster SOCS44A and SOCS5 from mosquitoes. A third SOCS-like sand fly sequence forms a cluster with D. melanogaster SOCS36E and SOCS6 sequences from mosquitoes (Supplementary Figure 2B).
The PTP61F amino acid sequence from L. longipalpis (LLOJ008161) was the only ortholog identified (Supplementary Table 3) and contains the PTP superfamily domain (IPR000242) (Supplementary Figure 3A). It formed a cluster with other PTP61F sequences from Aedes, Culex, and Anopheles species while separated from the Drosophila PTP61F cluster in the phylogenetic analysis (Supplementary Figure 3B).
Two putative STAT amino acid sequences were found through the blastp search against the L. longipalpis database (Supplementary Table 4). We chose one STAT-like sequence(LLOJ007428) to proceed with our analysis. This sequence contains STAT (IPR013801) and SH2 (IPR000980) domains forming the STAT family signature (Supplementary Figure 4A). In the phylogenetic analysis, the L. longipalpis STAT-like sequences form a separate branch from the STAT92E sequences from D. melanogaster and clusters containing STAT1 and STAT5 sequences identified in other organisms (Supplementary Figure 4B).
Among the Jak-STAT downstream molecules in L. longipalpis, the attacin sequence was previously identified. It contains the attacin family signature domain and is similar to other attacin sequences from N. neivai and Phlebotomus papatasi (41)..
Through the blastp search, we found one putative VIR-1 sequence in the L. longipalpis database (Supplementary Table 5). To date, VIR-1 has no specific domain associated with it. Nevertheless, the L. longipalpis VIR-1-like predicted amino acid sequence shares similarities with the Drosophila VIR-1 sequence. In our phylogenetic analysis, the sand fly VIR1-like sequence forms a branch distinct from the Drosophila cluster and is closer to the Culex quinquefasciatus VIR and other Aedes and Culex sequences not yet fully characterized (Supplementary Figure 5).
We also investigated the presence of signature domains in the sequences associated with the production of oxidative stress identified from previous studies (9, 33, 35, 45). The blast search for the L. longipalpis iNOS sequence in the VectorBase database (Supplementary Table 6) indicated one ortholog (LLOJ005465) that contains the N-terminal domain of the NOS superfamily (IPR036119). The phylogenetic analysis indicates that the L. longipalpis iNOS-like sequence forms a separate branch from the clusters containing Drosophila, Aedes, and Anopheles species (Supplementary Figure 6).
The DUOX amino acid sequence used in this study (LLOJ010494) showed high similarity with DUOX sequences from A. aegypti, A. gambiae, and D. melanogaster in our blastp search (Supplementary Table 7). This sequence contains the characteristic domains of the NOX-DUOX family including FAD-binding (IPR017927) and NADP binding (IPR013121) domains. The phylogenetic analysis showed that the L. longipalpis DUOX amino acid sequence formed a separate branch from the Drosophila, Lucilia, and Sarcophaga flies’ cluster. It was also separated from mosquito DUOX sequences (Supplementary Figure 7).
Multiple alignments and phylogenetic trees output files generated in the MEGA X software were deposited in a public repository: DOI: 10.6084/m9.figshare.16915891.
L. longipalpis PIAS, PTP61F, SOCS (or SOCS-like), STAT (or STAT-like), VIR-1 (or VIR1-like) genes were identified in this study by sequence similarity. Therefore, they were considered as putative genes. We use these acronyms only for text simplification purposes.
3.2 Silencing of PIAS in LL5 Cells and Consequent Expression of Jak-STAT-Related Genes
Our first approach was to test the activity of the Jak-STAT pathway in L. longipalpis by silencing the pathway repressor PIAS. We hypothesized that PIAS silencing would affect the gene expression of other Jak-STAT-related molecules. We chose LL5 cells, which were shown to be useful models to study L. longipalpis innate immunity (6). We transfected them with PIAS dsRNA to follow the expression of selected genes by qPCR.
We observed that PIAS silencing was significantly achieved at 24 h and 48 h post dsRNA transfection compared to the control group transfected with LacZ dsRNA (Figure 1A). The expression of the putative repressor SOCS did not alter significantly (Figure 1B), while the repressor PTP61F was significantly increased at 24 h (Figure 1C). The putative STAT transcription factor, attacin, and VIR-1 showed no significant alteration after the PIAS dsRNA transfection (Figures 1D–F).
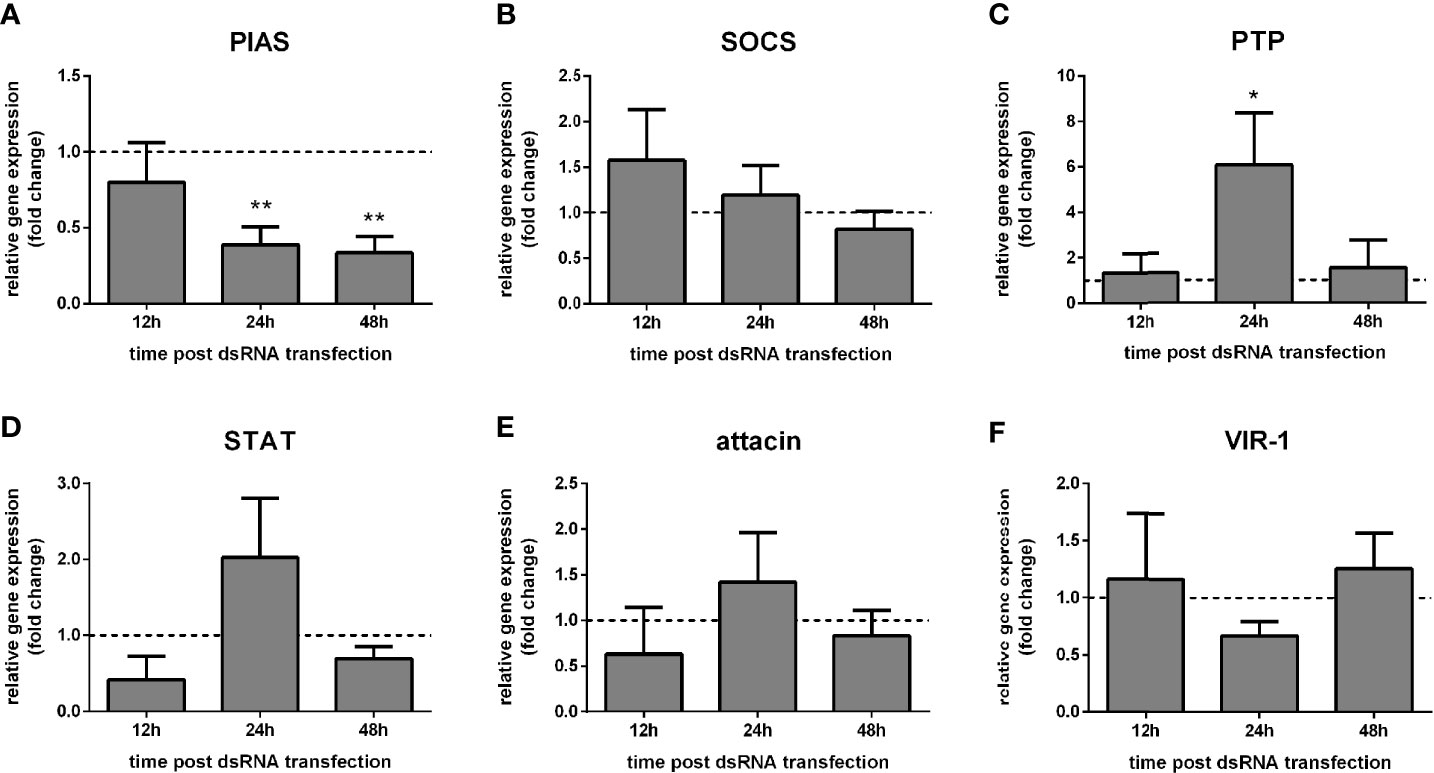
Figure 1 Gene expression of Jak-STAT regulators in LL5 cells after PIAS silencing: L. longipalpis relative gene expression was calculated compared to the endogenous reference gene RP49. (A) silencing of the PIAS gene; (B–F) expression of indicated genes after PIAS silencing. (A–F) relative expression of PIAS-dsRNA transfected LL5 cells (y-axis) was expressed as fold change compared to the control group transfected with a non-related dsRNA and collected at each corresponding time point (horizontal dotted line). Samples of experimental and control groups were collected at 12 h, 24 h, and 48 h post PIAS silencing (x-axis). Vertical bars represent the mean with standard error (SEM) of 3 biological replicates. Significant differences were calculated using two-way ANOVA (*p < 0.05; **p < 0.01).
3.3 Jak-STAT-Mediated Response to Leishmania in LL5 Cells
We also hypothesized that LL5 cells’ immune response mediated by the Jak-STAT pathway would be affected by the L. infantum challenge. Therefore, we co-cultured LL5 cells and Leishmania and assessed the expression of Jak-STAT-related genes by qPCR at subsequent times.
The putative PIAS and PTP61F expression significantly increased at 48 h and 72 h post-challenge compared to non-challenged control groups (Figures 2A, C), while SOCS expression showed a high variability (Figure 2B). The STAT-like transcription factor significantly increased at 24 h, while attacin showed no significant modulation (Figures 2D, E). Interestingly, VIR-1 was significantly reduced at 24 h, 48 h, and 72 h post-challenge (Figure 2F).
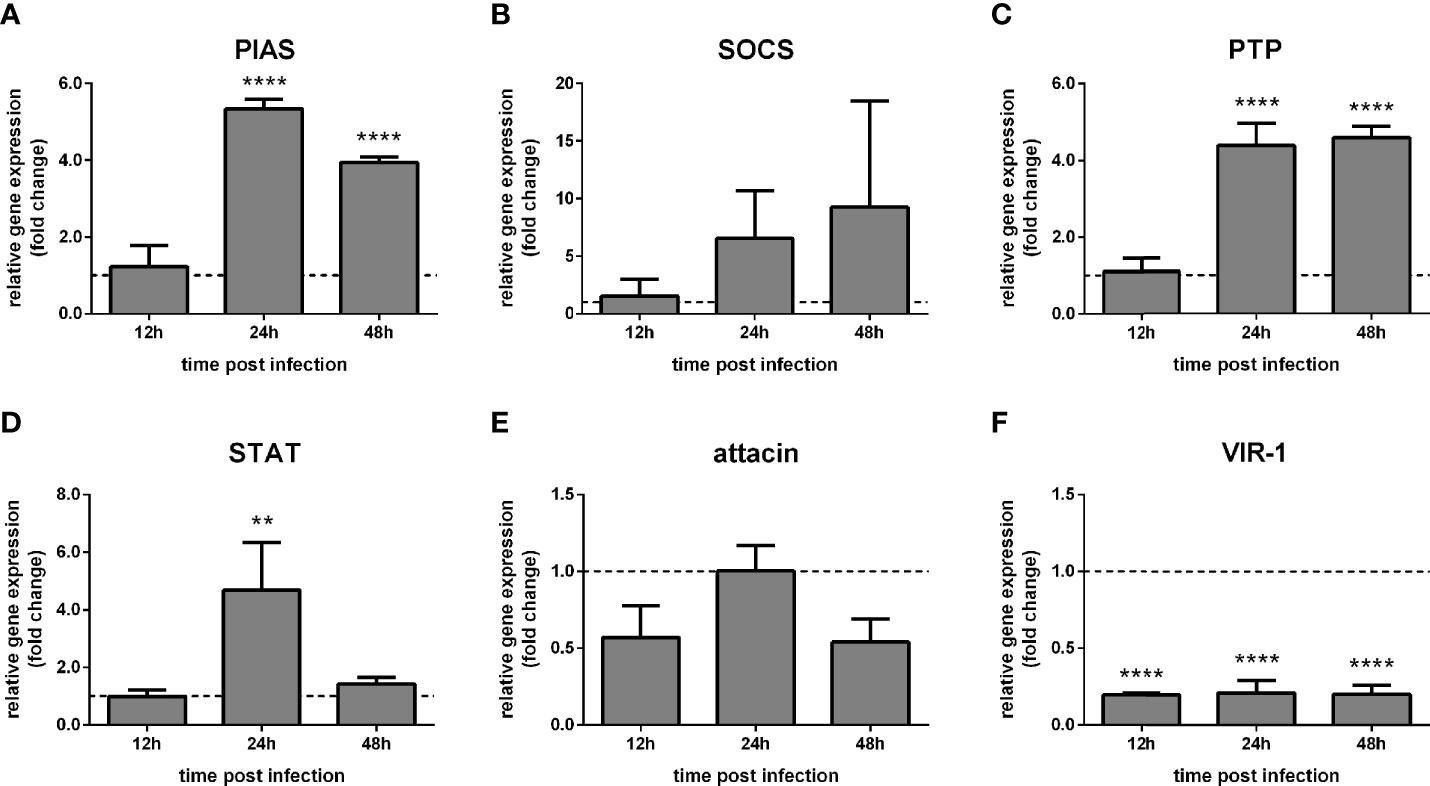
Figure 2 Gene expression of Jak-STAT-related genes in LL5 cells after Leishmania challenge: L. longipalpis relative gene expression was calculated compared to the endogenous reference gene RP49. (A–F) expression of indicated genes after Leishmania co-culture. Relative expression in Leishmania-challenged LL5 cells (y-axis) was expressed as fold change compared to the non-challenged control group collected at each corresponding time point (horizontal dotted line). Samples of experimental and control groups were collected at 24 h, 48 h, and 72 h post-Leishmania challenge (x-axis). Vertical bars represent the mean with standard error (SEM) of 3 biological replicates. Significant differences were calculated using two-way ANOVA (**p < 0.01; ****p < 0.0001).
3.4 Expression of Jak-STAT-Related Genes in L. longipalpis Females Infected With Leishmania
Our following approach investigated the Jak-STAT-related immune response in L. longipalpis adult females artificially infected with L. infantum. We hypothesized that the parasite infection would alter the pathway expression in the adult female.
The putative pathway regulators PIAS, SOCS, and PTP61F, as well as STAT, did not show significant differences in whole-body samples of sand flies infected with Leishmania compared to the blood-fed control group (Figures 3A–D). Attacin expression was reduced at 72 h (Figure 3E), while VIR-1 and iNOS have not altered post Leishmania infection (Figures 3F, G). We detected the bacteria load by the 16S ribosomal RNA gene expression, showing no significant differences between infected and non-infected groups (Figure 3H). In addition, we assessed the parasite load through the Leishmania actin gene expression compared to a control sample collected at 24 h post-infection. The parasite load was increased 48 h post-infection (Figure 3I).
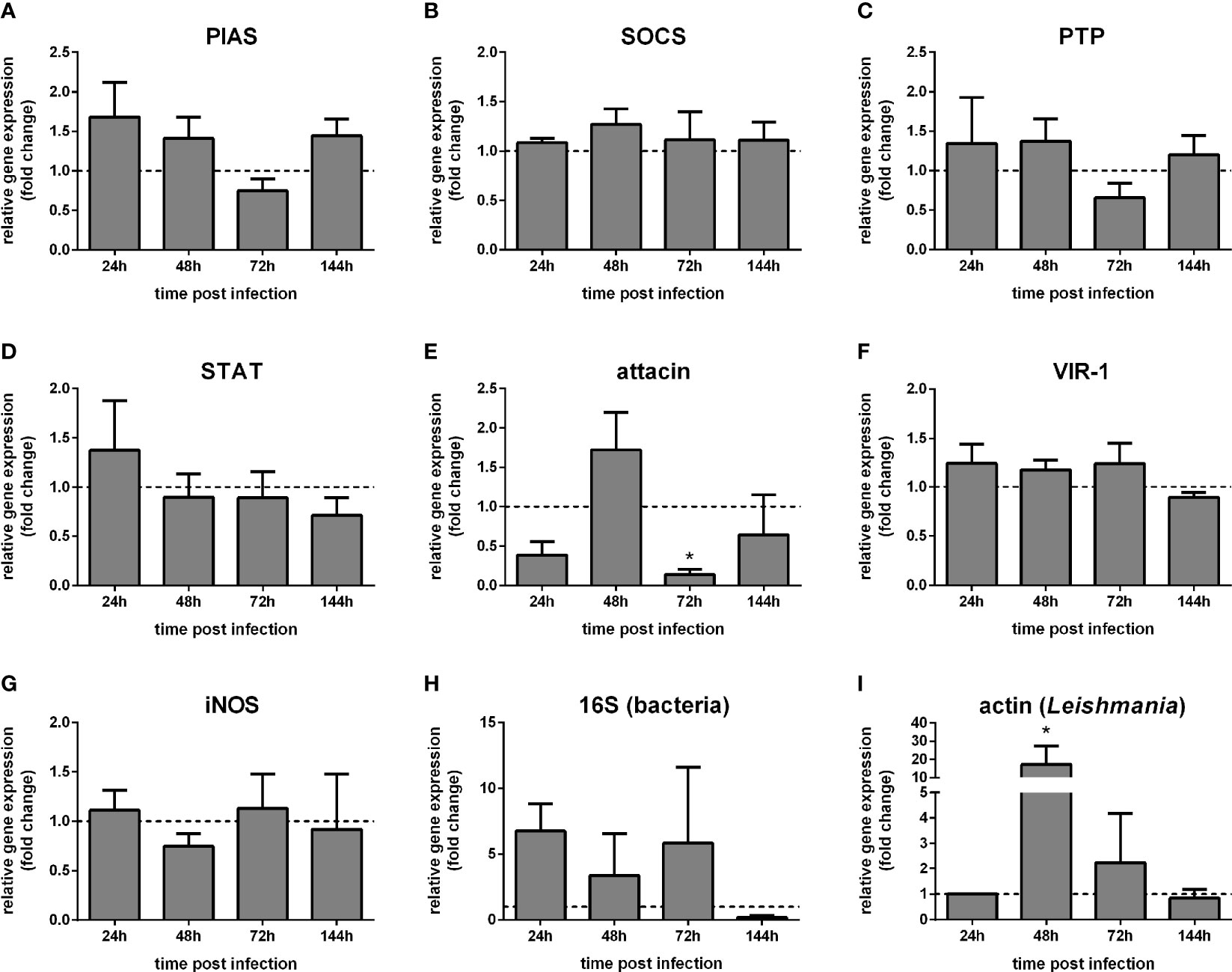
Figure 3 Gene expression of Jak-STAT-related genes in L. longipalpis infected with Leishmania: L. longipalpis relative gene expression was calculated compared to the endogenous reference gene RP49. (A–G) expression of indicated genes after Leishmania infection; quantification of bacteria (H) and Leishmania (I). Relative gene expression in Leishmania-infected females (y-axis) was expressed as fold change compared to the non-infected female control group collected at each corresponding time point (horizontal dotted line) (A–H). Leishmania actin expression was expressed as fold change compared to a control sample collected at 24 h post-infection (I). Samples of experimental and control groups were collected in pools of 10 whole body sand flies at 24 h, 48 h, 72 h, and 144 h post-infection (x-axis). Vertical bars represent the mean with standard error (SEM) of 3 biological replicates. Significant differences were calculated using two-way ANOVA (*p < 0.05).
It is well known that the gut microbial community plays a considerable role in balancing immune responses (reviewed in 46). In our study model, both experimental and control groups were infected with L. infantum. We chose to deplete the commensal bacteria in the experimental group by feeding with a mixture of antibiotics, while the control group was Leishmania-infected but not treated with antibiotics. We tested whether the suppression of gut bacteria would interfere with the expression of Jak-STAT-related molecules in infected sand flies.
The expression of pathway regulators PIAS and STAT-like were not significantly altered in whole sand flies after bacteria depletion (Supplementary Figures 8A, B). Attacin expression was also not changed significantly but was highly variable at 144 h post-infection (Supplementary Figure 8C). In addition, VIR-1 was also not significantly changed by the antibiotic treatment (Supplementary Figure 8D).
3.5 Expression of Jak-STAT-Related Genes in STAT-Silenced L. longipalpis Females Fed on Sucrose
To further explore the role of the Jak-STAT pathway, we hypothesized that the suppression of STAT-like transcription factor by RNAi-mediated gene silencing would reduce the gene expression of effector molecules associated with the pathway.
STAT expression was reduced in females at 24 h post STAT dsRNA injection compared to the control group injected with LacZ dsRNA (Figure 4A). Upon STAT silencing, attacin expression was unaltered at 24 h and increased significantly at 48 h (Figure 4B). VIR-1 expression showed a reduction at 24 h and 72 h and increased at 48 h (Figure 4C). iNOS expression was significantly reduced at 24 h and 48 h (Figure 4D), while DUOX was increased at 24 h (Figure 4E). Bacteria detection by 16S rRNA was not significantly altered (Figure 4F).
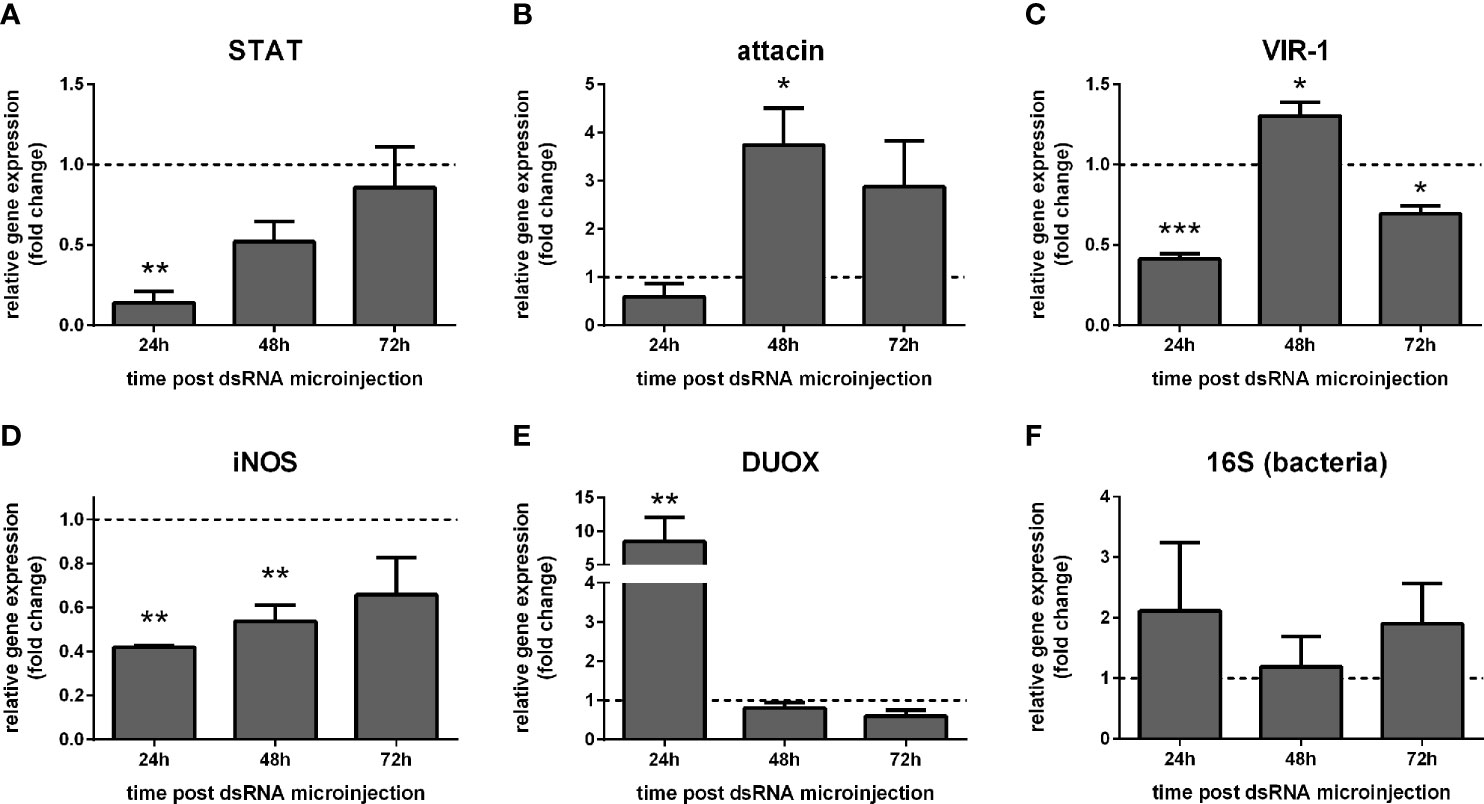
Figure 4 Gene expression of Jak-STAT-related genes in STAT-silenced sucrose fed L. longipalpis: L. longipalpis relative gene expression was calculated compared to the endogenous reference gene RP49. (A): silencing of the STAT-like gene; (B–E): expression of indicated genes after STAT-like gene silencing. (F) quantification of bacteria. (A–F) relative expression of STAT-like dsRNA injected females (y-axis) was expressed as fold change compared to the control group of LacZ dsRNA injected females. Both group samples were collected at each corresponding time point (horizontal dotted line). Samples of experimental and control groups were collected in pools of 10 whole body sand flies at 24 h, 48 h, and 72 h post-STAT silencing (x-axis). Vertical bars represent the mean with standard error (SEM) of 3 biological replicates. Significant differences were calculated using two-way ANOVA (*p < 0.05; **p< 0.01; ***p < 0.0001).
3.6 Expression of Jak-STAT-Related Genes in STAT-Silenced L. longipalpis Females Infected With Leishmania
We also investigated the effect of STAT-like silencing in the gene expression of STAT-related downstream molecules in dissected gut and carcasses of Leishmania-infected sand flies. In carcasses, STAT expression was significantly reduced at 24 h and 48 h (Figure 5A), while attacin was increased at 48 h post-infection (Figure 5B). VIR-1 was not significantly altered (Figure 5C), while iNOS was reduced at 24 h and 48 h, and DUOX at 24 h (Figures 5D, E).
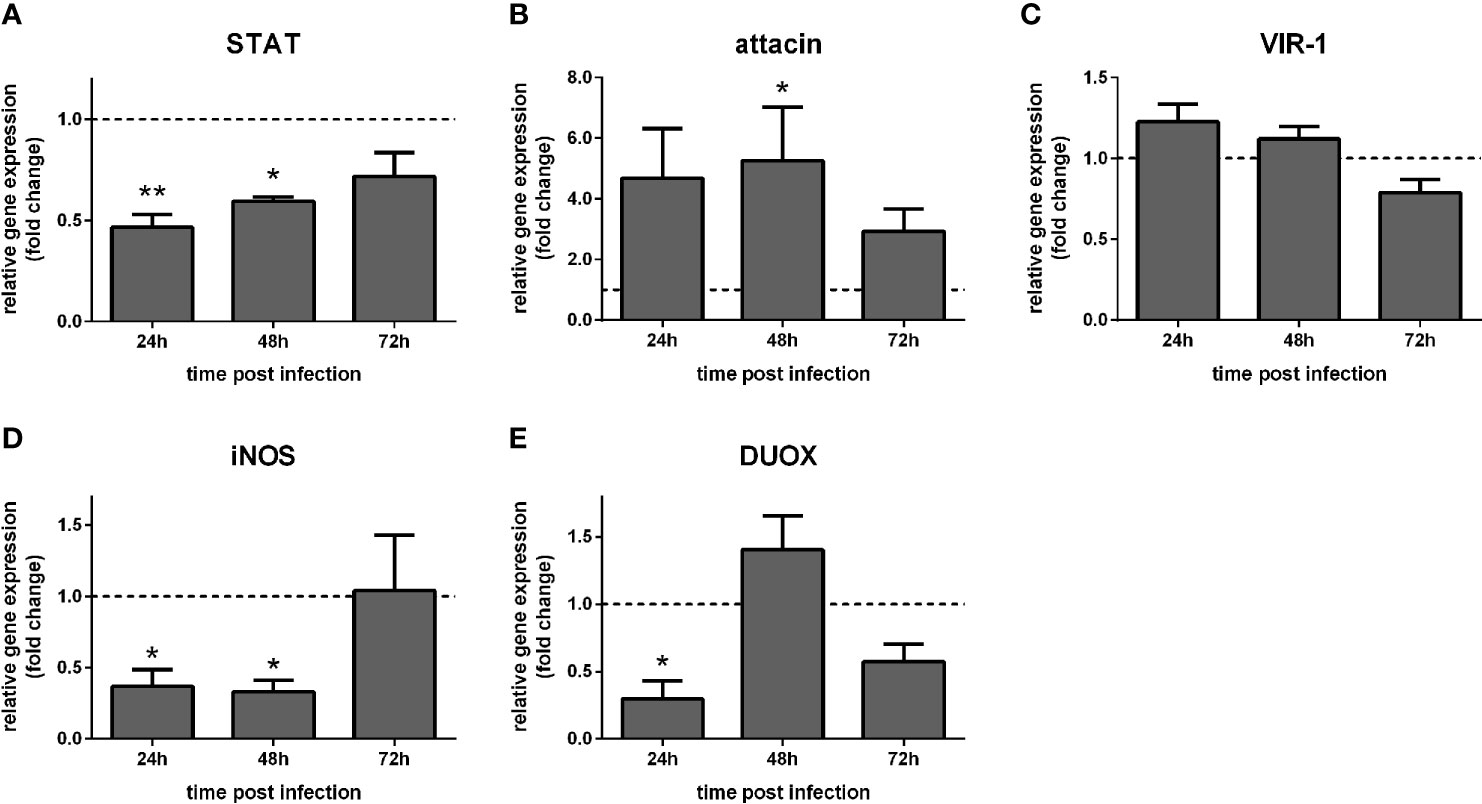
Figure 5 Gene expression of Jak-STAT-related genes in carcasses of STAT-silenced L. longipalpis and infected with L. infantum: L. longipalpis relative gene expression was calculated compared to the endogenous reference gene RP49. (A) silencing of the STAT-like gene; (B–E): expression of indicated genes after STAT-like gene silencing. (A–E) relative gene expression in STAT-like dsRNA injected females (y-axis) was expressed as fold change compared to the control group of LacZ dsRNA injected females. Samples were collected at 24 h, 48 h, and 72 h post-infection (x-axis). Vertical bars represent the mean with standard error (SEM) of 3 biological replicates. Significant differences were calculated using two-way ANOVA (*p < 0.05; **p< 0.01).
In dissected guts, STAT-like expression was reduced at 24 h and 48 h (Figure 6A). Attacin was increased at 48 h post-infection (Figure 6B). VIR-1, iNOS, and DUOX were not significantly altered (Figures 6C–E). Also, bacteria detection through 16S ribosomal RNA expression was not significantly altered (Figure 6F), while Leishmania detection through actin expression was significantly increased at 24 h (Figure 6G).
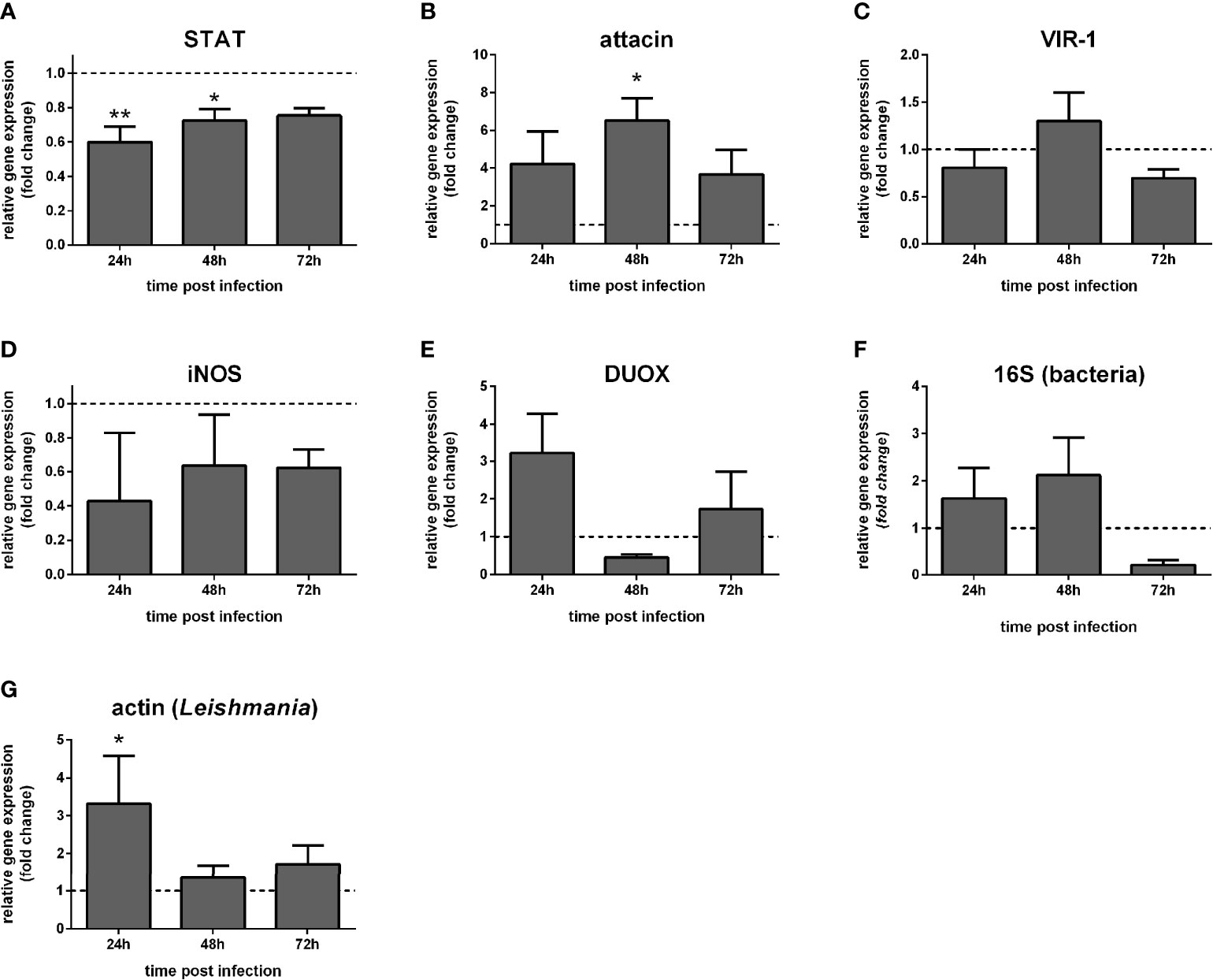
Figure 6 Gene expression of Jak-STAT-related genes in dissected guts of STAT-silenced L. longipalpis and infected with L. infantum: L. longipalpis relative gene expression was calculated compared to the endogenous reference gene RP49. (A) silencing of the STAT-like gene; (B–E) expression of indicated genes after the STAT-like gene silencing; quantification of bacteria (F) and Leishmania (G). (A–F) relative expression in STAT-like dsRNA injected females (y-axis) was expressed as fold change compared to the control group of LacZ dsRNA injected females. Samples were collected at 24 h, 48 h, and 72 h post-infection (x-axis). Vertical bars represent the mean with standard error (SEM) of 3 biological replicates. Significant differences were calculated using two-way ANOVA (*p < 0.05; **p < 0.01).
3.7 Leishmania Infection Development in STAT-Silenced L. longipalpis Females
In addition, we hypothesized that the downstream effects of STAT-like silencing could cause an alteration on L. infantum colonization in the sand fly gut since we observed an increase in Leishmania detection after STAT silencing. Therefore, we investigated the intensity and localization of parasites on six days post-infection in sand fly dissected guts. Leishmania infection estimation showed that proportions of STAT-silenced sand flies carrying heavy, moderate, or light infections, as well as non-infected, were not significantly different from the control group injected with LacZ dsRNA (Figure 7A). The parasite localization in the stomodeal valve, thoracic gut, and abdominal gut did not differ significantly between STAT-silenced and control groups (Figure 7B).
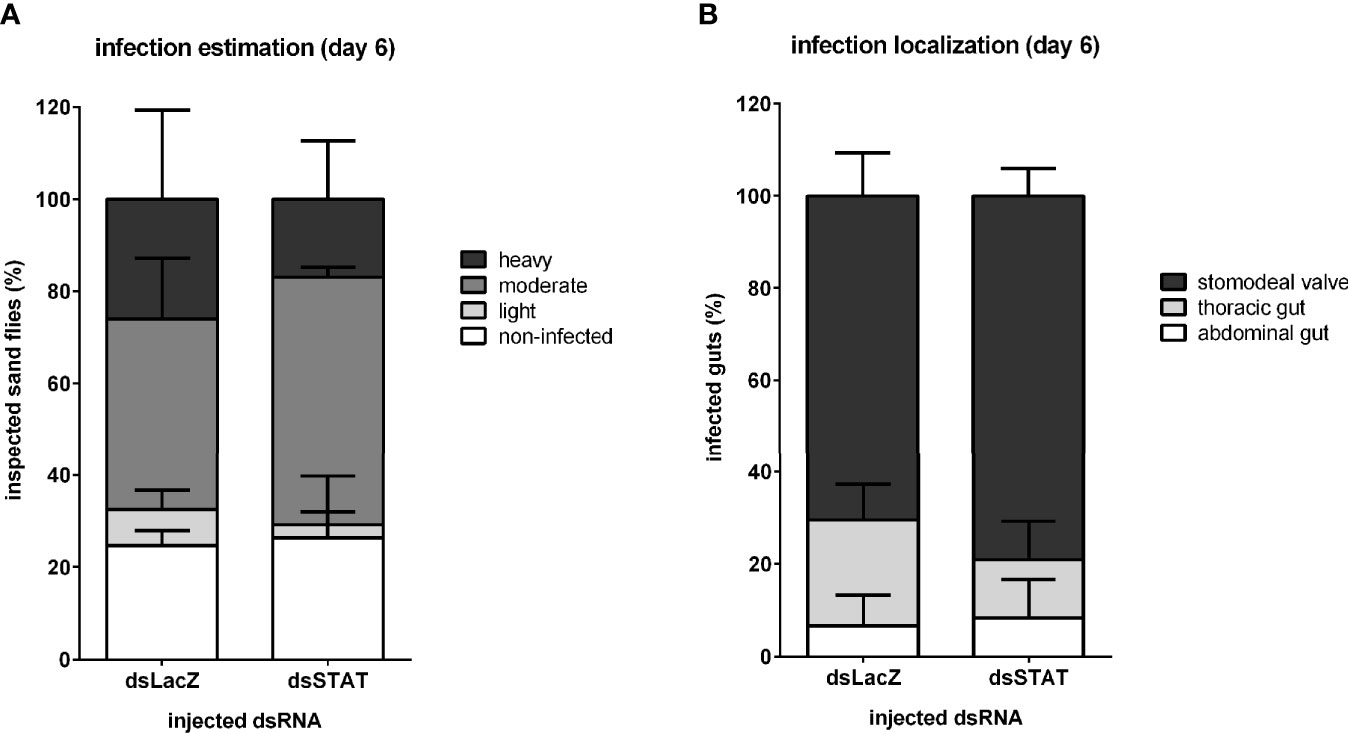
Figure 7 Leishmania infection development in STAT-like-silenced L. longipalpis: Infection intensity and development at late infection in dsRNA-injected sand flies. (A) The y-axis represents the percentage of all individually inspected insects (minimum of 20 sand flies in each dsRNA injected group). Bar colors indicate infection intensity: non-infected (white), with light (light grey), moderate (mid grey), and heavy (dark grey) infections. (B) The y-axis represents the percentage of infected insects used in infection progress evaluation in the gut. Bar colors indicate sand fly gut localization: parasites reached the stomodeal valve (dark grey), thoracic gut (light grey), or stayed in the abdominal gut (white). The x-axis represents dsRNA injected groups. No significant differences were found between experimental and control groups (two-way ANOVA).
A summarized workflow of the experimental approach and outcomes of the manuscript is shown in Supplementary Figure 9.
4 Discussion
Sand flies trigger a repertoire of molecular mechanisms to fight potential microbial harm (reviewed in 47). In L. longipalpis, these mechanisms include AMPs production regulated by the Toll and IMD pathways (6, 8, 41, 48). Nevertheless, the complexity of the immune response in this insect is not fully understood. We were interested in investigating the role of the Jak-STAT pathway in the context of L. longipalpis interaction with L. infantum.
4.1 Jak-STAT Pathway
We identified L. longipalpis transcripts involved in the Jak-STAT pathway based on their moderate similarity with genes identified in Drosophila, Aedes, and Anopheles, which are well-characterized models. These L. longipalpis sequences indicate that the pathways genes are transcribed and have moderate similarity with other dipterans, including vectors of zoonotic diseases. Such similarities are shared across invertebrates and vertebrate species (reviewed in 49).
In insects, this pathway is activated upon microbial infections. In Drosophila, it is triggered upon viral infections by DCV, Drosophila X virus (DXV), invertebrate iridescent virus 6 (IIV6) (50). In mosquitoes, it is triggered by the West Nile virus (WNV) in C. quinquefasciatus (51), dengue virus (DENV) (52, 53), and Zika virus (54) infection in Aedes aegypti, to cite a few. However, only a reduced number of studies showed the role of this pathway against parasitic infection. In Anopheles aquasalis and A. gambiae, Jak-STAT was activated toward P. vivax and P. berghei, respectively (23, 55), indicating the pathway role in the insect response against the parasitic infection.
4.2 PIAS Knockdown in LL5 Cells and effect on Jak-STAT-Related Genes
To test this pathway activity in L. longipalpis LL5 cells, we knocked down PIAS, a major pathway repressor, and followed the consequent effect on other Jak-STAT related genes. The LL5 is a suitable model for studying immune pathway modulation since experimental variables are easier to control in these cells than in insects. These cells were used to investigate arboviral infections (56, 57) and have an antiviral response (7, 57, 58). Moreover, they were used in studies investigating the interaction with Leishmania (59) and later shown to have Toll and IMD mediated immune response against bacteria, yeast, and Leishmania (6, 9). Previously, these cells were shown to respond efficiently to dsRNA transfection (6), and PIAS was efficiently silenced in the current experimental setting. Although the inhibitory action of PIAS occurs at the protein level, the suppression of PIAS could result in a consequent effect on the transcription of other pathway molecules, possibly by a feedback loop.
The Jak-STAT pathway has many key regulatory molecules, including repressors such as SOCS and PTP61F, that could be expressed to counterbalance PIAS silencing. While the putative SOCS showed no significant changes, PTP61F expression was increased after PIAS was silenced. PIAS downmodulation suggests that the pathway was activated, and PTP61F may be expressed to compensate for PIAS reduction. PTPs can dephosphorylate STAT tyrosine residues, therefore preventing this transcription factor translocation to the nucleus and consequently negatively regulating the pathway-dependent genes (reviewed in 60). STAT-like expression was not correlated with PIAS suppression or the increase of PTP61F. This finding shows that STAT expression was not directly related to PIAS silencing. Thus, the possibility of existing a feedback loop between PIAS and STAT is scarce. Attacin and VIR-1 showed no direct correlation with the repressors. Together these results suggest that the Jak-STAT pathway in LL5 cells was balanced between PIAS and PTP61F repressors.
4.3 Effect of Leishmania Challenge on Jak-STAT-Related Genes in LL5 Cells
It was previously shown that the interaction with Leishmania parasites caused dramatic changes to L. longipalpis embryonic Lulo cells (61, 62). In LL5 cells, we previously observed that the interaction with Leishmania triggered the expression of two transcription factors, dorsal and relish, involved in the Toll and IMD pathways, respectively, with a subsequent moderate effect on AMPs expression (6). We used the LL5 co-cultured with Leishmania to investigate whether the parasite interaction would trigger the Jak-STAT pathway. We observed that the expression of PIAS and PTP61F was increased after the second day, showing that the co-culture setting repressed the pathway. The possibility of a feedback loop influencing the STAT-like transcription was also considered in this experimental setting. We observed that STAT expression was not reduced, as the repressor was upregulated, but its expression was increased at 24 h post Leishmania challenge. These results are not sufficient to assess a direct correlation between the expression of PIAS or PTP61F with STAT-like transcription. Still, the STAT increase may be a result of a reduced expression of downstream effector molecules. A simultaneous increase between repressor and transcription factor also occurred in L. longipalpis Toll and IMD pathways (6).
Attacin expression was unaltered under co-culture with Leishmania, but VIR-1 was downregulated during the three time points investigated. It is in agreement with the upregulation of PIAS and PTP61F. VIR-1 is actively expressed in Drosophila S2 cells (19), and A. aegypti Aag2 cells (63) in response to DCV and Wolbachia infections, respectively, and might be under the effect of other signaling pathways. The downregulation of VIR-1 after a parasitic challenge was not previously shown in insect cells. However, it may result from the shedding of parasite exosomes containing virulence factors (reviewed in 64), as seen in L. infantum in culture (65). For instance, Leishmania secretes virulence factors such as elongation factor 1 alpha (EF1-alpha) (66) and the widely studied metalloprotease GP63 to activate the macrophage tyrosine phosphatases, which in turn represses the Toll and Jak-STAT pathways in the host cell (67, 68). Therefore, it is reasonable to consider that the parasite may cause suppression in the insect cell immune response. These results suggest that the Jak-STAT pathway in LL5 cells was repressed by L. infantum with a downstream suppression of VIR-1.
4.4 Effect of Leishmania Infection on Jak-STAT-Related Genes in L. longipalpis Females
We also tested the Jak-STAT pathway activation upon parasite infection using adult L. longipalpis as a model. Our results showed that the Leishmania infection in the sand fly caused no significant changes in the expression of the putative pathway repressors PIAS, SOCS, PTP61F, and STAT-like. Such nondramatic changes were also reported in the latest transcriptome study of L. longipalpis infected with Leishmania, where PIAS expression had no statistically significant modulation (45). Interestingly, in a recent investigation on P. papatasi infected with different trypanosomatid species (Leishmania major, Leishmania donovani, and Herpetomonas muscarum), a STAT transcript was reduced as blood digestion occurred. Still, its expression pattern did not show significant differences when compared to non-infected sand flies (69).
In our present study, attacin expression was reduced at the end of the blood digestion process, when the peritrophic matrix is degraded, and parasites get in close contact with the sand fly gut epithelium (70, 71). Therefore it is possible that attacin reduction was induced by the parasite, not through interfering with the Jak-STAT pathway, but possibly by Toll or IMD pathways as previously shown in Drosophila (72, 73). Our analysis of bacteria during the infection indicated no dramatic changes when compared to the non-fed females. The highest Leishmania detection occurred on the second day post-infection when multiplicative forms of the parasite are abundant (74). These results suggest that Leishmania infection did not significantly alter Jak-STAT-related gene expression in L. longipalpis.
4.5 Depletion of Commensal Gut Bacteria in Leishmania-Infected Sand Flies and Its Effect on Jak-STAT-Related Genes
Commensal microbiota harbored in the insect gut may add another level of complexity inherent to the L. longipalpis study model. We tested if the gut bacterial community created additive stimuli to the Jak-STAT mediated response during the parasite infection. We depleted bacteria by adding a combination of antibiotics to the sand fly sucrose- and blood-meals. Several antibiotic combinations were used in sand flies to deplete gut bacteria (8, 75–79). We chose a combination of penicillin, streptomycin, and gentamicin, and we tested its efficiency on sucrose-fed females in a pilot experiment (80). The antibiotic treatment effectively depleted gut bacteria up to the fourth day of treatment compared to a non-treated group, detected on LB-agar plates seeded with sand fly gut homogenates. Therefore, we used this same antibiotic treatment in our infection experiments.
None of the four genes investigated in the antibiotic-treated group showed significant differential expression compared to the non-treated control group. Nevertheless, there was a slight increase in the expression of the repressor PIAS and the STAT-like transcription factor at 24 h post-infection. This effect can be credited to the parasite. As the infection progressed, attacin showed a quite variable expression toward 144 h, and VIR-1 was highly variable in all time points investigated. This variability suggests that differences in the progress of Leishmania infection may be the cause. In addition, these changes may be associated with changes in parasite development caused by bacteria depletion. Kelly et al. (75) showed that depletion of bacteria caused by antibiotics impaired the development of L. infantum metacyclic forms on late-phase infection. Therefore, our results indicate that the Jak-STAT mediated response may be slightly changed by the reduction of gut bacteria but not playing the primary role.
4.6 Effect of STAT-Like Knockdown on Downstream Effector Genes in Non-Infected Sand Flies
One alternative to investigating the role of the Jak-STAT pathway in L. longipalpis response upon infection is to interfere with downstream transcription of effector molecules. For that, we silenced the STAT-like transcription factor using dsRNA, which also reduced the gene expression of VIR-1 and iNOS in non-infected insects on the first day post-injection. The iNOS reduction was maintained on the second day, indicating a direct correlation with STAT silencing. On the other hand, VIR-1 expression increased on the second day and reduced on the third day suggesting that other transcription factor may be involved in its regulation. Interestingly, while iNOS expression was downregulated, DUOX was upregulated on the first day. DUOX is responsible for the production of superoxide (H2O2) and is modulated by the Hedgehog (Hh) signaling pathway (81) which is vital for controlling gut infections in Drosophila (82). This finding reflects a possible balance between these two genes that are oxidative stress-inducing molecules.
The overall detection of bacteria in STAT silenced sand flies during the three days investigated did not significantly change compared to the control group and reflects the maintained balance between iNOS and DUOX expression. However, the attacin expression upregulation may be caused by another pathway such as Toll or IMD as indicated in our previous studies (6, 41) thus posing an additional microbial control. These results indicate that the L. longipalpis putative STAT is associated with the iNOS expression, while attacin and VIR-1 had no direct correlation with STAT silencing in sucrose-fed females. Since the Jak-STAT pathway could be differently activated in the fat body (83) and in gut cells (84), as is the case in Drosophila, we decided to investigate the pathway-related gene expression in L. longipalpis separated carcasses and dissected guts.
4.7 Effect of STAT-Like Knockdown in Leishmania-Infected Sand Flies
We also hypothesized that the suppression of the pathway would facilitate the development of the parasite in its vector. We observed that STAT silencing was achieved in sand fly carcasses on the first two days post-infection. We also observed the increase of attacin expression similar to what was observed in our results with non-infected STAT-silenced, possibly being regulated by the Toll and IMD pathways as mentioned above. This silencing also caused a reduction in iNOS. The consistent decrease in iNOS expression in STAT-silenced insects supports the idea that iNOS is under Jak-STAT regulation, as seen in A. gambiae (55). Nevertheless, attacin and VIR-1 expressions were not likely to be under the regulation of the STAT-like transcription factor.
The reduction in the putative STAT expression was achieved in dissected guts, but silencing levels were not as intense as in carcasses. Attacin expression was increased at 48 h post-infection, similar to carcasses, indicating the upregulation of this AMP occurred systemically. Although attacin expression was raised, it did not cause an effect against Leishmania. VIR-1 expression did not alter after STAT silencing in guts similar to what was seen in carcasses, thus adding more evidence that it is not regulated through this transcription factor in L. longipalpis. iNOS expression was shown to be quite variable in the first two days, and DUOX modulation also followed a variable pattern, possibly associated to iNOS modulation. The bacterial detection was not significantly altered, similar to what was seen in the non-infected and silenced sand flies, indicating that molecules associated to the putative STAT had a limited effect on the bacteria balance, and vice versa.
Most interestingly, on the first day of STAT silencing, there was an increase of Leishmania detection within the silenced group, indicating that an effector molecule regulated by this transcription factor was consequently suppressed and favored Leishmania survival in this early time. Although iNOS expression was quite variable in guts, it is possible that the nitric oxide production was altered and resulted in a less harmful environment to Leishmania. An analogous effect of increased nitric oxide in L. longipalpis caused a reduction of parasite detection in TGF-beta gene silenced sand flies (35). These results indicate that the STAT-like transcription factor did not regulate attacin and VIR-1 in the gut of infected females and suggest that the variation in iNOS expression may have favored the parasite on the first day after STAT suppression.
4.8 Effect of STAT-Like Knockdown on the Leishmania Infection Development in Sand Flies
We also hypothesized that the silencing effect on the early time of infection could further influence the progress of L. infantum infection in the sand fly. We assessed infection intensity on a later phase of infection (6 days post-infection) by light microscopy. The infection estimation in individual sand flies showed that parasitic loads vary, and both silenced and control groups shared approximately the same loads of parasites. In addition, the infection localization showed that both insect groups had similar percentages of insects harboring parasites in the stomodeal valve, thus presenting similar conditions to complete the parasite cycle in the vector. Our results indicate that the favored parasite growth in the early phase of infection had no significant effect on the late infection stage.
4.9 Concluding Remarks
In conclusion, we aimed to understand the role of the Jak-STAT pathway in L. longipalpis immune response, more specifically toward L. infantum parasites. We identified putative sand fly genes belonging to the main pathway regulators and possible downstream related effector molecules. The SOCS-like may not be involved in regulating the sand fly Jak-STAT pathway. PIAS and PTP61F repressors balanced the pathway in LL5 cells, and the Leishmania challenge upregulated the pathway repressors and reduced VIR-1 expression. The parasite infection and the bacteria suppression during the parasite infection in females did not cause significant changes in the Jak-STAT pathway. The putative STAT silencing caused a reduction in iNOS in whole bodies and carcasses, while it had no direct effect on attacin and VIR-1. In addition, parasite detection was increased on the first day post STAT silencing, although it was insufficient to yield a higher-rate infection on late-phase infection.
The present work is the first report on putative sand fly genes involved in the Jak-STAT pathway. It is possible that other STAT proteins not covered in this study may be expressed and could compensate for the knockdown of this STAT-like gene. Similarly, other attacin genes may be expressed under the control of other pathways such as Toll or IMD, but this possibility needs further investigation.
The sand fly immune response is constantly regulated to balance and respond to different microbial challenges [reviewed in (47)]. Simultaneously, Leishmania parasites can adapt to these changes expressing a plethora of genes during its cycle (85). We focused on a pathway not explored in sand flies and brought additional information on the complex interaction between the L. longipalpis immune response to L. infantum.
Data Availability Statement
The datasets presented in this study can be found in online repositories. The names of the repository/repositories and accession number(s) can be found in the article/Supplementary Material.
Ethics Statement
The animal study was reviewed and approved by “Committee on the Ethics of Laboratory Experiments of the Charles University” under permission No. MSMT-8604/2019-6 and “Committee on the Ethics in the use of Animals of the Institute Oswaldo Cruz (CEUA-IOC)” under permission No.CEUA/IOC-005/2019.
Author Contributions
Conceptualization, ET, AP, and YT-C.Methodology, ET and AP. Validation, ET. Formal analysis and investigation, ET, DA-B, BK, and BT-N. Resources, YT-C and PV. Data curation, ET and BT-N. Writing-original draft preparation, ET. Writing-review and editing, ET and YT-C. visualization, ET. Supervision and project administration, ET, AP, PV, and YT-C. Funding acquisition, YT-C, ET, and PV. All authors contributed to the article and approved the submitted version.
Funding
This research was funded by IOC-Fiocruz (PAEF Program), INCT-EM (Institutos Nacionais em Ciencia e Tecnologia - Entomologia Molecular), research grant number CNPq/Proc. 465678/2014-9 and Projeto Inova-Fiocruz. We are grateful for support from the Coordination for the Improvement of Higher Education Personnel (CAPES) - Brazil. DA-B and BT-N received a fellowship from CAPES. ET, BK, and PV were supported by the International Mobility of Researchers at Charles University (CZ.02.2.69/0.0/0.0/16_027/0008495), Czech Science Foundation (GACR 21-15700S), and by ERD Funds, project CePaViP (16_019/0000759).
Conflict of Interest
The authors declare that the research was conducted in the absence of any commercial or financial relationships that could be construed as a potential conflict of interest.
Publisher’s Note
All claims expressed in this article are solely those of the authors and do not necessarily represent those of their affiliated organizations, or those of the publisher, the editors and the reviewers. Any product that may be evaluated in this article, or claim that may be made by its manufacturer, is not guaranteed or endorsed by the publisher.
Acknowledgments
We thank Ms. Thais Lemos-Silva for the pilot experiment testing antibiotic treatment efficiency in sucrose-fed L. longipalpis. We thank Dr. Peter W. Mason, University of Texas Medical Branch, for critical reading of the manuscript.
Supplementary Material
The Supplementary Material for this article can be found online at: https://www.frontiersin.org/articles/10.3389/fitd.2021.747820/full#supplementary-material
Supplementary Figure 9 | Summarized workflow of experimental approach and outcomes
References
1. WHO. The Global Health Observatory - Leishmaniasis [Internet] (2019). Available at: https://www.who.int/data/gho/data/themes/topics/gho-ntd-leishmaniasis (Accessed 2021 Feb 14).
2. Akhoundi M, Kuhls K, Cannet A, Votýpka J, Marty P, Delaunay P, et al. A Historical Overview of the Classification, Evolution, and Dispersion of Leishmania Parasites and Sandflies. PloS Negl Trop Dis (2016) 10(3):e0004349. doi: 10.1371/journal.pntd.0004349
3. Lainson R, Rangel EF. Lutzomyia Longipalpis and the Eco-Epidemiology of American Visceral Leishmaniasis, With Particular Reference to Brazil: A Review. Mem Inst Oswaldo Cruz (2005) 100(8):811–27. doi: 10.1590/S0074-02762005000800001
4. Wilson AL, Courtenay O, Kelly-Hope LA, Scott TW, Takken W, Torr SJ, et al. The Importance of Vector Control for the Control and Elimination of Vector-Borne Diseases. PloS Negl Trop Dis (2020) 14(1):e0007831. doi: 10.1371/journal.pntd.0007831
5. Jones RT, Ant TH, Cameron MM, Logan JG. Novel Control Strategies for Mosquito-Borne Diseases. Philos Trans R Soc B Biol Sci (2021) 376:20190802. doi: 10.1098/rstb.2019.0802
6. Tinoco-Nunes B, Telleria EL, Da Silva-Neves M, Marques C, Azevedo-Brito DA, Pitaluga AN, et al. The Sandfly Lutzomyia Longipalpis LL5 Embryonic Cell Line has Active Toll and Imd Pathways and Shows Immune Responses to Bacteria, Yeast and Leishmania. Parasites Vectors [Internet] (2016) 9:222. doi: 10.1186/s13071-016-1507-4
7. Pitaluga AN, Mason PW, Traub-Cseko YM. Non-Specific Antiviral Response Detected in RNA-Treated Cultured Cells of the Sandfly, Lutzomyia Longipalpis. Dev Comp Immunol (2008) 32(3):191–7. doi: 10.1016/j.dci.2007.06.008
8. Telleria EL, Sant’Anna MRV, Ortigão-Farias JR, Pitaluga AN, Dillon VM, Bates PA, et al. Caspar-Like Gene Depletion Reduces Leishmania Infection in Sand Fly Host Lutzomyia Longipalpis. J Biol Chem [Internet] (2012) 287(16):12985–93. doi: 10.1074/jbc.M111.331561
9. da Silva Goncalves D, Iturbe-Ormaetxe I, Martins-da-Silva A, Telleria EL, Rocha MN, Traub-Cseko YM, et al. Wolbachia Introduction Into Lutzomyia Longipalpis (Diptera: Psychodidae) Cell Lines and Its Effects on Immune-Related Gene Expression and Interaction With Leishmania Infantum. Parasit Vectors (2019) 12(1):33. doi: 10.1186/s13071-018-3227-4
10. Bang IS. JAK/STAT Signaling in Insect Innate Immunity. Entomol Res (2019) 49:339–53. doi: 10.1111/1748-5967.12384
11. Lemaitre B, Hoffmann J. The Host Defense of Drosophila Melanogaster. Annu Rev Immunol (2007) 25:697–743. doi: 10.1146/annurev.immunol.25.022106.141615
12. Kingsolver MB, Hardy RW. Making Connections in Insect Innate Immunity. Proc Natl Acad Sci U.S.A. [Internet] (2012) 109(46):18639–40. doi: 10.1073/pnas.1216736109
13. Myllymaki H, Ramet M. JAK/STAT Pathway in Drosophila Immunity. Scand J Immunol [Internet] (2014) 79(6):377–85. doi: 10.1111/sji.12170
14. Morin-Poulard I, Vincent A, Crozatier M. The Drosophila JAK-STAT Pathway in Blood Cell Formation and Immunity. JAKSTAT [Internet] (2013) 2(3):e25700. doi: 10.4161/jkst.25700
15. Huang Z, Kingsolver MB, Avadhanula V, Hardy RW. An Antiviral Role for Antimicrobial Peptides During the Arthropod Response to Alphavirus Replication. J Virol [Internet] (2013) 87(8):4272–80. doi: 10.1128/JVI.03360-12
16. Hanson MA, Dostálová A, Ceroni C, Poidevin M, Kondo S, Lemaitre B. Synergy and Remarkable Specificity of Antimicrobial Peptides In Vivo Using a Systematic Knockout Approach. Elife (2019) 8:e44341. doi: 10.7554/eLife.44341
17. Hultmark D, Engstrom A, Andersson K, Steiner H, Bennich H, Boman HG. Insect Immunity. Attacins, a Family of Antibacterial Proteins From Hyalophora Cecropia. EMBO J (1983) 2(4):571–6. doi: 10.1002/j.1460-2075.1983.tb01465.x
18. Hu Y, Aksoy S. An Antimicrobial Peptide With Trypanocidal Activity Characterized From Glossina Morsitans Morsitans. Insect Biochem Mol Biol (2005) 35(2):105–15. doi: 10.1016/j.ibmb.2004.10.007
19. Dostert C, Jouanguy E, Irving P, Troxler L, Galiana-Arnoux D, Hetru C, et al. The Jak-STAT Signaling Pathway is Required But Not Sufficient for the Antiviral Response of Drosophila. Nat Immunol (2005) 6(9):946–53. doi: 10.1038/ni1237
20. Pautz A, Art J, Hahn S, Nowag S, Voss C, Kleinert H. Regulation of the Expression of Inducible Nitric Oxide Synthase. Nitric Oxide - Biol Chem (2010) 23(2):75–93. doi: 10.1016/j.niox.2010.04.007
21. Murali Krishna Rao K. Molecular Mechanisms Regulating Inos Expression in Various Cell Types. J Toxicol Environ Heal - Part B Crit Rev (2000) 3(1):27–58. doi: 10.1080/109374000281131
22. Rivero A. Nitric Oxide: An Antiparasitic Molecule of Invertebrates. Vol. 22, Trends in Parasitology. Elsevier Curr Trends (2006), 219–25. doi: 10.1016/j.pt.2006.02.014
23. Bahia AC, Kubota MS, Tempone AJ, Araujo HR, Guedes BA, Orfano AS, et al. The JAK-STAT Pathway Controls Plasmodium Vivax Load in Early Stages of Anopheles Aquasalis Infection. PloS Negl Trop Dis (2011) 5(11):e1317. doi: 10.1371/journal.pntd.0001317
24. Luckhart S, Vodovotz Y, Cui L, Rosenberg R. The Mosquito Anopheles Stephensi Limits Malaria Parasite Development With Inducible Synthesis of Nitric Oxide. Proc Natl Acad Sci USA (1998) 95(10):5700–5. doi: 10.1073/pnas.95.10.5700
25. Larkin A, Marygold SJ, Antonazzo G, Attrill H, dos Santos G, Garapati PV, et al. FlyBase: Updates to the Drosophila Melanogaster Knowledge Base. Nucleic Acids Res (2021) 49(D1):D899–907. doi: 10.1093/nar/gkaa1026
26. Giraldo-Calderón GI, Emrich SJ, MacCallum RM, Maslen G, Emrich S, Collins F, et al. VectorBase: An Updated Bioinformatics Resource for Invertebrate Vectors and Other Organisms Related With Human Diseases. Nucleic Acids Res (2015) 43(D1):D707–13. doi: 10.1093/nar/gku1117
27. Altschul SF, Gish W, Miller W, Myers EW, Lipman DJ. Basic Local Alignment Search Tool. J Mol Biol (1990) 215(3):403–10. doi: 10.1016/S0022-2836(05)80360-2
28. Blum M, Chang HY, Chuguransky S, Grego T, Kandasaamy S, Mitchell A, et al. The InterPro Protein Families and Domains Database: 20 Years on. Nucleic Acids Res (2021) 49(D1):D344–54. doi: 10.1093/nar/gkaa977
29. Edgar RC. MUSCLE: Multiple Sequence Alignment With High Accuracy and High Throughput. Nucleic Acids Res [Internet] (2004) 32(5):1792–7. doi: 10.1093/nar/gkh340
30. Kumar S, Stecher G, Li M, Knyaz C, Tamura K. MEGA X: Molecular Evolutionary Genetics Analysis Across Computing Platforms. Mol Biol Evol [Internet] (2018) 35:1547–9. doi: 10.1093/molbev/msy096
31. Kõressaar T, Lepamets M, Kaplinski L, Raime K, Andreson R, Remm M. Primer3-Masker: Integrating Masking of Template Sequence With Primer Design Software. Bioinformatics (2018) 34(11):1937–8. doi: 10.1093/bioinformatics/bty036
32. Nadkarni MA, Martin FE, Jacques NA, Hunter N. Determination of Bacterial Load by Real-Time PCR Using a Broad-Range (Universal) Probe and Primers Set. Microbiol [Internet] (2002) 148(1):257–66. doi: 10.1099/00221287-148-1-257
33. Heerman M, Weng JL, Hurwitz I, Durvasula R, Ramalho-Ortigao M. Bacterial Infection and Immune Responses in Lutzomyia Longipalpis Sand Fly Larvae Midgut. PloS Negl Trop Dis [Internet] (2015) 9(7):e0003923. doi: 10.1371/journal.pntd.0003923
34. Telleria EL, Pitaluga AN, Ortigão-Farias JR, De Araújo APO, Ramalho-Ortigão JM, Traub-Cseko YM. Constitutive and Blood Meal-Induced Trypsin Genes in Lutzomyia Longipalpis. Arch Insect Biochem Physiol (2007) 66(2):53–63. doi: 10.1002/arch.20198
35. Di-Blasi T, Telleria EL, Marques C, De Macedo Couto R, Da Silva-Neves M, Jancarova M, et al. Lutzomyia Longipalpis Tgf-β has a Role in Leishmania Infantum Chagasi Survival in the Vector. Front Cell Infect Microbiol (2019) 9(MAR):71. doi: 10.3389/fcimb.2019.00071
36. Di-Blasi T, Lobo AR, Nascimento LM, Córdova-Rojas JL, Pestana K, Marín-Villa M, et al. The Flagellar Protein FLAG1/SMP1 is a Candidate for Leishmania-Sand Fly Interaction. Vector-Borne Zoonotic Dis [Internet] (2015) 15(3):202–9. doi: 10.1089/vbz.2014.1736
37. Meireles-Filho AC, Amoretty PR, Souza NA, Kyriacou CP, Peixoto AA. Rhythmic Expression of the Cycle Gene in a Hematophagous Insect Vector. BMC Mol Biol (2006) 7:38. doi: 10.1186/1471-2199-7-38
38. Molina-Cruz A, DeJong RJ, Charles B, Gupta L, Kumar S, Jaramillo-Gutierrez G, et al. Reactive Oxygen Species Modulate Anopheles Gambiae Immunity Against Bacteria and Plasmodium. J Biol Chem (2008) 283(6):3217–23. doi: 10.1074/jbc.M705873200
39. Lawyer P, Killick-Kendrick M, Rowland T, Rowton E, Volf P. Laboratory Colonization and Mass Rearing of Phlebotomine Sand Flies (Diptera, Psychodidae). Parasite (2017) 24:42. doi: 10.1051/parasite/2017041
40. Sant’Anna MR, Alexander B, Bates PA, Dillon RJ. Gene Silencing in Phlebotomine Sand Flies: Xanthine Dehydrogenase Knock Down by dsRNA Microinjections. Insect Biochem Mol Biol (2008) 38(6):652–60. doi: 10.1016/j.ibmb.2008.03.012
41. Telleria EL, Tinoco-Nunes B, Leštinová T, de Avellar LM, Tempone AJ, Pitaluga AN, et al. Lutzomyia Longipalpis Antimicrobial Peptides: Differential Expression During Development and Potential Involvement in Vector Interaction With Microbiota and Leishmania. Microorganisms [Internet] (2021) 9(6):1271. doi: 10.3390/microorganisms9061271
42. Schmittgen TD, Livak KJ. Analyzing Real-Time PCR Data by the Comparative CT Method. Nat Protoc (2008) 3(6):1101–8. doi: 10.1038/nprot.2008.73
43. Myskova J, Votypka J, Volf P. Leishmania in Sand Flies: Comparison of Quantitative Polymerase Chain Reaction With Other Techniques to Determine the Intensity of Infection. J Med Entomol [Internet] (2008) 45(1):133–8. doi: 10.1093/jmedent/45.1.133
44. Sadlova J, Price HP, Smith BA, Votypka J, Volf P, Smith DF. The Stage-Regulated HASPB and SHERP Proteins Are Essential for Differentiation of the Protozoan Parasite Leishmania Major in Its Sand Fly Vector, Phlebotomus Papatasi. Cell Microbiol [Internet] (2010) 12(12):1765–79. doi: 10.1111/j.1462-5822.2010.01507.x
45. Coutinho-Abreu IV, Serafim TD, Meneses C, Kamhawi S, Oliveira F, Valenzuela JG. Leishmania Infection Induces a Limited Differential Gene Expression in the Sand Fly Midgut. BMC Genomics (2020) 21(1):1–16. doi: 10.1186/s12864-020-07025-8
46. Engel P, Moran NA. The Gut Microbiota of Insects - Diversity in Structure and Function. FEMS Microbiol Rev [Internet] (2013) 37(5):699–735. doi: 10.1111/1574-6976.12025
47. Telleria EL, Martins-Da-Silva A, Tempone AJ, Traub-Cseko YM. Leishmania, Microbiota and Sand Fly Immunity. Parasitol [Internet] (2018) 145(10):1336–53. doi: 10.1017/S0031182018001014
48. Telleria EL, Sant’Anna MRV, Alkurbi MO, Pitaluga AN, Dillon RJ, Traub-Csekö YM. Bacterial Feeding, Leishmania Infection and Distinct Infection Routes Induce Differential Defensin Expression in Lutzomyia Longipalpis. Parasites Vectors [Internet] (2013) 6:12. doi: 10.1186/1756-3305-6-12
49. Liongue C, Ward AC. Evolution of the JAK-STAT Pathway. JAK-STAT (2013) 2(1):e22756. doi: 10.4161/jkst.22756
50. Kemp C, Mueller S, Goto A, Barbier V, Paro S, Bonnay F, et al. Broad RNA Interference–Mediated Antiviral Immunity and Virus-Specific Inducible Responses in Drosophila. J Immunol (2013) 190(2):650–8. doi: 10.4049/jimmunol.1102486
51. Paradkar PN, Trinidad L, Voysey R, Duchemin JB, Walker PJ. Secreted Vago Restricts West Nile Virus Infection in Culex Mosquito Cells by Activating the Jak-STAT Pathway. Proc Natl Acad Sci USA (2012) 109(46):18915–20. doi: 10.1073/pnas.1205231109
52. Behura SK, Gomez-Machorro C, Harker BW, DeBruyn B, Lovin DD, Hemme RR, et al. Global Cross-Talk of Genes of the Mosquito Aedes Aegypti in Response to Dengue Virus Infection. PloS Negl Trop Dis (2011) 5(11):e1385. doi: 10.1371/journal.pntd.0001385
53. Souza-Neto JA, Sim S, Dimopoulos G. An Evolutionary Conserved Function of the JAK-STAT Pathway in Anti-Dengue Defense. Proc Natl Acad Sci USA (2009) 106(42):17841–6. doi: 10.1073/pnas.0905006106
54. Angleró-Rodríguez YI, MacLeod HJ, Kang S, Carlson JS, Jupatanakul N, Dimopoulos G. Aedes Aegypti Molecular Responses to Zika Virus: Modulation of Infection by the Toll and Jak/Stat Immune Pathways and Virus Host Factors. Front Microbiol (2017) 8(OCT). doi: 10.3389/fmicb.2017.02050
55. Gupta L, Molina-Cruz A, Kumar S, Rodrigues J, Dixit R, Zamora RE, et al. The STAT Pathway Mediates Late-Phase Immunity Against Plasmodium in the Mosquito Anopheles Gambiae. Cell Host Microbe (2009) 5(5):498–507. doi: 10.1016/j.chom.2009.04.003
56. Tesh RB, Modi GB. Development of a Continuous Cell Line From the Sand Fly Lutzomyia Longipalpis (Diptera: Psychodidae), and Its Susceptibility to Infection With Arboviruses. J Med Entomol [Internet] (1983) 20(2):199–202. doi: 10.1093/jmedent/20.2.199
57. Ferreira FV, Aguiar ERGR, Olmo RP, de Oliveira KPV, Silva EG, Sant’Anna MRV, et al. The Small Non-Coding RNA Response to Virus Infection in the Leishmania Vector Lutzomyia Longipalpis. PloS Negl Trop Dis (2018) 12(6):e0006569. doi: 10.1371/journal.pntd.0006569
58. Martins-da-Silva A, Telleria EL, Batista M, Marchini FK, Traub-Csekö YM, Tempone AJ. Identification of Secreted Proteins Involved in Nonspecific dsRNA-Mediated Lutzomyia Longipalpis LL5 Cell Antiviral Response. Viruses (2018) 10(1):43. doi: 10.3390/v10010043
59. Soares RP, Altoe ECF, Ennes-Vidal V, da Costa SM, Rangel EF, de Souza NA, et al. In Vitro Inhibition of Leishmania Attachment to Sandfly Midguts and LL-5 Cells by Divalent Metal Chelators, Anti-Gp63 and Phosphoglycans. Protist [Internet] (2017) 168(3):326–34. doi: 10.1016/j.protis.2017.03.004
60. Böhmer F-D, Friedrich K. Protein Tyrosine Phosphatases as Wardens of STAT Signaling. JAK-STAT (2014) 3(1):e28087. doi: 10.4161/jkst.28087
61. Rey GJ, Ferro C, Bello FJ. Establishment and Characterization of a New Continuous Cell Line From Lutzomyia Longipalpis (Diptera : Psychodidae) and Its Susceptibility to Infections With Arboviruses and Leishmania Chagasi. Mem Inst Oswaldo Cruz (2000) 95(1):103–10. doi: 10.1590/S0074-02762000000100017
62. Bello FJ, Mejia AJ, Corena Mdel P, Ayala M, Sarmiento L, Zuniga C, et al. Experimental Infection of Leishmania (L.) Chagasi in a Cell Line Derived From Lutzomyia Longipalpis (Diptera : Psychodidae). Mem Inst Oswaldo Cruz (2005) 100(6):519–25. doi: 10.1590/s0074-02762005000600004
63. Terradas G, Joubert DA, McGraw EA. The RNAi Pathway Plays a Small Part in Wolbachia-Mediated Blocking of Dengue Virus in Mosquito Cells. Sci Rep (2017) 7:43847. doi: 10.1038/srep43847
64. Atayde VD, Hassani K, da Silva Lira Filho A, Borges AR, Adhikari A, Martel C, et al. Leishmania Exosomes and Other Virulence Factors: Impact on Innate Immune Response and Macrophage Functions. Cell Immunol (2016) 309:7–18. doi: 10.1016/j.cellimm.2016.07.013
65. Forrest DM, Batista M, Marchini FK, Tempone AJ, Traub-Csekö YM. Proteomic Analysis of Exosomes Derived From Procyclic and Metacyclic-Like Cultured Leishmania Infantum Chagasi. J Proteomics (2020) 227:103902. doi: 10.1016/j.jprot.2020.103902
66. Nandan D, Yi T, Lopez M, Lai C, Reiner NE. Leishmania EF-1α Activates the Src Homology 2 Domain Containing Tyrosine Phosphatase SHP-1 Leading to Macrophage Deactivation. J Biol Chem (2002) 277(51):50190–7. doi: 10.1074/jbc.M209210200
67. Olivier M, Atayde VD, Isnard A, Hassani K, Shio MT. Leishmania Virulence Factors: Focus on the Metalloprotease GP63. Microbes Infect (2012) 14(15):1377–89. doi: 10.1016/j.micinf.2012.05.014
68. Yao C, Donelson JE, Wilson ME. The Major Surface Protease (MSP or GP63) of Leishmania Sp. Biosynthesis, Regulation of Expression, and Function. Mol Biochem Parasitol (2003) 132(1):1–16. doi: 10.1016/s0166-6851(03)00211-1
69. Sloan MA, Sadlova J, Lestinova T, Sanders MJ, Cotton JA, Volf P, et al. The Phlebotomus Papatasi Systemic Transcriptional Response to Trypanosomatid-Contaminated Blood Does Not Differ From the Non-Infected Blood Meal. Parasites Vectors [Internet] (2021) 14(1):1–14. doi: 10.1186/s13071-020-04498-0
70. Sadlova J, Volf P. Peritrophic Matrix of Phlebotomus Duboscqi and Its Kinetics During Leishmania Major Development. Cell Tissue Res (2009) 337(2):313–25. doi: 10.1007/s00441-009-0802-1
71. Secundino NF, Eger-Mangrich I, Braga EM, Santoro MM, Pimenta PF. Lutzomyia Longipalpis Peritrophic Matrix: Formation, Structure, and Chemical Composition. J Med Entomol (2005) 42(6):928–38. doi: 10.1093/jmedent/42.6.928
72. Tanji T, Hu XD, Weber ANR, Ip YT. Toll and IMD Pathways Synergistically Activate an Innate Immune Response in Drosophila Melanogaster. Mol Cell Biol [Internet] (2007) 27(12):4578–88. doi: 10.1128/MCB.01814-06
73. De Gregorio E, Spellman PT, Tzou P, Rubin GM, Lemaitre B. The Toll and Imd Pathways Are the Major Regulators of the Immune Response in Drosophila. EMBO J [Internet] (2002) 21(11):2568–79. doi: 10.1093/emboj/21.11.2568
74. Freitas VC, Parreiras KP, Duarte AP, Secundino NF, Pimenta PF. Development of Leishmania (Leishmania) Infantum Chagasi in Its Natural Sandfly Vector Lutzomyia Longipalpis. Am J Trop Med Hyg (2012) 86(4):606–12. doi: 10.4269/ajtmh.2012.11-0386
75. Kelly PH, Bahr SM, Serafim TD, Ajami NJ, Petrosino JF, Meneses C, et al. The Gut Microbiome of the Vector Lutzomyia Longipalpis Is Essential for Survival of Leishmania Infantum. MBio [Internet] (2017) 8(1):e01121–16. doi: 10.1128/mBio.01121-16
76. Dey R, Joshi AB, Oliveira F, Pereira L, Guimaraes-Costa AB, Serafim TD, et al. Gut Microbes Egested During Bites of Infected Sand Flies Augment Severity of Leishmaniasis via Inflammasome-Derived IL-1beta. Cell Host Microbe [Internet] (2018) 23(1):134–43.e6. doi: 10.1016/j.chom.2017.12.002
77. Monteiro CC, Inbar E, Ghosh K, Merkhofer R, Lawyer P, Paun A, et al. The Midgut Microbiota Plays an Essential Role in Sand Fly Vector Competence for Leishmania Major. Cell Microbiol (2017) 19(10):1–20. doi: 10.1111/cmi.12755
78. Campolina TB, Villegas LEM, Monteiro CC, Pimenta PFP, Secundino NFC. Tripartite Interactions: Leishmania, Microbiota and Lutzomyia Longipalpis. PloS Negl Trop Dis [Internet] (2020) 14(10):e0008666. doi: 10.1371/journal.pntd.0008666
79. Warburg A, Pimenta PF. A Cytoplasmic Polyhedrosis Virus in the Phlebotomine Sandfly Lutzomyia Longipalpis. Med Vet Entomol [Internet] (1995) 9(2):211–3. doi: 10.1111/j.1365-2915.1995.tb00182.x
80. da Silva L. Thais (Fundação Oswaldo Cruz. Instituto Oswaldo Cruz. Rio De Janeiro, RJ B. Estudo De Genes De Leishmania Infantum Chagasi Expressos Durante a Infecção Em Lutzomyia Longipalpis, Principal Vetor Da Leishmaniose Visceral No Brasil [Internet] (2017). Available at: https://www.arca.fiocruz.br/handle/icict/23792 (Accessed 2021 Nov 1).
81. Lee KA, Kim B, Bhin J, Kim DH, You H, Kim EK, et al. Bacterial Uracil Modulates Drosophila DUOX-Dependent Gut Immunity via Hedgehog-Induced Signaling Endosomes. Cell Host Microbe (2015) 17(2):191–204. doi: 10.1016/j.chom.2014.12.012
82. Ha EM, Oh CT, Bae YS, Lee WJ. A Direct Role for Dual Oxidase in Drosophila Gut Immunity. Science (80-) (2005) 310(5749):847–50. doi: 10.1126/science.1117311
83. Rajan A, Perrimon N. Drosophila Cytokine Unpaired 2 Regulates Physiological Homeostasis by Remotely Controlling Insulin Secretion. Cell (2012) 151(1):123–37. doi: 10.1016/j.cell.2012.08.019
84. Zhou F, Rasmussen A, Lee S, Agaisse H. The UPD3 Cytokine Couples Environmental Challenge and Intestinal Stem Cell Division Through Modulation of JAK/STAT Signaling in the Stem Cell Microenvironment. Dev Biol (2013) 373(2):383–93. doi: 10.1016/j.ydbio.2012.10.023
Keywords: sand fly, innate immunity, RNAi gene silencing, Leishmania, microbiota, vector-pathogen interaction
Citation: Telleria EL, Azevedo-Brito DA, Kykalová B, Tinoco-Nunes B, Pitaluga AN, Volf P and Traub-Csekö YM (2021) Leishmania infantum Infection Modulates the Jak-STAT Pathway in Lutzomyia longipalpis LL5 Embryonic Cells and Adult Females, and Affects Parasite Growth in the Sand Fly. Front. Trop. Dis 2:747820. doi: 10.3389/fitd.2021.747820
Received: 26 July 2021; Accepted: 10 November 2021;
Published: 02 December 2021.
Edited by:
Yoosook Lee, University of Florida, United StatesReviewed by:
Derrick Mathias, University of Florida, United StatesOscar David Kirstein, Emory University, United States
Copyright © 2021 Telleria, Azevedo-Brito, Kykalová, Tinoco-Nunes, Pitaluga, Volf and Traub-Csekö. This is an open-access article distributed under the terms of the Creative Commons Attribution License (CC BY). The use, distribution or reproduction in other forums is permitted, provided the original author(s) and the copyright owner(s) are credited and that the original publication in this journal is cited, in accordance with accepted academic practice. No use, distribution or reproduction is permitted which does not comply with these terms.
*Correspondence: Yara Maria Traub-Csekö, eXRyYXViQGlvYy5maW9jcnV6LmJy
†These authors share first authorship