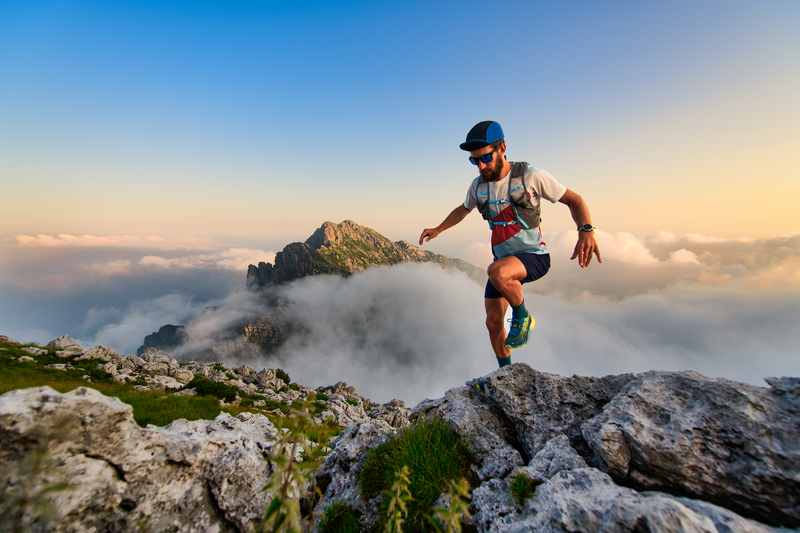
95% of researchers rate our articles as excellent or good
Learn more about the work of our research integrity team to safeguard the quality of each article we publish.
Find out more
ORIGINAL RESEARCH article
Front. Trop. Dis. , 17 December 2021
Sec. Vaccines for Tropical Diseases
Volume 2 - 2021 | https://doi.org/10.3389/fitd.2021.729731
This article is part of the Research Topic Vaccines against Enteric Infections View all 4 articles
Shigellosis is a severe diarrheal disease caused by members of the genus Shigella, with at least 80 million cases and 700,000 deaths annually around the world. The type III secretion system (T3SS) is the primary virulence factor used by the shigellae, and we have previously demonstrated that vaccination with the type T3SS proteins IpaB and IpaD, along with an IpaD/IpaB fusion protein (DBF), protects mice from Shigella infection in a lethal pulmonary model. To simplify the formulation and development of the DBF Shigella vaccine, we have genetically fused LTA1, the active subunit of heat-labile toxin from enterotoxigenic E. coli, with DBF to produce the self-adjuvanting antigen L-DBF. Here we immunized mice with L-DBF via the intranasal, intramuscular, and intradermal routes and challenged them with a lethal dose of S. flexneri 2a. While none of the mice vaccinated intramuscularly or intradermally were protected, mice vaccinated with L-DBF intranasally were protected from lethal challenges with S. flexneri 2a, S. flexneri 1b, S. flexneri 3a, S. flexneri 6, and S. sonnei. Intranasal L-DBF induced both B cell and T cell responses that correlated with protection against Shigella infection. Our results suggest that L-DBF is a candidate for developing an effective serotype-independent vaccine against Shigella spp.
The shigellae are intracellular bacteria that cause the intestinal disease shigellosis, which can result in severe diarrhea or dysentery. Shigellosis is a significant public health problem, with children especially vulnerable to increased morbidity and mortality (1, 2). While most cases of shigellosis occur in developing nations, the shigellae also cause diarrhea among travelers and military personnel from developed countries (3). Shigella spp. include S. dysenteriae (Group A), S. flexneri (Group B), S. boydii (Group C), and S. sonnei (Group D), which are further divided into more than 50 serotypes based on O-antigen composition (3). S. flexneri is the primary cause of endemic diarrhea in developing countries where there is limited access to hygienic resources, whereas S. sonnei is the leading cause of illness in developed countries (3). In addition, S. flexneri is responsible for a greater number of total deaths from shigellosis than the other species, with serotypes 1b, 2a, 3a, 4a, and 6 commonly found in less developed countries and serotype 2a dominant in moderately developed countries (1). Recent studies have shown that S. sonnei infections are increasing and replacing S. flexneri as a cause of shigellosis in areas as they undergo modernization (4), evincing the need for a serotype-independent Shigella vaccine.
Although there has been a reduction in the incidence of shigellosis globally due to improved sanitation, the rise of antimicrobial resistance in Shigella spp. warrants the development of a vaccine against this pathogen (5, 6). At present, there is no licensed Shigella spp. vaccine, however, some killed cell and live-attenuated vaccines are currently in clinical trials. Unfortunately, the lack of cross-protection, strict storage conditions, and potential risks of contamination limit their use in developing countries (7). To solve these issues, subunit vaccines, especially those utilizing proteins from the type three secretion system (T3SS) (see Supplementary Table S1 for the abbreviations used here), have been widely researched (7).
The T3SS is a required virulence factor for the shigellae (8). The T3SS apparatus (T3SA) tip protein, IpaD, and translocator, IpaB, are highly conserved among Shigella spp., making them excellent targets for the development of a serotype-independent subunit vaccine (8). Research in our lab has established that these two proteins, with the adjuvant dmLT (double-mutant heat-labile enterotoxin from enterotoxigenic E. coli (ETEC)), can elicit cross protection against S. flexneri and S. sonnei when delivered intranasally (IN) (9). To reduce production costs, we made DBF, a genetic fusion of IpaD and IpaB (10). DBF adjuvanted with dmLT induced comparable immune responses in both B and T cells to those stimulated by the mixture of IpaD and IpaB (10). Most importantly, DBF admixed with dmLT and administered IN protected mice in lethal challenges with the homologous S. flexneri, from which the IpaD and IpaB sequences are derived, and in challenges with the heterologous S. sonnei and S. dysenteriae (10).
The dmLT, an AB5 toxoid which induce anti-LT response, retains the native ADP-ribosyltransferase activity that induces a strong Th17 response (11). Studies have shown that pre-existing antibodies to dmLT did not disturb its adjuvanticity to a new antigen. This suggested that anti-LT antibodies from pre-exposures of ETEC, which commonly happen in developing countries, would not affect the adjuvant effects of LTA1 (12). Th17 responses are known to be especially important for protection against mucosal pathogens, including Shigella (13, 14). Unfortunately, recent studies showed that dmLT, when delivered IN, can cause Bell’s palsy (11, 15, 16), however, the development of Bell’s palsy from dmLT is related to the ability of the B subunit to bind to the gangliosides of neuron cells. It is the LTA1 (heat-labile enterotoxin A1) portion of the A subunit that is responsible for generating the Th17 response (15, 17, 18). To simplify and lower the costs of producing a Shigella spp. vaccine for use in developing nations, we genetically fused LTA1 to DBF to create a monomeric adjuvant-antigen conjugate called L-DBF.
In this study, we demonstrate that IN administration of L-DBF protects mice against a lethal pulmonary challenge with S. flexneri 2a, and this protection is associated with significant Th1 and Th17 responses. Additionally, we show that IN immunization with L-DBF protects mice against lethal challenges with heterologous S. flexneri 1b, S. flexneri 3a, S. flexneri 6, and S. sonnei. These results show that L-DBF elicits broad protective efficacy against multiple Shigella serotypes and is thus a viable vaccine candidate against shigellosis.
pACYCDuet-1 plasmid, ligation mix and competent E. coli were from EMD Millipore (Billerica, MA). Restriction endonucleases were from New England Biolabs (Ipswich, MA). Chromatography columns were from GE Healthcare (Piscataway, NJ). All other reagents were from Sigma or Fisher Scientific. dmLT was from J. Clements and E. Norton (Tulane School of Medicine, New Orleans, LA). S. flexneri 2a 2457T was from A.T. Maurelli (University of Florida, Gainesville, FL). S. flexneri 1b, S. flexneri 3a, S. flexneri 6, and S. sonnei were from Eileen Barry (University of Maryland School of Medicine, Baltimore, MD).
Production of IpaD, IpaB and DBF have been described previously (10, 19). To produce LTA1-GSAAS-DBF (L-DBF), eltA1 (the coding sequence for LTA1) was cloned in-frame with the linker gggtccgcggcatcc 5’ to ipaD in the IpaD-IpaB+IpgC/pACYCDuet-1 plasmid. The resulting plasmid (eltA1-ipaD-ipaB/ipgC//pACYCDuet-1) was used to transform E. coli Tuner (DE3) for co-expression of L-DBF and the IpgC chaperone with the latter possessing a His affinity tagee (HT-IpgC). The transformed bacteria were grown in a fed-batch mode using a 10L bioreactor (Labfors 5, Infors USA Inc., MD) equipped with polarographic dissolved oxygen probe (pO2), pH probe (Hamilton Company) and advanced fermentation software. Materials were prepared as per the manufacturer’s specifications (http://www.infors-ht.com.cn/uploadfile/2018/0515/INFORS-HT_cookbook_en.pdf). Briefly, pre-culture was prepared by inoculating a 25-μl aliquot of frozen glycerol stock into 50 mL of Terific broth (TB) supplemented with chloramphenicol (34 µg/ml) and allowed to grow overnight at 30°C with shaking at 200 rpm. The inoculum was made by transferring cells from the pre-culture to 1 L of TB with the same antibiotic and grown at 30°C until reaching an A600 of ∼2.0. Then, ~800 mL of inoculum was transferred to the sterilized bioreactor containing 9 L of TB containing chloramphenicol. The culture was maintained at 30°C and pH 7, and stirrer speed, gas mix, and gas flow were adjusted to maintain pO2 (30%). Protein expression was induced by addition of IPTG to 1 mM when the culture reached an A600 of ∼25. After 3 h, bacteria were collected by centrifugation, washed and resuspended in IMAC binding buffer (20 mM Tris-HCl pH 7.9, 500 mM NaCl, 10 mM imidazole) with 0.1 mM AEBSF Protease Inhibitor and lysed using a microfluidizer at 18,000 psi with three passes. The cellular debris was removed by centrifugation at 10,000xg for 30 min and loaded onto a 5 ml HisTrap FF column. The L-DBF/HT-IpgC was eluted with IMAC elution buffer (20 mM Tris-HCl pH 8.0, 500 mM NaCl, 500 mM imidazole), dialyzed into 50 mM Tris-HCl pH 8.0, and loaded onto a HiTrap Q FF column. The complex was eluted using a gradient of 50 mM Tris-HCl pH 8 containing 1M NaCl. Lauryldimethylamine oxide (LDAO) was then added to a final concentration of 0.1% to release the HT-IpgC. When the LDAO-treated L-DBF/HT-IpgC complex was passed over an IMAC column, the L-DBF was collected in the flow-through with the HT-IpgC being retained on the IMAC column. Finally, L-DBF was dialyzed into 20 mM phosphate, pH 7.2, with 150 mM NaCl (PBS) with 0.05% LDAO and stored at -80°C. LPS levels were determined using a NexGen PTS with EndoSafe cartridges (Charles River Laboratories, Wilmington, MA). All proteins had LPS levels <5 Endotoxin units/mg.
LTA1 from ETEC labile-toxin possesses ADP-ribosyltransferase (ADPr) activity, which is required for adjuvanticity (12). To assay for L-DBF ADPr activity, L-DBF was added to ADPr buffer (50 mM Tris-HCl pH 7.5, 1 mM EDTA, 1 mM DTT) to a concentration of 1.7 µM, with or without 1.7 µM ARF4, the LTA1 allosteric activator protein. Biotinylated-NAD+ was then added to a concentration of 8.3 µM and the mixture incubated at 37°C for one hour. The reaction mixtures were then subjected to SDS-PAGE and then electroblotted onto a nitrocellulose membrane. The membrane was then incubated in TBS buffer containing IRDye 800CW streptavidin (LI-COR, Lincoln, NE), washed, and imaged on a LI-COR Odyssey CLx gel scanner.
The mouse animal protocols were reviewed and approved by the University of Kansas Institutional Animal Care and Use Committee Practices (protocol AUS 222-01). Female 6-8 weeks BALB/c mice were used in this study (n=10/group). For the initial intranasal (IN) vaccination trial, 20 µg DBF + 2.5 µg dmLT and 25 µg L-DBF were prepared in 30 µl per mouse for each IN vaccination. For the intradermal (ID) trial, 100, 250 and 500 ng L-DBF were diluted to 50 µl per mouse. For the intramuscular (IM) trial, 80 µg L-DBF + 2.5 µg dmLT and 80 µg L-DBF were prepared in 30 µl volume for each mouse. For the IN dose escalation (n=14/group; 10 for challenge and 4 for pre-challenge immune response assessment), 1, 10, and 25 µg L-DBF were prepared in 30 µl volume for each mouse. In a separate experiment, (n=14/group; 10 for challenge and 4 for pre-challenge immune response assessment), 15, and 25 µg L-DBF were prepared in 30 µl volume for each mouse. To test for cross-protection, 25 µg L-DBF and PBS alone were prepared in 30 µl. All mice, regardless of route, were immunized on days 0, 14 and 28.
Shigella challenge strains were streaked onto tryptic soy agar containing 0.025% Congo red and incubated at 37°C overnight and subcultured in tryptic soy broth (TSB) at 37°C until A600 reached 1.0. Bacteria were harvested by centrifugation, resuspended in PBS and diluted to the desired concentration in a 30 µl volume for IN challenge (20). In the cross-protection study, L-DBF 25 µg and PBS vaccinated mice (n=10 of each group/serotype) were challenged on day 56 with S. flexneri 2a (6 x 106 CFU/30 µl), S. flexneri 3a (1 x 106 CFU/30 µl), S. flexneri 6 (1 x 106 CFU/30 µl), S. flexneri 1b (4 x 106 CFU/30 µl), or S. sonnei (1 x 106 CFU/30 µl). Mice were monitored twice a day for weight loss and health score for two weeks. Mice were euthanized if their weight loss exceeded 25% of their original weight for more than 72 h or their blood glucose reached ≤100 mg/dL with poor health scores.
Blood and feces were collected on days 0, 13, 27, 41, and 55 for antibody detection during the immunization, as described previously with minor modifications (19). Microtiter wells were coated with 100 ng IpaB or IpaD in 100 µl PBS, incubated at 37°C for 3 h and then blocked overnight with 10% nonfat dry milk in PBS. Sera were added to the wells in duplicates as the primary antibody for 2 h incubation at 37°C. After washing with PBS-0.05% Tween, HRP-conjugated secondary antibody (IgG(H+L), 1:1000; IgA, 1:500) was added and incubated for 1 h at 37°C. After an additional wash, OPD substrate (o-phenylenediamine dihydrochloride) was added and the resulting signal detected at 490nm. Endpoint titers were determined by fitting antibody titrations to a five-parameter logistic model.
Single cell suspensions from mouse spleens and lungs were isolated using Spleen or Lung Dissociation Kit (Miltenyi Biotec, Inc), and were incubated for 24 h at 37°C in the presence of 5 µg/ml IpaB or IpaD in plates coated with antibodies against IFN-γ or IL-17A using a FluoroSpot assay as per manufacture’s specifications (Cellular Technology Limited). The cytokine secreting cells were quantified using a CTL immunospot reader.
Splenocytes and lung cells were incubated with 10 µg/ml IpaB, IpaD or PBS for 48 h at 37°C. Supernatants were collected and analyzed with U-PLEX kits for cytokines: IFN-γ, IL-17A, IL-6, and TNF-α. Cytokine concentrations were determined using an MSD plate reader with associated analytical software (Meso Scale Discovery, Rockville, MD).
Graphs were generated using GraphPad Prism 9.0.1. ELISpot and cytokine secretion were rescaled to the range between zero and one using the minimum-maximum (min-max) normalization equation [Ynormal=(Yorigin-Ymin)/(Ymax-Ymin)] for the purpose of plotting comparative data. The significance of differences among treatment groups was determined using ANOVA. Post-hoc comparisons of unvaccinated (PBS) mice with antigen vaccinated mice were made with Dunnett’s test in R. A p-value of less than 0.05 was considered significant for all comparisons (*p < 0.05; **p < 0.01; ***p < 0.001). For bacterial challenges, vaccinated groups were compared to PBS using Log-rank (Mantel-Cox) tests in GraphPad Prism.
When incubated with biotin-labeled NAD+, L-DBF was found to conjugate biotinylated ADP-ribose to itself and to the LTA1 allosteric activator protein ARF4 (Supplementary Figure S1). This result does not in itself guarantee that the LTA1 domain retains adjuvanticity, however, the absence of enzymatic activity would have precluded the use of L-DBF as a self-adjuvanting vaccine candidate. It should be noted that recombinant LTA1 sequesters to E. coli inclusion bodies and must be refolded after solubilization in a denaturing agent such as urea. Because this would increase the difficulties encountered in formulation, the use of purified LTA1 with DBF was not included as a vaccine comparator in the studies that follow.
To demonstrate that L-DBF could protect mice against an S. flexneri challenge as well as DBF+dmLT does, we vaccinated mice intranasally (IN) with 20 µg DBF+2.5 µg dmLT, 25 µg L-DBF or PBS. After three vaccinations, the mice were challenged with of 6×106 CFU S. flexneri 2a 2457T (Figure 1). While 90% of the mice vaccinated with PBS died, all mice vaccinated with either DBF+dmLT or L-DBF survived (Figure 1). These results show that L-DBF has protective efficacy equivalent to that of DBF with dmLT and is a viable self-adjuvanting vaccine candidate.
Figure 1 Protective efficacy of L-DBF against lethal Shigella challenge in mice. Balb/C mice (n=10) were vaccinated intranasally (IN) on days 0, 14 and 28 with PBS, 20 µg DBF + 2.5 µg dmLT and 25 µg L-DBF. On day 56, the mice were challenged IN with 6 X 106 CFU/mouse of S. flexneri 2a 2457T. Significance was calculated by comparing vaccinated groups to the PBS group with Log-rank (Mantel-Cox) tests for survival (20 µg DBF + 2.5 µg dmLT vs. PBS: p < 0.001; 25 µg L-DBF vs. PBS: p < 0.001).
To determine whether other vaccination routes would also be protective, we immunized mice with L-DBF intramuscularly (IM) and intradermally (ID). Mice were vaccinated IM with 80 µg L-DBF or 80 µg L-DBF+2.5 µg dmLT, or ID with 100, 250 and 500 ng L-DBF. An additional 2.5 µg dmLT was added to one IM group to boost the production of IL-17A. As a positive control, mice were vaccinated IN with DBF+dmLT or L-DBF. In contrast to IN vaccinated mice, only 56% and <30% of the mice vaccinated IM and ID, respectively, survived (Table 1). The inclusion of additional adjuvant (dmLT) did not improve the protective capacity of the L-DBF administered IM (Table 1). Because the WHO has established >60% vaccine efficacy as a targeted cut-off value for a preferred Shigella spp. vaccine candidate (21), the IM and ID routes are thus considered not viable for L-DBF.
Five groups of ten mice were vaccinated three times IN with 25 µg L-DBF. An additional five groups were vaccinated with PBS as negative controls. Serum IgG and fecal IgA titers against IpaB and IpaD were then assessed (Supplementary Figure S2). All vaccinated mice had significantly higher serum anti-IpaB and anti-IpaD IgG and fecal IgA titers when compared to the negative control groups. One group of each of the L-DBF or PBS vaccinated mice were then challenged on day 56 with S. flexneri 2a (6 x 106 CFU), S. flexneri 3a (1 x 106 CFU), S. flexneri 6 (1 x 106 CFU), S. flexneri 1b (4 x 106 CFU), or S. sonnei 53G (1 x 106 CFU), based on the LD50 of each strain. All vaccinated groups showed >83% survival when challenged with an S. flexneri serotype, while protection against S. sonnei was 68% (Table 2 and Supplementary Figure S3). PBS vaccinated mice also showed greater weight loss and slower recovery than the L-DBF vaccinated mice (Supplementary Figure S3). The somewhat rapid weight gain seen for some of the PBS groups was a result of a small number of surviving mice, which skewed the average weight upward at later time points in the experiment. As with IpaB+IpaD or DBF, the protection seen here demonstrates the broad serotype-independent efficacy of L-DBF. Notably, there are no reports of any LPS-based vaccine formulations that provides protection against five Shigella serotypes simultaneously. Moreover, anti-Shigella immunity is serotype specific (22–24).
To characterize the dose response of L-DBF, we performed a dose escalation study by vaccinating mice IN with 1, 10, 15, or 25 µg of L-DBF. The resulting anti-IpaB and anti-IpaD serum IgG titers from the 10, 15 and 25 µg L-DBF doses were similar (Figures 2A, B – solid blue, yellow and red lines), while the titers for the 1 µg L-DBF dose were about one log unit lower (Figures 2A, B, solid green line). The anti-IpaB and anti-IpaD fecal IgA titers of 15 and 25 µg L-DBF doses groups were higher than 1 and 10 µg L-DBF doses groups on Day 42 and Day 55 (Figures 2A, B, dashed lines). Two challenge experiments were then performed. In the first, mice vaccinated IN with 1, 10, or 25 µg L-DBF were challenged with 1 x 107 CFU/mouse of S. flexneri 2a. The 25 µg L-DBF dose elicited 80% protection, while the 1 and 10 µg doses were not protective (1/10 mice was protected) (Supplementary Figure S4A). Because the high challenge dose resulted in some death within the mice receiving the highest vaccine dose, we performed a second challenge using a slightly lower challenge dose with mice vaccinated using 15 µg or 25 µg of L-DBF. In this case the mice were 100% protected from a challenge dose of 1.5 x 106 CFU/mouse (Supplementary Figure S4C). It is noted that while some mice failed to be protected at the higher challenge dose, the overall protection was not statistically different that the 100% protection at the lower challenge dose (p = 0.15). Likewise, while the 15 and 25 µg vaccine doses are somewhat high, it should be noted that the L-DBF is not yet formulated to maximize the host response to the vaccine. Formulation will be required prior to use in humans since monomeric subunit proteins are often not protective in humans (25, 26). We have determined that formulation of L-PaF, the Pseudomonas aeruginosa homologue of L-DBF, reduces the required antigen by 10-fold or more (27).
Figure 2 Kinetics of serum IgG and fecal IgA responses. Mice were vaccinated IN three times (Day 0, 14 and 28). Blood and fecal samples were collected and measured titers for anti-IpaB (A) or anti-IpaD (B) IgG (solid lines) and IgA (dotted lines) by ELISA. The individual titers are represented as EU ml-1. Each point represents the mean and error bars represent SD of each group (n=14/group).
Having demonstrated the successful protective efficacy of 15 and 25 µg L-DBF doses and the failure of 1 and 10 µg doses, the lungs and spleens from the mice were examined for cellular immune responses. Cell suspensions from each organ were stimulated with IpaB or IpaD and the frequency of cells secreting IL-17A and IFN-γ cells enumerated by ELISpot analysis (Figure 3 and Supplementary Figure S5). In both organs, mice vaccinated with 15 and 25 µg L-DBF generally have higher frequencies of IL-17a and IFN-γ secreting cells when stimulated with either IpaB or IpaD. Meanwhile the 1 and 10 μg doses do not generate secreting cell frequencies significantly higher than PBS.
Figure 3 Frequency of IL-17A and IFN-γ secreting cells after antigen-specific stimulation. Single cell spleen (left) and lung (right) suspensions were used to assess antigen specific IL-17A and IFN-γ secreting cells. Cells were incubated with 10 µg IpaB or IpaD. IL-17A and IFN-γ secreting cells were enumerated by ELISpot and are presented here as spot forming cells/106 cells. The original data were rescaled and normalized using the equation Ynormal=(Yorigin-Ymin)/(Ymax-Ymin). The data are boxed from minimum to maximum for each group after normalization. Significance was calculated by comparing groups that were unvaccinated (PBS) and mice vaccinated with antigens using Dunnett’s test. *p < 0.05; **p < 0.01; ***p < 0.001.
Using these same lung cells, we quantified secreted cytokine levels after stimulation with IpaB and IpaD (Figure 4 and Supplementary Figure S6). When compared to the PBS vaccinated group, significantly higher levels of IL-17A were secreted from lung cells from all four L-DBF vaccinated groups after stimulation with IpaB or IpaD. In contrast, IFN-γ secretion from lung cells was significantly higher in all four L-DBF groups as compared to PBS groups when stimulated with IpaB, but only in the 15 and 25 µg L-DBF vaccinated group after stimulation with IpaD. When examining other cytokines, only the 15 and 25 µg L-DBF vaccinated group elicited significantly higher IL-6 and TNF-α secretion after stimulation with IpaB or IpaD. No statistical difference was seen in secretion of these cytokines from splenocytes (Supplementary Figure S7).
Figure 4 Levels of T cell related cytokines secreted from lung cells after stimulation with IpaB or IpaD. The single lung cell suspensions were used to assess antigen specific IFN-γ, IL-17A, IL-6 and TNF-α secretion from lung cell suspensions. Cells were incubated with 10 µg IpaB (left) or IpaD (right). Cytokine levels were determined by Meso Scale Discovery analysis as per manufacturer’s specifications and are presented here as pg/ml/106 cells. The original data were rescaled and normalized for comparative purposes using the equation Ynormal=(Yorigin-Ymin)/(Ymax-Ymin). The data are boxed as minimum to maximum in each group after normalization. Significance was calculated by comparing groups that were unvaccinated (PBS) and mice vaccinated with antigens using Dunnett’s test. **p < 0.01; ***p < 0.001.
Diarrheal diseases are a severe global health problem. Shigellosis, in particular, is often lethal to children under five years of age, especially those living in developing countries where access to basic life-saving treatments and hygienic resources are limited (28). Although the morbidity and mortality of shigellosis have diminished in recent years, the emergence of antibiotic resistance among the shigellae, which can cause an infection with as few as 200 organisms, calls for an effective vaccine against shigellosis. Because target populations for vaccination reside in low and middle-income countries, a major concern in Shigella vaccine development is cost, which is negatively impacted by the need for a cold chain and the lack of public health resources. Broadly protective vaccines with simplified formulations and storage condition could meet the low-cost requirement.
In this study, we genetically fused the LTA1 subunit of dmLT with DBF. We found L-DBF provided comparable protection as DBF+dmLT when delivered IN but not when delivered via IM or ID routes. Vaccine efficacy depends on the route of administration. Before choosing the IN route, other routes, such as, ID, IM were tested with limited to no success, which was consistent with previous work (9) and unpublished results). Transport of the administered immunogen to the local lymph nodes are an important determinant towards generation of a strong humoral and cellular response. Previous studies showed IM injected immunogens to be transported to the local nodes and is not disseminated systematically. In case of mice, IM injected immunogens are generally processed in the subiliac and popliteal lymph nodes, which are far from mucosal sites. IN route, on the other hand, showed significantly better response due to the presence of a strong mucosal immune response against the immunogen (20, 29).
Additionally, L-DBF provides the much-desired cross-protection that is lacking with traditional LPS based vaccines including whole killed, live-attenuated and O-antigen based vaccines that are considered serotype specific. In contrast, the T3SS proteins IpaB and IpaD are conserved among all serotypes and have been considered attractive target antigens in subunit vaccine development (30, 31). Early studies showed that DBF with dmLT administered IN protected mice against S. flexneri, S. sonnei and S. dysenteriae. In this study, intranasal L-DBF vaccinated mice elicited effective protection against five Shigella spp. subtypes, S. flexneri 2a, S. flexneri 3a, S. flexneri 6, S. flexneri 1b, and S. sonnei 53G.
The increase in the frequency of IL-17A and IFN-γ secreting cells, as well as the secretion of these cytokines by lung cells from mice vaccinated with 15 and 25 µg L-DBF, suggests that IL-17 and IFN-γ responses are required for protection. Additionally, the lack of an IFN-γ response after stimulation of lung cells from the 1 and 10 µg vaccinated mice, coupled with the reduced protection at these doses, points to the importance of the IFN-γ response. While we have previously published that vaccine formulations containing IpaD and IpaB trigger IL-17A and IFN-γ responses (10, 19), we have not shown that the absence of such a response shows a parallel lack of protection. Furthermore, mice vaccinated with 15 and 25 µg L-DBF, which showed maximum protection against an S. flexneri challenge, elicited higher secretion of IL-6 and TNF-α. Pro-inflammatory mediators like TNF-α and IL-1β stimulate the expression of IL-6 (32). Recent studies showed that sIgA could induce IL-6 by normal human lung fibroblasts (NHLFs) (33). Moreover, sIgA has been found that could enhance Shigella taken up by M cells, which reduced the invasion of pathogens (34). Our results also detected higher titers of fecal IgA in mice vaccinated with 15 and 25 µg L-DBF, suggested a correlation between IgA and the IL-6 secretion. Further work will be required to demonstrate a correlation of protection of these cytokines.
A Th17 response is considered essential in the host immune defense against pathogens that target mucosal surfaces (35–37). Early studies suggested that a Th17 response was important for host survival in a lethal Shigella challenge (14, 38). Therefore, a vaccine that induces a mucosal Th17 response would be expected to increase its efficacy (13). Our lab has shown that intranasal DBF with the adjuvant dmLT induced significantly higher IL-17A in mouse models, which is associated with successful protection against lethal challenge (10). Because DBF alone does not induce IL-17 when administered IN, dmLT must be responsible for IL-17 stimulation. Similar outcomes were found in this study for L-DBF. The results shown here also suggest there is a dose-dependent increase in IFN-γ production in L-DBF vaccinated groups. Research has shown that Th1 plays a significant role in protection of mice after Shigella challenge (39, 40), with IFN-γ especially important for clearing intracellular Shigella via macrophage activation, which restricts intracellular growth of the pathogen (41, 42). Stimulation of high levels of both IL-17 and IFN-γ, as well as the Th17 and Th1 related cytokines IL-6 and TNF-α, demonstrate that L-DBF mainly elicits Th1 and Th17 responses to protect mice against S. flexneri infection. In this study, we did not detect IL-4 or IL-5 (data not shown), which are related to the Th2 differentiation and response (43).
These data show that the adjuvant-antigen conjugate L-DBF is a viable candidate for a broadly protective Shigella vaccine. Intranasal immunization with L-DBF induces strong anti-IpaB and anti-IpaD IgG responses, as well as significant Th1 and Th17 response in lung, yielding effective protection against lethal pulmonary challenges with five Shigella spp. strains. Furthermore, the single protein nature of the LTA1-DBF conjugate simplifies and reduces the cost of vaccine production and formulation. While the mouse pulmonary model is not ideal, it is accepted in the field (20). It is a simplified model to study pathobiology of human adapted Shigella spp. Like the intestine, the lung is a lymphoid organ with antigen presenting cells, T helper and suppressor cells and B cells. In addition, the bronchus constitutes a mucosal surface similar to the intestinal mucosa with occasional lymphoid follicles like Peyer’s Patches (PPs) (44).
The original contributions presented in the study are included in the article/Supplementary Material. Further inquiries can be directed to the corresponding author.
The animal study was reviewed and approved by Institutional Animal Care and Use Committee (IACUC).
WLP and WDP conceptualized the study. WLP, WDP, TL, and SD designed the study. TL, SD, DH, QZ, SR, and WDP performed experiments. TL, SD, DH, SW, WDP, and WLP analyzed the data. TL and SW performed statistical analysis. TL and WLP wrote the first draft of the manuscript with inputs from all authors. WDP and SW revised the manuscript. All authors contributed to the article and approved the submitted version.
This work was funded by NIAID grants R01AI138970 to WLP.
The authors declare that the research was conducted in the absence of any commercial or financial relationships that could be construed as a potential conflict of interest.
All claims expressed in this article are solely those of the authors and do not necessarily represent those of their affiliated organizations, or those of the publisher, the editors and the reviewers. Any product that may be evaluated in this article, or claim that may be made by its manufacturer, is not guaranteed or endorsed by the publisher.
We thank Francisco Martinez-Becerra and members of the Picking lab for critical reading of the manuscript. We also thank John Clements (Tulane University School of Medicine) providing the dmLT.
The Supplementary Material for this article can be found online at: https://www.frontiersin.org/articles/10.3389/fitd.2021.729731/full#supplementary-material
1. Jennison AV, Verma NK. Shigella Flexneri Infection: Pathogenesis and Vaccine Development. FEMS Microbiol Rev (2004) 28(1):43–58. doi: 10.1016/j.femsre.2003.07.002
2. WHO. Guidelines for the Control of Shigellosis, Including Epidemics Due to Shigella Dysenteriae Type 1. Geneva, Switzerland: WHO Document Production Services (2005).
3. Kotloff KL, Riddle MS, Platts-Mills JA, Pavlinac P, Zaidi AKM. Shigellosis. Lancet (2018) 391(10122):801–12. doi: 10.1016/S0140-6736(17)33296-8
4. Anderson M, Sansonetti PJ, Marteyn BS. Shigella Diversity and Changing Landscape: Insights for the Twenty-First Century. Front Cell Infect Microbiol (2016) 6:45. doi: 10.3389/fcimb.2016.00045
5. Sack DA, Lyke C, McLaughlin C, Suwanvanichkij V, World Health Organization. Antimicrobial Resistance in Shigellosis, Cholera and Campylobacteriosis. Geneva, Switzerland: World Health Organization (2001).
6. Tacconelli E, Magrini N, Kahlmeter G, Singh N. Global Priority List of Antibiotic-Resistant Bacteria to Guide Research, Discovery, and Development of New Antibiotics. Geneva, Switzerland: World Health Organization (2017).
7. Mani S, Wierzba T, Walker RI. Status of Vaccine Research and Development for Shigella. Vaccine (2016) 34(26):2887–94. doi: 10.1016/j.vaccine.2016.02.075
8. Muthuramalingam M, Whittier SK, Picking WL, Picking WD. The Shigella Type III Secretion System: An Overview From Top to Bottom. Microorganisms (2021) 9(2):451. doi: 10.3390/microorganisms9020451
9. Martinez-Becerra FJ, Scobey M, Harrison K, Choudhari SP, Quick AM, Joshi SB, et al. Parenteral Immunization With IpaB/IpaD Protects Mice Against Lethal Pulmonary Infection by Shigella. Vaccine (2013) 31(24):2667–72. doi: 10.1016/j.vaccine.2013.04.012
10. Martinez-Becerra FJ, Chen XT, Dickenson NE, Choudhari SP, Harrison K, Clements JD, et al. Characterization of a Novel Fusion Protein From IpaB and IpaD of Shigella Spp. And Its Potential as a Pan-Shigella Vaccine. Infect Immun (2013) 81(12):4470–7. doi: 10.1128/IAI.00859-13
11. Lewis DJ, Huo Z, Barnett S, Kromann I, Giemza R, Galiza E, et al. Transient Facial Nerve Paralysis (Bell’s Palsy) Following Intranasal Delivery of a Genetically Detoxified Mutant of Escherichia Coli Heat Labile Toxin. PloS One (2009) 4(9):e6999. doi: 10.1371/journal.pone.0006999
12. Clements JD, Norton EB. The Mucosal Vaccine Adjuvant LT(R192G/L211A) or dmLT. mSphere (2018) 3(4):e00215–18. doi: 10.1128/mSphere.00215-18
13. Lindenstrom T, Woodworth J, Dietrich J, Aagaard C, Andersen P, Agger EM. Vaccine-Induced Th17 Cells Are Maintained Long-Term Postvaccination as a Distinct and Phenotypically Stable Memory Subset. Infect Immun (2012) 80(10):3533–44. doi: 10.1128/IAI.00550-12
14. Sellge G, Magalhaes JG, Konradt C, Fritz JH, Salgado-Pabon W, Eberl G, et al. Th17 Cells are the Dominant T Cell Subtype Primed by Shigella Flexneri Mediating Protective Immunity. J Immunol (2010) 184(4):2076–85. doi: 10.4049/jimmunol.0900978
15. Valli E, Baudier RL, Harriett AJ, Norton EB. LTA1 and dmLT Enterotoxin-Based Proteins Activate Antigen-Presenting Cells Independent of PKA and Despite Distinct Cell Entry Mechanisms. PloS One (2020) 15(1):e0027047. doi: 10.1371/journal.pone.0227047
16. Mutsch M, Zhou WG, Rhodes P, Bopp M, Chen RT, Linder T, et al. Use of the Inactivated Intranasal Influenza Vaccine and the Risk of Bell’s Palsy in Switzerland. New Engl J Med (2004) 350(9):896–903. doi: 10.1056/NEJMoa030595
17. Valli E, Harriett AJ, Nowakowska MK, Baudier RL, Provosty WB, McSween Z, et al. LTA1 Is a Safe, Intranasal Enterotoxin-Based Adjuvant That Improves Vaccine Protection Against Influenza in Young, Old and B-Cell-Depleted (Mu MT) Mice. Sci Rep-Uk (2019) 9:15128. doi: 10.1038/s41598-019-51356-w
18. Norton EB, Lawson LB, Mahdi Z, Freytag LC, Clements JD. The A Subunit of Escherichia Coli Heat-Labile Enterotoxin Functions as a Mucosal Adjuvant and Promotes IgG2a, IgA, and Th17 Responses to Vaccine Antigens. Infect Immun (2012) 80(7):2426–35. doi: 10.1128/IAI.00181-12
19. Martinez-Becerra FJ, Kissmann JM, Diaz-McNair J, Choudhari SP, Quick AM, Mellado-Sanchez G, et al. Broadly Protective Shigella Vaccine Based on Type III Secretion Apparatus Proteins. Infect Immun (2012) 80(3):1222–31. doi: 10.1128/IAI.06174-11
20. van de Verg LL, Mallett CP, Collins HH, Larsen T, Hammack C, Hale TL. Antibody and Cytokine Responses in a Mouse Pulmonary Model of Shigella Flexneri Serotype 2a Infection. Infect Immun (1995) 63(5):1947–54. doi: 10.1128/iai.63.5.1947-1954.1995
21. WHO. DRAFT WHO Preferred Product Characteristics for Vaccines Against Shigella. Geneva, Switzerland: WHO (2020).
22. Ashkenazi S, Cohen D. An Update on Vaccines Against Shigella. Ther Adv Vaccines (2013) 1(3):113–23. doi: 10.1177/2051013613500428
23. Ferreccio C, Prado V, Ojeda A, Cayyazo M, Abrego P, Guers L, et al. Epidemiologic Patterns of Acute Diarrhea and Endemic Shigella Infections in Children in a Poor Periurban Setting in Santiago, Chile. Am J Epidemiol (1991) 134(6):614–27. doi: 10.1093/oxfordjournals.aje.a116134
24. Cohen D, Green MS, Block C, Slepon R, Ofek I. Prospective-Study of the Association Between Serum Antibodies to Lipopolysaccharide O-Antigen and the Attack Rate of Shigellosis. J Clin Microbiol (1991) 29(2):386–9. doi: 10.1128/jcm.29.2.386-389.1991
25. Corthesy B, Bioley G. Lipid-Based Particles: Versatile Delivery Systems for Mucosal Vaccination Against Infection. Front Immunol (2018) 9:431. doi: 10.3389/fimmu.2018.00431
26. Marasini N, Skwarczynski M, Toth I. Intranasal Delivery of Nanoparticle-Based Vaccines. Ther Delivery (2017) 8(3):151–67. doi: 10.4155/tde-2016-0068
27. Howlader DR, Das S, Lu T, Hu G, Varisco DJ, Dietz ZK, et al. Effect of Two Unique Nanoparticle Formulations on the Efficacy of a Broadly Protective Vaccine Against Pseudomonas Aeruginosa. Front Pharmacol (2021) 12:706157. doi: 10.3389/fphar.2021.706157
28. Hosangadi D, Smith PG, Giersing BK. Considerations for Using ETEC and Shigella Disease Burden Estimates to Guide Vaccine Development Strategy. Vaccine (2019) 37(50):7372–80. doi: 10.1016/j.vaccine.2017.09.083
29. Philpott DJ, Edgeworth JD, Sansonetti PJ. The Pathogenesis of Shigella Flexneri Infection: Lessons From In Vitro and In Vivo Studies. Philos Trans R Soc Lond B Biol Sci (2000) 355(1397):575–86. doi: 10.1142/9781848161610_0003
30. Levine MM, Kotloff KL, Barry EM, Pasetti MF, Sztein MB. Clinical Trials of Shigella Vaccines: Two Steps Forward and One Step Back on a Long, Hard Road. Nat Rev Microbiol (2007) 5(7):540–53. doi: 10.1038/nrmicro1662
31. Seo H, Duan Q, Zhang W. Vaccines Against Gastroenteritis, Current Progress and Challenges. Gut Microbes (2020) 11(6):1486–517. doi: 10.1080/19490976.2020.1770666
32. Su H, Lei CT, Zhang C. Interleukin-6 Signaling Pathway and Its Role in Kidney Disease: An Update. Front Immunol (2017) 8:405. doi: 10.3389/fimmu.2017.00405
33. Arakawa S, Suzukawa M, Watanabe K, Kobayashi K, Matsui H, Nagai H, et al. Secretory Immunoglobulin A Induces Human Lung Fibroblasts to Produce Inflammatory Cytokines and Undergo Activation. Clin Exp Immunol (2019) 195(3):287–301. doi: 10.1111/cei.13253
34. Pasetti MF, Simon JK, Sztein MB, Levine MM. Immunology of Gut Mucosal Vaccines. Immunol Rev (2011) 239(1):125–48. doi: 10.1111/j.1600-065X.2010.00970.x
35. Awasthi A, Kuchroo VK. Th17 Cells: From Precursors to Players in Inflammation and Infection. Int Immunol (2009) 21(5):489–98. doi: 10.1093/intimm/dxp021
36. Geddes K, Rubino SJ, Magalhaes JG, Streutker C, Le Bourhis L, Cho JH, et al. Identification of an Innate T Helper Type 17 Response to Intestinal Bacterial Pathogens. Nat Med (2011) 17(7):837–U202. doi: 10.1038/nm.2391
37. Li Y, Wei C, Xu H, Jia J, Wei Z, Guo R, et al. The Immunoregulation of Th17 in Host Against Intracellular Bacterial Infection. Mediators Inflammation (2018) 2018:6587296. doi: 10.1155/2018/6587296
38. Chakraborty S, Harro C, DeNearing B, Bream J, Bauers N, Dally L, et al. Evaluation of the Safety, Tolerability, and Immunogenicity of an Oral, Inactivated Whole-Cell Shigella Flexneri 2a Vaccine in Healthy Adult Subjects. Clin Vaccine Immunol (2016) 23(4):315–25. doi: 10.1128/CVI.00608-15
39. Pore D, Mahata N, Pal A, Chakrabarti MK. Outer Membrane Protein A (OmpA) of Shigella Flexneri 2a, Induces Protective Immune Response in a Mouse Model. PloS One (2011) 6(7):e22663. doi: 10.1371/journal.pone.0022663
40. Samandari T, Kotloff KL, Losonsky GA, Picking WD, Sansonetti PJ, Levine MM, et al. Production of IFN-Gamma and IL-10 to Shigella Invasins by Mononuclear Cells From Volunteers Orally Inoculated With a Shiga Toxin-Deleted Shigella Dysenteriae Type 1 Strain. J Immunol (2000) 164(4):2221–32. doi: 10.4049/jimmunol.164.4.2221
41. Way SS, Borczuk AC, Dominitz R, Goldberg MB. An Essential Role for Gamma Interferon in Innate Resistance to Shigella Flexneri Infection. Infect Immun (1998) 66(4):1342–8. doi: 10.1128/IAI.66.4.1342-1348.1998
42. Jehl SP, Nogueira CV, Zhang XQ, Starnbach MN. IFN Gamma Inhibits the Cytosolic Replication of Shigella Flexneri via the Cytoplasmic RNA Sensor RIG-I. PloS Pathog (2012) 8(8):e1002809. doi: 10.1371/journal.ppat.1002809
43. Rautajoki KJ, Kylaniemi MK, Raghav SK, Rao K, Lahesmaa R. An Insight Into Molecular Mechanisms of Human T Helper Cell Differentiation. Ann Med (2008) 40(5):322–35. doi: 10.1080/07853890802068582
Keywords: cross protection, IFN-γ, IL-17, vaccine, type III secretion system (T3SS), shigellosis
Citation: Lu T, Das S, Howlader DR, Zheng Q, Ratnakaram SSK, Whittier SK, Picking WD and Picking WL (2021) L-DBF Elicits Cross Protection Against Different Serotypes of Shigella spp. Front. Trop. Dis 2:729731. doi: 10.3389/fitd.2021.729731
Received: 23 June 2021; Accepted: 12 November 2021;
Published: 17 December 2021.
Edited by:
Sushant Sahastrabuddhe, International Vaccine Institute, South KoreaReviewed by:
Yun-Chi Chen, Morgan State University, United StatesCopyright © 2021 Lu, Das, Howlader, Zheng, Ratnakaram, Whittier, Picking and Picking. This is an open-access article distributed under the terms of the Creative Commons Attribution License (CC BY). The use, distribution or reproduction in other forums is permitted, provided the original author(s) and the copyright owner(s) are credited and that the original publication in this journal is cited, in accordance with accepted academic practice. No use, distribution or reproduction is permitted which does not comply with these terms.
*Correspondence: Wendy L. Picking, d2VuZHkucGlja2luZ0BrdS5lZHU=
†Present address: Qi Zheng, Deciphera Pharmaceuticals, LLC, Lawrence, KS, United States
Disclaimer: All claims expressed in this article are solely those of the authors and do not necessarily represent those of their affiliated organizations, or those of the publisher, the editors and the reviewers. Any product that may be evaluated in this article or claim that may be made by its manufacturer is not guaranteed or endorsed by the publisher.
Research integrity at Frontiers
Learn more about the work of our research integrity team to safeguard the quality of each article we publish.