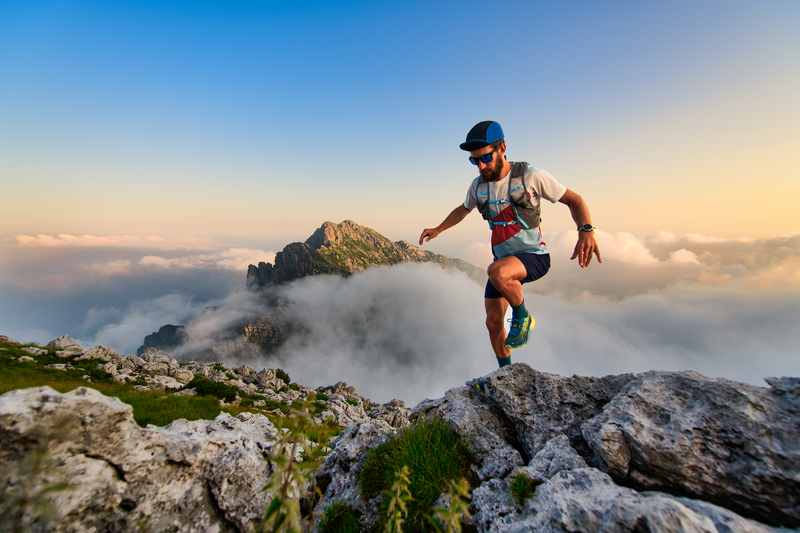
95% of researchers rate our articles as excellent or good
Learn more about the work of our research integrity team to safeguard the quality of each article we publish.
Find out more
REVIEW article
Front. Immunol. , 24 March 2025
Sec. Microbial Immunology
Volume 16 - 2025 | https://doi.org/10.3389/fimmu.2025.1550724
This article is part of the Research Topic Modulation of Pulmonary Immunity and Function by Bacterial and Host Metabolites View all 4 articles
A correction has been applied to this article in:
Corrigendum: Modulation of Pulmonary Immune Functions by the Pseudomonas aeruginosa Secondary Metabolite Pyocyanin
Pseudomonas aeruginosa is a prevalent opportunistic Gram-negative bacterial pathogen. One of its key virulence factors is pyocyanin, a redox-active phenazine secondary metabolite that plays a crucial role in the establishment and persistence of chronic infections. This review provides a synopsis of the mechanisms through which pyocyanin exacerbates pulmonary infections. Pyocyanin induces oxidative stress by generating reactive oxygen and nitrogen species which disrupt essential defense mechanisms in respiratory epithelium. Pyocyanin increases airway barrier permeability and facilitates bacterial invasion. Pyocyanin also impairs mucociliary clearance by damaging ciliary function, resulting in mucus accumulation and airway obstruction. Furthermore, it modulates immune responses by promoting the production of pro-inflammatory cytokines, accelerating neutrophil apoptosis, and inducing excessive neutrophil extracellular trap formation, which exacerbates lung tissue damage. Additionally, pyocyanin disrupts macrophage phagocytic function, hindering the clearance of apoptotic cells and perpetuating inflammation. It also triggers mucus hypersecretion by inactivating the transcription factor FOXA2 and enhancing the IL-4/IL-13-STAT6 and EGFR-AKT/ERK1/2 signaling pathways, leading to goblet cell metaplasia and increased mucin production. Insights into the role of pyocyanin in P. aeruginosa infections may reveal potential therapeutic strategies to alleviate the severity of infections in chronic respiratory diseases including cystic fibrosis (CF) and chronic obstructive pulmonary disease (COPD).
Pseudomonas aeruginosa is a ubiquitous Gram-negative bacterium in the environment and a major pathogen in individuals with chronic pulmonary diseases and immunocompromised health conditions. Pulmonary infections are a significant global health concern, especially in immunocompromised individuals and patients with preexisting respiratory diseases such as cystic fibrosis (CF), chronic obstructive pulmonary disease (COPD), primary ciliary dyskinesia, and chronic bronchitis (1), leading to high morbidity and mortality rates. P. aeruginosa is especially prevalent in CF patients, with infection rates rising from 20% in infants to nearly 80-90% in adulthood (2). Prior to the highly effective modulator therapy (HEMT), CF patients faced a worse prognosis due to chronic colonization and infection, particularly with P. aeruginosa, which is associated with increased mortality and poorer long-term survival outcomes (3, 4). HEMT (e.g., Trikafta) has significantly improved lung function in CF patients by restoring airway mucus homeostasis (5, 6). However, its long-term impact on the population dynamics of P. aeruginosa and other CF pathogens remains unclear. The economic impact of P. aeruginosa infections is substantial, with patients who developed nosocomial infections experienced a 66% increase in healthcare costs compared to those infected by other bacterial pathogens (7), which greatly impact the healthcare system. The significant economic burden of P. aeruginosa infections is further exacerbated by the emergence of multidrug-resistant (MDR) strains, with hospitalization costs for MDR cases being three times higher than those with non-resistant infections (8). P. aeruginosa infections often lead to further complications, including pneumonia and infections at multiple body sites. Some key factors that drive P. aeruginosa as a major pathogen include its ability to express a plethora of quorum sensing (QS)-regulated virulence factors, multiple drug efflux pumps, as well as its ability to form biofilms, which significantly enhance its resistance to antimicrobials, making infections more challenging to eradicate (9–11). QS allows bacteria to sense population density through the release of autoinducers, triggering the expression of virulence genes. In P. aeruginosa, the QS system consists of the LasI-LasR and RhlI-RhlR circuits, which utilize acylated homoserine lactones (Acyl-HSL) such as C4-HSL and 3Oc12-HSL to regulate gene expression. The PqsR (also known as MvfR), a LysR-type transcriptional regulator, is responsive to the Pseudomonas quinolone system (PQS) that integrates into this network by modulating quinolone synthesis and interacts with the Las and Rhl systems to regulate the secretion of virulence factors (12–17). QS-regulated P. aeruginosa virulence factors include, among others, phenazines (18), Exotoxin A (19), proteases (20), and phospholipases (21).
One of the most well-studied virulence factors is the phenazines pyocyanin, a bluish pigment secondary metabolite that enhances the ability of P. aeruginosa to promote chronic lung infection (22). The biosynthesis of pyocyanin begins with the conversion of precursor chorismic acid, an intermediate metabolite that is utilized by microorganisms, through both phzA1B1C1D1E1F1G1 (phz1) and phzA2B2C2D2E2F2G2 (phz2) operons, and the phzM and phzS genes. These operons are regulated by the P. aeruginosa QS molecule quinolone. At high cell density, quinolones are secreted and detected by PqsR, which triggers the transcription of the phz operons, where the chorismic acid is modified into a tricyclic compound, and eventually, pyocyanin (Figure 1) (22–24). Pyocyanin is a zwitterion that is capable of diffusing through cell membranes freely. Previous studies have found pyocyanin in millimolar concentrations in the sputum of individuals infected with P. aeruginosa (25), with 16.5 μg/ml and 27 μg/ml recovered from bronchiectasis patients and CF patients, respectively (26, 27). Although 27 µg/ml is the highest amount of pyocyanin retrieved from human sputum, some studies have employed pyocyanin concentrations exceeding 100 µg/ml on human lung bronchial epithelial cells, raising concerns about the clinical relevance (28).
Figure 1. P. aeruginosa quorum sensing network and pyocyanin biosynthesis. Pyocyanin biosynthesis is regulated by the quorum sensing (QS) molecule quinolones and the QS regulatory system LasR-LasI, RhlR-RhlI and PqsR (MvfR). At the threshold population bacterial density, phz1 and phz2 operons are activated. This leads to the production of phenazine biosynthetic enzymes that convert chorismic acid into pyocyanin. Figure generated with BioRender.com.
Pyocyanin is a redox-active tricyclic molecule structurally characterized by its nitrogen-containing heterocyclic core (29, 30). The redox cycling capabilities in pyocyanin enable it to become a potent inducer of oxidative stress that can reduce molecular oxygen quickly and generate reactive oxygen species (ROS) (31), primarily superoxide (O2•-) and hydrogen peroxide (H2O2); and reactive nitrogen species peroxynitrite (ONOO-). This effect significantly contributes to pathogenic outcomes, such as DNA damage (32), and disruption of membrane potential and redox balance in the host (33). In addition to damaging cellular structures, pyocyanin also activates several signaling pathways that promote inflammation, creating a vicious cycle of oxidative stress and immune dysregulation. Interestingly, P. aeruginosa has evolved multiple mechanisms to withstand the ROS effects generated by pyocyanin. The bacterium expresses various anti-oxidative enzymes, including superoxide dismutases (SOD), catalases, and peroxidases, that play a vital role in detoxifying the ROS produced by pyocyanin (34–37). These enzymes detoxify ROS into less harmful substances, such as water and oxygen. For instance, SOD catalyze the conversion of superoxide radicals into hydrogen peroxide, which is subsequently broken down into water and oxygen by catalases.
Research on pyocyanin has explored its virulence mechanism and interactions across various in vivo and in vitro models. In vivo model included diverse organisms such as the nematode Caenorhabditis elegans (18), the Arabidopsis thaliana leaf infiltration model (38), Galleria mellonella larvae (39) and brine shrimp Artemia salina (40). Additional more complex mammalian models include mouse strains such as C57BL/6J, BALB/c, and FVBN (41–43), and studies examining immunomodulatory effect of pyocyanin in sheep airways (44). In vitro studies using human cell lines have been extensively used to study pyocyanin, including NCI-H292 pulmonary carcinoma cells, 16HBE and NHBE (human bronchial epithelial cells), and A549 lung carcinoma epithelial cells (45, 46). Furthermore, pyocyanin exhibited cytotoxic effects against cancer cell lines such as SK-MEL-30 human melanoma and HT-29 human colon cancer cells, suggesting its potential as a cancer treatment (45). Unlike traditional 2D models, which lack the complexity of tissue architecture, 3D models such as the air-liquid interface (ALI) model of airway epithelial cells or lung organoids may provide a more realistic environment for assessing molecular modulation of pulmonary functions by pyocyanin and potential therapeutic effect in lung diseases by better replicating human lung tissue architecture and cellular interactions (47, 48). While in vitro studies provide controlled insights into cellular mechanisms and therapeutic effects, in vivo animal models with an intact immune system offer a comprehensive understanding of the biological impact of pyocyanin. Given the critical role of pyocyanin in P. aeruginosa pathogenesis, it is essential to understand the mechanisms by which this virulence factor disrupts pulmonary function and modulates the host immune response. In this article, we provide a critical synopsis on modulation of pulmonary immune response by pyocyanin.
Pyocyanin has a planar aromatic structure with conjugated double bonds that allow the displacement of electrons and facilitates in redox reactions. The phenazine rings can exist in different oxidation states, allowing pyocyanin to accept or donate electrons and cycling between reduced and oxidized states (29). This redox cycling process generates ROS, the primary mechanism underlying the toxicity of pyocyanin. Pyocyanin acts as a potent catalyst in cellular redox reactions, accepting electrons from NADH and NADPH and subsequently transferring them to molecular oxygen (O2). This process results in the formation of superoxide anions (O2-). These superoxide anions are then converted into hydrogen peroxide (H2O2) by superoxide dismutase (25, 49–52). Hydrogen peroxide can further undergo Fenton reactions, leading to the production of hydroxyl radicals (OH•), which are among the most damaging forms of ROS (53). In addition, O2- can interact with NO to produce reactive nitrogen species (RNS), such as the highly toxic peroxynitrite (Figure 2A) (54). Collectively, these ROS and RNS generated by pyocyanin contribute significantly to several detrimental cellular processes. These harmful effects include lipid peroxidation, protein modification, mitochondrial and DNA damage, and ciliary dysfunction. Ultimately, the accumulation of these damaging processes results in overall cellular dysfunction and apoptosis (46, 55–58) (Figure 2B). The disruption of redox balance also inhibits crucial functions such as the dual oxidase-based antimicrobial system (DUOX) and ATP synthesis (45, 59). This interference facilitates bacterial persistence and contributes to chronic lung inflammation in individuals with CF and COPD (60).
Figure 2. (A) Proposed mechanism of the pyocyanin (PCN) redox cycling. PCN can be reduced by NAD(P)H to form PCNH2. This reduced form can then be oxidized back to PCN by oxygen, generating an oxygen radical as a byproduct which is subsequently converted into hydrogen peroxide (H2O2). (B) Molecular basis of pyocyanin toxicity. Pyocyanin undergoes redox cycling by oxidizing NADPH to NADP+, generating reactive oxygen species (ROS) and reactive nitrogen species (RNS), contributing to the pathogenicity of the bacteria. Figures generated with BioRender.com.
The pulmonary epithelium serves as the first line of defense against inhaled pathogens and harmful particles, acting as a physical barrier, and an integral part of the innate immune system (61–63). However, the accumulation of ROS induced by the pyocyanin can significantly damage alveolar epithelial cells and subsequently disrupt the respiratory epithelial barrier function. This compromises the integrity of the lungs, making them more susceptible to infection and inflammation (64). In this section, we will explore the effects that pyocyanin-derived ROS exerted on the components of the airway immune system.
One critical consequence of pyocyanin-induced ROS production is the disruption of ciliary function in respiratory epithelial cells (65). The mucociliary clearance serves as the primary innate defense in clearing inhaled particles, pollutants, pathogens, and debris from the respiratory tract through coordinated beating (66, 67). Pyocyanin disrupts ciliary function by decreasing intracellular ATP levels, a crucial energy source for ciliary beating. This reduction in ATP level occurs as pyocyanin undergoes intracellular redox cycling, generating superoxide by depleting NADH. The release of ROS, which damages mitochondrial function and leads to ATP depletion, impairs the energy supply needed for proper ciliary movement (68–70). Dyneins are a family of cytoskeletal motor proteins that drive ciliary beating by converting the chemical energy stored in ATP into mechanical work with the help of dynein ATPases. Inadequate ATP supplies compromise the effectiveness of dynein-dependent ciliary motility (71, 72). This mechanism directly links the oxidative stress effects of pyocyanin to the impairment of ciliary function, contributing to defective mucociliary clearance and the persistence of respiratory infections in diseased lungs, such as CF and advanced stages of COPD (73). Furthermore, hydrogen peroxide has been shown to activate protein kinase C (PKC), which in turn leads to a reduction in the airway ciliary beat frequency. Research has also highlighted the phosphorylation of a key membrane-associated polypeptide, p37, by PKC, with phosphorylation occurring on both serine and tyrosine residues, decreasing ciliary beat frequency (74, 75). Additional studies have identified the biochemical pathways through which PKC regulates ciliary function, particularly in response to oxidative stress, aging, and alcohol consumption, all of which contribute to slowing ciliary beat frequency (76–78). These findings emphasize the significant role of PKC activation in mediating reduced ciliary activity in response to oxidative stress. The impairment of mucociliary function caused by pyocyanin-induced ROS leads to the accumulation of mucus, creating a niche for chronic microbial infection and vicious cycles of inflammation (79), particularly relevant in chronic respiratory diseases such as CF and COPD, where P. aeruginosa pyocyanin is expressed in abundance (79). The importance of cellular redox balance on ciliary function is further illustrated in the case of respiratory syncytial virus (RSV) infections, which the imbalanced ROS production in the airway epithelial microenvironment not only leads to oxidative damage to cilia but also disrupt the function of several redox-sensitive proteins such as protein kinase A (PKA), PKC, and protein phosphatase 1, which play crucial roles in regulating ciliary motility, eventually exacerbating airway mucociliary dysfunction (65, 80–83).
The epithelial layer forms a crucial physical barrier in the intestinal, respiratory, and skin tissues, which play a pivotal role in maintaining tissue homeostasis, immune response, and protecting the body from pathogens. Disruption of the barrier function by oxidative stress and ROS, including those generated by pyocyanin, impairs mucosal immunity (84, 85). This is partly due to the impact on the actin cytoskeletal network in epithelial cells, which is crucial for maintaining cell shape, adhesion, and motility. However, ROS alters actin polymerization and stability, which disrupts epithelial cell barrier functions. One example is the ROS-induced modifications at Cys-374 of β-actin create disulfide bonds between actin monomers and prevent actin filament formation (86, 87). As a result, the ability of actin filaments to form a cytoskeleton is compromised, which causes cells to be unable to maintain their shape and integrity, contributing to “leaky” epithelial barriers that allow pathogens to invade host tissues. Furthermore, epithelial cells are held together by tight junctions (TJs) and adherens junctions (AJs), both essential for maintaining epithelial barrier integrity. ROS can disrupt these junctions, resulting in a compromised mucosal immune barrier. TJs are composed of claudins, occludins, and zonula occludens-1 (ZO-1) proteins, preventing paracellular leakage between epithelial cells (88). Under oxidative stress, ROS modifies occludins and claudins and causes the formation of disulfide bonds, impairing their ability in forming tight junctions and weakening the barrier (89). Besides TJs, ROS can also alter the integrity of AJs that mediate cell-cell adhesion and contact. AJs are characterized by cadherin cell adhesion transmembrane receptors that bind to each other in the intercellular space, establishing a physical connection between cells. Under physiological conditions, cadherins associate with catenins, which bind to cytoskeletal components such as actin filaments and microtubules, providing mechanical support to the cell (90, 91). In the case of AJs, ROS activate Src kinase, which phosphorylates p120-catenin. Phosphorylated p120-catenin dissociates from N-cadherin, causing internalization of N-cadherin from the membrane (87, 92). This leads to the disruption of adherens junctions, further impairing cell-cell adhesion and compromising the epithelial barrier, rendering host tissue susceptible to pathogen invasion.
Under oxidative stress, ROS can modify immune-related proteins, affecting their signaling and response to infections. For example, exposure to pyocyanin mediated ROS to human airway epithelial cell activate NF-κB and other inflammatory signaling pathways, leading to the production of pro-inflammatory cytokines including tumor necrosis factor-alpha (TNF-α) and interleukins (IL-1β, IL-6, IL-8) (93). These cytokines promote inflammation and disrupt the mucosal barrier, leading to increased epithelial permeability and immune cell infiltration. Research using cell culture models such as Calu-3 airway epithelial cells, has demonstrated that these cytokines affect the integrity and permeability of pulmonary epithelial barrier integrity by regulating key TJs proteins like occludin, claudin-2, and ZO-1. These processes are driven by the activation of the epidermal growth factor receptor (EGFR) and the ERK1/2 MAPK signaling pathway. This signaling not only increases the permeability between cells but also contributes to the breakdown of tight junctions, weakening the barrier in the airways (94–97). Inhibitors targeting EGFR or the ERK1/2 MAPK pathway were shown to attenuate cytokine-induced degradation of tight junction proteins (31). The disruption of tight junctions by pyocyanin not only weakens the epithelial barrier but also promotes P. aeruginosa invasion, where it exacerbates chronic lung infection in CF and COPD.
Neutrophils are among the first immune cell population responding to microbial invasion. Upon detection, neutrophils move rapidly to the infection site to phagocytize and destroy these pathogens. Pyocyanin is strongly neutrophilic. Mechanistically, ROS generated by pyocyanin triggers the release of pro-inflammatory cytokines such as the neutrophil chemotactic factor IL-8 in a NF-κB-dependent manner through the MAPK/ERK signaling (98, 99), further contributing to the pathological effects observed during P. aeruginosa infections (99–102), which will be further discussed in Section 2.3. This rapid and excessive influx of neutrophils leads to persistent inflammation and damage to the infected tissue by releasing ROS, proteinase (such as elastase and cathepsin G), myeloperoxidase, and metalloproteinases (103, 104). Anti-inflammatory drugs like glucocorticoids have been shown to limit the pro-inflammatory effects of neutrophils by downregulating key inflammatory factors, including IL-1β, TNF-α, and IL-8, thus diminishing the neutrophil mediated proinflammatory damage caused by neutrophilic stimuli such as pyocyanin (105, 106).
In addition to being neutrophilic, pyocyanin has been shown to accelerate neutrophil apoptosis (107–110). Studies have shown that pyocyanin generates excessive mitochondrial ROS in neutrophils (107), damaging mitochondrial function and contributing to neutrophil apoptosis. The premature death of neutrophils (10-fold increase in apoptosis within five hours post-exposure to pyocyanin) attenuates their antimicrobial activity and contributes to the persistence of P. aeruginosa in the lungs (109). The mitochondrial acid sphingomyelinase (ASM) is the key mediator in this process. ASM hydrolyzes sphingomyelin into ceramide, the latter plays a role in activating the apoptotic cascade (111, 112). Ceramide, together with the mitochondrial cytochrome c, triggering apoptosis in neutrophils. Neutrophils lacking ASM are resistant to pyocyanin-induced cell death, suggesting that ASM is crucial for mediating this process (107). Neutrophil apoptosis severely impairs the ability of the body to clear bacterial infection and likely contributes to the P. aeruginosa evasion of host immune defenses.
Neutrophils employ defense mechanism against pathogens through the formation of neutrophil extracellular traps (NETs). These NETs are intricate networks of chromatin fibers decorated with antimicrobial proteins (e.g., neutrophil elastase, cathepsin G, and histones that have a high affinity for DNA) released by neutrophils during a programmed cell death called NETosis (113, 114). NETs play a crucial role in the immune response by entrapping and killing a wide range of pathogens, including bacteria, fungi, viruses, and parasites (115, 116). NETs formation involves the decondensation of nuclear chromatin through histone citrullination by the peptidyl arginine deiminase IV (PAD4) (117–119). This process leads to the release of chromatin through a ruptured nuclear envelope, where it combines with cytosolic antimicrobial proteins and extracellular DNA (116, 120). Together, these components form the extracellular structures, which trap and neutralize pathogens. NETs released during NETosis are particularly important in trapping and killing bacterial pathogens such as P. aeruginosa and Staphylococcus aureus in pneumonia and chronic lung diseases like CF and COPD (121, 122). Pyocyanin has been identified as the first secreted bacterial toxin that enhances NET formation by stimulating an increase in the production of ROS within neutrophils (123). While NET plays a crucial role in combating microbial invaders, excessive NET formation can have adverse negative effects, where prolonged NET presence contributes to tissue damage and persistent inflammation. Due to the release of DNA and various enzymes that damage host tissues, NETs are highly pro-inflammatory. Persistent NETosis leads to the release of large amounts of ROS and pro-inflammatory molecules, creating a feedback loop of chronic inflammation (124). The overproduction of NETs in response to P. aeruginosa colonization can exacerbate neutrophilic inflammation (125), increasing airway obstruction and causing mucociliary dysfunction (126). In diseases such as CF and advanced stages of COPD where P. aeruginosa is a prominent pathogen, pyocyanin-induced NETosis is predicted to exacerbate the disease progression. The excessive release of NETs contributes to the formation of thick, viscous mucus, a hallmark of the disease (124, 126). Over time, the ongoing NETosis and chronic inflammation lead to irreversible lung damage and a decline in pulmonary function.
Macrophages are crucial immune myeloid cells involved in both innate and adaptive immune responses (127–129). They originate from monocytes and are found throughout the body, acting as one of the first responders to infections (130). Macrophages phagocytize microbial pathogens, enclosing them in a phagosome that fuses with lysosomes, where microbes are destroyed by digestive enzymes and ROS (131, 132). In vitro, studies have shown that pyocyanin selectively disrupts the phagocytic function rather than overall macrophage impairment and dysfunction (133). Specifically, pyocyanin incapacitates macrophages to phagocytize apoptotic cells while maintaining their capacity to ingest inert particles such as latex beads. This impairment results in late apoptotic and necrotic cell accumulation in mouse tissues infected with pyocyanin-producing P. aeruginosa. In contrast, tissue infected with pyocyanin-deficient strains does not exhibit this accumulation (108). The failure of macrophages to clear apoptotic cells contributes to prolonged inflammation, as these unengulfed cells can undergo secondary necrosis, releasing inflammatory mediators that exacerbate tissue damage and promote a new cycle of inflammation (134, 135). The underlying mechanism of this impaired engulfment is linked to the disruption of the Rho GTPase signaling, which is crucial for phagocytosis by pyocyanin-generated ROS (108, 136). This disruption in the phagocytic clearance of apoptotic cells will likely cause chronic inflammation and weakened immune responses, potentially worsening tissue damage during infections.
Natural killer (NK) cells are lymphocytes in the innate immune system that play a critical role in defending the body against infections and malignancies. Unlike T and B cells, NK cells do not require prior exposure to a pathogen to recognize and kill infected or abnormal cells (137, 138). They possess the ability to detect stressed, infected, or cancerous cells through a balance of activating and inhibitory receptors (139). NK cells respond rapidly to infections by releasing cytotoxic molecules like perforin and granzymes, which induce apoptosis and necrotic cell death in target cells. In addition, NK cells produce cytokines such as interferon-gamma (IFN-γ), which helps activate other immune cells (140). While NK cells are traditionally associated with viral infections and tumor surveillance, they also play an important role in bacterial infections (137, 141). NK cells can directly kill bacteria-infected cells and secrete cytokines that enhance the response of macrophages and other immune cells. A key example of this is the production of IFN-γ by NK cells, which activates macrophages to enhance bacterial killing via phagocytosis and ROS production (142, 143). Pyocyanin was shown to induce apoptosis in the human NK cell line, NK92. Interestingly, studies have shown that pyocyanin-producing P. aeruginosa are more effective at inducing apoptosis in NK92 cells compared to the pyocyanin-deficient strain (144). This apoptosis is primarily mediated through mitochondrial damage, although interesting, ROS generated by pyocyanin do not appear to play a significant role in this process (145). The excessive NK cell apoptosis induced by pyocyanin may have serious implications for immune function, potentially leading to reduced immune surveillance and responsiveness. Consequently, this could increase the susceptibility to infections and highlight the importance of pyocyanin as a virulence factor in P. aeruginosa pathogenicity.
Pyocyanin-mediated oxidative stress has been shown to trigger the expression of pro-inflammatory cytokines through the activation of various host signaling pathways, among others, NF-κB and MAPK (93, 146–148). Under normal conditions, the production of cytokines such as IL-6, TNF-α, IL-1β, and IL-8 is crucial in the recruitment of immune cells, such as neutrophils and macrophages, to the site of infection and eliminating pathogens (98, 149–153). However, the overproduction or dysregulation of these cytokines induced by pyocyanin can lead to persistent inflammation, contributing to chronic inflammatory diseases such as CF and advanced stages of COPD. To better understand the consequences of pyocyanin-mediated inflammation, we highlighted the differential effects of this toxin on acute versus chronic inflammation. In acute inflammation, cytokine production is typically a short-term response aimed at combating infection and facilitating healing (154). However, when there is an excessive or sustained cytokine response, the inflammatory process becomes dysregulated and transition into chronic inflammation (155). This transition is a hallmark of chronic lung diseases such as CF and advanced stages of COPD, where prolonged P. aeruginosa colonization leads to a cycle of continuous immune activation and tissue damage.
Microarray analysis of airway epithelial cells exposed to pyocyanin has revealed several genes involved in inflammatory processes that are upregulated, including IL-6, TNF-α, IL-1β, IL-8, granulocyte colony-stimulating factor (G-CSF), granulocyte-macrophage colony-stimulating factor (GM-CSF) and CXCL1 (93). These cytokines and growth factors have significant roles in both innate and adaptive immunity. G-CSF and GM-CSF are critical for the activation and proliferation of granulocytes and macrophages, which are essential for the initial defense against infection. CXCL1 is a potent neutrophilic chemokine. As mentioned above, however, when produced in excess, these proinflammatory mediators exacerbate tissue damage, further perpetuating the proinflammatory cycles (156–158).
Acute inflammation is typically short-lived and resolved once the pathogen is eliminated. However, in the presence of pyocyanin, this initial acute response can be disrupted and transitioned to chronic inflammation. Pyocyanin induces the secretion of IL-23 to promote the differentiation and activation of Th17 cells, a subset of T-helper cells that play a key role in sustaining chronic inflammatory states (93, 159). During chronic inflammation, the immune system fails to eliminate infecting pathogens and leading to persistent inflammation. This phenomenon is particularly pronounced in diseases like CF and advanced stages of COPD, where chronic airway infection by P. aeruginosa, results in a vicious cycle of immune activation, tissue damage, and disease progression (160, 161). The impact of pyocyanin on both acute and chronic inflammation emphasizes the need for targeted therapeutic strategies that can modulate the inflammatory response and mitigate the harmful effects of pyocyanin on host tissues.
The cystic fibrosis transmembrane conductance regulator (CFTR) is an anion channel that allows ion flux, such as chloride (Cl-), at the apical side of airway epithelia (162–164). Increased chloride secretion is immediately followed by water movement. This process helps maintain the volume of airway surface liquid (ASL) and ensures the proper hydration of mucus. Proper Cl- transport ensures that the mucus layer covering the airways remains adequately hydrated, allowing optimal mucociliary clearance of bacteria and foreign particles (165–167). However, defective CFTR leads to dehydrated and thickened mucus, a hallmark of CF (162). Mucociliary clearance is a critical component of the innate defense system in the airway, protecting the respiratory tract by trapping inhaled pathogens, allergens, and particulate matter, which are removed through the mucociliary machinery (79, 168–170). However, mucus secretion is often dysfunctional, and mucociliary transport machinery is disabled in many chronic respiratory diseases (170–172). Goblet cell hyperplasia, metaplasia, and excessive mucus production are key pathological features of chronic airway diseases such as chronic obstructive bronchitis (part of COPD) and CF. Chronic respiratory infections often worsen these conditions, particularly with P. aeruginosa, which leads to persistent inflammation and increased mucus hypersecretion (173). Studies suggest that Cl- transport by CFTR is affected by pyocyanin-generated ROS production, which leads to glutathione and ATP depletion. Consequently, CFTR function is inhibited, impairing chloride transport in airway epithelia (174). Furthermore, pyocyanin has been found to disrupt CFTR Cl- transport by inhibiting the endocytic recycling of CFTR and inactivating the airway epithelial vacuolar ATPase, which reduces the expression and trafficking of CFTR, hence disrupting Cl- transport (175, 176). Excessive mucus clogs the airways, creating a favorable niche for microbial colonization and infection. Disruption of ciliary function by pyocyanin (see Section 2.1.1) further deteriorates mucociliary clearance of thickened mucus, leading to microbial-mediated acute exacerbation often seen in CF and COPD (177, 178).
Multiple studies conducted by our laboratory have demonstrated that chronic exposure to pyocyanin contributes to goblet cell metaplasia and hyperplasia, mucus hypersecretion (26, 173, 179–182), as well as emphysema (41). Pyocyanin inhibits the expression of Forkhead box protein A2 (FOXA2), a key transcription factor that regulates lung alveolarization and airway mucus homeostasis (183). In healthy airways, FOXA2 negatively regulates goblet cell development, maintaining a balance between the need for mucus production and the prevention of excessive secretion (183, 184). Pyocyanin inactivates FOXA2 via the activation of EGFR-AKT/ERK1/2 pathways and the IL-4/IL-13-STAT6-SAM-pointed domain–containing Ets-like factor (SPDEF) pathways (26, 179, 180, 185, 186) (Figure 3). Activated EGFR-AKT/ERK1/2 and IL-4/IL-13-STAT6-SPDEF convergently suppress FOXA2, in which the stability and transcriptional activity of FOXA2 are regulated through various posttranslational modifications. Phosphorylation of FOXA2 by AKT sequesters the modified protein from nuclei to cytoplasm, where it is ubiquitinated and degraded (26, 187–189), increasing the expression of airway mucin genes MUC5AC and MUC5B (180, 190, 191). The balance between the airway gel-forming mucin MUC5AC and MUC5B is a key factor in both COPD and CF, influencing disease progression and severity. In COPD patients, increased MUC5AC concentrations are more reliably associated with disease manifestations compared to MUC5B. Conversely, in CF patients, both MUC5AC and MUC5B are elevated, with a 30-fold increase for MUC5AC and an 8-fold increase for MUC5B, leading to a significantly higher MUC5AC/MUC5B ratio compared to healthy individuals. These distinct mucin profiles highlight the potential of MUC5AC and MUC5B, and especially their ratio, as biomarkers and therapeutic targets for managing these chronic respiratory diseases (192, 193). Additionally, ROS/RNS generated by pyocyanin modifies FOXA2 post-translationally, further contributing to mucus overproduction (26). These combined dysregulations lead to unchecked goblet cell proliferation, excessive mucus secretion, and pathological mucus accumulation observed in chronically diseased lungs infected by pyocyanin-producing P. aeruginosa.
Figure 3. Induction of the signaling pathways by pyocyanin that drives mucus hypersecretion in diseased airways. IL-4R/IL-13R and EGFR activation by pyocyanin trigger JAK-STAT6-SPDEF, MAPK, and AKT signaling pathways, respectively. These pathways convergently suppress the expression of FOXA2, a transcription factor that regulates airway mucus homeostasis. Posttranslational phosphorylation sequesters phosphorylated FOXA2 to the cytoplasm destined for ubiquitination and degradation. The loss of FOXA2 results in the increased expression of the MUC5AC and MUC5B mucin genes, leading to goblet cell metaplasia, hyperplasia, and mucin overproduction. Figure generated with BioRender.com.
The impact of pyocyanin on mucus hypersecretion is further intensified by its influence on the immune system, particularly by inducing Th2 cytokines secretion, such as IL-4 and IL-13 (41, 93). The IL-4/IL-13-STAT6-SPDEF pathway drives the Th2 response and goblet cell differentiation (93, 194). In a mouse chronic exposure model, pyocyanin polarizes an initially Th1 immune response towards the IL-4 and IL-13-dominated Th2 response, activating the IL-4/IL-13 receptor-STAT6-SPDEF signaling pathway (186, 195). SPDEF is responsible for triggering goblet cell metaplasia where airway epithelial multipotent basal, club, and ciliated cells are differentiated into mucus-secreting goblet cells and trigger a surge in mucus production (196, 197). Also, chronic oxidative stress causes a buildup of misfolded proteins in the endoplasmic reticulum (ER), triggering ER stress and activating the unfolded protein response (UPR) that intensifies mucus production and contributes to the development of chronic inflammation (198–200).
In CF and COPD where P. aeruginosa is a common and persistent colonizer, the consequences of pyocyanin-induced mucus hypersecretion are particularly severe (173). The resulting thick, sticky mucus not only obstructs airways but also creates an optimal environment for bacterial proliferation (201, 202), which in turn perpetuates inflammation and further increases mucus production. Consequently, this cascade promotes persistent infections and worsening lung function over time (203), which are hallmarks of diseases like CF and COPD.
Antibiotics are most commonly prescribed for bacterial infections. Poor antimicrobial stewardship has led to the rise of antibiotic resistance (204, 205). Various preclinical strategies have been experimented to disrupt the non-essential aspect of bacterial life cycle, e.g., targeting non-growth bacterial virulence, reducing the risk of resistance development and preserving the beneficial microbiota, making it a sustainable option for long-term infection management (Table 1). One approach involves antioxidant therapies aimed at neutralizing the ROS generated by pyocyanin or boosting endogenous antioxidant defenses such as glutathione (206). N-acetylcysteine (NAC), a well-known antioxidant, has been studied for its ability to protect cells from pyocyanin-induced oxidative damage (207–209). Another strategy focuses on inhibiting pyocyanin production itself. Raloxifene, an oestrogen receptor modulator, has been shown to target PhzB2 in the phenazine biosynthetic pathway, is capable to reduce the virulence of P. aeruginosa (210). As discussed in Section 2.3, pyocyanin disrupts FOXA2 expression, a regulator of mucus homeostasis. The incretin mimetic Exendin-4 has been shown to restore FOXA2 expression by activating the GLP1R-PKA-PPAR-γ-dependent phosphatases PTEN and PTP1B, which dephosphorylate key kinases in pro-goblet cell EGFR-AKT/ERK1/2 and IL-4/IL-13-STAT6-SPDEF signaling pathways, lowering mucin production, and reduce P. aeruginosa burden in mouse models, offering a promising therapeutic strategy for managing mucus in these conditions (173). Other studies have revealed that N-Aryl Malonamides (NAMs) are potent inhibitors of the QS transcriptional regulator, MvfR (PqsR). In a murine model, NAMs were shown to protect intestinal barrier function, prevent bacterial dissemination, and reduce inflammatory cytokines. Inhibition of MvfR by NAMs reduces the production of multiple secondary metabolites, including pyocyanin, demonstrating the potential efficacy of this class of drugs in reducing P. aeruginosa pathogenicity (211). Meta-bromo-thiolactone (mBTL), an analog of the P. aeruginosa QS auto-inducers 3OC12–HSL (which activates LasR) and C4–HSL (which activates RhlR), inhibits pyocyanin production, biofilm formation, and protects the immortalized human alveolar-type II-like A549 cells and the invertebrate host C. elegans from P. aeruginosa infection (212, 213). Paraoxonase (PON) is a mammalian enzyme that breaks down lactones. It has been demonstrated that the expression of PON2 with intact lactonase activity facilitates 3OC12-HSL degradation in human airway epithelial cells and murine tracheal epithelial cells, suggesting that PON can disrupt the P. aeruginosa QS system by degrading the QS signaling molecule 3OC12-HSL (214, 215). Separately, research has focused on identifying the inactivation of a P. aeruginosa quorum-sensing signal by human airway epithelia. Quorum quenching (QQ) enzymes may potentially prevent pyocyanin synthesis by disrupting the bacterial QS system that regulates its production. These enzymes, such as AHL lactonases and acylases, degrade the signaling molecules responsible for QS, inhibiting the coordinated expression of pyocyanin and other virulence factors. By reducing pyocyanin levels, QQ enzymes may mitigate the oxidative damage and tissue toxicity caused by pyocyanin (216, 217). Additionally, immunomodulation strategies are being explored to restore the ability of the immune system to fight infections. These include protecting neutrophil, macrophage, and T-cell functions from pyocyanin-mediated toxicity could boost P. aeruginosa clearance (218, 219). By combining antioxidant treatments, pyocyanin biosynthesis inhibition, and immunomodulation, these strategies may restore immune function, mitigate oxidative stress, and ultimately reduce the lung damage associated with chronic infections.
Pyocyanin plays a crucial role in chronic lung infections by P. aeruginosa (25, 220). Pyocyanin contributes to persistent infections and chronic inflammation by inducing oxidative stress and modulating the host airway epithelial physiology and immune response. Its ability to generate ROS leads to cellular damage, further exacerbating inflammatory responses and impairing the natural defense mechanisms of the lung (Figure 4). Understanding the molecular mechanisms by which pyocyanin manipulates host cell functions are vital for developing targeted therapeutic approaches to mitigate its harmful effects and improve patient outcomes.
Figure 4. The multifaceted impact of pyocyanin on lung cell function. Pyocyanin disrupts multiple cellular functions in the lung, leading to inflammation, tissue damage, and impaired immune response. Collectively, these effects contribute to the severity of P. aeruginosa infections, particularly in individuals who are immunocompromised or with underlying lung conditions. Figure generated with BioRender.com.
SL: Conceptualization, Formal analysis, Investigation, Methodology, Resources, Software, Validation, Visualization, Writing – original draft, Writing – review & editing. SC: Formal analysis, Investigation, Methodology, Resources, Software, Validation, Visualization, Writing – review & editing. GL: Conceptualization, Formal analysis, Funding acquisition, Investigation, Methodology, Project administration, Resources, Supervision, Validation, Visualization, Writing – review & editing.
The author(s) declare that financial support was received for the research and/or publication of this article. This work was supported by grants from the US National Institutes of Health (R01 HL142626 and R21AI171624) to GL. The funder has no role in the decision to publish the manuscript.
The authors declare that the research was conducted in the absence of any commercial or financial relationships that could be construed as a potential conflict of interest.
The author(s) declared that they were an editorial board member of Frontiers, at the time of submission. This had no impact on the peer review process and the final decision.
The author(s) declare that no Generative AI was used in the creation of this manuscript.
All claims expressed in this article are solely those of the authors and do not necessarily represent those of their affiliated organizations, or those of the publisher, the editors and the reviewers. Any product that may be evaluated in this article, or claim that may be made by its manufacturer, is not guaranteed or endorsed by the publisher.
2. Tramper-Stranders G, van der Ent C, Wolfs T. Detection of Pseudomonas aeruginosa in patients with cystic fibrosis. J Cystic Fibrosis. (2005) 4:37–43. doi: 10.1016/j.jcf.2005.05.009
3. Kang C-I, Kim SH, Kim HB, Park SW, Choe YJ, Oh MD, et al. Pseudomonas aeruginosa bacteremia: risk factors for mortality and influence of delayed receipt of effective antimicrobial therapy on clinical outcome. Clin Infect Dis. (2003) 37:745–51. doi: 10.1086/377200
4. Steele MP, Davis RD, Messier RH, Pietrobon R, Alexander BD, Rea JB, et al. Early colonization with pseudomonas in cystic fibrosis (CF) lung transplant recipients is associated with poor long-term survival. J Heart Lung Transplant. (2002) 21:107–8. doi: 10.1016/S1053-2498(01)00580-0
5. Szabo MM, Foushee SE, McPheeters CM, O'Hagan AR, Ramirez AM, O'Reilly EA, et al. Impact of elexacaftor/tezacaftor/ivacaftor on respiratory colonization in an adult cystic fibrosis clinic. Am J Med Sci. (2024) 367:337–42. doi: 10.1016/j.amjms.2024.02.001
6. Dittrich A-M, Sieber S, Naehrlich L, Burkhart M, Hafkemeyer S, Tümmler B, et al. Use of elexacaftor/tezacaftor/ivacaftor leads to changes in detection frequencies of Staphylococcus aureus and Pseudomonas aeruginosa dependent on age and lung function in people with cystic fibrosis. Int J Infect Dis. (2024) 139:124–31. doi: 10.1016/j.ijid.2023.11.013
7. Bou R, Lorente L, Aguilar A, Perpiñán J, Ramos P, Peris M, et al. Hospital economic impact of an outbreak of Pseudomonas aeruginosa infections. J Hosp Infection. (2009) 71:138–42. doi: 10.1016/j.jhin.2008.07.018
8. Morales E, Cots F, Sala M, Comas M, Belvis F, Riu M, et al. Hospital costs of nosocomial multi-drug resistant Pseudomonas aeruginosa acquisition. BMC Health Serv Res. (2012) 12:1–8. doi: 10.1186/1472-6963-12-122
9. Maurice NM, Bedi B, Sadikot RT. Pseudomonas aeruginosa biofilms: host response and clinical implications in lung infections. Am J Respir Cell Mol Biol. (2018) 58:428–39. doi: 10.1165/rcmb.2017-0321TR
10. Elfadadny A, Ragab RF, AlHarbi M, Badshah F, Ibáñez-Arancibia E, Farag A, et al. Antimicrobial resistance of Pseudomonas aeruginosa: navigating clinical impacts, current resistance trends, and innovations in breaking therapies. Front Microbiol. (2024) 15:1374466. doi: 10.3389/fmicb.2024.1374466
11. Miller WR, Arias CA. ESKAPE pathogens: antimicrobial resistance, epidemiology, clinical impact and therapeutics. Nat Rev Microbiol. (2024) 22:598–616. doi: 10.1038/s41579-024-01054-w
12. McKnight SL, Iglewski BH, Pesci EC. The Pseudomonas quinolone signal regulates rhl quorum sensing in Pseudomonas aeruginosa. J Bacteriol. (2000) 182:2702–8. doi: 10.1128/jb.182.10.2702-2708.2000
13. Pesci EC, Milbank JB, Pearson JP, McKnight S, Kende AS, Greenberg EP, et al. Quinolone signaling in the cell-to-cell communication system of Pseudomonas aeruginosa. Proc Natl Acad Sci. (1999) 96:11229–34. doi: 10.1073/pnas.96.20.11229
14. Déziel E, Gopalan S, Tampakaki AP, Lépine F, Padfield KE, Saucier M, et al. The contribution of MvfR to Pseudomonas aeruginosa pathogenesis and quorum sensing circuitry regulation: multiple quorum sensing-regulated genes are modulated without affecting lasRI, rhlRI or the production of N-acyl-l-homoserine lactones. Mol Microbiol. (2005) 55:998–1014. doi: 10.1111/j.1365-2958.2004.04448.x
15. Pearson JP, Gray KM, Passador L, Tucker KD, Eberhard A, Iglewski BH, et al. Structure of the autoinducer required for expression of Pseudomonas aeruginosa virulence genes. Proc Natl Acad Sci. (1994) 91:197–201. doi: 10.1073/pnas.91.1.197
16. Pearson JP, Pesci EC, Iglewski BH. Roles of Pseudomonas aeruginosa las and rhl quorum-sensing systems in control of elastase and rhamnolipid biosynthesis genes. J Bacteriol. (1997) 179:5756–67. doi: 10.1128/jb.179.18.5756-5767.1997
17. Xiao G, Déziel E, He J, Lépine F, Lesic B, Castonguay MH, et al. MvfR, a key Pseudomonas aeruginosa pathogenicity LTTR-class regulatory protein, has dual ligands. Mol Microbiol. (2006) 62:1689–99. doi: 10.1111/j.1365-2958.2006.05462.x
18. Mahajan-Miklos S, Tan M-W, Rahme LG, Ausubel FM. Molecular mechanisms of bacterial virulence elucidated using a Pseudomonas aeruginosa–Caenorhabditis elegans pathogenesis model. Cell. (1999) 96:47–56. doi: 10.1016/s0092-8674(00)80958-7
19. Pollack M. The role of exotoxin A in Pseudomonas disease and immunity. Rev Infect Dis. (1983) 5:S979–84. doi: 10.1093/clinids/5.Supplement_5.S979
20. Ołdak E, Trafny EA. Secretion of proteases by Pseudomonas aeruginosa biofilms exposed to ciprofloxacin. Antimicrobial Agents Chemother. (2005) 49:3281–8. doi: 10.1128/AAC.49.8.3281-3288.2005
21. Stinson MW, Hayden C. Secretion of phospholipase C by Pseudomonas aeruginosa. Infection Immun. (1979) 25:558–64. doi: 10.1128/iai.25.2.558-564.1979
22. Lau GW, Hassett DJ, Ran H, Kong F. The role of pyocyanin in Pseudomonas aeruginosa infection. Trends Mol Med. (2004) 10:599–606. doi: 10.1016/j.molmed.2004.10.002
23. Punchi Hewage AN, Fontenot L, Guidry J, Weldeghiorghis T, Mehta AK, Donnarumma F, et al. Mobilization of iron stored in bacterioferritin is required for metabolic homeostasis in Pseudomonas aeruginosa. Pathogens. (2020) 9:980. doi: 10.3390/pathogens9120980
24. Nadal Jimenez P, Koch G, Thompson JA, Xavier KB, Cool RH, Quax WJ, et al. The multiple signaling systems regulating virulence in Pseudomonas aeruginosa. Microbiol Mol Biol Rev. (2012) 76:46–65. doi: 10.1128/MMBR.05007-11
25. Rada B, Leto TL. Pyocyanin effects on respiratory epithelium: relevance in Pseudomonas aeruginosa airway infections. Trends Microbiol. (2013) 21:73–81. doi: 10.1016/j.tim.2012.10.004
26. Hao Y, Kuang Z, Xu Y, Walling BE, Lau GW. Pyocyanin-induced mucin production is associated with redox modification of FOXA2. Respir Res. (2013) 14:1–14. doi: 10.1186/1465-9921-14-82
27. Wilson R, et al. Measurement of Pseudomonas aeruginosa phenazine pigments in sputum and assessment of their contribution to sputum sol toxicity for respiratory epithelium. Infection Immun. (1988) 56:2515–7. doi: 10.1128/iai.56.9.2515-2517.1988
28. Yang Z-S, Ma L-Q, Zhu K, Yan J-Y, Bian L, Zhang K-Q, et al. Pseudomonas toxin pyocyanin triggers autophagy: implications for pathoadaptive mutations. Autophagy. (2016) 12:1015–28. doi: 10.1080/15548627.2016.1170256
29. Greenhagen BT, Shi K, Robinson H, Gamage S, Bera AK, Ladner JE, et al. Crystal structure of the pyocyanin biosynthetic protein PhzS. Biochemistry. (2008) 47:5281–9. doi: 10.1021/bi702480t
30. Jabłońska J, Augustyniak A, Dubrowska K, Rakoczy R. The two faces of pyocyanin-why and how to steer its production? World J Microbiol Biotechnol. (2023) 39:103. doi: 10.1007/s11274-023-03548-w
31. Petecchia L, Sabatini F, Usai C, Caci E, Varesio L, Rossi GA, et al. Cytokines induce tight junction disassembly in airway cells via an EGFR-dependent MAPK/ERK1/2-pathway. Lab Invest. (2012) 92:1140–8. doi: 10.1038/labinvest.2012.67
32. Meirelles LA, Newman DK. Both toxic and beneficial effects of pyocyanin contribute to the lifecycle of Pseudomonas aeruginosa. Mol Microbiol. (2018) 110:995–1010. doi: 10.1111/mmi.14132
33. Hassan HM, Fridovich I. Mechanism of the antibiotic action pyocyanine. J Bacteriol. (1980) 141:156–63. doi: 10.1128/jb.141.1.156-163.1980
34. Elkins JG, Hassett DJ, Stewart PS, Schweizer HP, McDermott TR. Protective role of catalase in Pseudomonas aeruginosa biofilm resistance to hydrogen peroxide. Appl Environ Microbiol. (1999) 65:4594–600. doi: 10.1128/AEM.65.10.4594-4600.1999
35. Hassett DJ, Schweizer HP, Ohman DE. Pseudomonas aeruginosa sodA and sodB mutants defective in manganese-and iron-cofactored superoxide dismutase activity demonstrate the importance of the iron-cofactored form in aerobic metabolism. J Bacteriol. (1995) 177:6330–7. doi: 10.1128/jb.177.22.6330-6337.1995
36. Ghorbal SKB, Maalej L, Chourabi K, Khefacha S, Ouzari H-I, Chatti A, et al. Antioxidant defense mechanisms in Pseudomonas aeruginosa: role of iron-cofactored superoxide dismutase in response to UV-C radiations. Curr Microbiol. (2016) 73:159–64. doi: 10.1007/s00284-016-1043-7
37. Somprasong N, Jittawuttipoka T, Duang-Nkern J, Romsang A, Chaiyen P, Schweizer HP, et al. Pseudomonas aeruginosa thiol peroxidase protects against hydrogen peroxide toxicity and displays atypical patterns of gene regulation. J Bacteriol. (2012) 194:3904–12. doi: 10.1128/JB.00347-12
38. Rahme LG, Tan M-W, Le L, Wong SM, Tompkins RG, Calderwood SB, et al. Use of model plant hosts to identify Pseudomonas aeruginosa virulence factors. Proc Natl Acad Sci. (1997) 94:13245–50. doi: 10.1073/pnas.94.24.13245
39. Thees A, Tan M-W, Le L, Wong SM, Tompkins RG, Calderwood SB, et al. PmtA regulates pyocyanin expression and biofilm formation in Pseudomonas aeruginosa. Front Microbiol. (2021) 12:789765. doi: 10.3389/fmicb.2021.789765
40. Hamad MN, Marrez DA, El-Sherbieny SM. Toxicity evaluation and antimicrobial activity of purified pyocyanin from Pseudomonas aeruginosa. Biointerface Res Appl Chem. (2020) 10:6974–90. doi: 10.33263/BRIAC106.69746990
41. Caldwell CC, Chen Y, Goetzmann HS, Hao Y, Borchers MT, Hassett DJ, et al. Pseudomonas aeruginosa exotoxin pyocyanin causes cystic fibrosis airway pathogenesis. Am J Pathol. (2009) 175:2473–88. doi: 10.2353/ajpath.2009.090166
42. Lin X, Song F, Wu Y, Xue D, Wang Y. Lycium barbarum polysaccharide attenuates Pseudomonas-aeruginosa pyocyanin-induced cellular injury in mice airway epithelial cells. Food Nutr Res. (2022) 66:4585. doi: 10.29219/fnr.v66.4585
43. Jeffries JL, Jia J, Choi W, Choe S, Miao J, Xu Y, et al. Pseudomonas aeruginosa pyocyanin modulates mucin glycosylation with sialyl-Lewisx to increase binding to airway epithelial cells. Mucosal Immunol. (2016) 9:1039–50. doi: 10.1038/mi.2015.119
44. Lauredo IT, Sabater JR, Ahmed A, Botvinnikova Y, Abraham WM. Mechanism of pyocyanin-and 1-hydroxyphenazine-induced lung neutrophilia in sheep airways. J Appl Physiol. (1998) 85:2298–304. doi: 10.1152/jappl.1998.85.6.2298
45. Rada B, Lekstrom K, Damian S, Dupuy C, Leto TL. The Pseudomonas toxin pyocyanin inhibits the dual oxidase-based antimicrobial system as it imposes oxidative stress on airway epithelial cells. J Immunol. (2008) 181:4883–93. doi: 10.4049/jimmunol.181.7.4883
46. O’Malley YQ, Reszka KJ, Spitz DR, Denning GM, Britigan BE. Pseudomonas aeruginosa pyocyanin directly oxidizes glutathione and decreases its levels in airway epithelial cells. Am J Physiol-Lung Cell Mol Physiol. (2004) 287:L94–L103. doi: 10.1152/ajplung.00025.2004
47. Li FJ, Surolia R, Li H, Wang Z, Liu G, Kulkarni T, et al. Citrullinated vimentin mediates development and progression of lung fibrosis. Sci Trans Med. (2021) 13:eaba2927. doi: 10.1126/scitranslmed.aba2927
48. Swart AL, Laventie B-J, Sütterlin R, Junne T, Lauer L, Manfredi P, et al. Pseudomonas aeruginosa breaches respiratory epithelia through goblet cell invasion in a microtissue model. Nat Microbiol. (2024) 9:1725–37. doi: 10.1038/s41564-024-01718-6
49. Muller M. Pyocyanin induces oxidative stress in human endothelial cells and modulates the glutathione redox cycle. Free Radical Biol Med. (2002) 33:1527–33. doi: 10.1016/s0891-5849(02)01087-0
50. Reszka KJ, O’Malley Y, McCormick ML, Denning GM, Britigan BE. Oxidation of pyocyanin, a cytotoxic product from Pseudomonas aeruginosa, by microperoxidase 11 and hydrogen peroxide. Free Radical Biol Med. (2004) 36:1448–59. doi: 10.1016/j.freeradbiomed.2004.03.011
51. Jacob C, Jamier V, Ba LA. Redox active secondary metabolites. Curr Opin Chem Biol. (2011) 15:149–55. doi: 10.1016/j.cbpa.2010.10.015
52. Barakat R, Goubet I, Manon S, Berges T, Rosenfeld E. Unsuspected pyocyanin effect in yeast under anaerobiosis. Microbiologyopen. (2014) 3:1–14. doi: 10.1002/mbo3.142
53. Imlay JA. The molecular mechanisms and physiological consequences of oxidative stress: lessons from a model bacterium. Nat Rev Microbiol. (2013) 11:443–54. doi: 10.1038/nrmicro3032
54. Ricciardolo FL, Di Stefano A, Sabatini F, Folkerts G. Reactive nitrogen species in the respiratory tract. Eur J Pharmacol. (2006) 533:240–52. doi: 10.1016/j.ejphar.2005.12.057
55. Srinivas US, Tan BW, Vellayappan BA, Jeyasekharan AD. ROS and the DNA damage response in cancer. Redox Biol. (2019) 25:101084. doi: 10.1016/j.redox.2018.101084
56. Su L-J, Zhang J-H, Gomez H, Murugan R, Hong X, Xu D, et al. Reactive oxygen species-induced lipid peroxidation in apoptosis, autophagy, and ferroptosis. Oxid Med Cell Longevity. (2019) 2019:1226044. doi: 10.3389/fcell.2023.1226044
57. Cai Z, Yan L-J. Protein oxidative modifications: beneficial roles in disease and health. J Biochem Pharmacol Res. (2013) 1:15.
58. Ran H, Hassett DJ, Lau GW. Human targets of Pseudomonas aeruginosa pyocyanin. Proc Natl Acad Sci. (2003) 100:14315–20. doi: 10.1073/pnas.2332354100
59. O’Malley YQ, Abdalla MY, McCormick ML, Reszka KJ, Denning GM, Britigan BE, et al. Subcellular localization of Pseudomonas pyocyanin cytotoxicity in human lung epithelial cells. Am J Physiol-Lung Cell Mol Physiol. (2003) 284:L420–30. doi: 10.1152/ajplung.00316.2002
60. Manni M, Oury T. Oxidative stress and pulmonary fibrosis. In: Systems biology of free radicals and antioxidants. Springer, Berlin, Heidelberg (2014). p. 1611–31. doi: 10.1016/j.bbadis.2012.11.021
61. Russell RJ, Boulet L-P, Brightling CE, Pavord ID, Porsbjerg C, Dorscheid D, et al. The airway epithelium: an orchestrator of inflammation, a key structural barrier and a therapeutic target in severe asthma. Eur Respir J. (2024) 63:2301397. doi: 10.1183/13993003.01397-2023
62. Xiao C, Puddicombe SM, Field S, Haywood J, Broughton-Head V, Puxeddu I, et al. Defective epithelial barrier function in asthma. J Allergy Clin Immunol. (2011) 128:549–56:e512. doi: 10.1016/j.jaci.2011.05.038
63. Frey A, Lunding LP, Ehlers JC, Weckmann M, Zissler UM, Wegmann M, et al. More than just a barrier: the immune functions of the airway epithelium in asthma pathogenesis. Front Immunol. (2020) 11761. doi: 10.3389/fimmu.2020.00761
64. Rogers LK, Cismowski MJ. Oxidative stress in the lung–the essential paradox. Curr Opin Toxicol. (2018) 7:37–43. doi: 10.1016/j.cotox.2017.09.001
65. Price ME, Sisson JH. Redox regulation of motile cilia in airway disease. Redox Biol. (2019) 27:101146. doi: 10.1016/j.redox.2019.101146
66. Bustamante-Marin XM, Ostrowski LE. Cilia and mucociliary clearance. Cold Spring Harbor Perspect Biol. (2017) 9:a028241. doi: 10.1101/cshperspect.a028241
67. Randell SH, Boucher RC. Effective mucus clearance is essential for respiratory health. Am J Respir Cell Mol Biol. (2006) 35:20–8. doi: 10.1165/rcmb.2006-0082SF
68. Wilson R, Pitt T, Taylor G, Watson D, MacDermot J, Sykes D, et al. Pyocyanin and 1-hydroxyphenazine produced by Pseudomonas aeruginosa inhibit the beating of human respiratory cilia in vitro. J Clin Invest. (1987) 79:221–9. doi: 10.1172/JCI112787
69. Kanthakumar K, Taylor G, Tsang K, Cundell D, Rutman A, Smith S, et al. Mechanisms of action of Pseudomonas aeruginosa pyocyanin on human ciliary beat in vitro. Infection Immun. (1993) 61:2848–53. doi: 10.1128/iai.61.7.2848-2853.1993
70. Murphy MP. How mitochondria produce reactive oxygen species. Biochem J. (2009) 417:1–13. doi: 10.1042/BJ20081386
71. Roberts AJ, Kon T, Knight PJ, Sutoh K, Burgess SA. Functions and mechanics of dynein motor proteins. Nat Rev Mol Cell Biol. (2013) 14:713–26. doi: 10.1038/nrm3667
73. Tilley AE, Walters MS, Shaykhiev R, Crystal RG. Cilia dysfunction in lung disease. Annu Rev Physiol. (2015) 77:379–406. doi: 10.1146/annurev-physiol-021014-071931
74. Kobayashi K, Salathé M, Pratt MM, Cartagena NJ, Soloni F, Seybold ZV, et al. Mechanism of hydrogen peroxide-induced inhibition of sheep airway cilia. Am J Respir Cell Mol Biol. (1992) 6:667–73. doi: 10.1165/ajrcmb/6.6.667
75. Salathe M, Pratt MM, Wanner A. Protein kinase C-dependent phosphorylation of a ciliary membrane protein and inhibition of ciliary beating. J Cell Sci. (1993) 106:1211–20. doi: 10.1242/jcs.106.4.1211
76. Bailey KL, Bonasera SJ, Wilderdyke M, Hanisch BW, Pavlik JA, DeVasure J, et al. Aging causes a slowing in ciliary beat frequency, mediated by PKCϵ. Am J Physiol-Lung Cell Mol Physiol. (2014) 306:L584–9. doi: 10.1152/ajplung.00175.2013
77. Bailey KL, Kharbanda KK, Katafiasz DM, Sisson JH, Wyatt TA. Oxidative stress associated with aging activates protein kinase Cϵ, leading to cilia slowing. Am J Physiol-Lung Cell Mol Physiol. (2018) 315:L882–90. doi: 10.1152/ajplung.00033.2018
78. Elliott MK, Sisson JH, Wyatt TA. Effects of cigarette smoke and alcohol on ciliated tracheal epithelium and inflammatory cell recruitment. Am J Respir Cell Mol Biol. (2007) 36:452–9. doi: 10.1165/rcmb.2005-0440OC
79. Fahy JV, Dickey BF. Airway mucus function and dysfunction. New Engl J Med. (2010) 363:2233–47. doi: 10.1056/NEJMra0910061
80. Yang X, Liu X, Nie Y, Zhan F, Zhu B. Oxidative stress and ROS-mediated cellular events in RSV infection: potential protective roles of antioxidants. Virol J. (2023) 20:224. doi: 10.1186/s12985-023-02194-w
81. Stout SL, Wyatt TA, Adams JJ, Sisson JH. Nitric oxide-dependent cilia regulatory enzyme localization in bovine bronchial epithelial cells. J Histochem Cytochem. (2007) 55:433–42. doi: 10.1369/jhc.6A7089.2007
82. Blackburn K, Bustamante-Marin X, Yin W, Goshe MB, Ostrowski LE. Quantitative proteomic analysis of human airway cilia identifies previously uncharacterized proteins of high abundance. J Proteome Res. (2017) 16:1579–92. doi: 10.1021/acs.jproteome.6b00972
83. Ostrowski LE, Blackburn K, Radde KM, Moyer MB, Schlatzer DM, Moseley A, et al. A proteomic analysis of human cilia: identification of novel components* S. Mol Cell Proteomics. (2002) 1:451–65. doi: 10.1074/mcp.m200037-mcp200
84. Lechuga S, Ivanov AI. Actin cytoskeleton dynamics during mucosal inflammation: A view from broken epithelial barriers. Curr Opin Physiol. (2021) 19:10–6. doi: 10.1016/j.cophys.2020.06.012
85. Ivanov AI, Parkos CA, Nusrat A. Cytoskeletal regulation of epithelial barrier function during inflammation. Am J Pathol. (2010) 177:512–24. doi: 10.2353/ajpath.2010.100168
86. Terman JR, Kashina A. Post-translational modification and regulation of actin. Curr Opin Cell Biol. (2013) 25:30–8. doi: 10.1016/j.ceb.2012.10.009
87. Hebbar S, Knust E. Reactive oxygen species (ROS) constitute an additional player in regulating epithelial development. BioEssays. (2021) 43:2100096. doi: 10.1002/bies.202100096
88. Fanning AS, Jameson BJ, Jesaitis LA, Anderson JM. The tight junction protein ZO-1 establishes a link between the transmembrane protein occludin and the actin cytoskeleton. J Biol Chem. (1998) 273:29745–53. doi: 10.1074/jbc.273.45.29745
89. Walter JK, Rueckert C, Voss M, Mueller S, Piontek J, Gast K, et al. The oligomerization of the coiled coil-domain of occluddin is redox sensitive. Ann New York Acad Sci. (2009) 1165:19–27. doi: 10.1111/j.1749-6632.2009.04058.x
90. Meng W, Takeichi M. Adherens junction: molecular architecture and regulation. Cold Spring Harbor Perspect Biol. (2009) 1:a002899. doi: 10.1101/cshperspect.a002899
91. Ferreri DM, Vincent PA. Signaling to and through the endothelial adherens junction. Cell Junctions: Adhesion, Development, and Disease. (2008) 169–95. doi: 10.1002/9783527622092.ch9
92. van Mourik JA, Leeksma O, Reinders J, De Groot PG, Zandbergen-Spaargaren J. Vascular endothelial cells synthesize a plasma membrane protein indistinguishable from the platelet membrane glycoprotein IIa. J Biol Chem. (1985) 260:11300–6. doi: 10.1016/S0021-9258(17)39180-9
93. Rada B, Gardina P, Myers TG, Leto TL. Reactive oxygen species mediate inflammatory cytokine release and EGFR-dependent mucin secretion in airway epithelial cells exposed to Pseudomonas pyocyanin. Mucosal Immunol. (2011) 4:158–71. doi: 10.1038/mi.2010.62
94. Yu H, Huang X, Ma Y, Gao M, Wang O, Gao T, et al. Interleukin-8 regulates endothelial permeability by down-regulation of tight junction but not dependent on integrins induced focal adhesions. Int J Biol Sci. (2013) 9:966. doi: 10.7150/ijbs.6996
95. Suzuki T, Yoshinaga N, Tanabe S. Interleukin-6 (IL-6) regulates claudin-2 expression and tight junction permeability in intestinal epithelium. J Biol Chem. (2011) 286:31263–71. doi: 10.1074/jbc.M111.238147
96. Al-Sadi RM, Ma TY. IL-1β causes an increase in intestinal epithelial tight junction permeability. J Immunol. (2007) 178:4641–9. doi: 10.4049/jimmunol.178.7.4641
97. Al-Sadi R, Guo S, Ye D, Ma TY. TNF-α modulation of intestinal epithelial tight junction barrier is regulated by ERK1/2 activation of Elk-1. Am J Pathol. (2013) 183:1871–84. doi: 10.1016/j.ajpath.2013.09.001
98. Bickel M. The role of interleukin-8 in inflammation and mechanisms of regulation. J Periodontol. (1993) 64:456–60.
99. Denning GM, Wollenweber LA, Railsback MA, Cox CD, Stoll LL, Britigan BE, et al. Pseudomonas pyocyanin increases interleukin-8 expression by human airway epithelial cells. Infection Immun. (1998) 66:5777–84. doi: 10.1128/IAI.66.12.5777-5784.1998
100. Ko JW, Lim SY, Chung KC, Lim JW, Kim H. Reactive oxygen species mediate IL-8 expression in Down syndrome candidate region-1-overexpressed cells. Int J Biochem Cell Biol. (2014) 55:164–70. doi: 10.1016/j.biocel.2014.08.017
101. DeForge LE, Preston AM, Takeuchi E, Kenney J, Boxer LA, Remick D, et al. Regulation of interleukin 8 gene expression by oxidant stress. J Biol Chem. (1993) 268:25568–76. doi: 10.1016/S0021-9258(19)74429-9
102. Lan C-CE, Wu C-S, Huang S-M, Wu I-H, Chen G-S. High-glucose environment enhanced oxidative stress and increased interleukin-8 secretion from keratinocytes: new insights into impaired diabetic wound healing. Diabetes. (2013) 62:2530–8. doi: 10.2337/db12-1714
103. Segal AW. How neutrophils kill microbes. Annu Rev Immunol. (2005) 23:197–223. doi: 10.1146/annurev.immunol.23.021704.115653
104. Sheshachalam A, Srivastava N, Mitchell T, Lacy P, Eitzen G. Granule protein processing and regulated secretion in neutrophils. Front Immunol. (2014) 5:448. doi: 10.3389/fimmu.2014.00448
105. Ronchetti S, Ricci E, Migliorati G, Gentili M, Riccardi C. How glucocorticoids affect the neutrophil life. Int J Mol Sci. (2018) 19:4090. doi: 10.3390/ijms19124090
106. Hirsch G, Lavoie-Lamoureux A, Beauchamp G, Lavoie J-P. Neutrophils are not less sensitive than other blood leukocytes to the genomic effects of glucocorticoids. PLoS One. (2012) 7:e44606. doi: 10.1371/journal.pone.0044606
107. Manago A, Becker KA, Carpinteiro A, Wilker B, Soddemann M, Seitz AP, et al. Pseudomonas aeruginosa pyocyanin induces neutrophil death via mitochondrial reactive oxygen species and mitochondrial acid sphingomyelinase. Antioxid Redox Signaling. (2015) 22:1097–110. doi: 10.1089/ars.2014.5979
108. Bianchi SM, Prince LR, McPhillips K, Allen L, Marriott HM, Taylor GW, et al. Impairment of apoptotic cell engulfment by pyocyanin, a toxic metabolite of Pseudomonas aeruginosa. Am J Respir Crit Care Med. (2008) 177:35–43. doi: 10.1164/rccm.200612-1804OC
109. Usher LR, Lawson RA, Geary I, Taylor CJ, Bingle CD, Taylor GW, et al. Induction of neutrophil apoptosis by the Pseudomonas aeruginosa exotoxin pyocyanin: a potential mechanism of persistent infection. J Immunol. (2002) 168:1861–8. doi: 10.4049/jimmunol.168.4.1861
110. Allen L, Dockrell DH, Pattery T, Lee DG, Cornelis P, Hellewell PG, et al. Pyocyanin production by Pseudomonas aeruginosa induces neutrophil apoptosis and impairs neutrophil-mediated host defenses. Vivo J Immunol. (2005) 174:3643–9. doi: 10.4049/jimmunol.174.6.3643
111. Taniguchi M, Okazaki T. Ceramide/sphingomyelin rheostat regulated by sphingomyelin synthases and chronic diseases in murine models. J Lipid Atheroscl. (2020) 9:380. doi: 10.12997/jla.2020.9.3.380
112. Haimovitz-Friedman A, Kolesnick RN, Fuks Z. Ceramide signaling in apoptosis. Br Med Bull. (1997) 53:539–53. doi: 10.1093/oxfordjournals.bmb.a011629
113. Rada B. Neutrophil extracellular traps. NADPH Oxidases: Methods Protoc. (2019) 1982:517–28. doi: 10.1007/978-1-4939-9424-3_31
114. Masucci MT, Minopoli M, Del Vecchio S, Carriero MV. The emerging role of neutrophil extracellular traps (NETs) in tumor progression and metastasis. Front Immunol. (2020) 111749. doi: 10.3389/fimmu.2020.01749
115. Remijsen Q, Kuijpers T, Wirawan E, Lippens S, Vandenabeele P, Vanden Berghe T, et al. Dying for a cause: NETosis, mechanisms behind an antimicrobial cell death modality. Cell Death Differentiation. (2011) 18:581–8. doi: 10.1038/cdd.2011.1
116. Brinkmann V, Reichard U, Goosmann C, Fauler B, Uhlemann Y, Weiss DS, et al. Neutrophil extracellular traps kill bacteria. Science. (2004) 303:1532–5. doi: 10.1126/science.1092385
117. Li Y, Li M, Weigel B, Mall M, Werth VP, Liu ML, et al. Nuclear envelope rupture and NET formation is driven by PKCα-mediated lamin B disassembly. EMBO Rep. (2020) 21:e48779. doi: 10.15252/embr.201948779
118. Neeli I, Khan SN, Radic M. Histone deimination as a response to inflammatory stimuli in neutrophils. J Immunol. (2008) 180:1895–902. doi: 10.4049/jimmunol.180.3.1895
119. Wang Y, Li M, Stadler S, Correll S, Li P, Wang D, et al. Histone hypercitrullination mediates chromatin decondensation and neutrophil extracellular trap formation. J Cell Biol. (2009) 184:205–13. doi: 10.1083/jcb.200806072
120. Boeltz S, Amini P, Anders H-J, Andrade F, Bilyy R, Chatfield S, et al. To NET or not to NET: current opinions and state of the science regarding the formation of neutrophil extracellular traps. Cell Death Differentiation. (2019) 26:395–408. doi: 10.1038/s41418-018-0261-x
121. Schultz BM, Acevedo OA, Kalergis AM, Bueno SM. Role of extracellular trap release during bacterial and viral infection. Front Microbiol. (2022) 13:798853. doi: 10.3389/fmicb.2022.798853
122. Thanabalasuriar A, Scott BNV, Peiseler M, Willson ME, Zeng Z, Warrener P, et al. Neutrophil extracellular traps confine Pseudomonas aeruginosa ocular biofilms and restrict brain invasion. Cell Host Microbe. (2019) 25:526–36:e524. doi: 10.1016/j.chom.2019.02.007
123. Rada B, Jendrysik MA, Pang L, Hayes CP, Yoo Park D-g JJ, et al. Pyocyanin-enhanced neutrophil extracellular trap formation requires the NADPH oxidase. PloS One. (2013) 8:e54205. doi: 10.1371/journal.pone.0054205
124. Keir HR, Chalmers JD. Neutrophil extracellular traps in chronic lung disease: implications for pathogenesis and therapy. Eur Respir Rev. (2022) 31:210241. doi: 10.1183/16000617.0241-2021
125. Gray RD, Hardisty G, Regan KH, Smith M, Robb CT, Duffin R, et al. Delayed neutrophil apoptosis enhances NET formation in cystic fibrosis. Thorax. (2018) 73:134–44. doi: 10.1136/thoraxjnl-2017-210134
126. Katsoulis O, Toussaint M, Jackson MM, Mallia P, Footitt J, Mincham KT, et al. Neutrophil extracellular traps promote immunopathogenesis of virus-induced COPD exacerbations. Nat Commun. (2024) 15:5766. doi: 10.1038/s41467-024-50197-0
127. Chen S, Saeed AF, Liu Q, Jiang Q, Xu H, Xiao GG, et al. Macrophages in immunoregulation and therapeutics. Signal Transduction Targeted Ther. (2023) 8:207. doi: 10.1038/s41392-023-01452-1
128. Arts RJ, Netea MG. Adaptive characteristics of innate immune responses in macrophages. Microbiol Spectr. (2016) 4. doi: 10.1128/microbiolspec.MCHD-0023-2015
129. Barker RN, Erwig LP, Hill K, Devine A, Pearce W, Rees AJ. Antigen presentation by macrophages is enhanced by the uptake of necrotic, but not apoptotic, cells. Clin Exp Immunol. (2002) 127:220–5. doi: 10.1046/j.1365-2249.2002.01774.x
130. Navegantes KC, de Souza Gomes R, Pereira PAT, Czaikoski PG, Azevedo CHM, Monteiro MC. Immune modulation of some autoimmune diseases: the critical role of macrophages and neutrophils in the innate and adaptive immunity. J Trans Med. (2017) 15:1–21. doi: 10.1186/s12967-017-1141-8
131. Gray MA, Choy CH, Dayam RM, Ospina-Escobar E, Somerville A, Xiao X, et al. Phagocytosis enhances lysosomal and bactericidal properties by activating the transcription factor TFEB. Curr Biol. (2016) 26:1955–64. doi: 10.1016/j.cub.2016.05.070
132. Canton M, Sánchez-Rodríguez R, Spera I, Venegas FC, Favia M, Viola A, et al. Reactive oxygen species in macrophages: sources and targets. Front Immunol. (2021) 12734229. doi: 10.3389/fimmu.2021.734229
133. Zhu F, Xiong F, He J, Liu K, You Y, Xu Q, et al. Brd4 inhibition ameliorates Pyocyanin-mediated macrophage dysfunction via transcriptional repression of reactive oxygen and nitrogen free radical pathways. Cell Death Dis. (2020) 11:459. doi: 10.1038/s41419-020-2672-0
134. Kourtzelis I, Hajishengallis G, Chavakis T. Phagocytosis of apoptotic cells in resolution of inflammation. Front Immunol. (2020) 11:553. doi: 10.3389/fimmu.2020.00553
135. Gordon S, Plüddemann A. Macrophage clearance of apoptotic cells: a critical assessment. Front Immunol. (2018) 9:127. doi: 10.3389/fimmu.2018.00127
136. Leverrier Y, Ridley AJ. Requirement for Rho GTPases and PI 3-kinases during apoptotic cell phagocytosis by macrophages. Curr Biol. (2001) 11:195–9. doi: 10.1016/s0960-9822(01)00047-1
137. Lodoen MB, Lanier LL. Natural killer cells as an initial defense against pathogens. Curr Opin Immunol. (2006) 18:391–8. doi: 10.1016/j.coi.2006.05.002
138. Cerwenka A, Lanier LL. Natural killer cells, viruses and cancer. Nat Rev Immunol. (2001) 1:41–9. doi: 10.1038/35095564
139. Kumar S. Natural killer cell cytotoxicity and its regulation by inhibitory receptors. Immunology. (2018) 154:383–93. doi: 10.1111/imm.12921
140. Vivier E, Tomasello E, Baratin M, Walzer T, Ugolini S. Functions of natural killer cells. Nat Immunol. (2008) 9:503–10. doi: 10.1038/ni1582
141. Garcia-Penarrubia P, Koster F, Kelley R, McDowell T, Bankhurst A. Antibacterial activity of human natural killer cells. J Exp Med. (1989) 169:99–113. doi: 10.1084/jem.169.1.99
142. Souza-Fonseca-Guimaraes F, Adib-Conquy M, Cavaillon J-M. Natural killer (NK) cells in antibacterial innate immunity: angels or devils? Mol Med. (2012) 18:270–85. doi: 10.2119/molmed.2011.00201
143. Reed S. In vivo administration of recombinant IFN-gamma induces macrophage activation, and prevents acute disease, immune suppression, and death in experimental Trypanosoma cruzi infections. J Immunol (Baltimore Md: 1950). (1988) 140:4342–7. doi: 10.4049/jimmunol.140.12.4342
144. Chung JW, Piao Z-H, Yoon SR, Kim MS, Jeong M, Lee SH, et al. Pseudomonas aeruginosa eliminates natural killer cells via phagocytosis-induced apoptosis. PloS Pathog. (2009) 5:e1000561. doi: 10.1371/journal.ppat.1000561
145. Li T, Huang X, Yuan Z, Wang L, Chen M, Su F, et al. Pyocyanin induces NK92 cell apoptosis via mitochondrial damage and elevated intracellular Ca2+. Innate Immun. (2019) 25:3–12. doi: 10.1177/1753425918809860
146. Hall S, McDermott C, Anoopkumar-Dukie S, McFarland AJ, Forbes A, Perkins AV, et al. Cellular effects of pyocyanin, a secreted virulence factor of Pseudomonas aeruginosa. Toxins. (2016) 8:236. doi: 10.3390/toxins8080236
147. Morgan MJ. amp]]amp; Liu, Z.-g. Crosstalk of reactive oxygen species and NF-κB signaling. Cell Res. (2011) 21:103–15. doi: 10.1038/cr.2010.178
148. Kanterewicz BI, Knapp LT, Klann E. Stimulation of p42 and p44 mitogen-activated protein kinases by reactive oxygen species and nitric oxide in hippocampus. J Neurochem. (1998) 70:1009–16. doi: 10.1046/j.1471-4159.1998.70031009.x
149. McDermott C, Chess-Williams R, Mills K, Kang S, Farr S, Grant G, et al. Alterations in acetylcholine, PGE2 and IL6 release from urothelial cells following treatment with pyocyanin and lipopolysaccharide. Toxicol Vitro. (2013) 27:1693–8. doi: 10.1016/j.tiv.2013.04.015
150. Ulmer AJ, Pryjma J, Tarnok Z, Ernst M, Flad H. Inhibitory and stimulatory effects of Pseudomonas aeruginosa pyocyanine on human T and B lymphocytes and human monocytes. Infection Immun. (1990) 58:808–15. doi: 10.1128/iai.58.3.808-815.1990
151. Leidal KG, Munson KL, Denning GM. Small molecular weight secretory factors from Pseudomonas aeruginosa have opposite effects on IL-8 and RANTES expression by human airway epithelial cells. Am J Respir Cell Mol Biol. (2001) 25:186–95. doi: 10.1165/ajrcmb.25.2.4273
152. Ren K, Torres R. Role of interleukin-1β during pain and inflammation. Brain Res Rev. (2009) 60:57–64. doi: 10.1016/j.brainresrev.2008.12.020
153. Tanaka T, Narazaki M, Kishimoto T. IL-6 in inflammation, immunity, and disease. Cold Spring Harbor Perspect Biol. (2014) 6:a016295. doi: 10.1101/cshperspect.a016295
154. Germolec DR, Shipkowski KA, Frawley RP, Evans E. Markers of inflammation. Immunotoxicity Testing: Methods Protoc. (2018) 1803:57–79. doi: 10.1007/978-1-4939-8549-4_5
155. Hannoodee S, Nasuruddin DN. Acute inflammatory response. Treasure Island (FL): StatPearls Publishing (2020).
156. Vliagoftis H, Befus AD, Hollenberg MD, Moqbel R. Airway epithelial cells release eosinophil survival–promoting factors (GM-CSF) after stimulation of proteinase-activated receptor 2. J Allergy Clin Immunol. (2001) 107:679–85. doi: 10.1067/mai.2001.114245
157. Saba S, Soong G, Greenberg S, Prince A. Bacterial stimulation of epithelial G-CSF and GM-CSF expression promotes PMN survival in CF airways. Am J Respir Cell Mol Biol. (2002) 27:561–7. doi: 10.1165/rcmb.2002-0019OC
158. Korbecki J, Gąssowska-Dobrowolska M, Wójcik J, Szatkowska I, Barczak K, Chlubek M, et al. The importance of CXCL1 in physiology and noncancerous diseases of bone, bone marrow, muscle and the nervous system. Int J Mol Sci. (2022) 23:4205. doi: 10.3390/ijms23084205
159. Dubin PJ, Kolls JK. IL-23 mediates inflammatory responses to mucoid Pseudomonas aeruginosa lung infection in mice. Am J Physiol-Lung Cell Mol Physiol. (2007) 292:L519–28. doi: 10.1152/ajplung.00312.2006
160. Waite JC, Skokos D. Th17 response and inflammatory autoimmune diseases. Int J Inflammation. (2012) 2012:819467. doi: 10.1155/2012/819467
161. Khader SA, Gaffen SL, Kolls JK. Th17 cells at the crossroads of innate and adaptive immunity against infectious diseases at the mucosa. Mucosal Immunol. (2009) 2:403–11. doi: 10.1038/mi.2009.100
162. Riordan JR, Rommens JM, Kerem Alon B-s N, Rozmahel R, Grzelczak Z, et al. Identification of the cystic fibrosis gene: cloning and characterization of complementary DNA. Science. (1989) 245:1066–73. doi: 10.1126/science.2475911
163. Welsh MJ, Smith AE. Molecular mechanisms of CFTR chloride channel dysfunction in cystic fibrosis. Cell. (1993) 73:1251–4. doi: 10.1016/0092-8674(93)90353-r
164. Csanády L, Vergani P, Gadsby DC. Structure, gating, and regulation of the CFTR anion channel. Physiol Rev. (2019) 99:707–38. doi: 10.1152/physrev.00007.2018
165. Tümmler B. Puzzle resolved: CFTR mediates chloride homeostasis by segregating absorption and secretion to different cell types. J Clin Invest. (2023) 133(20):e174667. doi: 10.1172/JCI174667
166. Stoltz DA, Meyerholz DK, Welsh MJ. Origins of cystic fibrosis lung disease. New Engl J Med. (2015) 372:351–62. doi: 10.1056/NEJMra1300109
167. Zajac M, Dreano E, Edwards A, Planelles G, Sermet-Gaudelus I. Airway surface liquid pH regulation in airway epithelium current understandings and gaps in knowledge. Int J Mol Sci. (2021) 22:3384. doi: 10.3390/ijms22073384
168. Knowles MR, Boucher RC. Mucus clearance as a primary innate defense mechanism for mammalian airways. J Clin Invest. (2002) 109:571–7. doi: 10.1172/JCI15217
169. Ma J, Rubin BK, Voynow JA. Mucins, mucus, and goblet cells. Chest. (2018) 154:169–76. doi: 10.1016/j.chest.2017.11.008
170. Wanner A, Salathé M, O’Riordan TG. Mucociliary clearance in the airways. Am J Respir Crit Care Med. (1996) 154:1868–902. doi: 10.1164/ajrccm.154.6.8970383
171. Meldrum OW, Chotirmall SH. Mucus, microbiomes and pulmonary disease. Biomedicines. (2021) 9:675. doi: 10.3390/biomedicines9060675
172. Sethi S, Maloney J, Grove L, Wrona C, Berenson CS. Airway inflammation and bronchial bacterial colonization in chronic obstructive pulmonary disease. Am J Respir Crit Care Med. (2006) 173:991–8. doi: 10.1164/rccm.200509-1525OC
173. Choi W, Choe S, Lin J, Borchers MT, Kosmider B, Vassallo R, et al. Exendin-4 restores airway mucus homeostasis through the GLP1R-PKA-PPARγ-FOXA2-phosphatase signaling. Mucosal Immunol. (2020) 13:637–51. doi: 10.1038/s41385-020-0262-1
174. Schwarzer C, Fischer H, Kim E-J, Barber KJ, Mills AD, Kurth MJ, et al. Oxidative stress caused by pyocyanin impairs CFTR Cl– transport in human bronchial epithelial cells. Free Radical Biol Med. (2008) 45:1653–62. doi: 10.1016/j.freeradbiomed.2008.09.011
175. Swiatecka-Urban A, Moreau-Marquis S, MacEachran DP, Connolly JP, Stanton CR, Su JR, et al. Pseudomonas aeruginosa inhibits endocytic recycling of CFTR in polarized human airway epithelial cells. Am J Physiol-cell Physiol. (2006) 290:C862–72. doi: 10.1152/ajpcell.00108.2005
176. Kong F, Young L, Chen Y, Ran H, Meyers M, Joseph P, et al. Pseudomonas aeruginosa pyocyanin inactivates lung epithelial vaculoar ATPase-dependent cystic fibrosis transmembrane conductance regulator expression and localization. Cell Microbiol. (2006) 8:1121–33. doi: 10.1111/j.1462-5822.2006.00696.x
177. Bonhiver R, Bricmont N, Pirotte M, Wuidart M-A, Monseur J, Benchimol L, et al. Evidence for secondary ciliary dyskinesia in patients with cystic fibrosis. J Cystic Fibrosis. (2024) 24:193–200. doi: 10.1016/j.jcf.2024.10.003
178. Shah BK, Singh B, Wang Y, Xie S, Wang C. Mucus hypersecretion in chronic obstructive pulmonary disease and its treatment. Mediators Inflammation. (2023) 2023:8840594. doi: 10.1155/2023/8840594
179. Hao Y, Kuang Z, Walling BE, Bhatia S, Sivaguru M, Chen Y, et al. Pseudomonas aeruginosa pyocyanin causes airway goblet cell hyperplasia and metaplasia and mucus hypersecretion by inactivating the transcriptional factor FoxA2. Cell Microbiol. (2012) 14:401–15. doi: 10.1111/j.1462-5822.2011.01727.x
180. Xu Y, Duan C, Kuang Z, Hao Y, Jeffries JL, Lau GW. Pseudomonas aeruginosa pyocyanin activates NRF2-ARE-mediated transcriptional response via the ROS-EGFR-PI3K-AKT/MEK-ERK MAP kinase signaling in pulmonary epithelial cells. PloS One. (2013) 8:e72528. doi: 10.1371/journal.pone.0072528
181. Choi W, Yang AX, Waltenburg MA, Choe S, Steiner M, Radwan A, et al. FOXA2 depletion leads to mucus hypersecretion in canine airways with respiratory diseases. Cell Microbiol. (2019) 21:e12957. doi: 10.1111/cmi.12957
182. Choi W, Choe S, Lau GW. Inactivation of FOXA2 by respiratory bacterial pathogens and dysregulation of pulmonary mucus homeostasis. Front Immunol. (2020) 11:515. doi: 10.3389/fimmu.2020.00515
183. Wan H, Kaestner KH, Ang S-L, Ikegami M, Finkelman FD, Stahlman MT, et al. Foxa2 regulates alveolarization and goblet cell hyperplasia. Development. (2004) 131:953‐64. doi: 10.1242/dev.00966
184. Park S-W, Verhaeghe C, Nguyenvu LT, Barbeau R, Eisley CJ, Nakagami Y, et al. Distinct roles of FOXA2 and FOXA3 in allergic airway disease and asthma. Am J Respir Crit Care Med. (2009) 180:603–10. doi: 10.1164/rccm.200811-1768OC
185. Chen G, Wan H, Luo F, Zhang L, Xu Y, Lewkowich I, et al. Foxa2 programs Th2 cell-mediated innate immunity in the developing lung. J Immunol. (2010) 184:6133–41. doi: 10.4049/jimmunol.1000223
186. Kuperman D, Schofield B, Wills-Karp M, Grusby MJ. Signal transducer and activator of transcription factor 6 (Stat6)-deficient mice are protected from antigen-induced airway hyperresponsiveness and mucus production. J Exp Med. (1998) 187:939–48. doi: 10.1084/jem.187.6.939
187. Choi W, Yang AX, Sieve A, Kuo SH, Mudalagiriyappa S, Vieson M, et al. Pulmonary mycosis drives forkhead box protein A2 degradation and mucus hypersecretion through activation of the spleen tyrosine kinase–epidermal growth factor receptor–AKT/extracellular signal-regulated kinase 1/2 signaling. Am J Pathol. (2021) 191:108–30. doi: 10.1016/j.ajpath.2020.09.013
188. van Gent R, Di Sanza C, van den Broek NJ, Fleskens V, Veenstra A, Stout GJ, et al. SIRT1 mediates FOXA2 breakdown by deacetylation in a nutrient-dependent manner. PloS One. (2014) 9:e98438. doi: 10.1371/journal.pone.0098438
189. von Meyenn F, Porstmann T, Gasser E, Selevsek N, Schmidt A, Aebersold R, et al. Glucagon-induced acetylation of Foxa2 regulates hepatic lipid metabolism. Cell Metab. (2013) 17:436–47. doi: 10.1016/j.cmet.2013.01.014
190. Takeyama K, Dabbagh K, Lee H-M, Agustí C, Lausier JA, Ueki IF, et al. Epidermal growth factor system regulates mucin production in airways. Proc Natl Acad Sci. (1999) 96:3081–6. doi: 10.1073/pnas.96.6.3081
191. Takeyama K, Fahy JV, Nadel JA. Relationship of epidermal growth factor receptors to goblet cell production in human bronchi. Am J Respir Crit Care Med. (2001) 163:511–6. doi: 10.1164/ajrccm.163.2.2001038
192. Batson BD, Zorn BT, Radicioni G, Livengood SS, Kumagai T, Dang H, et al. Cystic fibrosis airway mucus hyperconcentration produces a vicious cycle of mucin, pathogen, and inflammatory interactions that promotes disease persistence. Am J Respir Cell Mol Biol. (2022) 67:253–65. doi: 10.1165/rcmb.2021-0359OC
193. Radicioni G, Ceppe A, Ford AA, Alexis NE, Barr RG, Bleecker ER, et al. Airway mucin MUC5AC and MUC5B concentrations and the initiation and progression of chronic obstructive pulmonary disease: an analysis of the SPIROMICS cohort. Lancet Respir Med. (2021) 9:1241–54. doi: 10.1016/S2213-2600(21)00079-5
194. Blanchard C, Durual S, Estienne M, Bouzakri K, Heim MH, Blin N, et al. IL-4 and IL-13 up-regulate intestinal trefoil factor expression: requirement for STAT6 and de novo protein synthesis. J Immunol. (2004) 172:3775–83. doi: 10.4049/jimmunol.172.6.3775
195. Kis LL, Gerasimčik N, Salamon D, Persson EK, Nagy N, Klein G, et al. STAT6 signaling pathway activated by the cytokines IL-4 and IL-13 induces expression of the Epstein-Barr virus–encoded protein LMP-1 in absence of EBNA-2: Implications for the type II EBV latent gene expression in Hodgkin lymphoma. Blood J Am Soc Hematol. (2011) 117:165–74. doi: 10.1182/blood-2010-01-265272
196. Chen G, Volmer AS, Wilkinson KJ, Deng Y, Jones LC, Yu D, et al. Role of Spdef in the regulation of Muc5b expression in the airways of naive and mucoobstructed mice. Am J Respir Cell Mol Biol. (2018) 59:383–96. doi: 10.1165/rcmb.2017-0127OC
197. Chen G, Korfhagen TR, Xu Y, Kitzmiller J, Wert SE, Maeda Y, et al. SPDEF is required for mouse pulmonary goblet cell differentiation and regulates a network of genes associated with mucus production. J Clin Invest. (2009) 119:2914–24. doi: 10.1172/JCI39731
198. Grootjans J, Kaser A, Kaufman RJ, Blumberg RS. The unfolded protein response in immunity and inflammation. Nat Rev Immunol. (2016) 16:469–84. doi: 10.1038/nri.2016.62
199. Wang X, Yang X, Li Y, Wang X, Zhang Y, Dai X, et al. Lyn kinase represses mucus hypersecretion by regulating IL-13-induced endoplasmic reticulum stress in asthma. EBioMedicine. (2017) 15:137–49. doi: 10.1016/j.ebiom.2016.12.010
200. van ‘t Wout EF, van Schadewijk A, van Boxtel R, Dalton LE, Clarke HJ, Tommassen J, et al. Virulence factors of Pseudomonas aeruginosa induce both the unfolded protein and integrated stress responses in airway epithelial cells. PloS Pathog. (2015) 11:e1004946. doi: 10.1371/journal.ppat.1004946
201. Ohman D, Chakrabarty A. Utilization of human respiratory secretions by mucoid Pseudomonas aeruginosa of cystic fibrosis origin. Infection Immun. (1982) 37:662–9. doi: 10.1128/iai.37.2.662-669.1982
202. Jennings LK, Dreifus JE, Reichhardt C, Storek KM, Secor PR, Wozniak DJ, et al. Pseudomonas aeruginosa aggregates in cystic fibrosis sputum produce exopolysaccharides that likely impede current therapies. Cell Rep. (2021) 34(8):108782. doi: 10.1016/j.celrep.2021.108782
203. Uzeloto JS, Ramos D, de Alencar Silva BS, de Lima MBP, Silva RN, Camillo CA, et al. Mucociliary clearance of different respiratory conditions: A clinical study. Int Arch Otorhinolaryngol. (2021) 25:e35–40. doi: 10.1055/s-0039-3402495
204. Pancu DF, Scurtu A, Macasoi IG, Marti D, Mioc M, Soica C, et al. Antibiotics: conventional therapy and natural compounds with antibacterial activity—a pharmaco-toxicological screening. Antibiotics. (2021) 10:401. doi: 10.3390/antibiotics10040401
205. Salam MA, Al-Amin MY, Pawar JS, Akhter N, Lucy IB. Conventional methods and future trends in antimicrobial susceptibility testing. Saudi J Biol Sci. (2023) 30:103582. doi: 10.1016/j.sjbs.2023.103582
206. Kwon DH, Cha H-J, Lee H, Hong S-H, Park C, Park S-H, et al. Protective effect of glutathione against oxidative stress-induced cytotoxicity in RAW 264.7 macrophages through activating the nuclear factor erythroid 2-related factor-2/heme oxygenase-1 pathway. Antioxidants. (2019) 8:82. doi: 10.3390/antiox8040082
207. Lima EMF, de Almeida FA, Sircili MP, Bueris V, Pinto UM. N-acetylcysteine (NAC) attenuates quorum sensing regulated phenotypes in Pseudomonas aeruginosa PAO1. Heliyon. (2023) 9(3):e14152. doi: 10.1016/j.heliyon.2023.e14152
208. Aldini G, Altomare A, Baron G, Vistoli G, Carini M, Borsani L, et al. N-Acetylcysteine as an antioxidant and disulphide breaking agent: the reasons why. Free Radical Res. (2018) 52:751–62. doi: 10.1080/10715762.2018.1468564
209. Mokhtari V, Afsharian P, Shahhoseini M, Kalantar SM, Moini A. A review on various uses of N-acetyl cysteine. Cell J (Yakhteh). (2016) 19:11. doi: 10.22074/cellj.2016.4872
210. Sui SJH, Lo R, Fernandes AR, Caulfield MD, Lerman JA, Xie L, et al. Raloxifene attenuates Pseudomonas aeruginosa pyocyanin production and virulence. Int J Antimicrobial Agents. (2012) 40:246–51. doi: 10.1016/j.ijantimicag.2012.05.009
211. Singh VK, Almpani M, Maura D, Kitao T, Ferrari L, Fontana S, et al. Tackling recalcitrant Pseudomonas aeruginosa infections in critical illness via anti-virulence monotherapy. Nat Commun. (2022) 13:5103. doi: 10.1038/s41467-022-32833-9
212. O’Loughlin CT, Miller LC, Siryaporn A, Drescher K, Semmelhack MF, Bassler BL, et al. A quorum-sensing inhibitor blocks Pseudomonas aeruginosa virulence and biofilm formation. Proc Natl Acad Sci. (2013) 110:17981–6. doi: 10.1073/pnas.1316981110
213. Swem LR, Swem DL, O'Loughlin CT, Gatmaitan R, Zhao B, Ulrich SM, et al. A quorum-sensing antagonist targets both membrane-bound and cytoplasmic receptors and controls bacterial pathogenicity. Mol Cell. (2009) 35:143–53. doi: 10.1016/j.molcel.2009.05.029
214. Stoltz DA, Ozer EA, Ng CJ, Yu JM, Reddy ST, Lusis AJ, et al. Paraoxonase-2 deficiency enhances Pseudomonas aeruginosa quorum sensing in murine tracheal epithelia. Am J Physiol-Lung Cell Mol Physiol. (2007) 292:L852–60. doi: 10.1152/ajplung.00370.2006
215. Chun CK, Ozer EA, Welsh MJ, Zabner J, Greenberg EP. Inactivation of a Pseudomonas aeruginosa quorum-sensing signal by human airway epithelia. Proc Natl Acad Sci. (2004) 101:3587–90. doi: 10.1073/pnas.0308750101
216. Murugayah SA, Gerth ML. Engineering quorum quenching enzymes: progress and perspectives. Biochem Soc Trans. (2019) 47:793–800. doi: 10.1042/BST20180165
217. Liu N, Yu M, Zhao Y, Cheng J, An K, Zhang X-H. PfmA, a novel quorum-quenching N-acylhomoserine lactone acylase from Pseudoalteromonas flavipulchra. Microbiology. (2017) 163:1389–98. doi: 10.1099/mic.0.000535
218. Strzelec M, Detka J, Mieszczak P, Sobocińska MK, Majka M. Immunomodulation—a general review of the current state-of-the-art and new therapeutic strategies for targeting the immune system. Front Immunol. (2023) 14:1127704. doi: 10.3389/fimmu.2023.1127704
219. Zhang S, Yang H, Wang M, Mantovani D, Yang K, Witte F, et al. Immunomodulatory biomaterials against bacterial infections: Progress, challenges, and future perspectives. Innovation. (2023) 4:100503. doi: 10.1016/j.xinn.2023.100503
Keywords: Pseudomonas aeruginosa, pyocyanin, reactive oxygen and nitrogen species, oxidative stress, immune modulation, chronic lung diseases
Citation: Lew SQ, Chong SY and Lau GW (2025) Modulation of pulmonary immune functions by the Pseudomonas aeruginosa secondary metabolite pyocyanin. Front. Immunol. 16:1550724. doi: 10.3389/fimmu.2025.1550724
Received: 23 December 2024; Accepted: 05 March 2025;
Published: 24 March 2025.
Edited by:
Daniel P Potaczek, University of Marburg, GermanyReviewed by:
Tarek A. Ahmad, Bibliotheca Alexandrina, EgyptCopyright © 2025 Lew, Chong and Lau. This is an open-access article distributed under the terms of the Creative Commons Attribution License (CC BY). The use, distribution or reproduction in other forums is permitted, provided the original author(s) and the copyright owner(s) are credited and that the original publication in this journal is cited, in accordance with accepted academic practice. No use, distribution or reproduction is permitted which does not comply with these terms.
*Correspondence: Gee W. Lau, Z2VlbGF1QGlsbGlub2lzLmVkdQ==
Disclaimer: All claims expressed in this article are solely those of the authors and do not necessarily represent those of their affiliated organizations, or those of the publisher, the editors and the reviewers. Any product that may be evaluated in this article or claim that may be made by its manufacturer is not guaranteed or endorsed by the publisher.
Research integrity at Frontiers
Learn more about the work of our research integrity team to safeguard the quality of each article we publish.