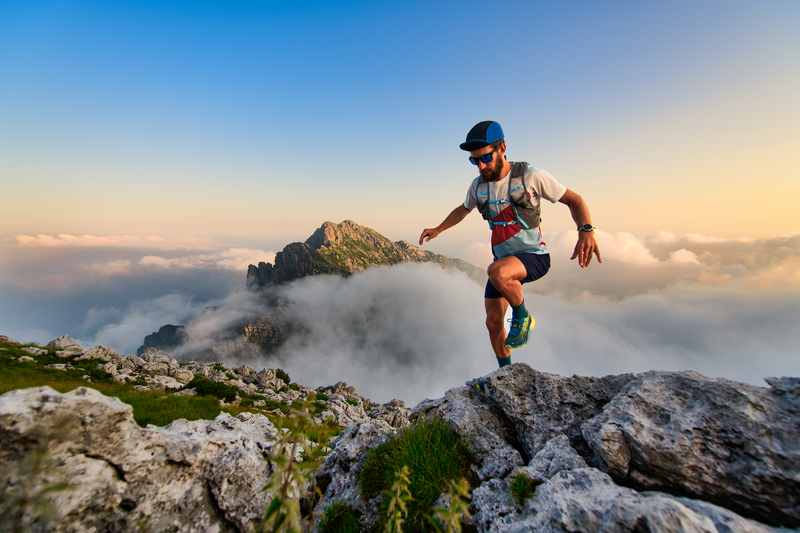
95% of researchers rate our articles as excellent or good
Learn more about the work of our research integrity team to safeguard the quality of each article we publish.
Find out more
REVIEW article
Front. Immunol. , 07 February 2025
Sec. Microbial Immunology
Volume 16 - 2025 | https://doi.org/10.3389/fimmu.2025.1519925
A correction has been applied to this article in:
Corrigendum: The role of short-chain fatty acid in metabolic syndrome and its complications: focusing on immunity and inflammation
Metabolic syndrome (Mets) is an important contributor to morbidity and mortality in cardiovascular, liver, neurological, and reproductive diseases. Short-chain fatty acid (SCFA), an organismal energy donor, has recently been demonstrated in an increasing number of studies to be an important molecule in ameliorating immuno-inflammation, an important causative factor of Mets, and to improve lipid distribution, blood glucose, and body weight levels in animal models of Mets. This study reviews recent research advances on SCFA in Mets from an immune-inflammatory perspective, including complications dominated by chronic inflammation, as well as the fact that these findings also contribute to the understanding of the specific mechanisms by which gut flora metabolites contribute to metabolic processes in humans. This review proposes an emerging role for SCFA in the inflammatory Mets, followed by the identification of major ambiguities to further understand the anti-inflammatory potential of this substance in Mets. In addition, this study proposes novel strategies to modulate SCFA for the treatment of Mets that may help to mitigate the prognosis of Mets and its complications.
Metabolic syndrome (Mets) is a group of clinical syndromes including abdominal obesity, hypertension, hyperlipidemia, hyperglycemia, and a series of risk factors for cardiovascular and cerebrovascular diseases, and its core mechanisms are disorders of glucose and lipid metabolism and insulin resistance. As a result of a complex interplay of genetic and lifestyle factors (1), mostly increased consumption of high-calorie, low-fiber fast food and reduced physical activity due to longer commutes and sedentary work–life habits, it is now a truly global problem, with prevalence rates in urban populations in some developing countries often higher than in Western countries, and with global prevalence rates estimated to be approximately a quarter of the world’s population (2). Previous studies have shown that Mets is an important risk factor for cerebrovascular diseases and chronic kidney diseases (3, 4). The study of Mets is important for human health issues.
In recent years, the role of intestinal flora in the human body has received more attention, and more research on SCFA has been conducted. Studies on the application of SCFA in Mets have gradually begun to appear in the public’s eye, and they have been found to improve the distribution of lipids, glucose, and body weight levels in animal models of Mets (5, 6). It is well known that Mets is a systemic chronic inflammatory disease, and SCFA can regulate immunity and inflammation, and the correlation between immunity and Mets has received more attention (7). However, the specific mechanisms are still unclear, and there is no summary report of anti-inflammatory and anti-immune mechanisms in the various complications of Mets. Recent advances in the regulation of immunity and inflammation by SCFA provide new insights into inflammation-related Mets. This review will discuss the effector pathways associated with SCFA in inflammatory Mets and its complications, the mechanisms by which SCFA ameliorates inflammation in Mets, and the treatment of the components of inflammatory Mets and its complications with SCFA.
This review followed the PRISMA guidelines. The primary search for article screening used in this review was conducted using PubMed (1,021), Web of Science (985), and Scopus (128) and the medical subject headings (short-chain fatty acid, inflammation, and metabolic syndrome). Using the PubMed database as an example, we present our search strategy: ((((((((((((Short Chain Fatty Acid[MeSH Terms]) OR (Short-Chain Fatty Acid[Title/Abstract])) OR (Volatile Fatty Acid[Title/Abstract])) AND (Inflammation[MeSH Terms])) OR (Innate Inflammatory Response[Title/Abstract])) AND (Metabolic Syndrome[MeSH Terms])) OR (Reaven Syndrome X[Title/Abstract])) OR (Metabolic Syndrome X[Title/Abstract])) OR (Insulin Resistance Syndrome X[Title/Abstract])) OR (Metabolic Cardiovascular Syndrome[Title/Abstract])) OR (Metabolic X Syndrome[Title/Abstract])) OR (Dysmetabolic Syndrome X[Title/Abstract])) OR (Cardiometabolic Syndrome[Title/Abstract]).
The literature screening was conducted collaboratively by two researchers. Following a rigorous selection process, 143 articles were included in our study. The PRISMA flowchart illustrates the process of identifying and screening articles related to the role of SCFA as an anti-inflammatory and anti-immune mechanism in the inflammatory metabolic syndrome (Figure 1).
Figure 1. Search strategy. The flowchart outlines the search and screening process for the study. The search was first performed in PubMed, Web of Science, and Scopus, then duplicate articles and articles that did not meet the research criteria were removed, and finally relevant articles were selected for further research.
High-fat diets and carbohydrate-rich diets are important triggers of Mets, which may weaken the adhesion of tight junction proteins in the gastrointestinal tract, resulting in leaky gut syndrome. Lipopolysaccharides (LPS) produced by harmful bacteria may infiltrate into the bloodstream through the portal vein, resulting in endotoxemia and inflammatory reactions, leading to inflammation of important organs related to metabolism, such as the liver and pancreas, as well as changes in the composition and number of intestinal flora. The metabolites produced by the altered intestinal microorganisms, such as SCFA and ethanol, may affect the bile acid and fat metabolism process in the liver, leading to the accumulation of fat, which, in turn, induces hepatic steatosis and triggers the development of Mets (8). In this process, immune imbalance plays an integral role in chronic inflammation, adipose tissue dysfunction, and gut flora disruption. Abdominal adipose tissue accumulation in Mets patients, especially visceral adipose tissue accumulation, exerts shear mechanical stress on the extracellular environment (9) and promotes intestinal uptake of antigenic substances therein, which, in turn, induces an inflammatory immune response, especially in tissues that are in close proximity to the intestinal tract, and this effect may be exacerbated by the abundance of lipids in a high-fat diet. Intestinal absorption of dietary fat promotes the uptake of LPS and protein antigens of intestinal bacterial origin (10, 11). Lack of immune tolerance to this antigen causes marked inflammatory responses in mesenteric adipose tissue, and a high-fat diet increases these inflammatory responses (12). Over time, these responses lead to reduced glucose tolerance. During diet-induced obesity, intestinal absorption of antigens is involved in T-cell activation and recruitment in visceral adipose tissue. The pro-inflammatory environment in visceral adipose may impair tolerance to these antigens by increasing free fatty acids and may lead to chronic inflammation. B lymphocytes are likewise recruited to adipose tissue after the onset of a high-fat diet, even before T cells (CD8+ T cells and TH1) are recruited (13). In the early stages of obesity, increased adipocytes release chemotactic adipokines and chemokines, such as CCL5, which help recruit pro-inflammatory cells of the adaptive immune system to adipose tissue (14). As obesity progresses, there is a progressive increase in the release of INF-γ and chemokines, such as CCL2, by CD8+ and TH1-type lymphocytes, which leads to the activation of NK cells and pro-inflammatory M1-type macrophages (e.g., Figure 2A). Activated M1-type macrophages infiltrate visceral adipose tissue and stimulate its release of large amounts of adipokines and chemotactic mediators such as tumor necrosis factor-α, interleukin-6, monocyte chemotactic protein-1, leptin, resistin, and vascular endothelial growth factor (15). Together, these factors induce a state of chronic, low-grade inflammation in the organism, which further contributes to insulin resistance and dyslipidemia, atherosclerosis, vascular dysfunction, and the development of nonalcoholic fatty liver disease (NAFLD) and Mets.
Figure 2. Inflammatory mechanisms of Mets and effector pathways of SCFA. The figure is drawn with Figdraw.com. (A) In Mets, hypertrophied adipose tissue disrupts intestinal barrier protection and promotes intestinal absorption of antigenic substances from the gut, which, in turn, induces an inflammatory immune response. (B) SCFA exerts its biological effects by inhibiting HDAC or activating GPCRs. LPS, lipopolysaccharides; OVA, ovalbumin; TG, triglycerides; NK, natural killer; TH, T-helper cell; Treg, T-regulatory cell; HDAC, histone deacetylase; GPCRs, G protein-coupled receptors.
It can be seen that immune and inflammatory responses lead to intestinal bacterial imbalance, and intestinal bacterial imbalance is more likely to exacerbate the inflammatory response and promote Mets. Therefore, immunity, inflammation, and intestinal bacterial disorders often affect each other, forming a vicious cycle, but we can improve the intestinal internal environment, regulate the composition and abundance of SCFA, enhance the intestinal barrier function, reduce the leakage of LPS, and at the same time exert its anti-inflammatory effect, which, in turn, reduces the emergence of inflammation.
Since the origin of all things, microorganisms have coexisted with human beings, as if the microbial community has also played an important role in the evolutionary process of human beings, and all aspects of human biological functions are affected by them, among which, the gastrointestinal tract is the place where the greatest number and variety of commensal microorganisms are found in the human body, especially in the colon (16). Moreover, the total abundance of intestinal microorganisms accounted for more than 90% of the total intestinal microorganisms, such as the phylum Thick-walled Bacteria and the phylum Mycobacterium, which are the dominant bacterial flora involved in the production of SCFA in the intestinal tract. It has been demonstrated that SCFA, as an important metabolite of the intestinal bacterial flora, has a significant role in the body’s immunity, metabolism, endocrinology, and signaling, and that SCFAs are an important communication substance on the intestinal-organ axis (17–19). SCFA, also known as volatile fatty acid, is a general term for organic fatty acids containing two to six carbon atoms, mainly including acetic acid, propionic acid, and butyric acid, and consists of the intestinal metabolites of dietary fiber and protein (20, 21), of which dietary fiber is the main source of SCFA that can be divided into soluble (such as gelatin and inulin) and insoluble dietary fibers (such as various forms of resistant starch) (22).
SCFAs are produced by various prebiotics through fermentation processes such as Wood-Ljungdahl, carbon dioxide fixation, and acetyl-S coenzyme A condensation processes (23, 24). Prebiotics include antidigestive oligosaccharides (oligofructose), dietary fibers (e.g., inulin, pectin, and arabinoxylan), and resistant starches from various plant sources. Bacterial species that utilize prebiotics express carbohydrate-active enzymes (CAZymes) that degrade dietary fiber and thus produce SCFA (25). DF-rich diets increase the expression levels of microbiome-encoded CAZymes (26). Thus, the level of SCFA production in the colon is highly dependent on the microbial composition as well as the type and amount of DF in the diet. In addition to branched-chain amino acids such as valine, leucine, and isoleucine, amino acids can also be metabolized by microorganisms to produce acetic, propionic, and butyric acids (27). For example, threonine can be metabolized to the three main SCFAs. Microbes that efficiently produce SCFA are generally considered beneficial and are enriched in the intestines of healthy hosts with diets containing adequate levels of DF (28, 29). Previous studies have known that SCFAs, particularly butyric acid, can have anti-inflammatory effects on immune cell function. However, some researchers have found that the truth is not so absolute; propionic acid and butyric acid also have pro-inflammatory effects. Depending on the type and dose of SCFA used, these compounds may not impede the immune response, and in fact may even be stimulatory, with the exception of specific cell types (30). Cavaglieri et al. (31) demonstrated that butyric acid inhibited the production of IFN-γ by activated rat lymphocytes, whereas acetic and propionic acids increased this cytokine’s release. Specific mechanisms of effect are described below.
Approximately 95% of SCFAs produced in the intestine are transported to intestinal epithelial cells by ion exchange, monocarboxylic acid transporter, or cell gap diffusion. Some of them are metabolized by intestinal cells to maintain intestinal homeostasis, while the other part is transported to various tissues and organs to play biological roles by inhibiting histone deacetylase (HDAC) or activating G protein-coupled receptors (GPCRs) (21) (e.g., Figure 2B) (32). The GPCRs such as GPR41, GPR43, and GPR109A have been found to be expressed on intestinal epithelial cells, adipose tissue, and immune cells including neutrophils, dendritic cells, macrophages, and lymphocytes. The expression of these GPCRs also varies in different tissues. A recent review has summarized the role of different receptors of SCFA in immune cells (33). Activation of these GPCRs can inhibit cAMP-dependent signaling pathways, activate the mTOR signaling pathway, and inhibit the NF-κB signaling pathway to reduce the inflammatory response (34, 35). NF-κB belongs to a family of nuclear transcription factors, including p50, p52, REL, REL-a, and REL-B, which is the primary response to noxious cellular stimuli. Several downstream mediators of the NF-κB pathway have been identified: tumor necrosis factor-α (TNF-α), interleukin (IL)-1, IL-2, IL-6, IL-8, IL-12, iNOS, COX2, chemokines, adhesion molecules, and colony-stimulating factors (36–38).
GPR41 is present in a wide range of tissues, whereas GPR43 is mainly expressed in lymphoid tissues and a variety of immune cells. Both GPR41 and GPR43 bind acetic, propionic, and butyric acids, whereas GPR109A is predominantly activated by butyric acid. The activation of GPR43 by SCFA can produce different effects according to different cell types. For example, it induces Nod-like receptor protein 3 (NLRP3) inflammatory vesicle activation and IL-18 secretion in colonic epithelial cells, promotes neutrophil recruitment to sites of inflammation, and enhances the differentiation and suppressive function of FOXP3+ regulatory T (regulatory T, Treg) cells (39). SCFAs (butyric and propionic acids) also exert their ability to modulate inflammatory and immune responses by inhibiting HDAC activity. When SCFAs enter cells by passive diffusion or through transmembrane vectors (e.g., SMCT1 and MCT1), they can directly bind to intracellular HDAC and inhibit its activity, which, in turn, inhibits the activation of the nuclear factor-κB (NF-κB). Emerging evidence suggests that butyric acid enhances p65 acetylation by inhibiting HDAC3 and HDAC6, also altering the lysine acetylation of non-histone proteins such as NF-κB subunit p65, leading to differential recruitment of NF-κB to pro-inflammatory gene promoters in vitro and in vivo (40). In addition, SCFAs, particularly butyric acid, have recently been found to be activators of intracellular receptors that control immune responses, such as peroxisome proliferator-activated receptor-γ (PPARγ) (41) and the aromatic hydrocarbon receptor (42). Additionally, butyric acid can provide acetyl groups for histone acetylation (43). Butyrate affects gene expression in intestinal epithelial cells, macrophages, dendritic cells, and lymphocytes (especially Treg cells) by increasing histone acetylation and increasing the amount of open chromatin (44–46). Studies have observed that the effects of butyrate on immune and nonimmune cells result in alterations in pathways related to the cell cycle, cellular differentiation, antimicrobial responses, inflammation, fatty acid metabolism, and oxidative stress-related alterations in various pathways (46–48).
Overall, the diverse molecular targets of SCFAs illustrate that different SCFAs in different tissues can target immune and non-immune cells, modulate immunity in the gut and distal organs, and potentially protect against immune-mediated diseases.
Gut microbes play a key role in maintaining physiological functions as they regulate host nutrition, energy harvesting, epithelial homeostasis, immune system, and drug metabolism while maintaining homeostasis (49). If the gut microbes are disturbed, the pre-existing homeostasis in the organism is disrupted and oxidative stress, mitochondrial dysfunction, epigenetic alterations, and, ultimately, Mets, occur (e.g., Figure 3).
Figure 3. Mechanisms associated with SCFA ameliorating Mets by modulating oxidative stress, mitochondrial dysfunction, and epigenetic alterations. The figure is drawn with Figdraw.com. ROS, reactive oxygen species.
Reactive oxygen species (ROS), including hydroxyl radicals, superoxide anion, and hydrogen peroxide, which are formed during the one-electron reduction of molecular oxygen, regulate a number of signaling pathways in the intestine and are considered to be central regulators of intestinal stem cell functions (50). Overproduction of ROS can occur in obesity, insulin resistance, hyperglycemia, chronic inflammation, dyslipidemia, and other pathologic diseases (51). Many studies have found that patients with Mets have lower plasma antioxidant enzyme activity and more biomarkers of oxidative damage compared to healthy individuals, which may contribute to oxidative stress (52). The overproduction of ROS leads to an oxidative stress environment, which also disrupts redox signaling and control, leading to an increase in growth factors and stress response components and activation of apoptotic pathways (53, 54). Disrupted redox signaling also promotes pro-inflammatory and pro-fibrotic pathways, affecting insulin metabolic signaling and endothelial dysfunction, and promoting cardiovascular and renal inflammation and fibrosis (54, 55), which can lead to target organ damage and the emergence of major components of Mets, such as hyperglycemia and hypoglycemia.
The direct and indirect mechanisms by which SCFAs regulate oxidative stress are through activation of the Keap1-Nrf2 cell signaling pathway (56, 57). In terms of the direct mechanism, SCFAs bind to the GPCR receptor to induce the direct activation of nuclear factor Nrf2 (58), and butyrate induces the activation of nuclear factor Nrf2 by recognizing the GPR109A receptor, which encodes an antioxidant enzyme that inactivates ROS (59). On the other hand, butyrate has a synergistic effect on Nrf2 activation because it diffuses into the cell lumen and indirectly activates Nrf2-dependent gene translocation and transcription by inhibiting HDAC and increasing the production of histone H3K9ac, which induces epigenetic modification of the Nrf2 promoter (56, 57, 60, 61). However, different types of SCFAs have different effects on ROS, and it has been shown that butyrate, propionate, and acetate treatments have reduced, no, or increased effects on ROS production in rat neutrophils (62). Among them, for acetate, which promotes the release of ROS from mouse neutrophils through the activation of GPR43 (63), the more plausible explanation for this phenomenon is the view of some researchers in the field of immunology, who believe that SCFAs may modulate inflammatory diseases by accelerating pathogen clearance through the activation of ROS (24). In fact, butyric acid does induce apoptosis or inhibit cell proliferation by increasing ROS levels, which, in turn, inhibits cancer progression (64, 65). The exact mechanism of SCFA and ROS needs to be continued to be explored by researchers.
Deletion of HDAC Sirtuin-1, an important target of SCFA, affects Mets as a result of activation of the phosphatidylinositol 3 kinase (PI3K)–mammalian target of rapamycin (mTOR) signaling pathway (66, 67). Activation of sirtuins and reduction of oxidative stress through the use of resveratrol are thought to prevent chronic inflammation (68), but paradoxically, the findings of oral resveratrol for improvement of glucose homeostasis and cardiovascular characteristics are divergent (69), but the feces of mice transplanted and fed with resveratrol were better than those of oral administration (70), which may be related to the route of administration, which reinforces the role of enterobacteria in the amelioration of inflammation-associated Mets. Recent studies have shown that seaweed cellulose (71), seaweed polysaccharides (72), acetate (73, 74), fermented sea buckthorn juice (75), and dry-cured ham (76) inhibit oxidative stress and therefore have great potential in controlling Mets as providing new perspectives in the prevention and treatment of Mets.
Mitochondria are the main site of biological oxidation and the center of energy metabolism in the organism. At the same time, mitochondria are also highly dynamic organelles that are remodeled through biosynthesis, division and fusion, autophagy, and other processes to achieve mitochondrial homeostasis (77). Mitochondrial dysfunction occurs when mtDNA mutations, kinetic imbalances, and oxidative stress occur in mitochondria. Mitochondrial dysfunction and subsequent excess ROS production promotes insulin resistance through activation of the c-Jun amino-terminal kinase (JNK) and NLRP3 inflammatory vesicles, leading to the inactivation of the insulin receptor substrate (IRS)1/PI3K/serine/threonine kinase (Akt) pathway, which promotes insulin resistance progression (78). The main pathogenic mechanism of Mets is closely related to insulin resistance, and the study of mitochondrial dysfunction is undoubtedly an important target for attacking Mets.
It has been shown that SCFAs such as propionate and butyrate enhance mitochondrial biogenesis (79). SCFA supplementation may prevent colonic inflammation and dysregulated mitochondrial energy metabolism by improving the balance between Treg cells and Th17 cells, increasing mitochondrial ETC activity and oxidative phosphorylation (80), but there are very few studies on SCFA and mitochondrial autophagy, among others. It has been well documented that mitochondrial dysfunction is associated with Mets and that abnormal mitochondrial autophagy leads to impaired mitochondrial function and is involved in the development and progression of Mets (81, 82). It has been demonstrated by flow cytometry analysis that propionate induced Δψ loss, leading to aberrant mitochondrial autophagy (83), and that butyrate restored PRKN expression by blocking RELA nuclear translocation and directly inhibiting HDAC8 in the nucleus, which, in turn, ameliorated mitochondrial autophagy in high-glucose-inhibited neuronal cells (84). Mitochondrial autophagy has many cellular pathways in Mets, such as the classical PINK1/Parkin pathway, the mitochondrial autophagy receptor FUNDC1, and the BINP3/NIX pathway, which are closely associated with Mets-associated metabolic disorders, such as type 2 diabetes (85), obesity (86), heart disease (87), and NAFLD (88), respectively. However, two important proteins related to the regulation of mitochondrial autophagy in glucolipid metabolism are currently underappreciated in the context of Mets. FK506-binding protein 8 (FKBP8), a mitochondrial autophagy-recognizing protein, mediates mitochondrial autophagy and fragmentation (89), which dynamically regulates pancreatic islet β-cells and glucose-stimulated insulin secretion (90). Theoretically, FKBP8 is important in the development of insulin resistance or T2DM. However, there are fewer studies on FKBP8. Similarly, BCL-2-like protein 13 (BCL2L13) is a mitochondrial outer membrane protein involved in mitochondrial division in mammalian cells; BCL2L13 is important in the control of apoptosis, mitochondrial fracture, and the promotion of mitochondrial autophagy (91); BCL2L13 can play a role in the promotion of adipogenesis by regulating mitochondrial autophagy process to maintain mitochondrial quality control (92, 93). BCL2L13 may be a promising biomarker as a potential drug therapeutic target for Mets. However, no relevant studies have emerged with Mets.
Since the common aggregation of obesity cannot be explained by common environmental conditions alone, the heritability of obesity is widely believed to be between 40% and 70%. However, the risk loci associated with BMI revealed in genome-wide association studies can only explain approximately 16% of heritability, even after taking into account their superimposed effects (94). As a result, epigenetic mechanisms have become the focus of obesity research in the past few years to explain inherited deletions. Epigenetic mechanisms describe processes that affect the DNA surrounding the transcription of a gene without changes in the DNA sequence itself. Examples include adding methyl groups to cytosine and then adding guanosine (CpG site) to the sequence. Other mechanisms alter the structure of chromatin, for example, through modifications such as methylation, acetylation, or phosphorylation of histones. Several studies have observed differences in methylation levels at different CpG sites between obese and lean individuals and after weight loss interventions (95–97).
SCFAs achieve epigenetic regulation through HDAC inhibition, increase mitochondrial β-oxidation, and prevent high-fat diet-induced insulin resistance, thereby improving glucose sensitivity and obesity. Epigenetic mechanisms have also been shown to play an important role in the regulation of genes involved in inflammatory processes and have been closely linked to SCFA-producing bacteria and metabolic diseases. SCFAs are thought to influence inflammation and chronic diseases (98), and butyric acid can regulate gene expression by inhibiting HDACs, which, in turn, prevents HDACs from suppressing the expansion of anti-inflammatory Treg cells. Butyrate and, to a lesser extent, propionate induced the differentiation of colonic Treg cells to suppress inflammatory and allergic responses in the gut (44). GPR43 and GPR41 also triggered the inhibition of HDAC 1 (99), which enhanced the apoptosis of activated T cells, thus possessing anti-inflammatory potential. It has been shown that mice lacking Toll-like receptor 4 (TLR4) or under antibiotic treatment display reduced LPS and are protected from systemic lipid infusion and alleviate Mets (100). Surprisingly the inhibition of TLR4 gene transcription can be mediated by DNA methylation and histone deacetylation (101), which are both associated with epigenetic mechanisms and have not been linked to SCFA by any investigator, which is a good therapeutic target.
After SCFA is absorbed by the intestine, part of it is utilized locally as fuel for intestinal epithelial cells, and the other part enters the portal vein to enter the blood circulation, which improves Mets-associated disorders of glucose and lipid metabolism as well as cardiovascular, reproductive, and neurological complications by exerting anti-inflammatory effects (e.g., Table 1, Figure 4).
Figure 4. SCFAs exert anti-inflammatory effects in components of Mets. The figure is drawn with Figdraw.com. LPS, lipopolysaccharides; OVA, ovalbumin; TG, triglycerides; NK, natural killer; TH, T-helper cell; Treg, T-regulatory cell.
Type 2 diabetes is strongly associated with insulin resistance. The potential mechanisms of intestinal microbial translocation to the pancreas are unknown, and one possibility that has been suggested is via the pancreatic ducts (102), while another may be via the portal vein via the bloodstream from the distal gastrointestinal tract (103). Translocation of flora triggers innate and adaptive immune responses and induces inflammation in colonized tissues or organs; interestingly, translocation of Enterococcus and Escherichia coli leads to acute pancreatitis (104). Inflammatory diseases, such as inflammatory bowel disease (IBD), are known to be associated with higher intestinal permeability (105), and increased intestinal permeability can also lead to increased commensal bacterial translocation (106). There is a large body of research showing that patients with IBD have a higher risk of developing a number of pancreatic diseases, including type 2 diabetes (107), pancreatitis (108), and pancreatic cancer (109). In terms of metabolic diseases, a national cohort study from Denmark showed that patients with IBD are at a higher risk of developing type 2 diabetes (110), suggesting that intestinal inflammation is strongly associated with insulin resistance. Notably, it was found that mutations in autoimmune-related genes overlap between IBD and type 1 diabetes (T1D), such as protein tyrosine phosphatase non-receptor type 2 (PTPN2) and PTPN22, which negatively regulate T-cell activation (111) and alter the composition of the gut microbiota in IBD patients (112). This suggests that the association between T1D and IBD may involve immune dysfunction and increases the likelihood of overlapping genes between IBD and diabetes.
SCFAs improve insulin sensitivity through multiple pathways. In terms of inflammation, SCFA can inhibit intestinal and systemic inflammatory responses and reduce the release of pro-inflammatory factors, thereby improving the mechanism of insulin action (113, 114). For example, SCFA can enhance the barrier function of intestinal epithelial cells, reduce intestinal permeability, and prevent endotoxin from entering the circulation (115). In addition, acetate, propionate, and butyrate also appear to regulate hepatic glucose metabolism through activation of AMP-activated protein kinase (AMPK) and promotion of PPARγ on gluconeogenesis (116).
Although clinical studies have demonstrated a relationship between SCFA and insulin resistance, these results of clinical interventions have been variable. Several systematic evaluations and meta-analyses suggest that increased intake of SCFA may be beneficial in reducing fasting insulin levels and improving insulin sensitivity (117, 118). However, many trials suffered from poorly considered experimental designs, and future studies need to pay attention to assessing several factors, such as the different types of SCFA (acetic acid, propionic acid, butyric acid, or mixtures thereof), the form of SCFA administration (fiber-rich diets or capsules containing sodium salts or inulin esters), the duration of the intervention, the route of administration (oral or intravenous infusion), and patient adherence.
SCFAs can also play a role in islet cytoprotection, and butyrate treatment of non-obese diabetic mice reduced the proportion of inflammatory/regulatory macrophages through the SHIP-1/PI3K pathway, which suppressed the frequency of diabetogenic IFN-γ+CD8+ T cells in the pancreas and reduced diabetes incidence (119). The addition of SCFAs (especially butyrate) to islet cell culture dishes increased the production of the antimicrobial peptide CRAMP by islet β-cells (119), but the exact mechanism involved in the regulation of the antimicrobial peptide CRAMP through which mechanism, GPCRs or HDAC, is not known and needs to be further investigated.
Aseptic inflammation promoted by a high-calorie or high-fat diet can disrupt the gut microbiota and lead to metabolic endotoxemia, which can trigger activation of innate immunity (120). A growing body of evidence indicates that a specific group of bacterial species are associated with obesity and related metabolic defects such as insulin sensitivity by regulating lipid metabolism and systemic inflammatory responses (121–123). Whereas SCFAs play an important role in lipid metabolism, SCFAs not only participate in lipid metabolism as substrates, but also act as regulators to modulate lipid metabolism. As a member of the fatty acid family, SCFAs provide substrates for lipid synthesis. SCFAs can be converted to acetyl coenzyme A to generate energy through the tricarboxylic acid cycle (124). In addition, acetate, as a precursor for the synthesis of palmitic and stearic acids, in turn promotes hepatic fatty acid metabolism (125). At the same time, acetyl coenzyme A converted by SCFAs can also generate palmitic acid through the action of the cytoplasmic enzyme system, and palmitic acid can be transferred to the mitochondria to promote the formation of triglycerides and be stored in adipose tissue (126). Li showed that butyric acid increased fatty acid oxidation in brown adipose tissue and improved diet-induced obesity and insulin resistance (127). Butyric acid also promotes white tissue browning, morphologically reduces adipocyte size, and increases the number of multicellular adipocytes (126).
In specific inflammation-related mechanisms, the AMPK signaling pathway is involved in lipid metabolism and increases PGC-1α expression in adipose tissue and skeletal muscle (128), and chronic AMPK activation via loss of FLCN induces functional beige adipose tissue through PGC-1α/ERRα, as PGC-1α regulates the transcriptional activity of various transcription factors, including PPARα and PPARγ (129). Furthermore, it has been shown that activation of the AMPK signaling pathway promotes the expression of hormone-sensitive lipase (HSL) and adipose triglyceride lipase (ATGL) as the main enzymes of lipolysis, and promotes lipolysis (130, 131).However, there are findings that are controversial with them, and one study found that SCFAs can reduce protein kinase A (PKA) activity by inhibiting adenylate cyclase (132), which, in turn, leads to dephosphorylation and inactivation of HSL in adipose tissue (133). Similarly, Jocken et al. found that acetate exerts anti-lipolytic effects by inhibiting the phosphorylation activity of HSL in human pluripotent adipose tissue-derived stem cells (134). Surprisingly, however, it was recently found that activation of AMPK in murine hepatocellular carcinoma cells inhibits hepatic fatty acid synthesis via suppression of sterol regulatory element-binding protein 1C (SREBP-1C), a major regulator of hepatic adipogenic gene expression (135), which is a potential therapeutic target.
Hypertension is one of the important components of Mets, and hypertensive patients have higher levels of IL-6, IL-8, and TNF-α in the blood (136). The systemic inflammatory state adversely affects the body’s RAAS system and the vascular endothelial system, leading to elevated blood pressure, which increases intestinal inflammation and permeability in patients (137); it can affect intestinal physiology and decrease the abundance and diversity of intestinal flora, thus decreasing the concentration of SCFAs. SCFAs exert their regulatory functions mainly through the inhibition of HDACs and the activation of Gpr43 and Gpr109a, and intervene in the key factors of kidney and brain through the mechanism of renal–intestinal axis and brain–intestinal axis, which not only control inflammation to regulate blood pressure, but also influence metabolism and immunity to regulate blood pressure. Specifically, in terms of immuno-inflammation, SCFA can achieve the occurrence and development of hypertension by regulating effector T cells, helper T cells (Th), and regulatory T cells (Treg).
In terms of effector T cells, in the active immune response state, SCFAs promote effector T-cell generation and, at the same time, can increase the cytotoxicity of CD8+ T cells and the ability to generate IL-17, whereas in the physiological state, SCFA promotes the body’s immune tolerance to effector T cells through the increase in the generation of IL-10, which, in turn, can have an inhibitory effect on chronic inflammatory responses in hypertension (138). In terms of Th, infusion of Ang II into the spleens of wild-type mice increased the level of CD4+ effector memory T cells (CD44+CD62−) and decreased the level of CD4+ initial T cells (CD44−CD62+), resulting in the release of the inflammatory factors IL-17a and IFN-γ from Th17 and Th1, respectively. This, in turn, promotes the development of hypertension and its target organ damage, which is reversed by propionate supplementation (139). This suggests that propionate can ameliorate the inflammatory response by inhibiting the increase in the number of effector T cells and Th17 cells, thereby decreasing IL-17a secretion. With regard to Treg, SCFA increases histone acetylation by inhibiting HDAC, which can differentiate primitive T cells into Treg and increase Foxp3 expression via Gpr43, thereby increasing Fox P3+ T-cell activity and IL-10 production (140), producing an anti-inflammatory effect and thus alleviating the progression of hypertension (139). It has also been suggested that its antihypertensive properties may be due to the binding of SCFA to Gpr43 in the lamina propria of intestinal epithelial cells, resulting in the polarization of T cells and their conversion to Treg, which then migrate and accumulate in the renal cortex (141). In addition, the proportion of methylated regions in genes related to Treg function increased to different degrees, and supplementation with acetate altered the level of DNA methylation in Treg-activated regions, suggesting that SCFA regulates the methylation of functional genes in T cells and thus activates Treg. However, Gill (142) found that short-term increases in systemic acetate and propionate levels did not alter Treg levels in mice, suggesting that the concentration of SCFA has a strong influence on the outcome of their effects in immunization. However, different types of SCFA have different effects on blood pressure, and propionate may have a better effect on blood pressure control compared with butyrate. In spontaneously hypertensive rats, butyrate treatment did not affect intraocular pressure and caused only a transient decrease in blood pressure (143). In contrast, propionate significantly reduced systolic and diastolic blood pressure in angiotensin (Ang) II mice, ameliorating systemic inflammation, cardiac damage, and vascular dysfunction (144).
Obesity-induced systemic inflammation can contribute to the development of a number of non-communicable chronic diseases, such as type 2 diabetes, chronic liver disease, arthritis, and certain types of cancer (145). In obesity, two main mechanisms are involved in systemic inflammation. First, inflammation is driven by adipose tissue macrophages (ATMs). Significant enlargement of adipose tissue due to excess energy intake stimulates macrophage polarization from the anti-inflammatory M2 type to the pro-inflammatory M1 type (146). M1-type macrophages cause further inflammation by producing pro-inflammatory cytokines such as TNF-α and IL-6 (147). In addition, the inability of M1-type macrophages to buffer lipids leads to lipid spillover into the circulation and activation of pro-inflammatory cascades (147). Second, systemic inflammation in obesity can also be caused by metabolic endotoxemia, a condition characterized by elevated levels of circulating endotoxins, including LPS. In obesity, increased intestinal permeability due to dysbiosis of the intestinal flora affects the tight junction proteins in the intestinal lumen, which, in turn, leads to infiltration of LPS into the blood circulation (148). Elevated metabolic danger signals, free fatty acids (FFAs), and the bacterial danger signal, LPS, activate the NF-κB and MAPK pro-inflammatory pathways, which are regulators of systemic inflammation in obesity, by binding to TLRs (149), resulting in the transcription of pro-inflammatory cytokines, such as TNF-α, IL-6, and IL-1β, which initiate systemic inflammation (150).
To date, one of the most effective treatments for obese chronic low-grade systemic inflammation is weight loss (151). Bariatric surgery results in significant weight loss, leading to a significant reduction in the systemic inflammatory response (152), with significant reductions in the pro-inflammatory cytokines CRP, TNF-α, and IL-6 (153). SCFAs are a non-invasive, novel, alternative treatment for systemic inflammation in obesity compared to the invasive treatment of bariatric surgery. Whereas SCFAs can inhibit LPS-induced inflammation (154), in addition to that, SCFAs have many anti-inflammatory mechanisms (155, 156). Treating SCFA modulates the expression of FFAR and HDAC genes involved in key inflammatory pathways in monocytes and ATMs of obese subjects. First, SCFAs play an important role in obesity systemic inflammation by regulating FFARs. Their key targets are free fatty acid receptor (FFAR) 2, which is mainly expressed in immune cells, and FFAR3, which is mainly expressed in pancreas, spleen, and adipose tissue, both of which are associated with metabolic diseases such as obesity and T2DM (156). FFAR2 and FFAR3 are upregulated by LPS stimulation in monocytes and macrophages (157). Second, SCFAs can inhibit HDACs, leading to downregulation of NF-κB and MAPK pro-inflammatory pathways (156). Jeong found that inhibition of HDAC1–3 reduced LPS-induced phosphorylation of p38MAPK, which, in turn, reduced LPS-induced expression of TNF-α and IL-1 β (158). Propionate- and butyrate-mediated inhibition of HDACs in SCFAs plays an important role in the downregulation of the MAPK pro-inflammatory pathway. The SCFAs butyrate and propionate interact predominantly with HDACs 1 and 2, which are located in the nucleus, in addition to HDACs 3, 4, 5, 6, 7, and 9, which are shuttled between the cytoplasm and the nucleus (159). Butyric and propionic acids, considered to be the most potent HDAC inhibitors, have previously been shown to inhibit TNF-α production and NF-κB activity, whereas evidence suggests that acetic acid has little ability to inhibit HDAC activity (45, 160, 161).
The type and concentration of SCFA also affect the concentration of inflammatory factors in obese patients, and several studies have shown that butyrate, propionate, and acetate all significantly reduced TNF-α and IL-6 production in LPS-stimulated monocytes from obese subjects. Only the degree of inflammatory factors reduced by different SCFA species varied, with acetate and butyrate significantly reducing TNF-α production by LPS-stimulated ATMs, and propionate and butyrate significantly reducing IL-6 (162, 163). However, Cox showed that SCFAs (0.2–20 mmol/L) were effective in reducing LPS-induced TNF-α in PBMCs, but not in human monocytes. Few studies have measured the effect of SCFAs on obese ATMs. Al-Lahham showed that 3 mM propionic acid significantly reduced LPS-stimulated TNF-α responses in ATMs (164). However, some studies did not observe an inhibitory effect of propionate on TNF-α production, which may be related to the concentration of propionate (163). Since ATMs are less responsive to LPS stimulation than monocytes and adipose tissue is difficult to obtain, there are few studies related to the effect of SCFAs on inflammation in human ATMs, but this study is related to the important mechanism of chronic inflammation in obesity, so attention should be paid to the techniques of adipose tissue extraction and the effect of SCFAs on inflammation in ATMs.
The liver is involved in fat synthesis and is therefore closely related to Mets. The intestine and liver are closely connected through the portal vein and the biliary system. Enteric substances such as digested food fragments (amino acids, lipid fragments, and monosaccharides), intestinal microbial products, and exogenous toxins enter the liver via the portal vein (165). Animal studies have also demonstrated that during high-fat diet-induced NAFLD, there is a gradual increase in LPS levels and changes in the composition of the intestinal flora (166) and that the exacerbation of NAFLD to NASH is also associated with an increase in LPS levels (166). It can be seen that rapidly proliferating bacteria and their metabolites enter the portal vein through the damaged intestinal wall, and the inflammatory response caused by the level of LPS, which activates the cascade immune response and causes hepatic inflammation, is the key to the occurrence as well as further deterioration of NAFLD. Intestinal SCFAs can reach the liver through the hepatic portal vein. In vivo and in vitro studies have found that the anti-inflammatory effects of butyrate on the liver are mainly mediated through Kupffer cells. In vivo in rats, butyric acid infusion through the portal vein enhances the production of the immunosuppressive arachidonic acid metabolite, prostaglandin E2 (PGE2), by Kupffer cells. In vitro, butyric acid supplementation of Kupffer cells also increased PGE2 production and inhibited TNF secretion (167).
Two inflammatory mechanisms can be found in NAFLD mouse models, both of which can be mediated by microbial components such as LPS, and they can be transferred from the intestinal lumen to the liver through the portal vein. One mechanism is that they are recognized by pattern recognition receptors such as TLRs in the liver, e.g., TLR4, leading to hepatic inflammation, hepatocellular injury, and liver fibrosis (168). Similar pathways have been suggested to contribute to the development of NAFLD. Activation of inflammatory vesicles, especially the NLRP3 inflammatory vesicle, is thought to be another trigger of the hepatic inflammatory response to LPS. Activation of NLRP3 in the liver leads to activation of caspase 1 and production of IL-1β and several other inflammatory cytokines, ultimately leading to programmed cell death, inflammation, and fibrosis. NLRP3 was significantly upregulated in the livers of NASH patients compared with steatosis alone (169), and hepatic inflammatory vesicle fractions correlated with ALD activity (170). On the other hand, NLRP6 and NLRP3 inflammatory vesicle defects regulate the gut microbiota, leading to gut barrier dysfunction and exacerbating steatohepatitis in mice (171).
SCFAs may exert an anti-inflammatory response through activation of GPR41/43 and AMPK and inhibition of HDACs, which is beneficial for the treatment of NAFLD (172). In rodents, enteral administration of acetate and butyrate has been shown to induce the expression of beta-defensin and histone inhibitor-related antimicrobial peptides in intestinal epithelial cells in a GPR43-mediated manner as a means of protecting the intestinal barrier and organizing the inflammatory response induced by, among others, LPS (173). Activation of GPR43 on adipocytes inhibits lipolysis and reduces plasma free fatty acid levels in the binding mechanism of SCFAs to the GPRs (174). Similarly, GRP41/43 knockout mice exhibit a smaller trend toward obesity (175, 176).
In addition to the immuno-inflammatory mechanisms associated with binding to GPRs, SCFAs enter the liver directly through the portal vein and promote triglyceride synthesis (from acetic acid) and gluconeogenesis (from propionic acid), which have been implicated in the development of NAFLD (177). Rau et al. (178) found that patients with NAFLD had a significantly higher abundance of SCFA-producing bacteria in their feces and that higher levels of fecal SCFAs (acetate and propionate) were associated with higher Th17/Treg cell ratios in the peripheral blood, convincingly suggesting that the gut microbiome may modulate the immune response and contribute to disease progression through the production of greater amounts of SCFAs. Compared to healthy human controls, patients with NAFLD and NASH (179) had increased concentrations of SCFAs in their feces, as well as an increased abundance of bacterial flora involved in their production, and the immune cell profiles of both were altered. In detail, this increased fecal SCFA observed in NASH was associated with a decreased number of resting Treg cells (CD4+CD45RA+CD25+) and a higher ratio of Th17 cells to resting Treg cells in the peripheral blood, which is a systemic immune feature observed in NASH (180).
SCFA also prevents and ameliorates NAFLD by protecting hepatocytes, and studies suggest that butyric acid has two mechanisms of action for PPAR receptors to protect hepatocytes. On the one hand, butyric acid prevents the decreased expression of PPAR-γ in the liver, which promotes fatty acid uptake, increases insulin sensitivity (181), and decreases the expression of pro-inflammatory cytokine gene (IL-1β and TNF-α) macrophage infiltration-specific markers F4/80, which leads to a significant reduction of F4/80+ cell infiltration and anti-inflammatory cytokines in the liver of mice (IL-4 and IL-10) and activation of hepatic Kupffer cells, thereby preventing liver injury and inflammation in NAFLD mice (182). On the other hand, butyric acid acts as an HDAC inhibitor, upregulates PPAR-α expression, and promotes the binding of the NF-κB classical subunit p65 by increasing the acetylation of H3K9Ac on the PPAR-α promoter, thereby inhibiting the inflammatory response (183). In addition, the finding that SCFAs can reduce the expression of hepatic genes involved in NAFLD, mainly lipogenic genes such as those encoding acetyl coenzyme A carboxylase, fatty acid synthetase, and sterol regulatory element-binding protein 1c (cholesterol regulatory element-binding protein-1c (184)) through the action of HDAC inhibitors, provides important mechanistic insights. The concern, however, is that butyric acid is strongly affected by antibiotics, and it has been shown that butyric acid levels are significantly reduced in the liver of mice treated with antibiotics early in life, leading to impaired IL-18 signaling, which, in turn, inhibits mitochondrial function and maturation of liver-resident natural killer (NK) cells, whereas ingesting dietary butyric acid can, in a GPR109A-dependent manner, stimulate IL-18 production in Kupffer cells and hepatocytes to reverse this process (185). However, the mechanisms involved are unclear and need to be continued to be explored by researchers.
Atherosclerosis (AS) is a complex disease with multiple etiologies, and one of the factors contributing to AS is metabolic disorders. AS is an inflammatory disease that is associated with chronic vascular inflammation, and its most common pathologic process leads to cardiovascular disease. The earliest event in AS is increased adhesion of monocytes to endothelial cells, which is mainly regulated by vascular inflammatory factors, including cytokines such as IL-6, chemokines such as IL-8 and monocyte chemotactic protein-1, as well as endothelial adhesion molecules such as vascular cell adhesion molecule-1 and intracellular adhesion molecule-1. In clinical studies, less endothelial activation and less low-grade inflammation have been associated with high fiber depletion, possibly due to the production of SCFA. SCFAs have recently emerged as important signaling molecules that regulate a variety of responses in the cardiovascular system (186), and SCFAs have been shown to play a beneficial role in decreasing endothelial activation, resulting in decreased cytokine production and adhesion molecule expression (187). It may regulate endothelial function by inhibiting HDAC and/or activating GPRs (160).
SCFAs also play an important role in immune system regulation in modulating cardiovascular disease. Notably, it has been shown that both HDAC and NF-κB contribute to immune and inflammatory responses (188), whereas butyrate inhibits the activation of HDAC and NF-κB in macrophages (189). SCFAs are also involved in anti-inflammatory responses by upregulating anti-inflammatory cytokines and downregulating pro-inflammatory cytokines. For example, binding of SCFAs to FFAR2 and GPR109A in intestinal epithelial cells stimulates K+ efflux and hyperpolarization, leading to activation of the inflammatory vesicle-activating protein NLRP3, which, in turn, induces the release of IL-18, contributing to the maintenance of integrity, repair, and intestinal homeostasis (190). Butyrate increases protein acetylation and TGF-β production in the intestinal epithelial cells, leading to a decrease in IL-8 production (191) and an increase in anti-inflammatory Treg cells in the intestinal epithelial cells, respectively (192). In human mature dendritic cells, butyric and propionic acids appeared to reduce the release of pro-inflammatory chemokines such as CXCL11, CXCL10, CXCL9, CCL5, CCL4, and CCL3, as did inhibiting the expression of LPS-induced cytokines, including IL-6 and IL12p40. In addition to the modulation of cytokine production, SCFAs inhibited, by lowering the luminal pH, the growth of pathogenic bacteria (193). Finally, SCFAs, especially butyrate, can contribute to host defense by inducing the antimicrobial protein histone inhibitor IL-37 (194) and increasing the levels of T regulatory cells in the gut (44). In summary, we can infer that SCFAs can exert benefits in metabolic cardiovascular disease characterized by dysregulation of blood pressure, glucolipid metabolism, inflammatory response, and/or gut barrier integrity. Indeed, several studies have demonstrated the benefits exerted by SCFAs in metabolic cardiovascular disease.
It is worth mentioning that recent studies on SCFA for polycystic ovary syndrome (PCOS) in relation to inflammation in the heart have become progressively more frequent, and it has been found that acetate can reverse cardiac energy depletion, alleviate nitric oxide (NO/eNOS) deficiency, elevate SIRT-1/HIF-1α levels, and decrease CTGF/TGFβ-1 in an experimental PCOS model by inhibiting HDAC2, oxidative stress (malondialdehyde)/inflammation (NF-κB/SDF-1) markers, and plasma troponin T levels (195). Acetate has also been found to prevent cardiac inflammation in a rat model of PCOS by inhibiting PCSK9 and NF-κB-dependent mechanisms (196).
Mets and major depressive disorder are two of the most serious disorders worldwide, often reported to have a high comorbidity rate, and studies have demonstrated a strong correlation with inflammation (197). In addition to influencing material metabolism, the gut microbiota affects the nervous system by regulating the hypothalamic–pituitary–adrenal axis and producing neuroactive substances (198). The interaction between the central nervous system (CNS) and the gut flora is known as the flora–gut–brain axis. A growing body of research suggests a complex interaction between the microbe–gut–brain axis and psychiatric disorders such as anxiety (199), obsessive–compulsive disorder (200), and depression (201). One study found that blood levels of cytokines, including IL-6 and TNF-α, were significantly higher in depressed patients than in healthy controls (202), and that these pro-inflammatory cytokines, especially IL-6, IL-1β, and TNF-α, promote the production of Th17, which has been implicated in depression and other CNS disorders closely associated with them (203). The products of Th17 cells, IFN-γ, and IL-17A contribute to microglia proliferation, polarization, and activation (204, 205), which could promote neuroinflammation, whereas microglia are the main source of cytokines in all glial cells in the CNS (206). Microglia are polarized to an M1 phenotype, releasing ROS and pro-inflammatory cytokines, including IL-1 β, IL-6, and TNF-α (207).
SCFAs are the main mediators of the microbial–gut–brain axis in the pathophysiologic process of depression. Chronic stress accompanied by dysbiosis of the gut flora interferes with the metabolism of SCFAs and accelerates the dysfunction of the microbial–gut–brain axis in depression. SCFAs have neuroprotective roles and are involved in the complex biological mechanisms and pathological processes involved in the onset and progression of depression including chronic cerebral hypoperfusion, neuroinflammation, epigenetic modifications, and neuroendocrine alterations, which are summarized in this section, with a focus on their inflammatory contributions.
Bilateral common carotid artery occlusion (BCCAO) has been used to establish a rat model of depression, and recent studies have evaluated the role of SCFAs in depression. Xiao et al. demonstrated that the intestinal flora of BCCAO rats was disturbed, and that the reduction of SCFA-producing flora led to cognitive impairment and depression-like behaviors in the hippocampus of BCCAO rats. Xiao et al. showed that the intestinal flora of BCCAO rats was disturbed, and the reduction of some representative SCFA-producing flora led to the reduction of SCFA in the hippocampus of BCCAO rats, which, in turn, led to cognitive deficits and depressive-like behavior (208). In contrast, researchers have found that SCFAs can reduce hippocampal neuroinflammation and neuronal apoptosis induced by depression models through inhibition of NF-κB and activation of the ERK1/2 pathway, with concomitant improvements in cognitive decline and degenerative processes (208).
Gut microbial-derived SCFAs can play a crucial role in anti-inflammatory actions through direct and indirect mechanisms, as they maintain gut–brain permeability and sustain inputs to the CNS to maintain microglia homeostasis (209). In the CNS, propionate protects the blood–brain barrier (BBB) via FFAR3 on the surface of endothelial cells (210), whereas in the intestinal barrier, SCFAs, especially acetate, propionate, and butyrate, are more protective of the intestinal barrier (211). Butyrate enhances the expression of aromatic hydrocarbon receptor and hypoxia-inducible factor 1α (HIF-1α), and upregulates the levels of IL-12 (212), a protective cytokine that helps to resist inflammatory stimuli and maintain intestinal homeostasis, through modulation of mammalian target proteins of rapamycin and signal transducers and activators of transcription. These findings suggest that SCFAs exert an inhibitory neuroinflammatory effect from an indirect mechanism by maintaining intestinal homeostasis. SCFAs have direct anti-inflammatory effects on microglia proliferation (213) and activation by binding to FFARs. SCFAs could bind to GPR41 on microglia, modulate microglia proliferation and inflammation, and inhibit pro-inflammatory signaling pathways by inhibiting NF-κB and activating Erk1/2 (208). In microglia, butyric acid also activates the GPR109A-mediated signaling pathway to downregulate the NF-κB signaling pathway, inhibits the production of pro-inflammatory enzymes (inducible nitric oxide synthase and cyclooxygenase-2) and pro-inflammatory cytokines (TNF-α, IL-1β, and IL-6) in microglia, and prevents the onset and progression of neuroinflammation. Markers reflecting the anti-inflammatory status of microglia IL-10 and CD26 were elevated after butyrate treatment, suggesting a neuroprotective effect of SCFAs in vivo (214, 215). SCFAs also enhance mitochondrial biogenesis (79) by protecting hippocampal neurons from damage to mitochondrial membrane potential and ROS accumulation (216). It follows that complex interactions between neuroinflammation and anti-inflammation via the SCFA-mediated microbiota–gut–brain axis are involved in the pathophysiology of depression.
Recent studies have shown that chronic inflammation is a risk factor for PCOS, and patients with PCOS are in a state of chronic low-grade inflammation (217), in which inflammatory factors, such as IL-6 and TNF-α, are consistently elevated, and this chronic low-grade inflammation promotes the development of ovarian and metabolic dysfunction in PCOS (218). In addition, higher concentrations of these cytokines and chemokines can lead to the development of reproductive abnormalities with multiple negative effects. On the one hand, abnormal expression of inflammatory factors in the peripheral circulation and ovarian tissues of patients with PCOS induces immune dysfunction and ovulation disorders (219). Dysfunction of the immune system affects follicular development or ovulation (220). On the other hand, inflammatory factors are increased in patients with PCOS, which alters the level of AMH and leads to disorders of glycolipid metabolism (221). Thus, suppression of inflammatory responses is very necessary.
With the deepening research on the pathogenesis of PCOS and its chronic inflammatory state, it has been found that a variety of signaling pathways are involved in the initiation and progression of inflammation (222), among which the more important ones such as PI3K/AKT, MAPK, and AMPK signaling pathways, and the SCFAs have anti-inflammatory roles in PCOS, but these signaling pathways have been reported less frequently in the study of SCFAs. Recent studies have shown that SCFA in PCOS inflammation-related currently has these regulatory mechanisms, such as NF-κB (195, 223), NLRP3 inflammatory vesicles (73, 224), and gamma aminobutyric acid (GABA) (225), but the specific inflammatory pathway mechanisms have not been specifically studied. However, surprisingly, it has been found that SCFAS play an ameliorative role in PCOS to ameliorate inflammation through epigenetic mechanisms. N6-methyladenosine (m6A) is one of the most common forms of RNA modification in mammals (226). Researchers find that m6A modifications can affect cellular inflammation by regulating inflammation-related genes (227). For example, the RNA methyltransferase METTL3 regulates the NF-κB inflammatory pathway by upregulating the level of m6A modification of TRAF6 (228). The addition of butyric acid led to a decrease in FOSL2 m6A methylation level and mRNA expression through inhibition of METTL3 expression and was accompanied by a decrease in NLRP3 and inflammatory factors IL-6 and TNF-α expression, whereas FOSL2, as an AP1 family member, participates in the immune response and promotes the expression of inflammatory factors (229). Furthermore, it has been shown that Clostridium perfringens reduces METTL3-mediated m6A modification by inhibiting the Hippo pathway and activating the Yes-Associated Protein (YAP) signaling pathway (230). Butyric acid activates YAP in human intestinal smooth muscle cells (231). However, whether butyric acid inhibits METTL3 through the YAP pathway or affects METTL3 expression through other proteins requires further study.
It has been shown that stroke may alter the composition of the gut flora, trigger inflammatory responses and microglia activation, and induce dysbiosis of the gut microbiota, which may influence the deterioration of functional stroke outcomes (232, 233). However, commensal bacterial colonization has a protective effect on the brain after stroke (232), and decreasing the number of pathogenic bacteria that inhibit neuronal apoptosis, oxidative stress, and cerebral infarct volume and increasing the number of beneficial bacteria can prevent neurological deficits (234), in which SCFAs play an important role. SCFAs are mediators between the microbiome and the brain and help to regulate prognostic outcomes after ischemic stroke (235). The gut microbiota plays an important role in microglia function, especially through SCFA as a mediator of microbiota–microglia communication (233).
SCFAs regulate microglia inflammation, glucose metabolism, maturation, and activation through activation of GPCRs or inhibition of HDACs, and affect the maintenance of the BBB (236). Studies have shown that the lack of butyrate-producing bacteria and low fecal butyrate levels are possible factors for increased risk of stroke (237). In a rat model of MCAO, researchers found that the gut microbiome stimulated a protective immune response in the brain after stroke, providing evidence that the gut microbiome plays a protective role in the brain region after experimental stroke (232). SCFAs have been identified as key regulators of intestinal immune cells, and in the context of neuroinflammation, SCFAs alter the balance between pro-inflammatory Th1 and Th17 cells and anti-inflammatory Treg cells (238). In mice, low levels of SCFAs, especially butyrate, were observed during middle cerebral artery occlusion (239), whereas high levels of gut microbiota metabolites, especially butyrate, acetate, and propionate, improved prognosis after ischemic stroke (240).
Several studies have demonstrated the contribution of SCFA to BBB integrity and glial function in ischemic stroke animals. Butyrate improved neurological function and significantly reduced ischemic lesions in aged rats after ischemic stroke. In addition, butyrate reduced the expression of occludin and ZO-1, thereby favoring the reduction of BBB permeability (241). Some scholars investigated the effects of sodium butyrate on ischemic stroke in middle-aged rats and found that treatment with sodium butyrate further inhibited the MCAO-induced increase of IL-1β, IL-17A, and IL-18 in the ischemic hemispheric brain lysates (cortex and striatum), and reduced the ischemia-induced upregulation of IL-1β and IL-18 in the circulation, which suggests that sodium butyrate, as an HDAC inhibitor, has a powerful anti-inflammatory effect and can exert neuroprotective effects (242). In another experimental animal model using adult male rats, high levels of SCFAs, particularly sodium butyrate, reduced infarct volume and improved neurological function and attenuated apoptosis through activation of the PI3K/Akt pathway at 24 and 72 h after MCAO (243). A study in male and female rats demonstrated that microbiota-derived SCFA modulate post-stroke recovery by affecting systemic and brain-resident immune cells, optimizing recovery and cortical reorganization, modulating plasticity and synaptic function, and improving motor function (244). It has been shown that a young microbiome can be restored even a few days after ischemic stroke, largely through the action of SCFA to reduce inflammation and promote recovery in older animals (245). Although there are many studies on the effectiveness of SCFA for stroke treatment, there are many gaps in the specific mechanisms and associated inflammatory-immune aspects of the pathways, and future studies could explore the exact mechanisms by which SCFAs exert their beneficial effects after stroke.
IBD, including Crohn’s disease and ulcerative colitis, is characterized by an abnormal inflammatory response in the components of the intestinal flora that play an important role (246). Disturbed and ecologically dysfunctional intestinal flora is a typical feature of IBD, where butyrate-producing bacteria (e.g., Faecalibacterium, Roseburia hominis, and Bifidobacterium) are in lower abundance, resulting in reduced butyrate levels (247, 248). It has been shown that inflammation reduces the responsiveness of the intestinal epithelium to butyrate and the ability to uptake butyrate in patients with IBD (249). Thus, intestinal inflammation may reduce the levels of butyrate-producing bacteria and the utilization of butyrate. Recent evidence suggests that healthy individuals at high genetic risk for IBD have reduced numbers of Roseburia spp. in their gut flora (250). Mouse studies have shown that the anti-inflammatory effects of F. prausnitzii in experimental IBD are directly mediated by butyrate downregulation of the pro-inflammatory IL-6–STAT3–IL-17 signaling pathway through HDAC1 inhibition and transcriptional repression that simultaneously promotes Foxp3 expression, thereby maintaining Treg cells (251). Thus, dysregulation of SCFA levels in the gut may be an important susceptibility factor for IBD.
SCFA can suppress intestinal inflammation by inhibiting excessive signaling by TLR. High intake of dietary fiber increases the level of SCFA in the intestine and effectively reduces the degree of TLR-mediated inflammation (252). It is important to note that TLR4 and TLR2 may be key targets of SCFA in preventing IBD (253). In particular, sodium butyrate, an HDAC inhibitor, can inhibit the expression of TLR4 (254). In IBD patients, butyrate has also been found to inhibit TLR2-mediated inflammatory factor release (255). Butyrate also reduces the expression level of junctional proteins (256). In addition, SCFA activates NLRP109 inflammasome by binding to the receptors GPR3 and GPR43A, ultimately maintaining intestinal health in mice (257). Further studies have shown that SCFA maintains intestinal health by regulating NLRP3 inflammasome assembly and attenuation.
Epidemiologic evidence suggests that gut flora disruption due to antibiotic use early in life is associated with an increased risk of developing IBD (258), particularly in the first year of life, as antibiotic use may cause intense and prolonged microbial disruption at a critical time and have long-term effects on the immune system, increasing its risk of future IBD. Further evidence (259) suggests that SCFA production is more affected by antibiotics, with studies in mice showing that SCFA levels are significantly reduced during antibiotic use, and that rebuilding of the flora after antibiotic treatment leads to overactivation of intestinal macrophages, which, in turn, leads to a long-term pro-inflammatory T-cell response. In this model, supplementation with SCFAs, specifically butyrate, prevented macrophage dysfunction and eliminated pro-inflammatory T-cell responses.
SCFA enemas have been shown to be effective in reducing symptoms in a subgroup of patients with ulcerative colitis (260). Butyric acid enemas also reduce the Disease Activity Index in these patients, but subsequent trials have demonstrated minimal effects on colonic inflammatory parameters (261). A preliminary trial investigated the efficacy of encapsulated butyrate as an adjuvant to conventional therapy in maintaining remission in patients with Crohn’s disease and ulcerative colitis (262). Adjunctive butyric acid therapy reduces fecal levels of the intestinal inflammatory marker calreticulin, stimulates the growth of butyric acid-producing bacteria, and improves quality of life in patients with ulcerative colitis. It is important to note, however, that SCFA alone is unlikely to be a universal solution for diseases associated with microbial ecological dysbiosis, and not all nutritional strategies known to be effective in Crohn’s disease have been associated with elevated butyrate or SCFA levels. For example, the first-line treatment for Crohn’s disease in children is pure enteral nutrition, an approach that results in reduced microbial diversity and lower butyrate levels (263). Thus, further research is needed to determine whether strategies that increase SCFA will improve overall treatment outcomes (264).
With the current advances in the field of intestinal flora research, modulation of SCFA levels holds promise for use in prevention strategies for inflammatory Mets and maintenance of remission of disease progression. There are many ways to increase SCFA levels in the gut, the most advanced of which are butyric acid administration and modulation of butyric acid metabolism using prebiotics and probiotics. Other routes such as specific diets, fecal bacteria transplantation, and genetically modified bacteria administration have also reached the preclinical or clinical trial stage.
Oral (262)or rectal administration (265) increases local butyric acid levels or suppositories, and enema application results in increased levels of intestinal luminal and portal venous butyric acid. However, this process does not increase butyric acid levels in the peripheral blood due to a first-pass effect in the liver. Whereas probiotics have received increasing attention in recent years, there have been reviews summarizing information about F. prausnitzii, R. hominis, and Clostridium spp. A large number of studies have been conducted on the role of probiotics in modulating intestinal inflammation, not the least of which are beneficial aspects for Mets (33). Thanks to advanced technologies in macrogenomics and metabolomics, researchers can improve the application of prebiotics and probiotics by analyzing and predicting metabolic networks and other interactions between individual bacterial species (266). Surprisingly, this allows one to go beyond traditional natural strains and obtain genetically engineered probiotics through transgenic technology, such as E. coli strains producing increased levels of butyric acid, which have demonstrated efficacy in decreasing disease activity and intestinal damage in colitis models (267). However, the relative effectiveness of genetically modified probiotics compared to natural probiotics is unknown, and there is a lack of clinical trials in this area (268).
Fecal flora transplantation aims to replace dysbiotic flora with healthy flora. In the context of Mets, transferring the gut flora of a lean individual to a patient with Mets, there have been several studies demonstrating its mechanism of action and clinical efficacy, such as epigenomic effects on host immune cells through methylation of AFAP1, which can lead to improvements in insulin resistance and mitochondrial function. However, compared to diet and prebiotics and probiotics, fecal flora transplantation has little appeal in patients with Mets, and thus dietary interventions offer a more viable pathway. The use of a diet rich in plant fiber may also enhance the growth of SCFA-producing bacteria (269) or be enriched with fermentable prebiotics such as resistant starch (270). In the case of Mets, maternal metabolism is closely related to that of her offspring due to the genetic nature of Mets, and it has been shown that maternal high-fat diet exacerbates inflammatory responses and Mets, disrupts intestinal barrier function, and alters the gut microbiota of the offspring (271). Therefore, intervening in maternal SCFA levels during pregnancy is gradually becoming a research direction for future development, but it is often unethical to test the effects of pharmaceutical compounds or other therapies on pregnant women; thus, the therapeutic benefits of pregnancy-specific diets may be substantial. Several recent studies have demonstrated direct beneficial effects on offspring and alteration of cognitive and social deficits (272) in offspring through a high-fiber diet by promoting Treg cell differentiation (273). In this context, targeting bifidobacteria may be a therapeutic option, as they can utilize human milk oligosaccharides in breast milk to generate SCFAs, thereby preventing systemic inflammation and immune dysregulation (274). It has also been shown that preventing allergies and atopy by supporting bifidobacteria or a high-fiber diet during pregnancy has a strong potential to counteract the intergenerational effects of microbial dysbiosis that may be induced by antibiotics during pregnancy or the early postpartum period (275).
Since SCFAs are involved in both substance metabolism and enterobacterial products, it is unclear whether the benefits of the SCFA-centered therapies described above are related only to the presence of SCFAs or microbial metabolites themselves. In the future, we can focus more on the mechanistic level and use HDAC inhibitors or GPCR ligands more selectively to study these biological effects, to find more fine-grained targets, to find out the specific mechanism of their action with the help of SCFA, and to treat inflammatory Mets by targeting interventions through their biological effects at a higher level.
Considerable progress has been made in the last decades to better understand the fundamental role of gut flora in health and disease. It is now well established that SCFA, a gut flora derivative, is important in regulating inflammation-associated factors in Mets and its complications such as NAFLD, PCOS, and neurological and cardiovascular diseases. Numerous efforts have been made by basic and clinical translational researchers to determine whether supplementation with SCFA can alleviate or even reverse Mets. In this paper, we summarize the pathways of effect of SCFA in modulating immuno-inflammation in inflammatory Mets; analyze the pathomechanisms of obesity-induced chronic low-grade inflammation leading to oxidative stress, mitochondrial dysfunction, and epigenetic alterations; and itemize the mechanisms of inflammation in the various components and complications of Mets and the roles of SCFA therein; furthermore, we highlight the future directions of research and knowledge gaps.
However, there are still many obstacles and problems from laboratory to clinical applications, especially in terms of the precise effects of different types and concentrations of SCFA in different components and complications, and the sensitivity of different cells to SCFA. Although the effects exerted by the metabolites of specific flora can be analyzed by the latest technology, the substance activity and duration are also issues to be taken into consideration, and the discovery of different targets of SCFA, the use of their targeting mechanisms to guide the treatment of new drugs, and the collaboration of multiple fields including metabolism, microbiology, immunology, genetics, and therapeutic diets will determine the routes of administration and dosages to obtain SCFAs as an anti-inflammatory and anti-immune mechanism in Mets. The optimal benefit of SCFAs in Mets could provide a refreshing opportunity for future prevention and treatment of Mets.
WY: Conceptualization, Software, Writing – original draft, Writing – review & editing. SS: Data curation, Writing – original draft, Writing – review & editing. YY: Data curation, Writing – revised draft, Writing – review & editing. HZ: Data curation, Writing – revised draft, Writing – review & editing. ZL: Data curation, Writing – revised draft, Writing – review & editing. QF: Methodology, Writing – original draft, Writing – review & editing.
The author(s) declare financial support was received for the research, authorship, and/or publication of this article. This study was supported by Dongzhimen Hospital Science and Technology Innovation Project (DZMKJCX-2024-021), National Natural Science Foundation of China (NSFC) (8200151369) and Beijing University of Chinese Medicine unveils list of marshal programs (2023-JYB-JBZD-003).
We would like to thank Jiaying Yan for her contribution to section 5.2 during the revision of this paper.
The authors declare that the research was conducted in the absence of any commercial or financial relationships that could be construed as a potential conflict of interest.
The author(s) declare that no Generative AI was used in the creation of this manuscript.
All claims expressed in this article are solely those of the authors and do not necessarily represent those of their affiliated organizations, or those of the publisher, the editors and the reviewers. Any product that may be evaluated in this article, or claim that may be made by its manufacturer, is not guaranteed or endorsed by the publisher.
1. Carnethon MR, Loria CM, Hill JO, Sidney S, Savage PJ, Liu K. Risk factors for the metabolic syndrome. Diabetes Care. (2004) 27:2707–15. doi: 10.2337/diacare.27.11.2707
2. Saklayen MG. The global epidemic of the metabolic syndrome. Curr Hypertens Rep. (2018) 20:12. doi: 10.1007/s11906-018-0812-z
3. Guembe MJ, Fernandez-Lazaro CI, Sayon-Orea C, Toledo E, Moreno-Iribas C, for the RIVANA Study Investigators, Cosials JB, et al. Risk for cardiovascular disease associated with metabolic syndrome and its components: a 13-year prospective study in the RIVANA cohort. Cardiovasc Diabetol. (2020) 19:195. doi: 10.1186/s12933-020-01166-6
4. Scurt FG, Ganz MJ, Herzog C, Bose K, Mertens PR, Chatzikyrkou C. Association of metabolic syndrome and chronic kidney disease. Obes Rev. (2024) 25:e13649. doi: 10.1111/obr.13649
5. Bridgeman SC, Northrop W, Melton PE, Ellison GC, Newsholme P, Mamotte CDS. Butyrate generated by gut microbiota and its therapeutic role in metabolic syndrome. Pharmacol Res. (2020) 160:105174. doi: 10.1016/j.phrs.2020.105174
6. Zhou H, Yu B, Sun J, Liu Z, Chen H, Ge L, et al. Short-chain fatty acids can improve lipid and glucose metabolism independently of the pig gut microbiota. J Anim Sci Biotechnol. (2021) 12:61. doi: 10.1186/s40104-021-00581-3
7. Kawano Y, Edwards M, Huang Y, Bilate AM, Araujo LP, Tanoue T, et al. Microbiota imbalance induced by dietary sugar disrupts immune-mediated protection from metabolic syndrome. Cell. (2022) 185:3501–3519.e20. doi: 10.1016/j.cell.2022.08.005
8. Li X-Y, He C, Zhu Y, Lu N-H. Role of gut microbiota on intestinal barrier function in acute pancreatitis. World J Gastroenterol. (2020) 26:2187–93. doi: 10.3748/wjg.v26.i18.2187
9. Kvietys PR, Specian RD, Grisham MB, Tso P. Jejunal mucosal injury and restitution: role of hydrolytic products of food digestion. Am J Physiology-Gastrointestinal Liver Physiol. (1991) 261:G384–91. doi: 10.1152/ajpgi.1991.261.3.G384
10. Wang Y, Ghoshal S, Ward M, De Villiers W, Woodward J, Eckhardt E. Chylomicrons promote intestinal absorption and systemic dissemination of dietary antigen (Ovalbumin) in mice. PloS One. (2009) 4:e8442. doi: 10.1371/journal.pone.0008442
11. Ghoshal S, Witta J, Zhong J, De Villiers W, Eckhardt E. Chylomicrons promote intestinal absorption of lipopolysaccharides. J Lipid Res. (2009) 50:90–7. doi: 10.1194/jlr.M800156-JLR200
12. Wang Y, Li J, Tang L, Wang Y, Charnigo R, De Villiers W, et al. T-lymphocyte responses to intestinally absorbed antigens can contribute to adipose tissue inflammation and glucose intolerance during high fat feeding. PloS One. (2010) 5:e13951. doi: 10.1371/journal.pone.0013951
13. Duffaut C, Galitzky J, Lafontan M, Bouloumié A. Unexpected trafficking of immune cells within the adipose tissue during the onset of obesity. Biochem Biophys Res Commun. (2009) 384:482–5. doi: 10.1016/j.bbrc.2009.05.002
14. Wu H, Ghosh S, Perrard XD, Feng L, Garcia GE, Perrard JL, et al. T-cell accumulation and regulated on activation, normal T cell expressed and secreted upregulation in adipose tissue in obesity. Circulation. (2007) 115:1029–38. doi: 10.1161/CIRCULATIONAHA.106.638379
15. Ouchi N, Parker JL, Lugus JJ, Walsh K. Adipokines in inflammation and metabolic disease. Nat Rev Immunol. (2011) 11:85–97. doi: 10.1038/nri2921
16. Fan Y, Pedersen O. Gut microbiota in human metabolic health and disease. Nat Rev Microbiol. (2021) 19:55–71. doi: 10.1038/s41579-020-0433-9
17. Luo L, Chang Y, Sheng L. Gut-liver axis in the progression of nonalcoholic fatty liver disease: From the microbial derivatives-centered perspective. Life Sci. (2023) 321:121614. doi: 10.1016/j.lfs.2023.121614
18. Pinna G. Role of PPAR-allopregnanolone signaling in behavioral and inflammatory gut-brain axis communications. Biol Psychiatry. (2023) 94:609–18. doi: 10.1016/j.biopsych.2023.04.025
19. Zhang Z, Tanaka I, Pan Z, Ernst PB, Kiyono H, Kurashima Y. Intestinal homeostasis and inflammation: Gut microbiota at the crossroads of pancreas–intestinal barrier axis. Eur J Immunol. (2022) 52:1035–46. doi: 10.1002/eji.202149532
20. Nicholson JK, Holmes E, Kinross J, Burcelin R, Gibson G, Jia W, et al. Host-gut microbiota metabolic interactions. Science. (2012) 336:1262–7. doi: 10.1126/science.1223813
21. Yamamura R, Nakamura K, Kitada N, Aizawa T, Shimizu Y, Nakamura K, et al. Associations of gut microbiota, dietary intake, and serum short-chain fatty acids with fecal short-chain fatty acids. Biosci Microbiota Food Health. (2020) 39:11–7. doi: 10.12938/bmfh.19-010
22. Wang M, Wichienchot S, He X, Fu X, Huang Q, Zhang B. In vitro colonic fermentation of dietary fibers: Fermentation rate, short-chain fatty acid production and changes in microbiota. Trends Food Sci Technol. (2019) 88:1–9. doi: 10.1016/j.tifs.2019.03.005
23. Macfarlane S, Macfarlane GT. Regulation of short-chain fatty acid production. Proc Nutr Soc. (2003) 62:67–72. doi: 10.1079/PNS2002207
24. Tan J, McKenzie C, Potamitis M, Thorburn AN, Mackay CR, Macia L. The role of short-chain fatty acids in health and disease. Adv Immunol. (2014) 121:91–119. doi: 10.1016/B978-0-12-800100-4.00003-9
25. Wardman JF, Bains RK, Rahfeld P, Withers SG. Carbohydrate-active enzymes (CAZymes) in the gut microbiome. Nat Rev Microbiol. (2022) 20:542–56. doi: 10.1038/s41579-022-00712-1
26. Wastyk HC, Fragiadakis GK, Perelman D, Dahan D, Merrill BD, Yu FB, et al. Gut-microbiota-targeted diets modulate human immune status. Cell. (2021) 184:4137–4153.e14. doi: 10.1016/j.cell.2021.06.019
27. Barker HA. Amino acid degradation by anaerobic bacteria. Annu Rev Biochem. (1981) 50:23–40. doi: 10.1146/annurev.bi.50.070181.000323
28. De Filippo C, Cavalieri D, Di Paola M, Ramazzotti M, Poullet JB, Massart S, et al. Impact of diet in shaping gut microbiota revealed by a comparative study in children from Europe and rural Africa. Proc Natl Acad Sci USA. (2010) 107:14691–6. doi: 10.1073/pnas.1005963107
29. Frank DN, St. Amand AL, Feldman RA, Boedeker EC, Harpaz N, Pace NR. Molecular-phylogenetic characterization of microbial community imbalances in human inflammatory bowel diseases. Proc Natl Acad Sci USA. (2007) 104:13780–5. doi: 10.1073/pnas.0706625104
30. Meijer K, de Vos P, Priebe MG. Butyrate and other short-chain fatty acids as modulators of immunity: what relevance for health? Curr Opin Clin Nutr Metab Care. (2010) 13:715–21. doi: 10.1097/MCO.0b013e32833eebe5
31. Cavaglieri CR, Nishiyama A, Fernandes LC, Curi R, Miles EA, Calder PC. Differential effects of short-chain fatty acids on proliferation and production of pro- and anti-inflammatory cytokines by cultured lymphocytes. Life Sci. (2003) 73:1683–90. doi: 10.1016/S0024-3205(03)00490-9
32. Yao Y, Cai X, Fei W, Ye Y, Zhao M, Zheng C. The role of short-chain fatty acids in immunity, inflammation and metabolism. Crit Rev Food Sci Nutr. (2022) 62:1–12. doi: 10.1080/10408398.2020.1854675
33. Mann ER, Lam YK, Uhlig HH. Short-chain fatty acids: linking diet, the microbiome and immunity. Nat Rev Immunol. (2024) 24:577–95 . doi: 10.1038/s41577-024-01014-8
34. Hagar JA, Powell DA, Aachoui Y, Ernst RK, Miao EA. Cytoplasmic LPS activates caspase-11: implications in TLR4-independent endotoxic shock. Science. (2013) 341:1250–3. doi: 10.1126/science.1240988
35. Park BS, Lee J-O. Recognition of lipopolysaccharide pattern by TLR4 complexes. Exp Mol Med. (2013) 45:e66. doi: 10.1038/emm.2013.97
36. Liu SF, Malik AB. NF-κB activation as a pathological mechanism of septic shock and inflammation. Am J Physiology-Lung Cell Mol Physiol. (2006) 290:L622–45. doi: 10.1152/ajplung.00477.2005
37. Adrie C, Pinsky MR. The inflammatory balance in human sepsis. Intensive Care Med. (2000) 26:364–75. doi: 10.1007/s001340051169
38. Pahl HL. Activators and target genes of Rel/NF-κB transcription factors. Oncogene. (1999) 18:6853–66. doi: 10.1038/sj.onc.1203239
39. Sun M, Wu W, Liu Z, Cong Y. Microbiota metabolite short chain fatty acids, GPCR, and inflammatory bowel diseases. J Gastroenterol. (2017) 52:1–8. doi: 10.1007/s00535-016-1242-9
40. Sarkar A, Mitra P, Lahiri A, Das T, Sarkar J, Paul S, et al. Butyrate limits inflammatory macrophage niche in NASH. Cell Death Dis. (2023) 14:332. doi: 10.1038/s41419-023-05853-6
41. Byndloss MX, Olsan EE, Rivera-Chávez F, Tiffany CR, Cevallos SA, Lokken KL, et al. Microbiota-activated PPAR-γ signaling inhibits dysbiotic Enterobacteriaceae expansion. Science. (2017) 357:570–5. doi: 10.1126/science.aam9949
42. Chen Y, Wang Y, Fu Y, Yin Y, Xu K. Modulating AHR function offers exciting therapeutic potential in gut immunity and inflammation. Cell Biosci. (2023) 13:85. doi: 10.1186/s13578-023-01046-y
43. Lund PJ, Gates LA, Leboeuf M, Smith SA, Chau L, Lopes M, et al. Stable isotope tracing in vivo reveals a metabolic bridge linking the microbiota to host histone acetylation. Cell Rep. (2022) 41:111809. doi: 10.1016/j.celrep.2022.111809
44. Furusawa Y, Obata Y, Fukuda S, Endo TA, Nakato G, Takahashi D, et al. Commensal microbe-derived butyrate induces the differentiation of colonic regulatory T cells. Nature. (2013) 504:446–50. doi: 10.1038/nature12721
45. Chang PV, Hao L, Offermanns S, Medzhitov R. The microbial metabolite butyrate regulates intestinal macrophage function via histone deacetylase inhibition. Proc Natl Acad Sci USA. (2014) 111:2247–52. doi: 10.1073/pnas.1322269111
46. Schulthess J, Pandey S, Capitani M, Rue-Albrecht KC, Arnold I, Franchini F, et al. The short chain fatty acid butyrate imprints an antimicrobial program in macrophages. Immunity. (2019) 50:432–445.e7. doi: 10.1016/j.immuni.2018.12.018
47. Elce A, Amato F, Zarrilli F, Calignano A, Troncone R, Castaldo G, et al. Butyrate modulating effects on pro-inflammatory pathways in human intestinal epithelial cells. BM. (2017) 8:841–8. doi: 10.3920/BM2016.0197
48. Grouls M, Janssen AWF, Duivenvoorde LPM, Hooiveld GJEJ, Bouwmeester H, Van Der Zande M. Differential gene expression in iPSC-derived human intestinal epithelial cell layers following exposure to two concentrations of butyrate, propionate and acetate. Sci Rep. (2022) 12:13988. doi: 10.1038/s41598-022-17296-8
49. Festi D, et al. Gut microbiota and metabolic syndrome. WJG. (2014) 20:16079. doi: 10.3748/wjg.v20.i43.16079
50. Magliocca G, Mone P, Di Iorio BR, Heidland A, Marzocco S. Short-chain fatty acids in chronic kidney disease: focus on inflammation and oxidative stress regulation. IJMS. (2022) 23:5354. doi: 10.3390/ijms23105354
51. Marinissen MJ, Gutkind JS. G-protein-coupled receptors and signaling networks: emerging paradigms. Trends Pharmacol Sci. (2001) 22:368–76. doi: 10.1016/S0165-6147(00)01678-3
52. Thangaraju M, Cresci GA, Liu K, Ananth S, Gnanaprakasam JP, Browning DD, et al. GPR109A is a G-protein–coupled receptor for the bacterial fermentation product butyrate and functions as a tumor suppressor in colon. Cancer Res. (2009) 69:2826–32. doi: 10.1158/0008-5472.CAN-08-4466
53. Park J, Kim M, Kang SG, Jannasch AH, Cooper B, Patterson J, et al. Short-chain fatty acids induce both effector and regulatory T cells by suppression of histone deacetylases and regulation of the mTOR–S6K pathway. Mucosal Immunol. (2015) 8:80–93. doi: 10.1038/mi.2014.44
54. Ramezani A, Massy ZA, Meijers B, Evenepoel P, Vanholder R, Raj DS. Role of the gut microbiome in uremia: A potential therapeutic target. Am J Kidney Dis. (2016) 67:483–98. doi: 10.1053/j.ajkd.2015.09.027
55. Tolhurst G, Heffron H, Lam YS, Parker HE, Habib AM, Diakogiannaki E, et al. Short-chain fatty acids stimulate glucagon-like peptide-1 secretion via the G-protein–coupled receptor FFAR2. Diabetes. (2012) 61:364–71. doi: 10.2337/db11-1019
56. Wang Y, Li C, Li J, Wang G, Li L. Non-esterified fatty acid-induced reactive oxygen species mediated granulosa cells apoptosis is regulated by nrf2/p53 signaling pathway. Antioxidants. (2020) 9:523. doi: 10.3390/antiox9060523
57. Song Y, Li X, Li Y, Li N, Shi X, Ding H, et al. Non-esterified fatty acids activate the ROS–p38–p53/Nrf2 signaling pathway to induce bovine hepatocyte apoptosis. vitro Apoptosis. (2014) 19:984–97. doi: 10.1007/s10495-014-0982-3
58. Logsdon AF, Erickson MA, Rhea EM, Salameh TS, Banks WA. Gut reactions: How the blood–brain barrier connects the microbiome and the brain. Exp Biol Med (Maywood). (2018) 243:159–65. doi: 10.1177/1535370217743766
59. Krata N, Zagożdżon R, Foroncewicz B, Mucha K. Oxidative stress in kidney diseases: the cause or the consequence? Arch Immunol Ther Exp. (2018) 66:211–20. doi: 10.1007/s00005-017-0496-0
60. Guo W, Liu J, Sun J, Gong Q, Ma H, Kan X, et al. Butyrate alleviates oxidative stress by regulating NRF2 nuclear accumulation and H3K9/14 acetylation via GPR109A in bovine mammary epithelial cells and mammary glands. Free Radical Biol Med. (2020) 152:728–42. doi: 10.1016/j.freeradbiomed.2020.01.016
61. Srivastava S, Alfieri A, Siow RCM, Mann GE, Fraser PA. Temporal and spatial distribution of Nrf2 in rat brain following stroke: quantification of nuclear to cytoplasmic Nrf2 content using a novel immunohistochemical technique. J Physiol. (2013) 591:3525–38. doi: 10.1113/tjp.2013.591.issue-14
62. Vinolo MAR, Hatanaka E, Lambertucci RH, Newsholme P, Curi R. Effects of short chain fatty acids on effector mechanisms of neutrophils. Cell Biochem Funct. (2009) 27:48–55. doi: 10.1002/cbf.v27:1
63. Maslowski KM, Vieira AT, Ng A, Kranich J, Sierro F, Di Yu , et al. Regulation of inflammatory responses by gut microbiota and chemoattractant receptor GPR43. Nature. (2009) 461:1282–6. doi: 10.1038/nature08530
64. Wang W, Fang D, Zhang H, Xue J, Wangchuk D, Du J, et al. Sodium butyrate selectively kills cancer cells and inhibits migration in colorectal cancer by targeting thioredoxin-1. OTT Volume. (2020) 13:4691–704. doi: 10.2147/OTT.S235575
65. Matthews GM, Howarth GS, Butler RN. Short-chain fatty acid modulation of apoptosis in the kato III human gastric carcinoma cell line. Cancer Biol Ther. (2007) 6:1051–7. doi: 10.4161/cbt.6.7.4318
66. Baker JR, Vuppusetty C, Colley T, Papaioannou AI, Fenwick P, Donnelly L, et al. Oxidative stress dependent microRNA-34a activation via PI3Kα reduces the expression of sirtuin-1 and sirtuin-6 in epithelial cells. Sci Rep. (2016) 6:35871. doi: 10.1038/srep35871
67. Bent EH, Gilbert LA, Hemann MT. A senescence secretory switch mediated by PI3K/AKT/mTOR activation controls chemoprotective endothelial secretory responses. Genes Dev. (2016) 30:1811–21. doi: 10.1101/gad.284851.116
68. Lagouge M, Argmann C, Gerhart-Hines Z, Meziane H, Lerin C, Daussin F, et al. Resveratrol improves mitochondrial function and protects against metabolic disease by activating SIRT1 and PGC-1α. Cell. (2006) 127:1109–22. doi: 10.1016/j.cell.2006.11.013
69. Kjær TN, Ornstrup MJ, Poulsen MM, Stødkilde-Jørgensen H, Jessen N, Jørgensen JOL, et al. No beneficial effects of resveratrol on the metabolic syndrome: A randomized placebo-controlled clinical trial. J Clin Endocrinol Metab. (2017) 102:1642–51. doi: 10.1210/jc.2016-2160
70. Kim TT, Parajuli N, Sung MM, Bairwa SC, Levasseur J, Soltys C-LM, et al. Fecal transplant from resveratrol-fed donors improves glycaemia and cardiovascular features of the metabolic syndrome in mice. Am J Physiology-Endocrinology Metab. (2018) 315:E511–9. doi: 10.1152/ajpendo.00471.2017
71. Ding J, Liu J, Chen J, Cheng X, Cao H, Guo X, et al. Sodium butyrate alleviates free fatty acid-induced steatosis in primary chicken hepatocytes via the AMPK/PPARα pathway. Poult Sci. (2024) 103:103482. doi: 10.1016/j.psj.2024.103482
72. Wang S, Zhang B, Chang X, Zhao H, Zhang H, Zhao T, et al. Potential use of seaweed polysaccharides as prebiotics for management of metabolic syndrome: a review. Crit Rev Food Sci Nutr. (2023) 64:7707–27. doi: 10.1080/10408398.2023.2191135
73. Olaniyi KS, Areloegbe SE. Acetate circumvents impaired metabolic switch in skeletal muscle of letrozole-induced PCOS rat model by suppression of PDK4/NLRP3. Nutrition. (2023) 107:111914. doi: 10.1016/j.nut.2022.111914
74. Olaniyi KS, Areloegbe SE. Acetate: A therapeutic candidate against renal disorder in a rat model of polycystic ovarian syndrome. J Steroid Biochem Mol Biol. (2023) 225:106179. doi: 10.1016/j.jsbmb.2022.106179
75. Pate T, Anthony DC, Radford-Smith DE. cFOS expression in the prefrontal cortex correlates with altered cerebral metabolism in developing germ-free mice. Front Mol Neurosci. (2023) 16:1155620. doi: 10.3389/fnmol.2023.1155620
76. Guo J, Xu F, Xie Y, Chen B, Wang Y, Nie W, et al. Effect of xuanwei ham proteins with different ripening periods on lipid metabolism, oxidative stress, and gut microbiota in mice. Mol Nutr Food Res. (2022) 66:e2101020. doi: 10.1002/mnfr.202101020
77. Richter-Stretton GL, Fenning AS, Vella RK. Skeletal muscle – A bystander or influencer of metabolic syndrome? Diabetes Metab Syndrome: Clin Res Rev. (2020) 14:867–75. doi: 10.1016/j.dsx.2020.06.006
78. Kim J, Wei Y, Sowers JR. Role of mitochondrial dysfunction in insulin resistance. Circ Res. (2008) 102:401–14. doi: 10.1161/CIRCRESAHA.107.165472
79. Ribeiro MF, Santos AA, Afonso MB, Rodrigues PM, Sá Santos S, Castro RE, et al. Diet-dependent gut microbiota impacts on adult neurogenesis through mitochondrial stress modulation. Brain Commun. (2020) 2:fcaa165. doi: 10.1093/braincomms/fcaa165
80. Su S-H, Wu Y-F, Lin Q, Zhang L, Wang D-P, Hai J. Fecal microbiota transplantation and replenishment of short-chain fatty acids protect against chronic cerebral hypoperfusion-induced colonic dysfunction by regulating gut microbiota, differentiation of Th17 cells, and mitochondrial energy metabolism. J Neuroinflamm. (2022) 19:313. doi: 10.1186/s12974-022-02675-9
81. Wu H, Wang Y, Li W, Chen H, Du L, Liu D, et al. Deficiency of mitophagy receptor FUNDC1 impairs mitochondrial quality and aggravates dietary-induced obesity and metabolic syndrome. Autophagy. (2019) 15:1882–98. doi: 10.1080/15548627.2019.1596482
82. Su Z, Nie Y, Huang X, Zhu Y, Feng B, Tang L, et al. Mitophagy in hepatic insulin resistance: therapeutic potential and concerns. Front Pharmacol. (2019) 10:1193. doi: 10.3389/fphar.2019.01193
83. Tang Y, Chen Y, Jiang H, Nie D. Short-chain fatty acids induced autophagy serves as an adaptive strategy for retarding mitochondria-mediated apoptotic cell death. Cell Death Differ. (2011) 18:602–18. doi: 10.1038/cdd.2010.117
84. Cho JH, Chae CW, Lim JR, Jung YH, Han SJ, Yoon JH, et al. Sodium butyrate ameliorates high glucose-suppressed neuronal mitophagy by restoring PRKN expression via inhibiting the RELA-HDAC8 complex. Autophagy. (2024) 20:1505–22. doi: 10.1080/15548627.2024.2323785
85. Hoshino A, Ariyoshi M, Okawa Y, Kaimoto S, Uchihashi M, Fukai K, et al. Inhibition of p53 preserves Parkin-mediated mitophagy and pancreatic β-cell function in diabetes. Proc Natl Acad Sci USA. (2014) 111:3116–21. doi: 10.1073/pnas.1318951111
86. Wang S-H, Zhu X-L, Wang F, Chen S-X, Chen Z-T, Qiu Q, et al. LncRNA H19 governs mitophagy and restores mitochondrial respiration in the heart through Pink1/Parkin signaling during obesity. Cell Death Dis. (2021) 12:557. doi: 10.1038/s41419-021-03821-6
87. Ren J, Sun M, Zhou H, Ajoolabady A, Zhou Y, Tao J, et al. FUNDC1 interacts with FBXL2 to govern mitochondrial integrity and cardiac function through an IP3R3-dependent manner in obesity. Sci Adv. (2020) 6:eabc8561. doi: 10.1126/sciadv.abc8561
88. Li R, Xin T, Li D, Wang C, Zhu H, Zhou H. Therapeutic effect of Sirtuin 3 on ameliorating nonalcoholic fatty liver disease: The role of the ERK-CREB pathway and Bnip3-mediated mitophagy. Redox Biol. (2018) 18:229–43. doi: 10.1016/j.redox.2018.07.011
89. Bhujabal Z, Birgisdottir ÅB, Sjøttem E, Brenne HB, Øvervatn A, Habisov S, et al. FKBP8 recruits LC3A to mediate Parkin-independent mitophagy. EMBO Rep. (2017) 18:947–61. doi: 10.15252/embr.201643147
90. Brand MD, Parker N, Affourtit C, Mookerjee SA, Azzu V. Mitochondrial uncoupling protein 2 in pancreatic β-cells. Diabetes Obes Metab. (2010) 12 Suppl:2, 134–140. doi: 10.1111/j.1463-1326.2010.01264.x
91. Meng F, Sun N, Liu D, Jia J, Xiao J, Dai H. BCL2L13: physiological and pathological meanings. Cell Mol Life Sci. (2021) 78:2419–28. doi: 10.1007/s00018-020-03702-9
92. Ju L, Chen S, Alimujiang M, Bai N, Yan H, Fang Q, et al. A novel role for Bcl2l13 in promoting beige adipocyte biogenesis. Biochem Biophys Res Commun. (2018) 506:485–91. doi: 10.1016/j.bbrc.2018.10.034
93. Fujiwara M, Tian L, Le PT, DeMambro VE, Becker KA, Rosen CJ, et al. The mitophagy receptor Bcl-2–like protein 13 stimulates adipogenesis by regulating mitochondrial oxidative phosphorylation and apoptosis in mice. J Biol Chem. (2019) 294:12683–94. doi: 10.1074/jbc.RA119.008630
94. Yang J, Manolio TA, Pasquale LR, Boerwinkle E, Caporaso N, Cunningham JM, et al. Genome partitioning of genetic variation for complex traits using common SNPs. Nat Genet. (2011) 43:519–25. doi: 10.1038/ng.823
95. Rönn T, Volkov P, Davegårdh C, Dayeh T, Hall E, Olsson AH, et al. A six months exercise intervention influences the genome-wide DNA methylation pattern in human adipose tissue. PloS Genet. (2013) 9:e1003572. doi: 10.1371/journal.pgen.1003572
96. Feinberg AP, Irizarry RA, Fradin D, Aryee MJ, Murakami P, Aspelund T, et al. Personalized epigenomic signatures that are stable over time and covary with body mass index. Sci Transl Med. (2010) 2:49ra67. doi: 10.1126/scitranslmed.3001262
97. Soubry A, Schildkraut JM, Murtha A, Wang F, Huang Z, Bernal A, et al. Paternal obesity is associated with IGF2hypomethylation in newborns: results from a Newborn Epigenetics Study (NEST) cohort. BMC Med. (2013) 11:29. doi: 10.1186/1741-7015-11-29
98. Lin HV, Frassetto A, Kowalik EJ Jr, Nawrocki AR, Lu MM, Kosinski JR, et al. Butyrate and Propionate Protect against Diet-Induced Obesity and Regulate Gut Hormones via Free Fatty Acid Receptor 3-Independent Mechanisms. PloS One. (2012) 7:e35240. doi: 10.1371/journal.pone.0035240
99. Hippe B, Remely M, Aumueller E, Pointner A, Haslberger AG. SCFA Producing Gut Microbiota and its Effects on the Epigenetic Regulation of Inflammation. In: Liong M-T, editor. Beneficial Microorganisms in Medical and Health Applications, vol. 28 . Springer International Publishing, Cham (2015). p. 181–97.
100. Diamant M, Blaak EE, De Vos WM. Do nutrient–gut–microbiota interactions play a role in human obesity, insulin resistance and type 2 diabetes? Obes Rev. (2011) 12:272–81. doi: 10.1111/j.1467-789X.2010.00797.x
101. Takahashi K, Sugi Y, Nakano K, Tsuda M, Kurihara K, Hosono A, et al. Epigenetic Control of the host gene by commensal bacteria in large intestinal epithelial cells. J Biol Chem. (2011) 286:35755–62. doi: 10.1074/jbc.M111.271007
102. Pushalkar S, Hundeyin M, Daley D, Zambirinis CP, Kurz E, Mishra A, et al. The pancreatic cancer microbiome promotes oncogenesis by induction of innate and adaptive immune suppression. Cancer Discovery. (2018) 8:403–16. doi: 10.1158/2159-8290.CD-17-1134
103. Panpetch W, Kullapanich C, Dang CP, Visitchanakun P, Saisorn W, Wongphoom J, et al. Candida administration worsens uremia-induced gut leakage in bilateral nephrectomy mice, an impact of gut fungi and organismal molecules in uremia. mSystems. (2021) 6:e01187–20. doi: 10.1128/mSystems.01187-20
104. Li X, He C, Li N, Ding L, Chen H, Wan J, et al. The interplay between the gut microbiota and NLRP3 activation affects the severity of acute pancreatitis in mice. Gut Microbes. (2020) 11:1774–89. doi: 10.1080/19490976.2020.1770042
105. Michielan A, D’Incà R. Intestinal permeability in inflammatory bowel disease: pathogenesis, clinical evaluation, and therapy of leaky gut. Mediators Inflammation. (2015) 2015:628157. doi: 10.1155/2015/628157
106. Brenchley JM, Douek DC. Microbial translocation across the GI tract. Annu Rev Immunol. (2012) 30:149–73. doi: 10.1146/annurev-immunol-020711-075001
107. Li Z, Qiao L, Yun X, Du F, Xing S, Yang M. Increased risk of ischemic heart disease and diabetes in inflammatory bowel disease. Z Gastroenterol. (2021) 59:117–24. doi: 10.1055/a-1283-6966
108. Garcia Garcia De Paredes A, Rodriguez De Santiago E, Rodriguez-Escaja C, Iborra M, Algaba A, Cameo JI, et al. Idiopathic acute pancreatitis in patients with inflammatory bowel disease: A multicenter cohort study. Pancreatology. (2020) 20:331–7. doi: 10.1016/j.pan.2020.02.007
109. Everhov Å.H, Erichsen R, Sachs MC, Pedersen L, Halfvarson J, Askling J, et al. Inflammatory bowel disease and pancreatic cancer: a Scandinavian register-based cohort study 1969-2017. Aliment Pharmacol Ther. (2020) 52:143–54. doi: 10.1111/apt.15785
110. Jess T, Jensen BW, Andersson M, Villumsen M, Allin KH. Inflammatory bowel diseases increase risk of type 2 diabetes in a nationwide cohort study. Clin Gastroenterol Hepatol. (2020) 18:881–888.e1. doi: 10.1016/j.cgh.2019.07.052
111. Wang K, Baldassano R, Zhang H, Qu H-Q, Imielinski M, Kugathasan S, et al. Comparative genetic analysis of inflammatory bowel disease and type 1 diabetes implicates multiple loci with opposite effects. Hum Mol Genet. (2010) 19:2059–67. doi: 10.1093/hmg/ddq078
112. Yilmaz B, Spalinger MR, Biedermann L, Franc Y, Fournier N, Rossel J-B, et al. The presence of genetic risk variants within PTPN2 and PTPN22 is associated with intestinal microbiota alterations in Swiss IBD cohort patients. PloS One. (2018) 13:e0199664. doi: 10.1371/journal.pone.0199664
113. Campos-Perez W, Martinez-Lopez E. Effects of short chain fatty acids on metabolic and inflammatory processes in human health. Biochim Biophys Acta (BBA) - Mol Cell Biol Lipids. (2021) 1866:158900. doi: 10.1016/j.bbalip.2021.158900
114. Yang Q, Ouyang J, Sun F, Yang J. Short-chain fatty acids: A soldier fighting against inflammation and protecting from tumorigenesis in people with diabetes. Front Immunol. (2020) 11:590685. doi: 10.3389/fimmu.2020.590685
115. Kopczyńska J, Kowalczyk M. The potential of short-chain fatty acid epigenetic regulation in chronic low-grade inflammation and obesity. Front Immunol. (2024) 15:1380476. doi: 10.3389/fimmu.2024.1380476
116. Den Besten G, Bleeker A, Gerding A, Van Eunen K, Havinga R, Van Dijk TH, et al. Short-chain fatty acids protect against high-fat diet–induced obesity via a PPARγ-dependent switch from lipogenesis to fat oxidation. Diabetes. (2015) 64:2398–408. doi: 10.2337/db14-1213
117. Pham NHT, Joglekar MV, Wong WKM, Nassif NT, Simpson AM, Hardikar AA. Short-chain fatty acids and insulin sensitivity: a systematic review and meta-analysis. Nutr Rev. (2024) 82:193–209. doi: 10.1093/nutrit/nuad042
118. Ojo O, Feng Q-Q, Ojo OO, Wang X-H. The role of dietary fibre in modulating gut microbiota dysbiosis in patients with type 2 diabetes: A systematic review and meta-analysis of randomised controlled trials. Nutrients. (2020) 12:3239. doi: 10.3390/nu12113239
119. Sun J, Furio L, Mecheri R, van der Does AM, Lundeberg E, Saveanu L, et al. Pancreatic β-Cells Limit Autoimmune Diabetes via an Immunoregulatory Antimicrobial Peptide Expressed under the Influence of the Gut Microbiota. Immunity. (2015) 43:304–17. doi: 10.1016/j.immuni.2015.07.013
120. Tran HQ, Bretin A, Adeshirlarijaney A, Yeoh BS, Vijay-Kumar M, Zou J, et al. Western diet”-induced adipose inflammation requires a complex gut microbiota. Cell Mol Gastroenterol Hepatol. (2020) 9:313–33. doi: 10.1016/j.jcmgh.2019.09.009
121. Pereira DIA, Gibson GR. Effects of consumption of probiotics and prebiotics on serum lipid levels in humans. Crit Rev Biochem Mol Biol. (2002) 37:259–81. doi: 10.1080/10409230290771519
122. Cani PD, Neyrinck AM, Fava F, Knauf C, Burcelin RG, Tuohy KM, et al. Selective increases of bifidobacteria in gut microflora improve high-fat-diet-induced diabetes in mice through a mechanism associated with endotoxaemia. Diabetologia. (2007) 50:2374–83. doi: 10.1007/s00125-007-0791-0
123. Cerdó T, García-Santos JA, G Bermúdez M, Campoy C. The role of probiotics and prebiotics in the prevention and treatment of obesity. Nutrients. (2019) 11:635. doi: 10.3390/nu11030635
124. Zambell KL, Fitch MD, Fleming SE. Acetate and butyrate are the major substrates for de novo lipogenesis in rat colonic epithelial cells. J Nutr. (2003) 133:3509–15. doi: 10.1093/jn/133.11.3509
125. Kindt A, Liebisch G, Clavel T, Haller D, Hörmannsperger G, Yoon H, et al. The gut microbiota promotes hepatic fatty acid desaturation and elongation in mice. Nat Commun. (2018) 9:3760. doi: 10.1038/s41467-018-05767-4
126. Gao Z, Yin J, Zhang J, Ward RE, Martin RJ, Lefevre M, et al. Butyrate improves insulin sensitivity and increases energy expenditure in mice. Diabetes. (2009) 58:1509–17. doi: 10.2337/db08-1637
127. Li Z, Yi C-X, Katiraei S, Kooijman S, Zhou E, Chung CK, et al. Butyrate reduces appetite and activates brown adipose tissue via the gut-brain neural circuit. Gut. (2018) 67:1269–79. doi: 10.1136/gutjnl-2017-314050
128. Wan Z, Root-McCaig J, Castellani L, Kemp BE, Steinberg GR, Wright DC. Evidence for the role of AMPK in regulating PGC-1 alpha expression and mitochondrial proteins in mouse epididymal adipose tissue. Obes (Silver Spring). (2014) 22:730–8. doi: 10.1002/oby.20605
129. Muoio DM, Way JM, Tanner CJ, Winegar DA, Kliewer SA, Houmard JA, et al. Peroxisome proliferator-activated receptor-alpha regulates fatty acid utilization in primary human skeletal muscle cells. Diabetes. (2002) 51:901–9. doi: 10.2337/diabetes.51.4.901
130. Cantó C, Auwerx J. AMP-activated protein kinase and its downstream transcriptional pathways. Cell Mol Life Sci. (2010) 67:3407–23. doi: 10.1007/s00018-010-0454-z
131. Tang T, Song J, Li J, Wang H, Zhang Y, Suo H. A synbiotic consisting of Lactobacillus plantarum S58 and hull-less barley β-glucan ameliorates lipid accumulation in mice fed with a high-fat diet by activating AMPK signaling and modulating the gut microbiota. Carbohydr Polym. (2020) 243:116398. doi: 10.1016/j.carbpol.2020.116398
132. Houslay MD, Milligan G. Tailoring cAMP-signalling responses through isoform multiplicity. Trends Biochem Sci. (1997) 22:217–24. doi: 10.1016/S0968-0004(97)01050-5
133. Carmen G-Y, Víctor S-M. Signalling mechanisms regulating lipolysis. Cell Signalling. (2006) 18:401–8. doi: 10.1016/j.cellsig.2005.08.009
134. Jocken JWE, González Hernández MA, Hoebers NTH, Van Der Beek CM, Essers YPG, et al. Short-chain fatty acids differentially affect intracellular lipolysis in a human white adipocyte model. Front Endocrinol. (2018) 8:372. doi: 10.3389/fendo.2017.00372
135. Yap F, Craddock L, Yang J. Mechanism of AMPK suppression of LXR-dependent Srebp-1c transcription. Int J Biol Sci. (2011) 7:645–50. doi: 10.7150/ijbs.7.645
136. Jaworska K, Huc T, Samborowska E, Dobrowolski L, Bielinska K, Gawlak M, et al. Hypertension in rats is associated with an increased permeability of the colon to TMA, a gut bacteria metabolite. PloS One. (2017) 12:e0189310. doi: 10.1371/journal.pone.0189310
137. Kim S, Goel R, Kumar A, Qi Y, Lobaton G, Hosaka K, et al. Imbalance of gut microbiome and intestinal epithelial barrier dysfunction in patients with high blood pressure. Clin Sci (Lond). (2018) 132:701–18. doi: 10.1042/CS20180087
138. Luu M, Weigand K, Wedi F, Breidenbend C, Leister H, Pautz S, et al. Regulation of the effector function of CD8+ T cells by gut microbiota-derived metabolite butyrate. Sci Rep. (2018) 8:14430. doi: 10.1038/s41598-018-32860-x
139. Bartolomaeus H, Balogh A, Yakoub M, Homann S, Markó L, Höges S, et al. Short-chain fatty acid propionate protects from hypertensive cardiovascular damage. Circulation. (2019) 139:1407–21. doi: 10.1161/CIRCULATIONAHA.118.036652
140. Kim CH. Control of lymphocyte functions by gut microbiota-derived short-chain fatty acids. Cell Mol Immunol. (2021) 18:1161–71. doi: 10.1038/s41423-020-00625-0
141. Norlander AE, Madhur MS, Harrison DG. The immunology of hypertension. J Exp Med. (2018) 215:21–33. doi: 10.1084/jem.20171773
142. Gill PA, Van Zelm MC, Ffrench RA, Muir JG, Gibson PR. Successful elevation of circulating acetate and propionate by dietary modulation does not alter T-regulatory cell or cytokine profiles in healthy humans: a pilot study. Eur J Nutr. (2020) 59:2651–61. doi: 10.1007/s00394-019-02113-2
143. Skrzypecki J, Żera T, Ufnal M. Butyrate, a gut bacterial metabolite, lowers intraocular pressure in normotensive but not in hypertensive rats. J Glaucoma. (2018) 27:823–7. doi: 10.1097/IJG.0000000000001025
144. Yang T, Magee KL, Colon-Perez LM, Larkin R, Liao Y, Balazic E, et al. Impaired butyrate absorption in the proximal colon, low serum butyrate and diminished central effects of butyrate on blood pressure in spontaneously hypertensive rats. Acta Physiologica. (2019) 226:e13256. doi: 10.1111/apha.2019.226.issue-2
145. Kanneganti T-D, Dixit VD. Immunological complications of obesity. Nat Immunol. (2012) 13:707–12. doi: 10.1038/ni.2343
146. Boutens L, Stienstra R. Adipose tissue macrophages: going off track during obesity. Diabetologia. (2016) 59:879–94. doi: 10.1007/s00125-016-3904-9
147. Johnson AR, Justin Milner J, Makowski L. The inflammation highway: metabolism accelerates inflammatory traffic in obesity. Immunol Rev. (2012) 249:218–38. doi: 10.1111/j.1600-065X.2012.01151.x
148. Boutagy NE, McMillan RP, Frisard MI, Hulver MW. Metabolic endotoxemia with obesity: Is it real and is it relevant? Biochimie. (2016) 124:11–20. doi: 10.1016/j.biochi.2015.06.020
149. Gil A, María Aguilera C, Gil-Campos M, Cañete R. Altered signalling and gene expression associated with the immune system and the inflammatory response in obesity. Br J Nutr. (2007) 98:S121–6. doi: 10.1017/S0007114507838050
150. Catrysse L, van Loo G. Inflammation and the metabolic syndrome: the tissue-specific functions of NF-κB. Trends Cell Biol. (2017) 27:417–29. doi: 10.1016/j.tcb.2017.01.006
151. Forsythe LK, Wallace JMW, Livingstone MBE. Obesity and inflammation: the effects of weight loss. Nutr Res Rev. (2008) 21:117–33. doi: 10.1017/S0954422408138732
152. Wolfe BM, Kvach E, Eckel RH. Treatment of obesity: weight loss and bariatric surgery. Circ Res. (2016) 118:1844–55. doi: 10.1161/CIRCRESAHA.116.307591
153. Askarpour M, Khani D, Sheikhi A, Ghaedi E, Alizadeh S. Effect of bariatric surgery on serum inflammatory factors of obese patients: a systematic review and meta-analysis. Obes Surg. (2019) 29:2631–47. doi: 10.1007/s11695-019-03926-0
154. Al-Lahham SH, Roelofsen H, Priebe M, Weening D, Dijkstra M, Hoek A, et al. Regulation of adipokine production in human adipose tissue by propionic acid. Eur J Clin Invest. (2010) 40:401–7. doi: 10.1111/j.1365-2362.2010.02278.x
155. Janssen AWF, Kersten S. Potential mediators linking gut bacteria to metabolic health: a critical view. J Physiol. (2017) 595:477–87. doi: 10.1113/tjp.2017.595.issue-2
156. Li M, van Esch BCAM, Wagenaar GTM, Garssen J, Folkerts G, Henricks PAJ. Pro- and anti-inflammatory effects of short chain fatty acids on immune and endothelial cells. Eur J Pharmacol. (2018) 831:52–9. doi: 10.1016/j.ejphar.2018.05.003
157. Ang Z, Er JZ, Tan NS, Lu J, Liou Y-C, Grosse J, et al. Human and mouse monocytes display distinct signalling and cytokine profiles upon stimulation with FFAR2/FFAR3 short-chain fatty acid receptor agonists. Sci Rep. (2016) 6:34145. doi: 10.1038/srep34145
158. Jeong Y, Du R, Zhu X, Yin S, Wang J, Cui H, et al. Histone deacetylase isoforms regulate innate immune responses by deacetylating mitogen-activated protein kinase phosphatase-1. J Leukocyte Biol. (2013) 95:651–9. doi: 10.1189/jlb.1013565
159. Schilderink R, Verseijden C, De Jonge WJ. Dietary inhibitors of histone deacetylases in intestinal immunity and homeostasis. Front Immunol. (2013) 4. doi: 10.3389/fimmu.2013.00226
160. Vinolo MAR, Rodrigues HG, Nachbar RT, Curi R. Regulation of inflammation by short chain fatty acids. Nutrients. (2011) 3:858–76. doi: 10.3390/nu3100858
161. Ohira H, Fujioka Y, Katagiri C, Mamoto R, Aoyama-Ishikawa M, Amako K, et al. Butyrate attenuates inflammation and lipolysis generated by the interaction of adipocytes and macrophages. JAT. (2013) 20:425–42. doi: 10.5551/jat.15065
162. Tedelind S, Westberg F, Kjerrulf M, Vidal A. Anti-inflammatory properties of the short-chain fatty acids acetate and propionate: A study with relevance to inflammatory bowel disease. WJG. (2007) 13:2826. doi: 10.3748/wjg.v13.i20.2826
163. Eslick S, Williams EJ, Berthon BS, Wright T, Karihaloo C, Gately M, et al. Weight loss and short-chain fatty acids reduce systemic inflammation in monocytes and adipose tissue macrophages from obese subjects. Nutrients. (2022) 14:765. doi: 10.3390/nu14040765
164. Al-Lahham S, Roelofsen H, Rezaee F, Weening D, Hoek A, Vonk R, et al. Propionic acid affects immune status and metabolism in adipose tissue from overweight subjects. Eur J Clin Invest. (2012) 42:357–64. doi: 10.1111/j.1365-2362.2011.02590.x
165. Albillos A, De Gottardi A, Rescigno M. The gut-liver axis in liver disease: Pathophysiological basis for therapy. J Hepatol. (2020) 72:558–77. doi: 10.1016/j.jhep.2019.10.003
166. Hambruch E, Miyazaki-Anzai S, Hahn U, Matysik S, Boettcher A, Perović-Ottstadt S, et al. Synthetic farnesoid X receptor agonists induce high-density lipoprotein-mediated transhepatic cholesterol efflux in mice and monkeys and prevent atherosclerosis in cholesteryl ester transfer protein transgenic low-density lipoprotein receptor (-/-) mice. J Pharmacol Exp Ther. (2012) 343:556–67. doi: 10.1124/jpet.112.196519
167. Perez R, Stevenson F, Johnson J, Morgan M, Erickson K, Hubbard NE, et al. Sodium butyrate upregulates Kupffer cell PGE2 production and modulates immune function. J Surg Res. (1998) 78:1–6. doi: 10.1006/jsre.1998.5316
168. Demir M, Lang S, Hartmann P, Duan Y, Martin A, Miyamoto Y, et al. The fecal mycobiome in non-alcoholic fatty liver disease. J Hepatol. (2022) 76:788–99. doi: 10.1016/j.jhep.2021.11.029
169. Wree A, McGeough MD, Peña CA, Schlattjan M, Li H, Inzaugarat ME, et al. NLRP3 inflammasome activation is required for fibrosis development in NAFLD. J Mol Med. (2014) 92:1069–82. doi: 10.1007/s00109-014-1170-1
170. Voican CS, Njiké-Nakseu M, Boujedidi H, Barri-Ova N, Bouchet-Delbos L, Agostini H, et al. Alcohol withdrawal alleviates adipose tissue inflammation in patients with alcoholic liver disease. Liver Int. (2015) 35:967–78. doi: 10.1111/liv.2015.35.issue-3
171. Henao-Mejia J, Elinav E, Jin C, Hao L, Mehal WZ, Strowig T, et al. Inflammasome-mediated dysbiosis regulates progression of NAFLD and obesity. Nature. (2012) 482:179–85. doi: 10.1038/nature10809
172. Zheng M, Yang X, Wu Q, Gong Y, Pang N, Ge X, et al. Butyrate attenuates hepatic steatosis induced by a high-fat and fiber-deficient diet via the hepatic GPR41/43-caMKII/HDAC1-CREB pathway. Mol Nutr Food Res. (2023) 67:2200597. doi: 10.1002/mnfr.202200597
173. Kumari A, Pal Pathak D, Asthana S. Bile acids mediated potential functional interaction between FXR and FATP5 in the regulation of Lipid Metabolism. Int J Biol Sci. (2020) 16:2308–22. doi: 10.7150/ijbs.44774
174. Sun W, Dong H, Wolfrum C. Local acetate inhibits brown adipose tissue function. Proc Natl Acad Sci USA. (2021) 118:e2116125118. doi: 10.1073/pnas.2116125118
175. Samuel BS, Shaito A, Motoike T, Rey FE, Backhed F, Manchester JK, et al. Effects of the gut microbiota on host adiposity are modulated by the short-chain fatty-acid binding G protein-coupled receptor, Gpr41. Proc Natl Acad Sci USA. (2008) 105:16767–72. doi: 10.1073/pnas.0808567105
176. Bjursell M, Admyre T, Göransson M, Marley AE, Smith DM, Oscarsson J, et al. Improved glucose control and reduced body fat mass in free fatty acid receptor 2-deficient mice fed a high-fat diet. Am J Physiology-Endocrinology Metab. (2011) 300:E211–20. doi: 10.1152/ajpendo.00229.2010
177. Den Besten G, Lange K, Havinga R, Van Dijk TH, Gerding A, Van Eunen K, et al. Gut-derived short-chain fatty acids are vividly assimilated into host carbohydrates and lipids. Am J Physiology-Gastrointestinal Liver Physiol. (2013) 305:G900–10. doi: 10.1152/ajpgi.00265.2013
178. Rau M, Rehman A, Levels H, Weiß J, Beyersdorf N, Rosenstiel P, et al. Short-chain fatty acids and SCFA-producing bacteria in NAFLD patients are associated with an increased Th17/rTreg ratio and hepatic disease progression. Z Gastroenterol. (2017) 55:e1–e27. doi: 10.1055/s-0037-1603048
179. Rau M, Rehman A, Dittrich M, Groen AK, Hermanns HM, Seyfried F, et al. Fecal SCFAs and SCFA-producing bacteria in gut microbiome of human NAFLD as a putative link to systemic T-cell activation and advanced disease. United Eur Gastroenterol J. (2018) 6:1496–507. doi: 10.1177/2050640618804444
180. Rau M, Schilling A-K, Meertens J, Hering I, Weiss J, Jurowich C, et al. Progression from nonalcoholic fatty liver to nonalcoholic steatohepatitis is marked by a higher frequency of th17 cells in the liver and an increased th17/resting regulatory T cell ratio in peripheral blood and in the liver. J Immunol. (2016) 196:97–105. doi: 10.4049/jimmunol.1501175
181. Gurnell M. PPARγ and metabolism: insights from the study of human genetic variants. Clin Endocrinol. (2003) 59:267–77. doi: 10.1046/j.1365-2265.2003.01767.x
182. Ye J, Lv L, Wu W, Li Y, Shi D, Fang D, et al. Butyrate protects mice against methionine-choline-deficient diet-induced non-alcoholic steatohepatitis by improving gut barrier function, attenuating inflammation and reducing endotoxin levels. Front Microbiol. (2018) 9:1967. doi: 10.3389/fmicb.2018.01967
183. Sun B, Jia Y, Hong J, Sun Q, Gao S, Hu Y, et al. Sodium butyrate ameliorates high-fat-diet-induced non-alcoholic fatty liver disease through peroxisome proliferator-activated receptor α-mediated activation of β Oxidation and suppression of inflammation. J Agric Food Chem. (2018) 66:7633–42. doi: 10.1021/acs.jafc.8b01189
184. Kim M, Lee H-A, Cho H-M, Kang S-H, Lee E, Kim IK. Histone deacetylase inhibition attenuates hepatic steatosis in rats with experimental Cushing’s syndrome. Korean J Physiol Pharmacol. (2018) 22:23. doi: 10.4196/kjpp.2018.22.1.23
185. Tian P, Yang W, Guo X, Wang T, Tan S, Sun R, et al. Early life gut microbiota sustains liver-resident natural killer cells maturation via the butyrate-IL-18 axis. Nat Commun. (2023) 14:1710. doi: 10.1038/s41467-023-37419-7
186. Chambers ES, Preston T, Frost G, Morrison DJ. Role of gut microbiota-generated short-chain fatty acids in metabolic and cardiovascular health. Curr Nutr Rep. (2018) 7:198–206. doi: 10.1007/s13668-018-0248-8
187. Li M, Van Esch BCAM, Henricks PAJ, Garssen J, Folkerts G. Time and concentration dependent effects of short chain fatty acids on lipopolysaccharide- or tumor necrosis factor α-induced endothelial activation. Front Pharmacol. (2018) 9:233. doi: 10.3389/fphar.2018.00233
188. Glauben R, Siegmund B. Inhibition of histone deacetylases in inflammatory bowel diseases. Mol Med. (2011) 17:426–33. doi: 10.2119/molmed.2011.00069
189. Lührs H, Gerke T, Müller JG, Melcher R, Schauber J, Boxberger F, et al. Butyrate inhibits NF-κB activation in lamina propria macrophages of patients with ulcerative colitis. Scandinavian J Gastroenterol. (2002) 37:458–66. doi: 10.1080/003655202317316105
190. Zaki MH, Boyd KL, Vogel P, Kastan MB, Lamkanfi M, Kanneganti T-D. The NLRP3 inflammasome protects against loss of epithelial integrity and mortality during experimental colitis. Immunity. (2010) 32:379–91. doi: 10.1016/j.immuni.2010.03.003
191. Huang N, Katz JP, Martin DR, Wu GD. Inhibition of IL-8 gene expression in Caco-2 cells by compounds which induce histone hyperacetylation. Cytokine. (1997) 9:27–36. doi: 10.1006/cyto.1996.0132
192. Atarashi K, Tanoue T, Oshima K, Suda W, Nagano Y, Nishikawa H, et al. Treg induction by a rationally selected mixture of Clostridia strains from the human microbiota. Nature. (2013) 500:232–6. doi: 10.1038/nature12331
193. Slavin J. Fiber and prebiotics: mechanisms and health benefits. Nutrients. (2013) 5:1417–35. doi: 10.3390/nu5041417
194. Cobo ER, Kissoon-Singh V, Moreau F, Holani R, Chadee K. MUC2 mucin and butyrate contribute to the synthesis of the antimicrobial peptide cathelicidin in response to entamoeba histolytica- and dextran sodium sulfate-induced colitis. Infect Immun. (2017) 85:e00905–16. doi: 10.1128/IAI.00905-16
195. Olaniyi KS, Areloegbe SE, Fiemotongha FE. Cardiac energy depletion in a rat model of polycystic ovarian syndrome is reversed by acetate and associated with inhibitory effect of HDAC2/mTOR. Eur J Pharmacol. (2024) 962:176243. doi: 10.1016/j.ejphar.2023.176243
196. Olaniyi KS, Areloegbe SE. Suppression of PCSK9/NF-kB-dependent pathways by acetate ameliorates cardiac inflammation in a rat model of polycystic ovarian syndrome. Life Sci. (2022) 300:120560. doi: 10.1016/j.lfs.2022.120560
197. Chan KL, Cathomas F, Russo SJ. Central and peripheral inflammation link metabolic syndrome and major depressive disorder. Physiol (Bethesda). (2019) 34:123–33. doi: 10.1152/physiol.00047.2018
198. Farzi A, Fröhlich EE, Holzer P. Gut microbiota and the neuroendocrine system. Neurotherapeutics. (2018) 15:5–22. doi: 10.1007/s13311-017-0600-5
199. Needham BD, Funabashi M, Adame MD, Wang Z, Boktor JC, Haney J, et al. A gut-derived metabolite alters brain activity and anxiety behaviour in mice. Nature. (2022) 602:647–53. doi: 10.1038/s41586-022-04396-8
200. Turna J, Grosman Kaplan K, Anglin R, Patterson B, Soreni N, et al. The gut microbiome and inflammation in obsessive-compulsive disorder patients compared to age- and sex-matched controls: a pilot study. Acta Psychiatr Scand. (2020) 142:337–47. doi: 10.1111/acps.v142.4
201. Cheng J, Hu H, Ju Y, Liu J, Wang M, Liu B, et al. Gut microbiota-derived short-chain fatty acids and depression: deep insight into biological mechanisms and potential applications. Gen Psych. (2024) 37:e101374. doi: 10.1136/gpsych-2023-101374
202. Goldsmith DR, Rapaport MH, Miller BJ. A meta-analysis of blood cytokine network alterations in psychiatric patients: comparisons between schizophrenia, bipolar disorder and depression. Mol Psychiatry. (2016) 21:1696–709. doi: 10.1038/mp.2016.3
203. Beurel E, Lowell JA. Th17 cells in depression. Brain Behavior Immun. (2018) 69:28–34. doi: 10.1016/j.bbi.2017.08.001
204. Wachholz S, Eßlinger M, Plümper J, Manitz M-P, Juckel G, Friebe A. Microglia activation is associated with IFN-α induced depressive-like behavior. Brain Behavior Immun. (2016) 55:105–13. doi: 10.1016/j.bbi.2015.09.016
205. Liu Z, Qiu A-W, Huang Y, Yang Y, Chen J-N, Gu T-T, et al. IL-17A exacerbates neuroinflammation and neurodegeneration by activating microglia in rodent models of Parkinson’s disease. Brain Behavior Immun. (2019) 81:630–45. doi: 10.1016/j.bbi.2019.07.026
206. Wu J, Li J, Gaurav C, Muhammad U, Chen Y, Li X, et al. CUMS and dexamethasone induce depression-like phenotypes in mice by differentially altering gut microbiota and triggering macroglia activation. Gen Psych. (2021) 34:e100529. doi: 10.1136/gpsych-2021-100529
207. Wang H, He Y, Sun Z, Ren S, Liu M, Wang G, et al. Microglia in depression: an overview of microglia in the pathogenesis and treatment of depression. J Neuroinflamm. (2022) 19:132. doi: 10.1186/s12974-022-02492-0
208. Xiao W, Su J, Gao X, Yang H, Weng R, Ni W, et al. The microbiota-gut-brain axis participates in chronic cerebral hypoperfusion by disrupting the metabolism of short-chain fatty acids. Microbiome. (2022) 10:62. doi: 10.1186/s40168-022-01255-6
209. Li J-M, Yu R, Zhang L-P, Wen S-Y, Wang S-J, Zhang X-Y, et al. Dietary fructose-induced gut dysbiosis promotes mouse hippocampal neuroinflammation: a benefit of short-chain fatty acids. Microbiome. (2019) 7:98. doi: 10.1186/s40168-019-0713-7
210. Hoyles L, Snelling T, Umlai U-K, Nicholson JK, Carding SR, Glen RC, et al. Microbiome–host systems interactions: protective effects of propionate upon the blood–brain barrier. Microbiome. (2018) 6:55. doi: 10.1186/s40168-018-0439-y
211. Aho VTE, Houser MC, Pereira PAB, Chang J, Rudi K, Paulin L, et al. Relationships of gut microbiota, short-chain fatty acids, inflammation, and the gut barrier in Parkinson’s disease. Mol Neurodegeneration. (2021) 16:6. doi: 10.1186/s13024-021-00427-6
212. Yang W, Yu T, Huang X, Bilotta AJ, Xu L, Lu Y, et al. Intestinal microbiota-derived short-chain fatty acids regulation of immune cell IL-22 production and gut immunity. Nat Commun. (2020) 11:4457. doi: 10.1038/s41467-020-18262-6
213. Erny D, Hrabě De Angelis AL, Jaitin D, Wieghofer P, Staszewski O, David E, et al. Host microbiota constantly control maturation and function of microglia in the CNS. Nat Neurosci. (2015) 18:965–77. doi: 10.1038/nn.4030
214. Fu S-P, Wang J-F, Xue W-J, Liu H-M, Liu B, Zeng Y-L, et al. Anti-inflammatory effects of BHBA in both in vivo and in vitro Parkinson’s disease models are mediated by GPR109A-dependent mechanisms. J Neuroinflamm. (2015) 12:9. doi: 10.1186/s12974-014-0230-3
215. Wang P, Zhang Y, Gong Y, Yang R, Chen Z, Hu W, et al. Sodium butyrate triggers a functional elongation of microglial process via Akt-small RhoGTPase activation and HDACs inhibition. Neurobiol Dis. (2018) 111:12–25. doi: 10.1016/j.nbd.2017.12.006
216. Su S-H, Chen M, Wu Y-F, Lin Q, Wang D-P, Sun J, et al. Fecal microbiota transplantation and short-chain fatty acids protected against cognitive dysfunction in a rat model of chronic cerebral hypoperfusion. CNS Neurosci Ther. (2023) 29 Suppl:1, 98–114. doi: 10.1111/cns.14089
217. Rostamtabar M, Esmaeilzadeh S, Tourani M, Rahmani A, Baee M, Shirafkan F, et al. Pathophysiological roles of chronic low-grade inflammation mediators in polycystic ovary syndrome. J Cell Physiol. (2021) 236:824–38. doi: 10.1002/jcp.v236.2
218. Popovic M, Sartorius G, Christ-Crain M. Chronic low-grade inflammation in polycystic ovary syndrome: is there a (patho)-physiological role for interleukin-1? Semin Immunopathol. (2019) 41:447–59. doi: 10.1007/s00281-019-00737-4
219. Zhai Y, Pang Y. Systemic and ovarian inflammation in women with polycystic ovary syndrome. J Reprod Immunol. (2022) 151:103628. doi: 10.1016/j.jri.2022.103628
220. Cirillo F, Catellani C, Lazzeroni P, Sartori C, Nicoli A, Amarri S, et al. MiRNAs regulating insulin sensitivity are dysregulated in polycystic ovary syndrome (PCOS) ovaries and are associated with markers of inflammation and insulin sensitivity. Front Endocrinol (Lausanne). (2019) 10:879. doi: 10.3389/fendo.2019.00879
221. Ou M, Xu P, Lin H, Ma K, Liu M. AMH is a good predictor of metabolic risk in women with PCOS: A cross-sectional study. Int J Endocrinol. (2021) 2021:9511772. doi: 10.1155/2021/9511772
222. Margină D, Ungurianu A, Purdel C, Tsoukalas D, Sarandi E, Thanasoula M, et al. Chronic inflammation in the context of everyday life: dietary changes as mitigating factors. IJERPH. (2020) 17:4135. doi: 10.3390/ijerph17114135
223. Olaniyi KS, Areloegbe SE. Acetate ameliorates ovarian mitochondrial dysfunction in letrozole-induced polycystic ovarian syndrome rat model by improving mitofusin-2. J Physiol Sci. (2024) 74:22. doi: 10.1186/s12576-024-00908-5
224. Olaniyi KS, Areloegbe SE, Oyeleke MB. Acetate restores hypothalamic-adipose kisspeptin status in a rat model of PCOS by suppression of NLRP3 immunoreactivity. Endocrine. (2022) 78:628–40. doi: 10.1007/s12020-022-03191-9
225. Eepho O-I, Bashir A-AM, Oniyide AA, Aturamu A, Owolabi OV, Ajadi IO, et al. Modulation of GABA by sodium butyrate ameliorates hypothalamic inflammation in experimental model of PCOS. BMC Neurosci. (2023) 24:62. doi: 10.1186/s12868-023-00834-z
226. Jiang X, Liu B, Nie Z, Duan L, Xiong Q, Jin Z, et al. The role of m6A modification in the biological functions and diseases. Signal Transduct Target Ther. (2021) 6:74. doi: 10.1038/s41392-020-00450-x
227. Luo J, Xu T, Sun K. N6-methyladenosine RNA modification in inflammation: roles, mechanisms, and applications. Front Cell Dev Biol. (2021) 9:670711. doi: 10.3389/fcell.2021.670711
228. Wen L, Sun W, Xia D, Wang Y, Li J, Yang S. The m6A methyltransferase METTL3 promotes LPS-induced microglia inflammation through TRAF6/NF-κB pathway. Neuroreport. (2022) 33:243–51. doi: 10.1097/WNR.0000000000001550
229. Renoux F, Stellato M, Haftmann C, Vogetseder A, Huang R, Subramaniam A, et al. The AP1 transcription factor fosl2 promotes systemic autoimmunity and inflammation by repressing treg development. Cell Rep. (2020) 31:107826. doi: 10.1016/j.celrep.2020.107826
230. Chen S, Zhang L, Li M, Zhang Y, Sun M, Wang L, et al. Fusobacterium nucleatum reduces METTL3-mediated m6A modification and contributes to colorectal cancer metastasis. Nat Commun. (2022) 13:1248. doi: 10.1038/s41467-022-28913-5
231. Dai L-N, Yan J-K, Xiao Y-T, Wen J, Zhang T, Zhou K-J, et al. Butyrate stimulates the growth of human intestinal smooth muscle cells by activation of yes-associated protein. J Cell Physiol. (2018) 233:3119–28. doi: 10.1002/jcp.v233.4
232. Singh V, Sadler R, Heindl S, Llovera G, Roth S, Benakis C, et al. The gut microbiome primes a cerebroprotective immune response after stroke. J Cereb Blood Flow Metab. (2018) 38:1293–8. doi: 10.1177/0271678X18780130
233. Singh V, Roth S, Llovera G, Sadler R, Garzetti D, Stecher B, et al. Microbiota dysbiosis controls the neuroinflammatory response after stroke. J Neurosci. (2016) 36:7428–40. doi: 10.1523/JNEUROSCI.1114-16.2016
234. Chidambaram SB, Rathipriya AG, Mahalakshmi AM, Sharma S, Hediyal TA, Ray B, et al. The influence of gut dysbiosis in the pathogenesis and management of ischemic stroke. Cells. (2022) 11:1239. doi: 10.3390/cells11071239
235. Fang Z, Chen M, Qian J, Wang C, Zhang J. The bridge between ischemic stroke and gut microbes: short-chain fatty acids. Cell Mol Neurobiol. (2023) 43:543–59. doi: 10.1007/s10571-022-01209-4
236. Yamashiro K, Kurita N, Urabe T, Hattori N. Role of the gut microbiota in stroke pathogenesis and potential therapeutic implications. Ann Nutr Metab. (2021) 77 Suppl:2, 36–44. doi: 10.1159/000516398
237. Zeng X, Gao X, Peng Y, Wu Q, Zhu J, Tan C, et al. Higher risk of stroke is correlated with increased opportunistic pathogen load and reduced levels of butyrate-producing bacteria in the gut. Front Cell Infect Microbiol. (2019) 9:4. doi: 10.3389/fcimb.2019.00004
238. Haghikia A, Jörg S, Duscha A, Berg J, Manzel A, Waschbisch A, et al. Dietary fatty acids directly impact central nervous system autoimmunity via the small intestine. Immunity. (2015) 43:817–29. doi: 10.1016/j.immuni.2015.09.007
239. Chen R, Xu Y, Wu P, Zhou H, Lasanajak Y, Fang Y, et al. Transplantation of fecal microbiota rich in short chain fatty acids and butyric acid treat cerebral ischemic stroke by regulating gut microbiota. Pharmacol Res. (2019) 148:104403. doi: 10.1016/j.phrs.2019.104403
240. Peh A, O’Donnell JA, Broughton BRS, Marques FZ. Gut microbiota and their metabolites in stroke: A double-edged sword. Stroke. (2022) 53:1788–801. doi: 10.1161/STROKEAHA.121.036800
241. Chen Z, Xin L, Yang L, Xu M, Li F, Zhou M, et al. Butyrate promotes post-stroke outcomes in aged mice via interleukin-22. Exp Neurol. (2023) 363:114351. doi: 10.1016/j.expneurol.2023.114351
242. Park MJ, Sohrabji F. The histone deacetylase inhibitor, sodium butyrate, exhibits neuroprotective effects for ischemic stroke in middle-aged female rats. J Neuroinflamm. (2016) 13:300. doi: 10.1186/s12974-016-0765-6
243. Zhou Z, Xu N, Matei N, McBride DW, Ding Y, Liang H, et al. Sodium butyrate attenuated neuronal apoptosis via GPR41/Gβγ/PI3K/Akt pathway after MCAO in rats. J Cereb Blood Flow Metab. (2021) 41:267–81. doi: 10.1177/0271678X20910533
244. Sadler R, Cramer JV, Heindl S, Kostidis S, Betz D, Zuurbier KR, et al. Short-chain fatty acids improve poststroke recovery via immunological mechanisms. J Neurosci. (2020) 40:1162–73. doi: 10.1523/JNEUROSCI.1359-19.2019
245. Lee J, d’Aigle J, Atadja L, Quaicoe V, Honarpisheh P, Ganesh BP, et al. Gut microbiota-derived short-chain fatty acids promote poststroke recovery in aged mice. Circ Res. (2020) 127:453–65. doi: 10.1161/CIRCRESAHA.119.316448
246. Uhlig HH, Powrie F. Translating immunology into therapeutic concepts for inflammatory bowel disease. Annu Rev Immunol. (2018) 36:755–81. doi: 10.1146/annurev-immunol-042617-053055
247. Machiels K, Joossens M, Sabino J, De Preter V, Arijs I, Eeckhaut V, et al. A decrease of the butyrate-producing species Roseburia hominis and Faecalibacterium prausnitzii defines dysbiosis in patients with ulcerative colitis. Gut. (2014) 63:1275–83. doi: 10.1136/gutjnl-2013-304833
248. Yilmaz B, Juillerat P, Øyås O, Ramon C, Bravo FD, Franc Y, et al. Microbial network disturbances in relapsing refractory Crohn’s disease. Nat Med. (2019) 25:323–36. doi: 10.1038/s41591-018-0308-z
249. Ferrer-Picón E, Dotti I, Corraliza AM, Mayorgas A, Esteller M, Perales JC, et al. Intestinal inflammation modulates the epithelial response to butyrate in patients with inflammatory bowel disease. Inflammation Bowel Dis. (2020) 26:43–55. doi: 10.1093/ibd/izz119
250. Imhann F, Vich Vila A, Bonder MJ, Fu J, Gevers D, Visschedijk MC, et al. Interplay of host genetics and gut microbiota underlying the onset and clinical presentation of inflammatory bowel disease. Gut. (2018) 67:108–19. doi: 10.1136/gutjnl-2016-312135
251. Zhou L, Zhang M, Wang Y, Dorfman RG, Liu H, Yu T, et al. Faecalibacterium prausnitzii produces butyrate to maintain th17/treg balance and to ameliorate colorectal colitis by inhibiting histone deacetylase 1. Inflammation Bowel Dis. (2018) 24:1926–40. doi: 10.1093/ibd/izy182
252. Shen H, Lu Z, Chen Z, Wu Y, Shen Z. Rapid fermentable substance modulates interactions between ruminal commensals and toll-like receptors in promotion of immune tolerance of goat rumen. Front Microbiol. (2016) 7. doi: 10.3389/fmicb.2016.01812
253. Rösch C, Taverne N, Venema K, Gruppen H, Wells JM, Schols HA. Effects of in vitro fermentation of barley β-glucan and sugar beet pectin using human fecal inocula on cytokine expression by dendritic cells. Mol Nutr Food Res. (2017) 61:1600243. doi: 10.1002/mnfr.201600243
254. Kazemi Sefat NA, Mohammadi MM, Hadjati J, Talebi S, Ajami M, Daneshvar H. Sodium butyrate as a histone deacetylase inhibitor affects toll-like receptor 4 expression in colorectal cancer cell lines. Immunol Investigations. (2019) 48:759–69. doi: 10.1080/08820139.2019.1595643
255. Kovarik JJ, Tillinger W, Hofer J, Hölzl MA, Heinzl H, Saemann MD, et al. Impaired anti-inflammatory efficacy of n-butyrate in patients with IBD: ANTI-INFLAMMATORY EFFICACY OF N- BUTYRATE. Eur J Clin Invest. (2011) 41:291–8. doi: 10.1111/j.1365-2362.2010.02407.x
256. Sam QH, Ling H, Yew WS, Tan Z, Ravikumar S, Chang MW, et al. The divergent immunomodulatory effects of short chain fatty acids and medium chain fatty acids. IJMS. (2021) 22:6453. doi: 10.3390/ijms22126453
257. Macia L, Tan J, Vieira AT, Leach K, Stanley D, Luong S, et al. Metabolite-sensing receptors GPR43 and GPR109A facilitate dietary fibre-induced gut homeostasis through regulation of the inflammasome. Nat Commun. (2015) 6:6734. doi: 10.1038/ncomms7734
258. Mark-Christensen A, Lange A, Erichsen R, Frøslev T, Esen BÖ, Sørensen HT, et al. Early-life exposure to antibiotics and risk for crohn’s disease: A nationwide danish birth cohort study. Inflammation Bowel Dis. (2022) 28:415–22. doi: 10.1093/ibd/izab085
259. Scott NA, Andrusaite A, Andersen P, Lawson M, Alcon-Giner C, Leclaire C, et al. Antibiotics induce sustained dysregulation of intestinal T cell immunity by perturbing macrophage homeostasis. Sci Transl Med. (2018) 10:eaao4755. doi: 10.1126/scitranslmed.aao4755
260. Breuer RI, Soergel KH, Lashner BA, Christ ML, Hanauer SB, Vanagunas A, et al. Short chain fatty acid rectal irrigation for left-sided ulcerative colitis: a randomised, placebo controlled trial. Gut. (1997) 40:485–91. doi: 10.1136/gut.40.4.485
261. Hamer HM, Jonkers DMAE, Vanhoutvin SALW, Troost FJ, Rijkers G, de Bruïne A, et al. Effect of butyrate enemas on inflammation and antioxidant status in the colonic mucosa of patients with ulcerative colitis in remission. Clin Nutr. (2010) 29:738–44. doi: 10.1016/j.clnu.2010.04.002
262. Facchin S, Vitulo N, Calgaro M, Buda A, Romualdi C, Pohl D, et al. Microbiota changes induced by microencapsulated sodium butyrate in patients with inflammatory bowel disease. Neurogastroenterol Motil. (2020) 32:e13914. doi: 10.1111/nmo.v32.10
263. Gerasimidis K, Bertz M, Hanske L, Junick J, Biskou O, Aguilera M, et al. Decline in presumptively protective gut bacterial species and metabolites are paradoxically associated with disease improvement in pediatric Crohn’s disease during enteral nutrition. Inflammation Bowel Dis. (2014) 20:861–71. doi: 10.1097/MIB.0000000000000023
264. Svolos V, Hansen R, Nichols B, Quince C, Ijaz UZ, Papadopoulou RT, et al. Treatment of active crohn’s disease with an ordinary food-based diet that replicates exclusive enteral nutrition. Gastroenterology. (2019) 156:1354–1367.e6. doi: 10.1053/j.gastro.2018.12.002
265. van der Beek CM, Bloemen JG, van den Broek MA, Lenaerts K, Venema K, Buurman WA, et al. Hepatic uptake of rectally administered butyrate prevents an increase in systemic butyrate concentrations in humans. J Nutr. (2015) 145:2019–24. doi: 10.3945/jn.115.211193
266. Mallick H, Franzosa EA, Mclver LJ, Banerjee S, Sirota-Madi A, Kostic AD, et al. Predictive metabolomic profiling of microbial communities using amplicon or metagenomic sequences. Nat Commun. (2019) 10:3136. doi: 10.1038/s41467-019-10927-1
267. Park Y-T, Kim T, Ham J, Choi J, Lee H-S, Yeon YJ, et al. Physiological activity of E. coli engineered to produce butyric acid. Microb Biotechnol. (2022) 15:832–43. doi: 10.1111/1751-7915.13795
268. Zhang T, Zhang J, Duan L. The role of genetically engineered probiotics for treatment of inflammatory bowel disease: A systematic review. Nutrients. (2023) 15:1566. doi: 10.3390/nu15071566
269. De Filippis F, Pellegrini N, Vannini L, Jeffery IB, La Storia A, Laghi L, et al. High-level adherence to a Mediterranean diet beneficially impacts the gut microbiota and associated metabolome. Gut. (2016) 65:1812–21. doi: 10.1136/gutjnl-2015-309957
270. Sobh M, Montroy J, Daham Z, Sibbald S, Lalu M, Stintzi A, et al. Tolerability and SCFA production after resistant starch supplementation in humans: a systematic review of randomized controlled studies. Am J Clin Nutr. (2022) 115:608–18. doi: 10.1093/ajcn/nqab402
271. Gong H, Yuan Q, Du M, Mao X. Polar lipid-enriched milk fat globule membrane supplementation in maternal high-fat diet promotes intestinal barrier function and modulates gut microbiota in male offspring. Food Funct. (2023) 14:10204–20. doi: 10.1039/D2FO04026C
272. Liu X, Li X, Xia B, Jin X, Zou Q, Zeng Z, et al. High-fiber diet mitigates maternal obesity-induced cognitive and social dysfunction in the offspring via gut-brain axis. Cell Metab. (2021) 33:923–938.e6. doi: 10.1016/j.cmet.2021.02.002
273. Nakajima A, Kaga N, Nakanishi Y, Ohno H, Miyamoto J, Kimura I, et al. Maternal high fiber diet during pregnancy and lactation influences regulatory T cell differentiation in offspring in mice. J Immunol. (2017) 199:3516–24. doi: 10.4049/jimmunol.1700248
274. Henrick BM, Rodriguez L, Lakshmikanth T, Pou C, Henckel E, Arzoomand A, et al. Bifidobacteria-mediated immune system imprinting early in life. Cell. (2021) 184:3884–3898.e11. doi: 10.1016/j.cell.2021.05.030
275. Metsälä J, Lundqvist A, Virta LJ, Kaila M, Gissler M, Virtanen SM. Prenatal and post-natal exposure to antibiotics and risk of asthma in childhood. Clin Exp Allergy. (2015) 45:137–45. doi: 10.1111/cea.12356
276. Tang S, Dong X, Ma Y, Zhou H, He Y, Ren D, et al. Highly crystalline cellulose microparticles from dealginated seaweed waste ameliorate high fat-sugar diet-induced hyperlipidemia in mice by modulating gut microbiota. Int J Biol Macromol. (2024) 263:130485. doi: 10.1016/j.ijbiomac.2024.130485
277. Shen F, Zhuang J, Wang Q, Zhang J, Huang Y, Mo Q, et al. Enhancement in the metabolic profile of sea buckthorn juice via fermentation for its better efficacy on attenuating diet-induced metabolic syndrome by targeting gut microbiota. Food Res Int. (2022) 162:111948. doi: 10.1016/j.foodres.2022.111948
278. Liu K, He X, Huang J, Yu S, Cui M, Gao M, et al. Short-chain fatty acid-butyric acid ameliorates granulosa cells inflammation through regulating METTL3-mediated N6-methyladenosine modification of FOSL2 in polycystic ovarian syndrome. Clin Epigenet. (2023) 15:86. doi: 10.1186/s13148-023-01487-9
279. Guo J, Li M, Zhao Y, Kang S-G, Huang K, Tong T. Dietary supplementation of cedryl acetate ameliorates adiposity and improves glucose homeostasis in high-fat diet-fed mice. Nutrients. (2023) 15:980. doi: 10.3390/nu15040980
280. Liu J, Ding H, Yan C, He Z, Zhu H, Ma KY. Effect of tea catechins on gut microbiota in high fat diet-induced obese mice. J Sci Food Agric. (2023) 103:2436–45. doi: 10.1002/jsfa.v103.5
281. Du J, Zhang P, Luo J, Shen L, Zhang S, Gu H, et al. Dietary betaine prevents obesity through gut microbiota-drived microRNA-378a family. Gut Microbes. (2021) 13:1–19. doi: 10.1080/19490976.2020.1862612
282. Hu B, Ye C, Leung EL-H, Zhu L, Hu H, Zhang Z, et al. Bletilla striata oligosaccharides improve metabolic syndrome through modulation of gut microbiota and intestinal metabolites in high fat diet-fed mice. Pharmacol Res. (2020) 159:104942. doi: 10.1016/j.phrs.2020.104942
Keywords: short chain fatty acids, metabolic syndrome, inflammation, immunity, complications
Citation: Yu W, Sun S, Yan Y, Zhou H, Liu Z and Fu Q (2025) The role of short-chain fatty acid in metabolic syndrome and its complications: focusing on immunity and inflammation. Front. Immunol. 16:1519925. doi: 10.3389/fimmu.2025.1519925
Received: 30 October 2024; Accepted: 09 January 2025;
Published: 07 February 2025.
Edited by:
Christoph Reinhardt, Johannes Gutenberg University Mainz, GermanyReviewed by:
Minakshi Rana, Hospital for Special Surgery, United StatesCopyright © 2025 Yu, Sun, Yan, Zhou, Liu and Fu. This is an open-access article distributed under the terms of the Creative Commons Attribution License (CC BY). The use, distribution or reproduction in other forums is permitted, provided the original author(s) and the copyright owner(s) are credited and that the original publication in this journal is cited, in accordance with accepted academic practice. No use, distribution or reproduction is permitted which does not comply with these terms.
*Correspondence: Qiang Fu, MTM2OTMzMzIwNTlAMTYzLmNvbQ==
†These authors have contributed equally to this work and share first authorship
Disclaimer: All claims expressed in this article are solely those of the authors and do not necessarily represent those of their affiliated organizations, or those of the publisher, the editors and the reviewers. Any product that may be evaluated in this article or claim that may be made by its manufacturer is not guaranteed or endorsed by the publisher.
Research integrity at Frontiers
Learn more about the work of our research integrity team to safeguard the quality of each article we publish.