- 1Department of Human Medicine, School of Medicine and Health Sciences, Carl von Ossietzky Universität Oldenburg and University Hospital for General and Visceral Surgery, Oldenburg, Germany
- 2School of Psychology and Life Sciences, Canterbury Christ Church University, Canterbury, United Kingdom
- 3Section of HPB Surgery and Liver Transplantation, Department of Surgery, University Medical Center Groningen, University of Groningen, Groningen, Netherlands
Pancreatic ductal adenocarcinoma (PDAC) remains one of the most lethal malignancies, characterized by an extremely poor prognosis and limited therapeutic options. Central to the progression and immune evasion of PDAC is the tumor (immune) microenvironment (TIME), where immune checkpoint proteins such as galectin-9 (Gal-9) play pivotal roles. Gal-9 significantly contributes to the immunosuppressive milieu of PDAC by interacting with various immune cells, such as T cells, macrophages, and myeloid-derived suppressor cells (MDSCs). These interactions suppress anti-tumor immunity, thus facilitating tumor growth and metastasis. This review comprehensively examines the multifaceted role of Gal-9 in the TIME of PDAC, detailing its mechanisms of action, including the induction of regulatory T cells, polarization of tumor-associated macrophages, and modulation of apoptotic pathways via Tim-3 and caspase activation. The therapeutic potential of targeting Gal-9, either alone or in combination with other immune checkpoint inhibitors such as anti-PD-L1, is also discussed, highlighting preclinical findings that suggest promising avenues for enhancing anti-tumor immune responses. By elucidating the complex biological activities of Gal-9 and its interactions within the TIME, this review underscores the importance of innovative therapeutic strategies aimed at mitigating the immunosuppressive effects of Gal-9 in PDAC.
1 Background
1.1 Pancreatic ductal adenocarcinoma
Pancreatic ductal adenocarcinoma (PDAC) is a significant global health burden, being the third leading cause of cancer-related deaths in the United States with continued increase in incidence and mortality. On a global scale, data from 2022 also reflect the pervasive impact of PDAC – being the 6th leading cause of cancer death, emphasizing the need for enhanced diagnostic and therapeutic strategies. According to recent statistics on PDAC, the five-year overall survival rate for PDAC in the USA is currently only 13%, reflecting the aggressive nature and late-stage diagnosis common with this cancer. For 2024, it is projected that there will be approximately 66,440 new cases of PDAC in the USA, with an estimated 51,750 deaths attributed to the disease. These figures underscore the critical need for advancements in early detection and treatment strategies (1). The prognosis for PDAC is particularly poor, especially in cases where the disease has metastasized. At the time of initial diagnosis, approximately 50% of PDAC patients present with synchronous distant metastases. A recent study analyzed data from the SEER database and confirmed that, at the initial diagnosis, many PDAC patients present with metastases, primarily in the liver which significantly impacts their prognosis. These patients have a drastically reduced overall survival rate, often less than 6 months, compared to those without metastases, whose median survival can extend beyond a year.
1.2 Galectin-9
Galectin-9 (Gal-9) is a 36 kDa protein with its two carbohydrate recognition domains (CRDs), connected by an unstructured linker peptide (2, 3). These CRDs form glycan-binding pockets, which confer different affinities and biological activities depending on their glycan partners (3, 4). The structural specificity of these CRDs is crucial, as variations significantly influence receptor recognition, signaling pathways, and cell death mechanisms in immune responses (4, 5). In normal physiology, Gal-9 is involved in various cellular functions, including cell aggregation, adhesion, and apoptosis (6). Gal-9 is expressed in various cell types, including T cells, fibroblasts, and activated endothelial cells, and is notably present in tumor tissues, where it can contribute to tumor progression and immune evasion (3).
1.3 Aims of this review
This review aims to provide a comprehensive analysis of the TIME in PDAC and the roles of Gal-9 within this context. PDAC is known for its poor prognosis and resistance to conventional therapies, largely due to its complex TIME, which includes various immune cells, cytokines, cancer-associated fibroblasts (CAFs), and the extracellular matrix. Understanding the interactions and signaling pathways within this microenvironment is crucial for identifying new therapeutic targets. This review will detail the structure and function of Gal-9, highlighting its dual role in immune modulation and tumor pathogenesis, and its specific contributions to PDAC and other cancers. Additionally, the review will explore the potential of Gal-9 as a therapeutic target in immunotherapy, addressing current challenges and the promise of combination strategies to enhance treatment efficacy and overcome resistance. By integrating insights into PDAC biology, Gal-9 functions, and immunotherapeutic approaches, this review seeks to offer a detailed understanding of potential advancements in PDAC treatment.
2 Expression of Gal-9 in PDAC is dependent on tumor stage
Gal-9 has been extensively studied in the context of pancreatic cancer, but its role at different stages of the disease remains unclear, largely due to the challenges associated with late diagnosis (7). Histopathological studies have shown that Gal-9 is highly expressed in aggressive tumors (8, 9). In muscle-invasive bladder cancer, infiltration with Gal-9-expressing tumor-associated macrophages (TAMs) correlated with increased Treg cell numbers and poorer survival outcomes. In PDAC Gal-9 expression is significantly elevated in the tumor compared to normal tissue. Human cancer cells, particularly from aggressive tumors like PDAC, secrete Gal-9 in response to interactions with T lymphocytes, which leads to suppression of the immune response through mechanisms such as inhibition of T cell function and macrophage programming.
The serum levels of Gal-9 have been found to be a potential biomarker for PDAC. Elevated levels of Gal-9 in the serum can distinguish PDAC from healthy tissue and are prognostic for advanced stages of the disease (10, 11). Recent research has shown that Gal-9 expression and Treg levels increase in both the blood and pancreas of KC mice in correlation with the progression of precancerous lesions. In addition, Gal-9 expression was highly expressed in the early metaplastic stage in pancreatic intraepithelial neoplasm (PanIN), highlighting a potential role for Gal-9 in the early stages of PDAC. During these early stages, Gal-9 may facilitate immune evasion by recruiting regulatory T cells (Tregs) and suppressing anti-tumor immune responses (8). Gal-9’s interaction with the extracellular matrix (ECM) also plays a significant role in shaping the TME, influencing cell adhesion, migration, and signaling pathways that support tumor growth and metastasis (12, 13). As pancreatic ductal adenocarcinoma (PDAC) progresses, Gal-9 plays a critical role in establishing an immunosuppressive tumor microenvironment by driving macrophage polarization toward a protumoral M2 phenotype and suppressing cytotoxic T-cell activity. Furthermore, Gal-9 expression on blood-derived CD4+ and CD8+ T cells has been found to correlate with tumor size, lymph node metastasis, and UICC stage. Particularly, patients with larger tumor sizes exhibit significantly higher Gal-9 expression levels on CD4+ T cells (11). This makes Gal-9 not only a crucial player in the TME of PDAC but also a valuable marker for diagnosis and prognosis.
Moreover, expression and function of Gal-9 are dynamically regulated in response to various physiological stimuli. For example, endothelial cells can alter their expression of Gal-9 upon activation, which is significant in the context of tumor vasculature and angiogenesis (14). Such regulation ensures that Gal-9 can adapt to the changing microenvironment, thereby sustaining its role in immune modulation and tumor progression. Understanding the precise molecular structure and function of Gal-9, alongside its cellular localization, is critical for developing targeted therapies that can modulate its activity in cancer and other diseases.
3 Role of Galectin-9 in the tumor immune microenvironment of PDAC
TIME in PDAC is a complex and dynamic system comprising various immune cells and cytokines that play crucial roles in tumor progression, immune evasion, and therapy resistance. Effector T cells are notoriously inactive in PDAC. Instead, key components include TAMs, MDSCs, and Tregs shaping an immunosuppressive TIME. TAMs display high cellular plasticity that can be broadly categorized into two subtypes: M1 and M2 macrophages. M1 macrophages are generally pro-inflammatory and possess anti-tumor activity, whereas M2 macrophages are associated with anti-inflammatory responses and tumor progression.
3.1 Interaction of Gal-9 with T cells
Gal-9 is a negative regulator of anti-tumor immunity, suppressing the immune response by targeting T cells and inducing Tregs, which are known for their immunosuppressive capabilities in the TME (15). The suppression of effector T cell activity by Gal-9 is crucial in the context of PDAC, where the TME is notoriously immunosuppressive.
One of the critical mechanisms by which Gal-9 facilitates immune evasion in tumors is through its interaction with the immune checkpoint receptor Tim-3 on T cells. The Gal-9/Tim-3 pathway inhibits T cell function and induces apoptosis of effector T cells, thereby reducing the anti-tumor immune response and contributing to the creation of an immunosuppressive tumor microenvironment (9). In addition to Tim-3, Gal-9 binds to PD-1 which promotes the survival of exhausted T cell populations. (16). Gal-9 can also specifically interact with V-domain Ig-containing suppressor of T cell activation (VISTA), adding another layer to Gal-9’s role in immune suppression (9, 17). In the context of acute myeloid leukemia (AML), VISTA binds to Gal-9 secreted by AML cells as a ligand. Importantly, soluble VISTA released by AML cells enhances the effect of Gal-9, likely by forming multiprotein complexes on the surface of T cells and possibly creating a molecular barrier. These events cause changes in the plasma membrane potential of T cells, leading to the activation of granzyme B inside cytotoxic T cells and resulting in apoptosis (17). The immune-evasive effects of Gal-9 are also associated with other immunoregulatory molecules such as the amino acid L-kynurenine (LKU), which also suppresses anti-cancer T cell functions (18). This cooperation is also highly relevant to PDAC since IDO1, the enzyme which regulates LKU generation, is expressed by pancreatic cancer cells (19).
Further research has demonstrated that Gal-9 and VISTA co-expression on T cells is associated with a terminally exhausted phenotype, particularly noted in HIV-1 patients and virus associated tumors (20, 21). This phenotype is characterized by impaired effector functions and the upregulation of exhaustion markers such as EOMES, Blimp-1, and Glut-1. Gal-9 and VISTA co-expression leads to a profound reduction in T cell activity, significantly attenuating the immune response against tumors. This exhausted T cell phenotype includes effector T cells (CD45RA+, CD45RO-/lo, CD62L-, CD27lo) with high T-bet expression, indicating a dysfunctional state that is likely mirrored in tumor immunology. (9, 21, 22).
Interaction of Gal-9 with CD44 enhances the stability and function of adaptive regulatory T cells, reinforcing immunosuppression within the tumor microenvironment through TGF- β and Smad3 activation (23).
These findings suggest that the interaction between Gal-9 and VISTA might also contribute to immune evasion in solid tumors, reinforcing the immunosuppressive environment and promoting tumor progression (9, 21).
3.2 Interactions of Gal-9 with myeloid cells
Gal-9 expression in PDAC is elevated not only in tumor cells but also in myeloid cells, including TAMs and MDSCs, particularly in areas adjacent to tumors (8, 11, 24). This upregulation correlates with poor prognosis, as Gal-9 contributes to tumor growth, immune evasion, and the modulation of myeloid cell behavior within the tumor microenvironment (25).
3.2.1 TAMs
In PDAC, TAMs are predominantly skewed towards an M2-like phenotype, contributing to an immunosuppressive environment that supports tumor growth and metastasis. Key signaling molecules such as TGF-β and CSF-1 play pivotal roles in the reprogramming of TAMs towards the M2 subtype. The ability of Gal-9 to polarize macrophages into an M2-like phenotype may significantly contribute to the immunosuppressive environment in PDAC (11, 26). An integrated analysis of transcriptomic datasets, including bulk and single cell RNA-seq data, identified Gal-9 as the primary component in cross-talk between TAMs and other cells. (27) While M2 macrophages contribute to tissue repair in normal physiology, in cancer, they promote tumor growth by suppressing immune responses In PDAC, Gal-9 interacts with Dectin-1 on macrophages, potentially driving a tolerogenic state that supports tumor progression. Notably, blocking Dectin-1 signaling in a PDAC mouse model reduced the infiltration of immunosuppressive CD206+ macrophages (24).
3.2.2 MDSCs
MDSCs are a heterogeneous group of immature myeloid cells with potent immunosuppressive functions (28). In PDAC, MDSCs directly induce Treg cells through cell-cell interactions, while Treg cells reciprocally influence MDSC survival and proliferation (29). These interactions have been observed at multiple stages of cancer progression (29). Interestingly, while Gal-9 polarizes macrophages towards an M2 phenotype, high levels of LGALS9 (Gal-9) mRNA correlated with reduced expression of the M2 markers CD163 and CD206, but with elevated expression of the MDSC marker CD15 (11). This suggests that Gal-9 skews macrophages into a unique, partially polarized state that balances immunosuppression and support for tumor growth.
3.3 Targeting Galectin-9 in PDAC
PDAC presents numerous challenges in its treatment, largely due to inherent barriers and resistance mechanisms that reduce the efficacy of both chemotherapy and immunotherapy (30, 31). Particularly, the dense stromal environment of PDAC acts as a physical barrier. This fibrotic environment can inhibit angiogenesis, resulting in a sparse and dysfunctional vascular network (32). Consequently, the delivery of chemotherapeutic agents to the tumor cells is limited. Additionally, the tumor immune microenvironment (TIME) supports various immunosuppressive mechanisms that protect the cancer cells from immune attack, leading to resistance against immunotherapies (33, 34).
One of the primary approaches in immunotherapy is the use of immune checkpoint inhibitors (ICI), such as PD-(L)1 and CTLA-4 inhibitors, that target effector T cells. These inhibitors have revolutionized the treatment of various cancers by blocking pathways that inhibit T cell activation, thus enhancing the immune system’s ability to attack tumor cells. In cancers such as melanoma and lung cancer, these treatments have shown substantial success. However, their effectiveness in PDAC has been limited as the immunosuppressive TIME hampers the efficacy of these checkpoint inhibitors (16, 35, 36). A detailed comparison of Gal-9 with other immune checkpoints in PDAC, including mechanisms, roles, and clinical implications, is summarized in Table 1. Consequently, these traditional ICIs have shown limited success in clinical trials for PDAC patients, underscoring the need for alternative therapeutic targets (48).
3.4 Immunotherapeutic potential of Galectin-9
Gal-9 has already shown significant therapeutic potential in various cancers. Enhanced expression of Gal-9 has been associated with improved overall survival in various solid cancers, including colon and hepatocellular cancer, as well as improved disease-free survival in gastric and non-small-cell lung cancer (49). Gal-9 mediates its tumor-suppressive effects through multiple mechanisms, including the induction of apoptosis in tumor cells, modulation of immune responses, and inhibition of metastasis. Particularly, increased levels of caspase-cleaved keratin 18 levels, as a measure of apoptosis, have been observed in Gal-9 treated colon cancer cells. Gal-9 dependent increase of caspase activity has also been observed in HCC cells in vitro and in vivo (50). Anti-metastatic potential of Gal-9 has been observed in triple negative breast cancer (TNBC), HCC (51) and melanoma (52). Additionally, in triple-negative breast (TNBC) cancer Gal-9 expression correlates with increased immune cell infiltration and positive PD-L1 expression on tumor cells. This dual role of promoting tumor cell death while modulating the immune environment makes Gal-9 a compelling target for therapeutic intervention. Furthermore, combining anti-Gal-9 therapy with anthracycline-based chemotherapy, such as doxorubicin, significantly enhances antitumor activity in breast cancer. This combination therapy leverages the complementary mechanisms of action of both treatments (53).
In the context of PDAC, preclinical studies have demonstrated that targeting Gal-9 can disrupt its interactions with Tim-3 and other immune modulators, thereby enhancing anti-tumor immune responses. This approach aims to mitigate the immunosuppressive effects of Gal-9, allowing the immune system to effectively target and eliminate cancer cells (8, 26). Moreover, the blockade of Gal-9 can potentially enhance the efficacy of other immunotherapeutic agents, such as PD-(L)1 and CTLA-4 inhibitors, by relieving the suppression of immune cell activity within the tumor microenvironment. Blockade of Gal-9 can be achieved with neutralizing antibodies or lactose (54–56).
Given the limitations of current immunotherapy in PDAC, targeting components of the TIME with nanomaterials can significantly enhance immunotherapeutic efficacy and optimize drug delivery outcomes. Therefore, combining anti-Gal-9 therapies with nanoparticle-based delivery systems may further enhance this approach in PDAC. Nanoparticles can precisely target tumor cells and modulate the immune response, providing a dual attack on the cancer. This method improves drug delivery, reduces off-target effects, and increases the overall efficacy of the treatment (34, 57). Early studies suggest that these combination therapies may improve patient outcomes by reducing tumor growth and metastasis, representing a novel and promising approach to overcoming the inherent barriers in PDAC treatment and enhancing the overall effectiveness of existing therapies (8, 16). Additionally, in a mouse model, T7 peptide-decorated exosome-based nanoparticles delivering Gal-9 siRNA significantly inhibit glioblastoma and enhance antitumor immunity.
Overall, the therapeutic potential of Gal-9 in cancer treatment is substantial, particularly in PDAC where traditional therapies have limited effectiveness. The development of anti-Gal-9 antibodies and other Gal-9-targeted therapies holds promise for improving outcomes in patients with PDAC and other malignancies by harnessing the immune system’s power to combat cancer. Continued research is necessary to fully understand the mechanisms by which Gal-9 modulates tumor and immune cell interactions and to translate these findings into effective clinical treatments. Despite the significant therapeutic potential of Gal-9, a search in ClinicalTrials.gov revealed only two current clinical trials that are interventional (see Table 2), while the other registered trials involving Gal-9 are observational (see Table 3). This underscores the need for expanded research and a greater number of clinical trials to fully explore and harness Gal-9’s therapeutic capabilities. Increasing the focus on Gal-9 in clinical settings is crucial for unlocking its potential in cancer treatment and other diseases.
3.5 Immunotherapeutic combination strategies
Combining various immunotherapeutic agents can help overcome the limitations of single-agent therapies and address mechanisms of resistance that tumors often develop. Interestingly, a phase II study in PDAC using combination of radiation therapy and anti-PD-1 or anti-CTLA-4 antibodies, indicated increased protein levels of circulating FasL, Gal-1 and Gal-9. Conversely, Gal-9 and PD-L1 combination treatment inhibits PDAC tumor progression in a murine PDAC model (37).
A promising strategy might thus involve the simultaneous blockade of Gal-9, Tim-3 or other ICIs including other galectins, to prevent compensatory mechanisms and resistance. Tumors often exploit multiple pathways to evade immune detection and destruction, so targeting several of these pathways at once can be more effective. For example, blocking Gal-9 and Tim-3 can disrupt the immunosuppressive signals that inhibit T-cell function, while ICIs like anti-PD-1 can further enhance T-cell activity against the tumor. However, ICI combination therapy can increase the risk of immune-related adverse events. In a meta-analysis of 18 studies spanning 10 cancer types, combination ICI therapy was linked to a modestly increased risk of all-grade adverse events and a significantly higher risk of grade 3 or more severe adverse events compared to ICI monotherapy (58).
Another innovative approach is the use of anti-Gal-9 in combination with reprogrammed macrophages or chimeric antigen receptor macrophages (CAR-M). These therapies aim to transform TAMs, which typically promote tumor growth and suppress immune responses, into a phenotype that supports anti-tumor immunity. Reprogrammed macrophages or CAR-M can enhance the phagocytosis of cancer cells and stimulate other immune cells to attack the tumor (59–62). When combined with anti-Gal-9, these therapies can further disrupt the immunosuppressive environment created by the tumor and enhance the overall immune response.
These combination strategies highlight the potential of leveraging multiple facets of the immune system to combat cancer more effectively. By targeting different pathways and using innovative delivery methods, these approaches aim to improve patient outcomes in PDAC and other challenging cancers. Continued research and clinical trials are necessary to optimize these combinations and determine the most effective protocols for different patient populations.
4 Conclusion
Gal-9 emerges as a pivotal modulator within the tumor immune microenvironment (TME) of pancreatic ductal adenocarcinoma (PDAC), orchestrating immunosuppressive mechanisms that promote tumor progression and therapy resistance Figure 1. Its multifaceted roles in regulating immune responses, including the induction of regulatory T cells (Tregs), polarization of TAMs to an M2-like phenotype, and interaction with immune checkpoints such as Tim-3, underscore its centrality in PDAC’s notoriously challenging TME. Elevated Gal-9 expression in PDAC, coupled with its association with poor prognosis, reinforces its potential as a prognostic biomarker and therapeutic target.
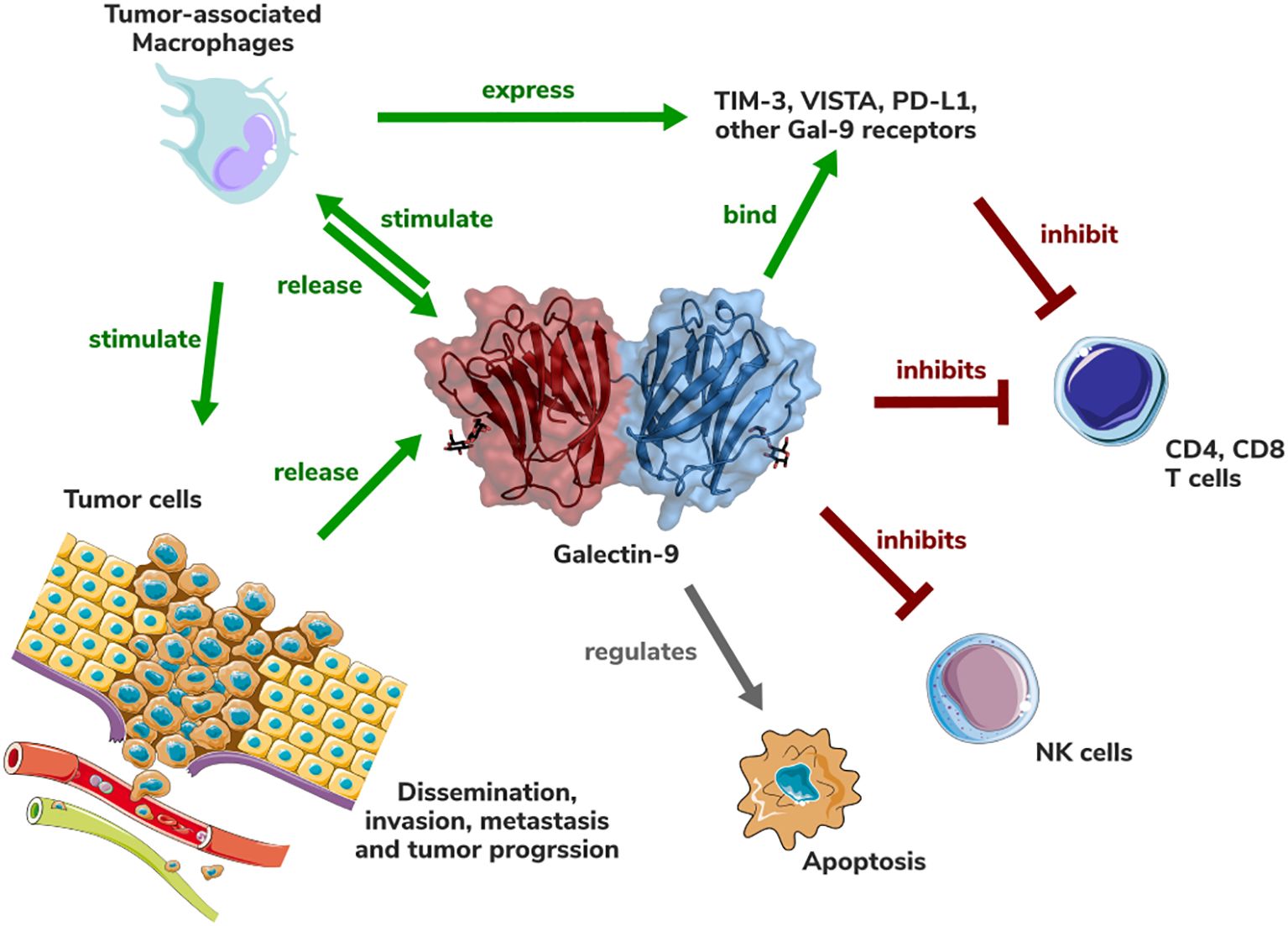
Figure 1. Schematic representation of the role of Galectin-9 in the PDAC tumor (immune) microenvironment. Gal-9 structure (PDB accession number: 3WV6) is shown in cartoon and surface representation.
A critical question in targeting Gal-9 is whether its opposing roles—promoting immune suppression in the TME while also exhibiting immune-stimulatory and anti-metastatic effects under certain contexts—can be therapeutically separated. Current evidence suggests that these divergent outcomes are highly dependent on the tumor type, stage, and specific microenvironmental factors. In PDAC, Gal-9 predominantly acts as an immune suppressor, and therapeutic strategies must focus on blocking its immunosuppressive interactions, particularly with Tim-3, Tregs, and TAMs. However, a more nuanced understanding of how Gal-9’s dual roles are regulated will be essential for developing precise interventions that maximize therapeutic benefits while minimizing unintended consequences.
Therapeutic targeting of Gal-9 presents several opportunities. Blocking Gal-9 can enhance the efficacy of immune checkpoint inhibitors such as PD-(L)1 and CTLA-4 inhibitors, thereby overcoming resistance to immunotherapy in PDAC. Combining Gal-9 inhibitors with advanced drug delivery systems, such as nanotechnology, holds potential for enhancing both the specificity and potency of treatments. Additionally, novel strategies like reprogramming TAMs or leveraging chimeric antigen receptor macrophages (CAR-M) in conjunction with Gal-9 inhibition could further disrupt the immunosuppressive TME and stimulate anti-tumor immunity. Early preclinical evidence is promising, but significant translational work is needed to validate these approaches in clinical settings.
In summary, Gal-9 represents a compelling and multifaceted therapeutic target in PDAC. To fully exploit its potential, future research must prioritize understanding the mechanisms underlying its dual roles and focus on refining therapeutic strategies to selectively neutralize its immunosuppressive functions. By targeting Gal-9, there is a tangible opportunity to overcome the inherent barriers of PDAC’s TME, paving the way for more effective and durable treatment options in this challenging malignancy.
Author contributions
CK: Writing – original draft. GN: Writing – review & editing. BG: Writing – review & editing. FH: Writing – review & editing. MN: Writing – review & editing. MB: Writing – review & editing. NM: Conceptualization, Writing – original draft, Writing – review & editing.
Funding
The author(s) declare that no financial support was received for the research, authorship, and/or publication of this article.
Acknowledgments
We gratefully acknowledge the support and insightful discussions with Vadim Sumbayev, whose contributions were invaluable to this work. Additionally, we acknowledge the use of generative AI tools (ChatGPT, based on the GPT-4 architecture) for language improvement. Figure 1 was partly generated using Servier Medical Art, provided by Servier, licensed under a Creative Commons Attribution 3.0 unported license.
Conflict of interest
The authors declare that the research was conducted in the absence of any commercial or financial relationships that could be construed as a potential conflict of interest.
Publisher’s note
All claims expressed in this article are solely those of the authors and do not necessarily represent those of their affiliated organizations, or those of the publisher, the editors and the reviewers. Any product that may be evaluated in this article, or claim that may be made by its manufacturer, is not guaranteed or endorsed by the publisher.
References
1. Siegel RL, Giaquinto AN, Jemal A. Cancer statistics 2024. CA: A Cancer J Clin. (2024) 74:12–49. doi: 10.3322/caac.21820
2. Wada J, Kanwar YS. Identification and characterization of galectin-9, a novel beta-galactoside-binding mammalian lectin. J Biol Chem. (1997) 272:6078–86. doi: 10.1074/jbc.272.9.6078
3. Morishita A, Oura K, Tadokoro T, Shi T, Fujita K, Tani J, et al. Galectin-9 in gastroenterological cancer. Int J Mol Sci. (2023) 24:1–17. doi: 10.3390/ijms24076174
4. Bi S, Earl LA, Jacobs L, Baum LG. Structural features of galectin-9 and galectin-1 that determine distinct T cell death pathways. J Biol Chem. (2008) 283:12248–58. doi: 10.1074/jbc.M800523200
5. Yang RY, Rabinovich GA, Liu FT. Galectins: structure, function and therapeutic potential. Expert Rev Mol Med. (2008) 10:e17. doi: 10.1017/S1462399408000719
6. Hirashima M, Kashio Y, Nishi N, Yamauchi A, Imaizumi T-A, Kageshita T, et al. Galectin-9 in physiological and pathological conditions. Glycoconjugate J. (2002) 19:593–600. doi: 10.1023/B:GLYC.0000014090.63206.2f
7. Zhang Q, Zeng L, Chen Y, Lian G, Qian C, Chen S, et al. Pancreatic cancer epidemiology, detection, and management. Gastroenterol Res Pract. (2016) 2016:8962321. doi: 10.1155/2016/8962321
8. Quilbe A, Mustapha R, Duchene B, Kumar A, Werkmeister E, Leteurtre E, et al. A novel anti-galectin-9 immunotherapy limits the early progression of pancreatic neoplastic lesions in transgenic mice. Front Immunol. (2023) 14:1267279. doi: 10.3389/fimmu.2023.1267279
9. Schlichtner S, Yasinska IM, Lall GS, Berger SM, Ruggiero S, Cholewa D, et al. T lymphocytes induce human cancer cells derived from solid Malignant tumors to secrete galectin-9 which facilitates immunosuppression in cooperation with other immune checkpoint proteins. J Immunother Cancer. (2023) 11:1–13. doi: 10.1136/jitc-2022-005714
10. Tavares LB, Silva-Filho AF, Martins MR, Vilar KM, Pitta MGR, Rêgo MJBM. Patients with pancreatic ductal adenocarcinoma have high serum galectin-9 levels: A sweet molecule to keep an eye on. Pancreas. (2018) 47:e59–60. doi: 10.1097/mpa.0000000000001126
11. Seifert AM, Reiche C, Heiduk M, Tannert A, Meinecke AC, Baier S, et al. Detection of pancreatic ductal adenocarcinoma with galectin-9 serum levels. Oncogene. (2020) 39:3102–13. doi: 10.1038/s41388-020-1186-7
12. Chou FC, Chen HY, Kuo CC, Sytwu HK. Role of galectins in tumors and in clinical immunotherapy. Int J Mol Sci. (2018) 19:1–11. doi: 10.3390/ijms19020430
13. Kapetanakis NI, Busson P. Galectins as pivotal components in oncogenesis and immune exclusion in human Malignancies. Front Immunol. (2023) 14:1145268. doi: 10.3389/fimmu.2023.1145268
14. Thijssen VL, Hulsmans S, Griffioen AW. The galectin profile of the endothelium: altered expression and localization in activated and tumor endothelial cells. Am J Pathol. (2008) 172:545–53. doi: 10.2353/ajpath.2008.070938
15. Cedeno-Laurent F, Dimitroff CJ. Galectins and their ligands: negative regulators of anti-tumor immunity. Glycoconj J. (2012) 29:619–25. doi: 10.1007/s10719-012-9379-0
16. Yang R, Sun L, Li CF, Wang YH, Yao J, Li H, et al. Galectin-9 interacts with PD-1 and TIM-3 to regulate T cell death and is a target for cancer immunotherapy. Nat Commun. (2021) 12:832. doi: 10.1038/s41467-021-21099-2
17. Yasinska IM, Meyer NH, Schlichtner S, Hussain R, Siligardi G, Casely-Hayford M, et al. Ligand-receptor interactions of galectin-9 and VISTA suppress human T lymphocyte cytotoxic activity. Front Immunol. (2020) 11:580557. doi: 10.3389/fimmu.2020.580557
18. Schlichtner S, Yasinska IM, Klenova E, Abooali M, Lall GS, Berger SM, et al. L-Kynurenine participates in cancer immune evasion by downregulating hypoxic signaling in T lymphocytes. Oncoimmunology. (2023) 12:2244330. doi: 10.1080/2162402X.2023.2244330
19. Newman AC, Falcone M, Huerta Uribe A, Zhang T, Athineos D, Pietzke M, et al. Immune-regulated IDO1-dependent tryptophan metabolism is source of one-carbon units for pancreatic cancer and stellate cells. Mol Cell. (2021) 81:2290–2302.e2297. doi: 10.1016/j.molcel.2021.03.019
20. Okoye I, Xu L, Motamedi M, Parashar P, Walker JW, Elahi S. Galectin-9 expression defines exhausted T cells and impaired cytotoxic NK cells in patients with virus-associated solid tumors. J Immunother Cancer. (2020) 8:1–13. doi: 10.1136/jitc-2020-001849
21. Shahbaz S, Dunsmore G, Koleva P, Xu L, Houston S, Elahi S. Galectin-9 and VISTA expression define terminally exhausted T cells in HIV-1 infection. J Immunol. (2020) 204:2474–91. doi: 10.4049/jimmunol.1901481
22. Schlichtner S, Yasinska IM, Ruggiero S, Berger SM, Aliu N, Prunk M, et al. Expression of the immune checkpoint protein VISTA is differentially regulated by the TGF-beta1 - smad3 signaling pathway in rapidly proliferating human cells and T lymphocytes. Front Med (Lausanne). (2022) 9:790995. doi: 10.3389/fmed.2022.790995
23. Wu C, Thalhamer T, Franca RF, Xiao S, Wang C, Hotta C, et al. Galectin-9-CD44 interaction enhances stability and function of adaptive regulatory T cells. Immunity. (2014) 41:270–82. doi: 10.1016/j.immuni.2014.06.011
24. Daley D, Mani VR, Mohan N, Akkad N, Ochi A, Heindel DW, et al. Dectin 1 activation on macrophages by galectin 9 promotes pancreatic carcinoma and peritumoral immune tolerance. Nat Med. (2017) 23:556–67. doi: 10.1038/nm.4314
25. Sun Q, Zhang Y, Liu M, Ye Z, Yu X, Xu X, et al. Prognostic and diagnostic significance of galectins in pancreatic cancer: a systematic review and meta-analysis. Cancer Cell Int. (2019) 19:309. doi: 10.1186/s12935-019-1025-5
26. Zhang M, Liu C, Li Y, Li H, Zhang W, Liu J, et al. Galectin-9 in cancer therapy: from immune checkpoint ligand to promising therapeutic target. Front Cell Dev Biol. (2024) 11:1332205. doi: 10.3389/fcell.2023.1332205
27. Avsar G, Pir P. An integrated study to decipher immunosuppressive cellular communication in the PDAC environment. NPJ Syst Biol Appl. (2023) 9:56. doi: 10.1038/s41540-023-00320-6
28. Ostrand-Rosenberg S, Fenselau C. Myeloid-derived suppressor cells: immune-suppressive cells that impair antitumor immunity and are sculpted by their environment. J Immunol. (2018) 200:422–31. doi: 10.4049/jimmunol.1701019
29. Siret C, Collignon A, Silvy F, Robert S, Cheyrol T, Andre P, et al. Deciphering the crosstalk between myeloid-derived suppressor cells and regulatory T cells in pancreatic ductal adenocarcinoma. Front Immunol. (2019) 10:3070. doi: 10.3389/fimmu.2019.03070
30. Quinonero F, Mesas C, Doello K, Cabeza L, Perazzoli G, Jimenez-Luna C, et al. The challenge of drug resistance in pancreatic ductal adenocarcinoma: a current overview. Cancer Biol Med. (2019) 16:688–99. doi: 10.20892/j.issn.2095-3941.2019.0252
31. Ye X, Yu Y, Zheng X, Ma H. Clinical immunotherapy in pancreatic cancer. Cancer Immunol Immunother. (2024) 73:64. doi: 10.1007/s00262-024-03632-6
32. Katsuta E, Qi Q, Peng X, Hochwald SN, Yan L, Takabe K. Pancreatic adenocarcinomas with mature blood vessels have better overall survival. Sci Rep. (2019) 9:1310, 1-12. doi: 10.1038/s41598-018-37909-5
33. Murakami T, Hiroshima Y, Matsuyama R, Homma Y, Hoffman RM, Endo I. Role of the tumor microenvironment in pancreatic cancer. Ann Gastroenterological Surg. (2019) 3:130–7. doi: 10.1002/ags3.12225
34. Noubissi Nzeteu GA, Gibbs BF, Kotnik N, Troja A, Bockhorn M, Meyer NH. Nanoparticle-based immunotherapy of pancreatic cancer. Front Mol Biosci. (2022) 9:948898. doi: 10.3389/fmolb.2022.948898
35. Siegel RL, Miller KD, Wagle NS, Jemal A. Cancer statistics 2023. CA Cancer J Clin. (2023) 73:17–48. doi: 10.3322/caac.21763
36. Hartupee C, Nagalo BM, Chabu CY, Tesfay MZ, Coleman-Barnett J, West JT, et al. Pancreatic cancer tumor microenvironment is a major therapeutic barrier and target. Front Immunol. (2024) 15:1287459. doi: 10.3389/fimmu.2024.1287459
37. Li E, Xu J, Chen Q, Zhang X, Xu X, Liang T. Galectin-9 and PD-L1 antibody blockade combination therapy inhibits tumour progression in pancreatic cancer. Immunotherapy. (2023) 15:135–47. doi: 10.2217/imt-2021-0075
38. Feng M, Xiong G, Cao Z, Yang G, Zheng S, Song X, et al. PD-1/PD-L1 and immunotherapy for pancreatic cancer. Cancer Lett. (2017) 407:57–65. doi: 10.1016/j.canlet.2017.08.006
39. Lu C, Paschall AV, Shi H, Savage N, Waller JL, Sabbatini ME, et al. The MLL1-H3K4me3 axis-mediated PD-L1 expression and pancreatic cancer immune evasion. J Natl Cancer Inst. (2017) 109:1–12. doi: 10.1093/jnci/djw283
40. Mucileanu A, Chira R, Mircea PA. PD-1/PD-L1 expression in pancreatic cancer and its implication in novel therapies. Med Pharm Rep. (2021) 94:402–10. doi: 10.15386/mpr-2116
41. Walunas TL, Lenschow DJ, Bakker CY, Linsley PS, Freeman GJ, Green JM, et al. CTLA-4 can function as a negative regulator of T cell activation. Immunity. (1994) 1:405–13. doi: 10.1016/1074-7613(94)90071-x
42. Ware MB, Phillips M, McQuinn C, Zaidi MY, Knochelmann HM, Greene E, et al. Dual IL-6 and CTLA-4 blockade regresses pancreatic tumors in a T cell- and CXCR3-dependent manner. JCI Insight. (2023) 8:1–17. doi: 10.1172/jci.insight.155006
43. Seifert L, Plesca I, Muller L, Sommer U, Heiduk M, von Renesse J, et al. LAG-3-expressing tumor-infiltrating T cells are associated with reduced disease-free survival in pancreatic cancer. Cancers (Basel). (2021) 13:1–12. doi: 10.3390/cancers13061297
44. Mariuzza RA, Shahid S, Karade SS. The immune checkpoint receptor LAG3: Structure, function, and target for cancer immunotherapy. J Biol Chem. (2024) 300:107241. doi: 10.1016/j.jbc.2024.107241
45. Peng PJ, Li Y, Sun S. On the significance of Tim-3 expression in pancreatic cancer. Saudi J Biol Sci. (2017) 24:1754–7. doi: 10.1016/j.sjbs.2017.11.006
46. Zhang T, Tan XL, Xu Y, Wang ZZ, Xiao CH, Liu R. Expression and prognostic value of indoleamine 2,3-dioxygenase in pancreatic cancer. Chin Med J (Engl). (2017) 130:710–6. doi: 10.4103/0366-6999.201613
47. Jonescheit H, Oberg HH, Gonnermann D, Hermes M, Sulaj V, Peters C, et al. Influence of Indoleamine-2,3-Dioxygenase and Its Metabolite Kynurenine on gammadelta T Cell Cytotoxicity against Ductal Pancreatic Adenocarcinoma Cells. Cells. (2020) 9:1–22. doi: 10.3390/cells9051140
48. Farhangnia P, Khorramdelazad H, Nickho H, Delbandi AA. Current and future immunotherapeutic approaches in pancreatic cancer treatment. J Hematol Oncol. (2024) 17:40. doi: 10.1186/s13045-024-01561-6
49. Zhou X, Sun L, Jing D, Xu G, Zhang J, Lin L, et al. Galectin-9 expression predicts favorable clinical outcome in solid tumors: A systematic review and meta-analysis. Front Physiol. (2018) 9:452. doi: 10.3389/fphys.2018.00452
50. Fujita K, Iwama H, Sakamoto T, Okura R, Kobayashi K, Takano J, et al. Galectin-9 suppresses the growth of hepatocellular carcinoma via apoptosis in vitro and in vivo. Int J Oncol. (2015) 46:2419–30. doi: 10.3892/ijo.2015.2941
51. Zhang L, Jin R, Yang X, Ying D. A population-based study of synchronous distant metastases and prognosis in patients with PDAC at initial diagnosis. Front Oncol. (2023) 13:1087700. doi: 10.3389/fonc.2023.1087700
52. Kageshita T, Kashio Y, Yamauchi A, Seki M, Abedin MJ, Nishi N, et al. Possible role of galectin-9 in cell aggregation and apoptosis of human melanoma cell lines and its clinical significance. Int J Cancer. (2002) 99:809–16. doi: 10.1002/ijc.10436
53. Sun X, Wang W-J, Lang J, Riyao Yang 2,7, Shen W-J, Sun L, et al. Inhibition of Galectin-9 sensitizes tumors to anthracycline treatment via inducing antitumor immunity. Int J Biol Sci. (2023) 19:4644–56. doi: 10.7150/ijbs.84108
54. Bailly C, Thuru X, Quesnel B. Modulation of the gal-9/TIM-3 immune checkpoint with α-lactose. Does anomery of lactose matter? Cancers. (2021) 13:1–22. doi: 10.3390/cancers13246365
55. Schlichtner S, Meyer NH, Yasinska IM, Aliu N, Berger SM, Gibbs BF, et al. Functional role of galectin-9 in directing human innate immune reactions to Gram-negative bacteria and T cell apoptosis. Int Immunopharmacol. (2021) 100:108155. doi: 10.1016/j.intimp.2021.108155
56. Yang R, Sun L, Li C-F, Wang Y-H, Xia W, Liu B, et al. Development and characterization of anti-galectin-9 antibodies that protect T cells from galectin-9-induced cell death. J Biol Chem. (2022) 298:1–12. doi: 10.1016/j.jbc.2022.101821
57. Das M, Shen L, Liu Q, Goodwin TJ, Huang L. Nanoparticle delivery of RIG-I agonist enables effective and safe adjuvant therapy in pancreatic cancer. Mol Ther. (2019) 27:507–17. doi: 10.1016/j.ymthe.2018.11.012
58. Park R, Lopes L, Cristancho CR, Riano IM, Saeed A. Treatment-related adverse events of combination immune checkpoint inhibitors: systematic review and meta-analysis. Front Oncol. (2020) 10:258. doi: 10.3389/fonc.2020.00258
59. Klichinsky M, Ruella M, Shestova O, Lu XM, Best A, Zeeman M, et al. Human chimeric antigen receptor macrophages for cancer immunotherapy. Nat Biotechnol. (2020) 38:947–53. doi: 10.1038/s41587-020-0462-y
60. O'Hara MH, O'Reilly EM, Varadhachary G, Wolff RA, Wainberg ZA, Ko AH, et al. CD40 agonistic monoclonal antibody APX005M (sotigalimab) and chemotherapy, with or without nivolumab, for the treatment of metastatic pancreatic adenocarcinoma: an open-label, multicentre, phase 1b study. Lancet Oncol. (2021) 22:118–31. doi: 10.1016/s1470-2045(20)30532-5
61. Sloas C, Gill S, Klichinsky M. Engineered CAR-macrophages as adoptive immunotherapies for solid tumors. Front Immunol. (2021) 12:783305. doi: 10.3389/fimmu.2021.783305
Keywords: pancreatic ductal adenocarcinoma, tumor immune microenvironment, immunotherapy, immune checkpoints, galectin-9, myeloid-derived suppressor cells, macrophage (re-)polarization
Citation: Knickmeier C, Noubissi Nzeteu GA, Gibbs BF, Hoogwater FJH, Nijkamp MW, Bockhorn M and Meyer NH (2025) It’s about TIME – Gal-9 as a potential immunotherapeutic target in pancreatic ductal adenocarcinoma. Front. Immunol. 16:1495907. doi: 10.3389/fimmu.2025.1495907
Received: 13 September 2024; Accepted: 13 January 2025;
Published: 31 January 2025.
Edited by:
Jesse Haramati, University of Guadalajara, MexicoReviewed by:
Graham Robert Leggatt, The University of Queensland, AustraliaVikas Somani, Washington University in St. Louis, United States
Copyright © 2025 Knickmeier, Noubissi Nzeteu, Gibbs, Hoogwater, Nijkamp, Bockhorn and Meyer. This is an open-access article distributed under the terms of the Creative Commons Attribution License (CC BY). The use, distribution or reproduction in other forums is permitted, provided the original author(s) and the copyright owner(s) are credited and that the original publication in this journal is cited, in accordance with accepted academic practice. No use, distribution or reproduction is permitted which does not comply with these terms.
*Correspondence: N. Helge Meyer, aGVsZ2UubWV5ZXJAdW5pLW9sZGVuYnVyZy5kZQ==
†These authors share first authorship