- 1Department of Dermatology, Northwestern University, Feinberg School of Medicine, Chicago, IL, United States
- 2Department of Radiation and Cellular Oncology, University of Chicago Medicine, Chicago, IL, United States
- 3Genomics and Microbiome Core Facility, Rush University Medical Center, Chicago, IL, United States
- 4Stanley Manne Children’s Research Institute, Ann and Robert H. Lurie Children’s Hospital of Chicago, Chicago, IL, United States
- 5Department of Pediatrics, Northwestern University, Feinberg School of Medicine, Chicago, IL, United States
- 6The Ludwig Center for Metastasis, University of Chicago Medicine, Chicago, IL, United States
- 7Department of Radiation Oncology, Northwestern University, Feinberg School of Medicine, Chicago, IL, United States
- 8Department of Biology, Loyola University Chicago, Chicago, IL, United States
Introduction: Cutaneous T-cell lymphoma (CTCL) is closely associated with the host microbiome. While recent evidence suggests that shifts in specific bacterial taxa are associated with response to UV-B, a form of non-ionizing radiation, the impact of ionizing radiation (IR) has not been investigated.
Methods: 16S rRNA and tuf gene amplicon sequencing were performed on DNA extracted from swabs of lesional/non-lesional skin of 12 CTCL patients before/after TSEBT or local IR and from 25 matched healthy controls (HC). Microbial diversity and taxonomic profiles were analyzed.
Results: Radiation exposure increased CTCL skin α-diversity to levels approximating HC. TSEBT appeared to carry the greatest effect compared to local IR. Both α and β-diversity differed significantly post versus pre-IR for TSEBT, but not for local IR. IR was associated with decreases in known pathogenic bacteria such as Streptococcus and S. aureus and increases in healthy commensal bacteria such as Anaerococcus, Bifidobacterium and commensal staphylococci including S. pettenkoferi. Substantially more taxa shifts were seen with TSEBT versus local IR.
Discussion: IR not only eliminates CTCL lesions via induction of apoptosis, but also facilitates skin barrier restoration and recolonization of bacterial taxa associated with a healthy skin microbiome. Local IR does not have as strong an effect on the skin microbiome as TSEBT. As skin microbiota act as immunomodulators with local and potentially systemic influence, TSEBT may also improve CTCL lesions via global effects on the skin microbiome. Future larger-scale studies are required to fully elucidate the relationship between cutaneous microbes and IR treatment in CTCL.
Introduction
Cutaneous T-cell lymphoma (CTCL) encompasses a heterogenous group of non-Hodgkin T-cell lymphomas characterized by malignant T-cells in the skin (1). Evidence increasingly suggests an intimate connection between the host microbiome and CTCL disease pathogenesis. Although the etiology of CTCL remains unknown, skin bacteria can fuel disease progression (2–4). Skin, nasal and gut microbiome changes correlate with CTCL disease progression and severity (5–7). Additionally, broad-spectrum antibiotics may reduce tumor burden in some patients (8).
Ionizing radiation (IR) is one of the most effective treatments for CTCL (9). Local IR is often used for solitary lesions, whereas total skin electron beam therapy (TSEBT), a procedure which delivers IR to the entire skin surface, is typically utilized in patients with more diffuse disease (9). We previously demonstrated that narrowband ultraviolet B (nbUVB), a form of non-ionizing radiation utilized for treatment of CTCL, alters the skin and gut microbiomes of CTCL patients, and others have shown that the skin microbiome modulates the effect of UV on inflammation (10–13). Moreover, the skin microbiome of nbUVB responders, but not non-responders, has increased microbial diversity and shifts in the relative abundance of certain bacterial taxa, including increased pre-treatment abundances of S. capitis and S. warneri, and decreased post-treatment S. aureus and S. lugdunensis, that may be predictive of response to nbUVB treatment (10). Given these findings, we hypothesize that similar shifts may be identified with IR, which remains unexplored.
Prior studies have cross-sectionally examined skin microbial changes with respect to radiation injury, but none to our knowledge have addressed the longitudinal changes associated with palliative IR (14). For example, cancer patients experiencing IR-induced dermatitis have significantly reduced bacterial diversity and overrepresentation of Staphylococcus, Pseudomonas, and Stenotrophomonas when compared to healthy controls (15). Furthermore, patients experiencing radiation-induced skin injury have significantly different relative abundances of bacterial taxa when compared to healthy controls (16).
Herein, we performed a longitudinal study of the skin bacterial microbiome of CTCL patients receiving IR and compared them with matched healthy controls. This knowledge may improve our understanding of the skin microbiome in CTCL and the effects of IR on the skin microbiome.
Materials and methods
Participants
Ethical approval was obtained from the Northwestern University Institutional Review Board (IRB) (STU00209226) & University of Chicago IRB (IRB22-0595). Patients were consented and enrolled at the Northwestern University Cutaneous Lymphoma clinic and University of Chicago Radiation Oncology clinic between 2019 and 2023 in compliance with the Declaration of Helsinki. Demographic/clinical data and lesional and contralateral non-lesional skin samples were collected from 12 patients with biopsy-confirmed CTCL (Supplementary Table S1). Six participants received local IR, and 6 patients received TSEBT (Supplementary Table S2). Eleven patients were concurrently on topical steroids and 8 on systemic therapy (i.e., acitretin and interferon) but no new treatments were introduced within 6 months prior to or during the study interval and any concurrent therapies were present for at least 6 months prior to IR. Additionally, there were no changes in diet, bathing habits, or non-CTCL medications based on chart review and survey data collected during the study interval. Patients who utilized antibiotics within the 4 weeks prior to collections were excluded. Healthy controls (HC) were comprised of 25 volunteers without CTCL or other active skin disease (Supplementary Table S3).
Sample collection and DNA extraction
Skin samples from 12 CTCL patients and 25 HC were obtained through sterile swabs. All specimens were placed immediately in sterile cryovials and stored at -80°C until DNA extraction. Genomic DNA was extracted using a Maxwell 16 LEV Blood DNA Kit (Promega, Madison, WI) implemented on a Maxwell 16 Instrument, following the manufacturer’s instructions with minor modifications: a lysozyme incubation (10 ng/μl lysozyme; Thermo Fisher Scientific, Waltham, MA) for 30 minutes at 37°C and bead beating (40 seconds at 6 min/sec) using a FastPrep-24 System (MP Biomedicals, Irvine, CA). Homogenized samples were transferred to the Maxwell cartridges for final DNA purification.
16S rRNA amplification and sequencing
Genomic DNA was prepared for sequencing using a two-stage amplicon workflow and targeting the V4 variable region of microbial 16S rRNA genes as described previously (10, 17, 18).
Basic processing
Sequencing resulted in a total of 9,257,316 reads with an average of 31,275 reads per sample. Forward (F) and reverse (R) reads were trimmed using cutadapt v3.5 to remove primer sequences (19). Processing, decontamination, and filtering were performed in a matter identical to our previous work examining nbUVB and the CTCL skin microbiome (10, 20–23). Following QC processing, decontamination, and filtering, there were a total of 5,125,206 merged read pairs with an average of 18,982 per sample. To maximize data retention, while removing uninformative patient samples, only samples with minimum 1000 reads following processing were retained. Patient samples were paired across disease status (lesional, non-lesional) and time (pre-IR, post-IR).
Tuf2 amplicon next generation sequencing
Genomic DNA was PCR amplified with primers CS1_tuf2-F (ACACTGACGACATGGTTCTACAACAGGCCGTGTTGA ACGTG) and CS2_tuf2-R (TACGGTAGCAGAGACTTGG TCTACAGTACGTCCACCTTCACG) targeting the Staphylococcus tuf gene (24, 25). Amplicons were generated using a two-stage PCR amplification protocol, as previously described and utilized in our previous work (10, 17).
Tuf2 amplicon processing
Tuf2 sequencing generated a total of 10,289,743 reads with an average of 34,880 raw reads per sample. Primer trimming and denoising were accomplished using the same procedure as above for the 16S amplicon data with the following modifications: during filtering and trimming, the maxEE for all reads was set to 3,3 due to their increased length, read merging used default parameters, and the read length cutoff window range was 460-470 nucleotides for merged read pairs. Following processing there were a total of 3,914,382 reads retained for an average sample read count of 13,269. ASVs were taxonomically annotated using BLAST alignments against NCBI prokaryotic (nr) refseq database (online access 10 April 2023). Only sequences that were annotated as Staphylococcus were retained.
Statistical analysis
The samples in the cleaned ASV table were visually evaluated using phyloseq v1.42.0 (26). α-diversity metrics were generated using the ASV table rarefied to 1000 sequences. Differences in α-diversity between patient sets were calculated using Wilcoxon rank-sum non-paired tests from the stats R package while differences within patient-matched samples were calculated using Pairwise Wilcoxon rank-sum tests. β-diversity metrics were generated using the rarefied ASV table (27). Principal coordinate analysis (PCoA) with Bray-Curtis dissimilarity was performed to identify β-diversity using an ADONIS2 method with default parameters (28).
Differential abundance analysis was conducted by DESeq2 v1.44.0 using the non-rarefied, CLR-transformed ASV table, with ASVs removed if they had less than 4 counts or a prevalence below 10% across the sample set (29). A linear model was implemented within the approach to compare abundance of taxa among different groups. Significant ASVs were only considered if they achieved a false discovery rate (BH-FDR)-adjusted p-value of <0.05 (q-value).
The post-process tuf2 ASV and taxa table was filtered using phyloseq v1.38 (26). To identify the most abundant Staphylococcus species, data were centered log-ratio (CLR) transformed and the final species-level table including only species present at 10% abundance or greater was used for statistical analysis. Differences in abundance between patient groups were calculated using Wilcoxon rank-sum non-paired tests from the stats R package while Pairwise Wilcoxon rank-sum tests were used to calculate differences between patient-matched samples (27). Differential abundance analysis was conducted using the CLR transformed abundance table. A linear model as described above was implemented to compare abundance of the top Staphylococcus species among different groups. Significant ASVs were only considered if they achieved an FDR of <0.05.
Results
Patient and IR characteristics
The median age of CTCL patients was 56 years (range 21-77) and 9 patients (75%) were male. Ten (83%) patients had mycosis fungoides, and 2 had other CTCL types. Median time between pre-IR sample collection and IR was 20 days; median time between IR and post-IR sample collection was 30 days. Modified Severity Weighted Assessment Tool (mSWAT) decreased by 25.3 points, on average, after IR. Patients receiving local IR received an average of 9.5 Gray over 1-12 fractions; patients receiving TSEBT received an average of 20 Gray over 6-16 fractions. There were no significant differences between age, sex, race, Fitzpatrick skin type (FST), CTCL subtype, stage, or non-IR therapies between patient’s receiving local IR versus TSEBT (p>0.05) (Supplementary Table S4). There were no statistically significant differences between HC and patient age, sex, race, or comorbidities (p>0.05) (Supplementary Table S5).
IR is associated with increased microbial diversity and greater microbial shifts in non-lesional skin
Across all individuals, Staphylococcus was the most abundant genus pre- and post-IR, followed by Corynebacterium and Streptococcus (Figure 1A).
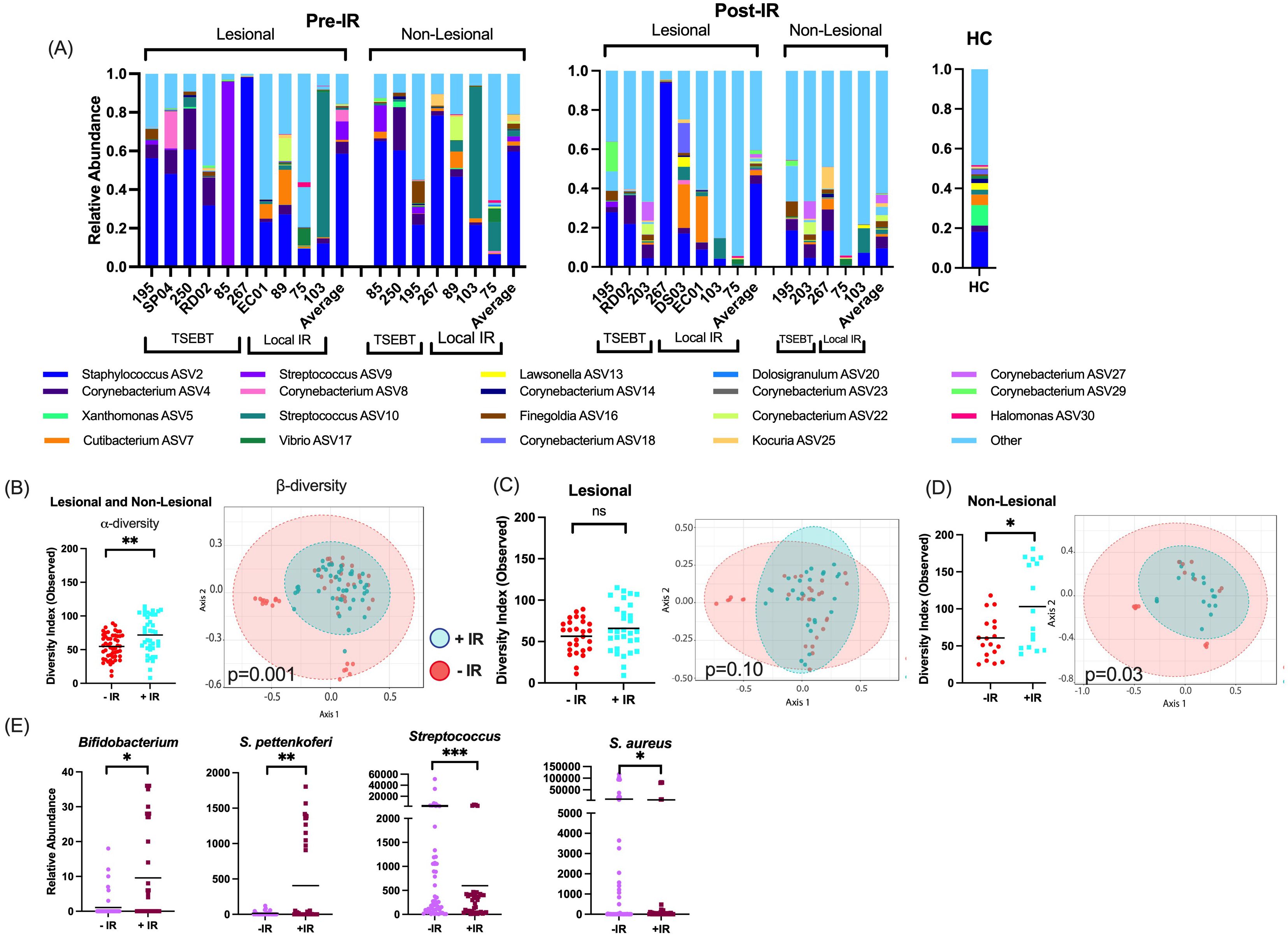
Figure 1. Taxon-by-taxon and α- and β-diversity analyses for skin exposed to any form of ionizing radiation (IR). (A) At the taxonomic level of genus, Staphylococcus, Corynebacterium, and Streptococcus were the most prevalent and abundant genera amongst all samples. Bar charts indicate the relevant abundance of the 19 most abundant genera, remaining genera were grouped as “other.” (B) For any skin (both lesional & non-lesional) exposed to any form of IR, α-diversity is higher with IR exposure, and β-diversity is significantly different between the two communities. (C) For only lesional skin exposed to IR, α- and β-diversities are not significantly different between skin with and without IR exposure. (D) For non-lesional skin exposed to any form of radiotherapy, α-diversity and β-diversity were significantly different between skin with and without IR exposure. (E) Taxa-by-taxa analyses with and without IR exposure reveals significantly different relative abundances of specific bacterial taxa. *p <0.05; **p<0.01; ***p<0.001; ns, not significant.
We first analyzed any skin (both lesional and non-lesional) exposed to any form of IR (both local IR and TSEBT). Phylogenetic diversity (α-diversity) was significantly higher with IR exposure (Observed p=0.0019), and community structure (β-diversity) differed significantly between skin with and without IR exposure (p=0.001) (Figure 1B). Next, we analyzed only lesional skin exposed to IR. In this analysis, there were no significant differences in α- or β-diversity between lesions with and without exposure (Figure 1C). Finally, we analyzed only non-lesional skin exposed to IR. Phylogenetic diversity was significantly higher with IR exposure (p=0.02), and β-diversity differed significantly between non-lesional skin with and without IR exposure (p=0.03) (Figure 1D).
When observing changes to taxa, healthy commensal bacteria such as Bifidobacterium and coagulase negative species S. pettenkoferi were significantly more abundant in skin with IR exposure, and known pathogenic bacteria such as Streptococcus and S. aureus were either significantly lower (p<0.01, q<0.05) or trended lower (p<0.05, q>0.05), respectively, in skin with IR exposure. (Figure 1E). Comparatively, very few bacteria with pathogenic potential were increased post-IR exposure. These included Trueperella and Porphyromonas which have only been reported to cause skin infections in rare opportunistic cases (30, 31).
TSEBT, but not local IR, increases bacterial diversity and modifies bacterial community structure
Next, we compared α- and β-diversity between pre-TSEBT and post-TSEBT and between lesional and non-lesional skin. α-diversity was significantly higher post-TSEBT compared to pre-TSEBT in both lesional (Observed p=0.024) and non-lesional (p=0.0015) skin (Figure 2A). Conversely, α-diversity did not differ between non-lesional and lesional skin at either the pre-TSEBT (p=0.23) or post-TSEBT (p=0.07) time points (Figure 2A).
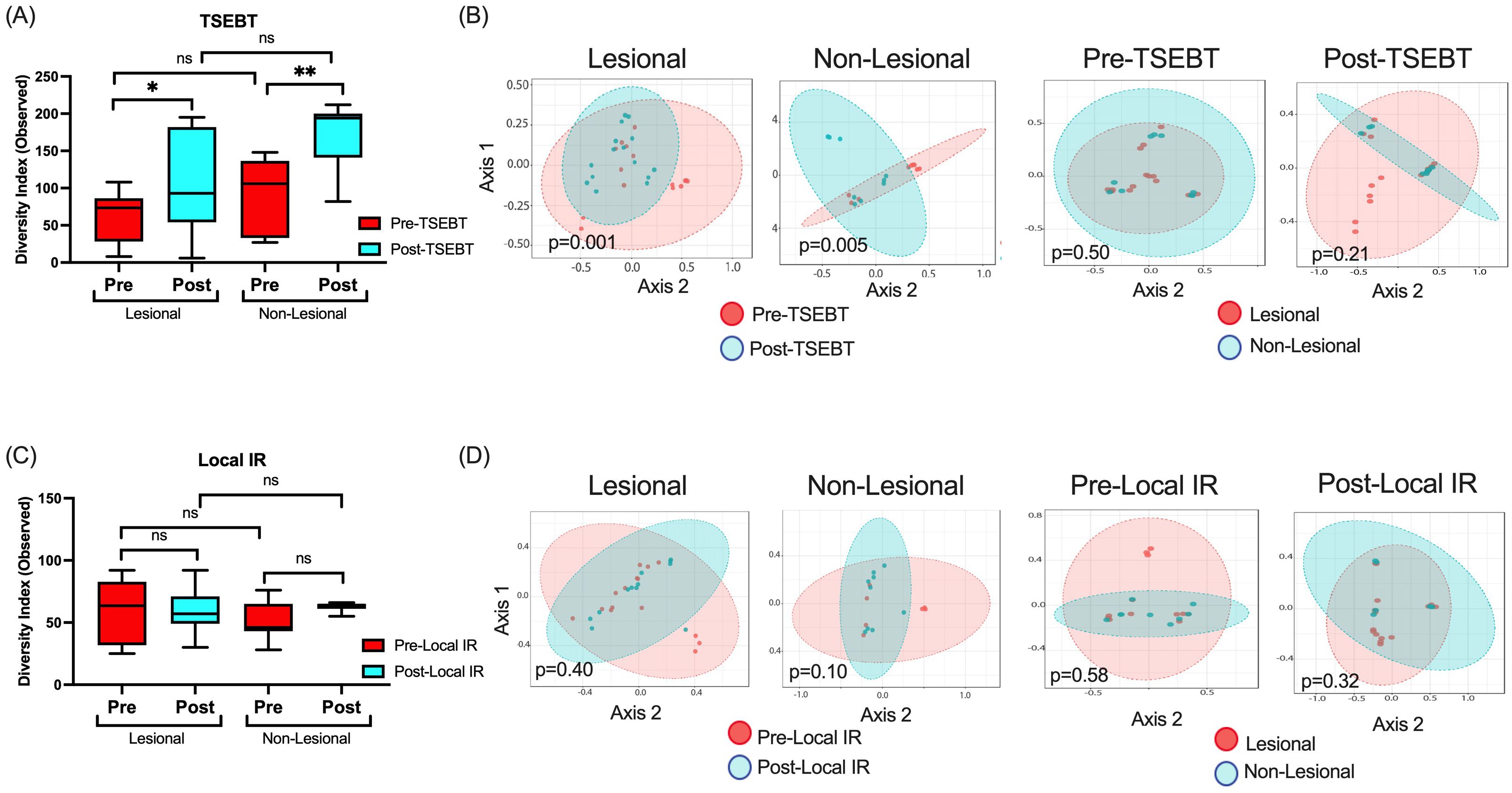
Figure 2. α- and β-diversity analyses for the TSEBT and local-IR study groups analyzed. (A) Analyses of both lesional and non-lesional skin before and after TSEBT demonstrates higher α-diversity post-TSEBT for both groups. There was no significant difference in α-diversity between lesional and non-lesional skin at either pre-TSEBT or post-TSEBT time points. (B) β-diversity for lesional and non-lesional skin reveals different microbial communities for pre- versus post-TSEBT but not between lesional and non-lesional skin at pre-TSEBT and post-TSEBT timepoints. (C) Analyses of α-diversity amongst local IR samples demonstrate no change with treatment or between lesional and non-lesional samples. (D) There were no differences in β-diversity between pre and post-local IR for either lesional or non-lesional samples, or between lesional and non-lesional samples at either pre-local IR or post-local IR timepoints. *p <0.05; **p<0.01; ns, not significant.
Pre-TSEBT versus post-TSEBT β-diversity differed significantly for lesional (p=0.001) and for non-lesional skin (p=0.005). By comparison, lesional versus non-lesional skin β-diversity did not differ significantly before (p=0.50) or after (p=0.21) TSEBT exposure (Figure 2B).
Pre- versus post-local IR did not demonstrate significant differences in α- or β-diversity (Figures 2C, D). This was true for both lesional pre- versus post-local IR and non-lesional pre versus post-local IR. Importantly, non-lesional pre- versus post-local IR serves as an internal control as these sites were not exposed to IR.
TSEBT increases the relative abundance of healthy commensal skin bacteria
In lesional skin, Streptococcus, Acinetobacter, and Roseomonas were significantly less abundant post- versus pre-TSEBT (p<0.01, q<0.05) while S. lugdunensis trended lower (p<0.05, q>0.05). Healthy commensals including genera Peptococcus and Cutibacterium, and family Anaerovoracaceae were higher in post-TSEBT lesional skin (p<0.0001, q<0.0001), and genera Moryella, Anaerococcus, and DNF00809, a member of the Eggerthellaceae family, trended higher (Figure 3A). Other bacteria that increased post-TSEBT included Trueperella, Olsenella, and Porphyromonas.
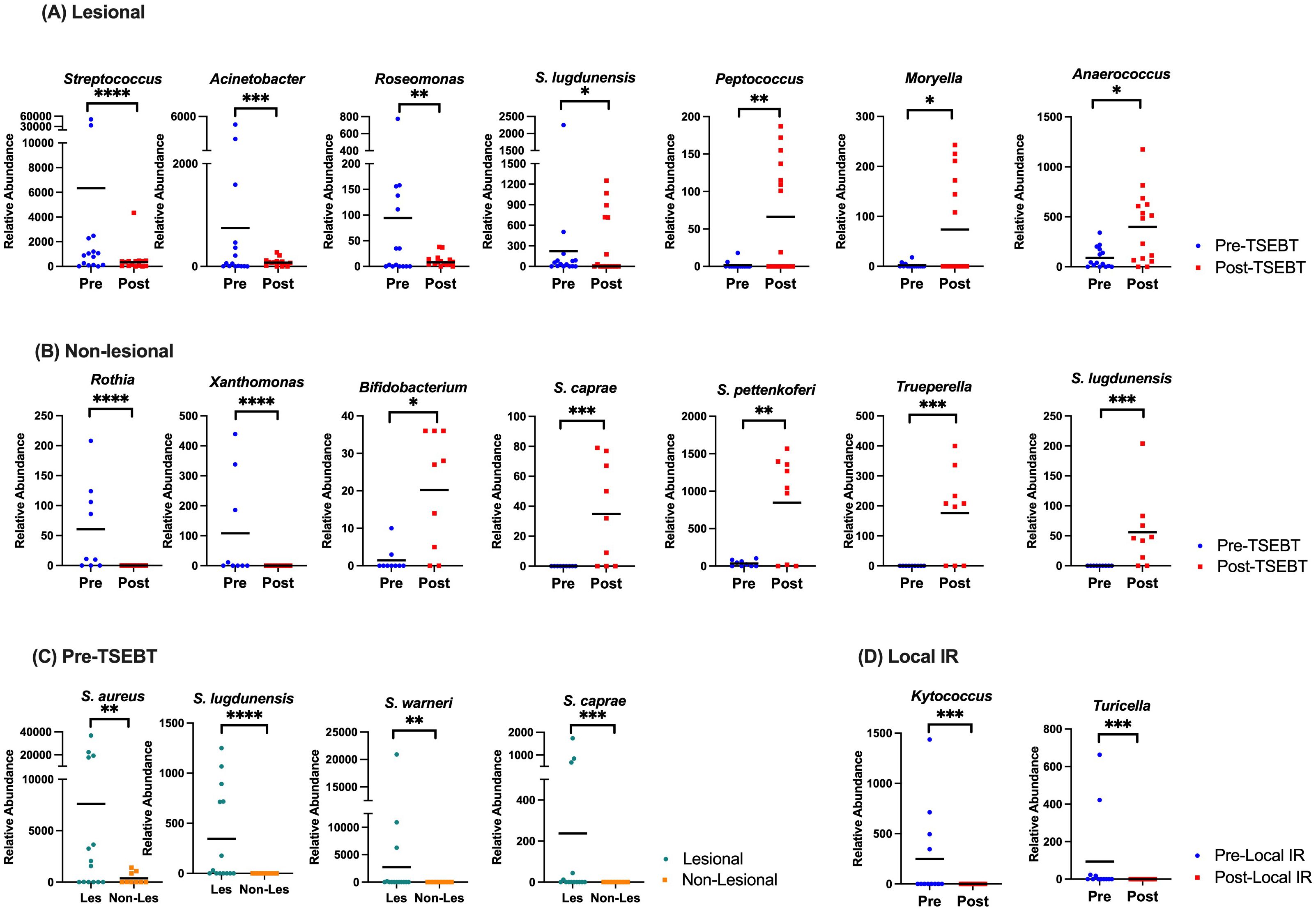
Figure 3. Taxon-by-taxon analyses amongst pre versus post-TSEBT skin and lesional versus non-lesional skin. (A) Analysis of lesional skin before TSEBT reveals increased relative abundance of Streptococcus and Roseomonas and other potentially pathogenic skin genera pre-TSEBT, and increases in the relative abundance of multiple anti-inflammatory taxa post-TSEBT. (B) Analysis of non-lesional skin reveals increased relative abundance of Rothia and other inflammatory taxa pre-TSEBT and increases in healthy commensals Bifidobacterium, S. caprae, and S. pettenkoferi post-TSEBT. (C) Species level Staphylococcus analyses of lesional versus non-lesional skin before TSEBT reveals increases in multiple taxa, including S. aureus. (D) Analysis of lesional skin reveals decrease in pathogenic Kytococcus and Turicella post-local IR compared to pre-local IR. The black line represents mean relative abundance. *p <0.05; **p<0.01; ***p<0.001; ****p<0.0001.
In non-lesional skin, Rothia, Xanthomonas, and Streptococcus were increased pre-TSEBT relative to post-TSEBT (p<0.01, q<0.05). In contrast, Bifidobacterium, S. caprae, S. pettenkoferi, S. lugdunensis, Trueperella and several members of the order Lactobacillales were increased post-TSEBT relative to pre-TSEBT (p<0.01, q<0.05) (Figure 3B).
At the species level, S. aureus, S. lugdunensis, S. warneri, and S. caprae were significantly more abundant in pre-TSEBT lesional versus non-lesional skin (p<0.001, q<0.05) (Figure 3C). Post-TSEBT, there were no significant differences between lesional and non-lesional skin.
In treated lesions, Kytococcus and Turicella were lower post-local IR compared to pre-local IR (p<0.0001, q<0.0001) (Figure 3D).
Healthy controls have significantly higher α-diversity than patients before, but not after, IR
Finally, we compared CTCL skin before and after any form of IR to matched HC skin. HC had significantly higher α-diversity compared to CTCL pre-IR (Observed p<0.0001), and microbial communities differed significantly (p=0.001) (Figure 4A). Relative abundances of S. aureus and S. haemolyticus were increased in CTCL patients, while S. hominis, Bifidobacterium, and several members of the order Lactobacillales were higher in HC. After IR, α-diversity no longer differed between HC and CTCL patients (p=0.53), whereas microbial communities remained distinct (p=0.001) (Figure 4B). DeSeq2 identified increased relative abundance of S. aureus, S. caprae, S. haemolyticus, and S. lugdunensis amongst CTCL patients. Comparison of pre-TSEBT patients versus HC and post-TSEBT versus HC revealed similar results to those seen in pre-IR versus HC and post-IR versus HC (Figures 4C, D).
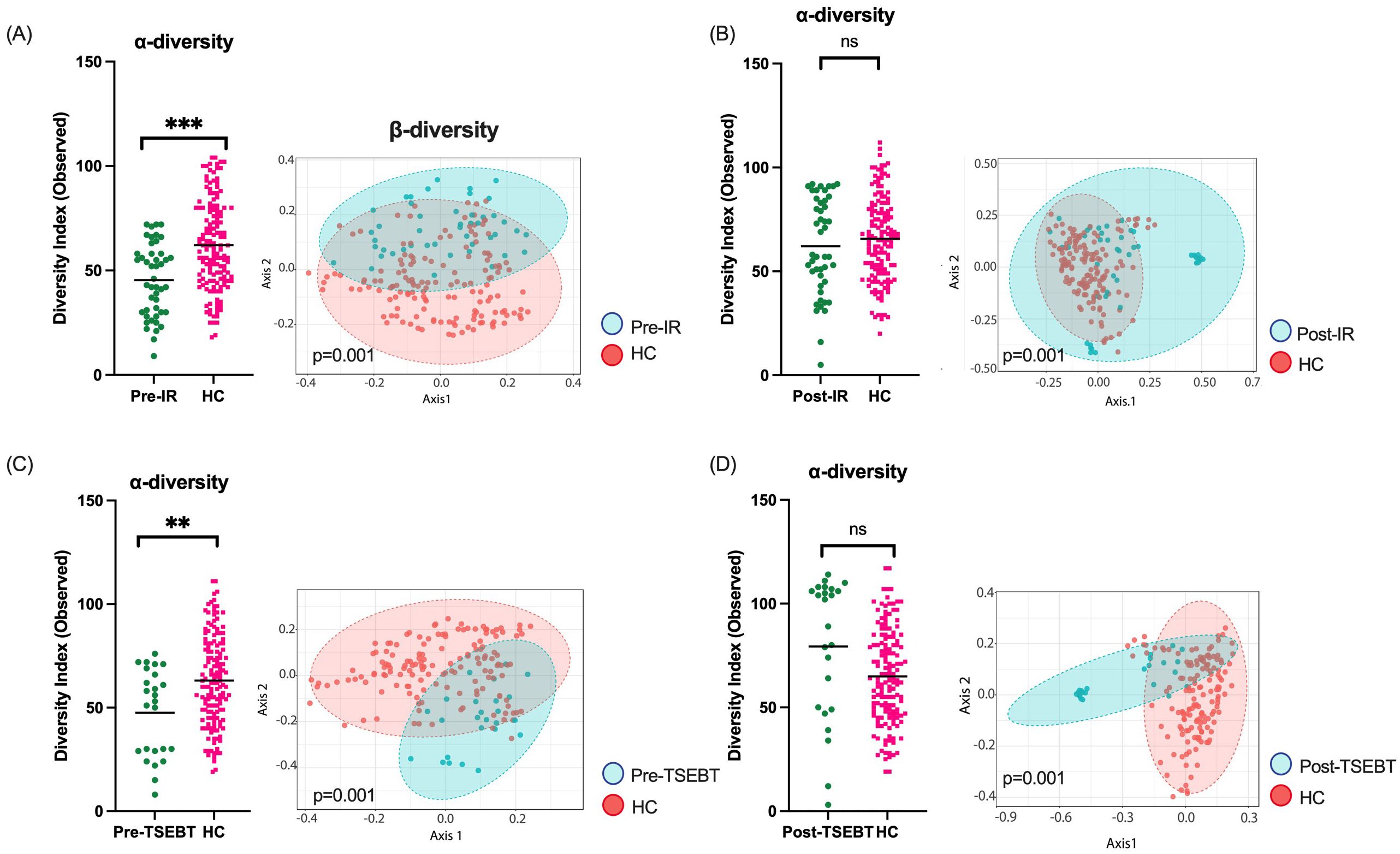
Figure 4. Healthy controls (HC) skin compared to CTCL skin before and after any form of IR and before and after TSEBT. (A) α-diversity was significantly lower in pre-IR patients than HC, and β-diversity differed significantly between the groups. (B) After any form of IR, CTCL skin α-diversity was no longer lower than that of HC, but β-diversities remained significantly different. (C) α-diversity was significantly lower in pre-TSEBT compared to HC skin, and β-diversity differed significantly between the groups. (D) Post-TSEBT α-diversity was no longer significantly different compared to HC but β-diversity remained different between post-TSEBT patients and HC. **p<0.01; ***p<0.001; ns, not significant.
Discussion
We utilized 16S rRNA and tuf amplicon sequencing to explore changes to the skin microbiome after IR. Having previously established changes to the CTCL microbiome with exposure to non-ionizing radiation (i.e. nbUVB), we sought to describe the relationship between the CTCL microbiome and IR (10, 11). To our knowledge, this is the first study to longitudinally describe the skin microbiome of CTCL patients before and after IR. Our results support our hypothesis that shifts in microbial communities reflect post-IR lesion improvement.
We previously demonstrated increased α-diversity in the skin of CTCL patients who responded to nbUVB therapy (10). Regardless of whether ionizing or non-ionizing, CTCL skin improvement with total body irradiation appears to increase α-diversity. Furthermore, given that all study patients improved after IR (average mSWAT change -25.3), we expected to see similar changes to those observed in nbUVB responders. Anaerococcus, which reduces S. aureus growth and maintains skin homeostasis (32), and S. pettenkoferi, a healthy skin commensal, tracked higher in post-TSEBT lesional skin and were both increased amongst post-nbUVB responders (10, 33). Furthermore, S. aureus appears to decrease with response to both IR and nbUVB (10).
While the α-diversity of both lesional and non-lesional skin increased following TSEBT, this finding was not replicated in lesions targeted with local IR. In TSEBT, electron beams penetrate the entire skin surface (34). Local IR, given its targeted nature, may not have as strong or as lasting of an effect. A small amount of radiation to a targeted location is likely unable to overcome the homeostasis maintained by the microbial community existing across the entire skin surface (35). At either the pre- or post-TSEBT timepoints, α-diversity did not differ between lesional and non-lesional skin, similar to prior CTCL microbiome studies. This is consistent with the paradigm that skin dysbiosis in inflammatory skin diseases manifests at both lesional and non-lesional sites (7, 36, 37).
For both skin types, the relative abundance of genera implicated in skin infection, including Streptococcus, Acinetobacter, Xanthomonas, and Rothia, were decreased post-TSEBT versus pre-TSEBT (38–41). A notable increase following TSEBT includes Moryella. Moryella is a member of the Lachnospiraceae family, which produces butyrate – a short chain fatty acid with potent histone deacetylase inhibitor activity, the mechanism of two FDA-approved treatments for CTCL (42). Furthermore, in a study examining radiation-induced skin injury, Lachnospiraceae correlated with rapid healing of radiation injuries as opposed to chronic ulcer formation (16). At the species level, S. aureus, a known potentiator of CTCL, was significantly higher in pre-TSEBT lesional skin compared to non-lesional skin, but this was not the case post-TSEBT (3, 4, 43). Notably, few potential opportunistic pathogens, such as Trueperella and Porphyromonas, were increased post-IR and post-TSEBT skin. However, these shifts were relatively few in comparison to reduction of other pathogenic bacteria and increase of healthy commensals. As microbial richness is recouped in both lesional and non-lesional skin after TSEBT, this taxonomic shift may reflect attenuation of pathogenic bacteria like Staphylococcus in favor of communities enriched with protective and anti-inflammatory taxa like Moryella. These findings also suggest that TSEBT does not drive additional skin dysbiosis in CTCL or create a persistent microbe-barren environment but rather allows for recolonization of commensal bacteria associated with healthy individuals.
When analyzing all CTCL skin exposed to any form of IR, α-diversity was significantly higher post-IR. However, when only analyzing lesional skin exposed to any form of IR, increased α-diversity was not observed. As such, changes in non-lesional CTCL skin likely account for the overall increase in α-diversity observed after IR. This conclusion is supported by our findings that in non-lesional skin exposed to IR, α-diversity was significantly higher post-IR. It is possible that the microbiome of lesional skin may take longer to repopulate healthy bacteria than non-lesional skin, as non-lesional CTCL skin may have milder dysbiosis. Post-IR collections were completed, on average, 30 days after treatment. Thus, it is conceivable that had collections occurred at time points further out from IR, α-diversity may have been higher in post-IR lesional skin when compared to pre-IR lesional skin. Genera enriched after IR exposure included Moryella and Bifidobacterium. It has been suggested that Bifidobacterium may suppress the Th2 phenotype and improve skin barrier function in an AD skin model (44, 45). Notably, CTCL is also driven by a Th2 phenotype (46). Additionally, the trend towards lower S. aureus abundance may also allow for healthy commensal recolonization.
Lastly, our results demonstrated HC had significantly higher α-diversity than CTCL patients before any form of IR. However, post-IR, α-diversity no longer differed between the two groups. These results suggest that in part, successful IR treatment may result from normalization of the CTCL skin microbiome towards that of HC.
A limitation of this study includes its relatively small sample size. However, longitudinal analyses of patients given a common intervention eliminates inter-individual variability and provides a far more robust dataset compared to typical cross-sectional datasets for determining microbes associated with disease progression and response. To reduce cofounders, our cohort was well-characterized and rigorously controlled for factors outside of IR which may impact the CTCL microbiome. We excluded patients who used any form of antibiotics within the prior 4 weeks. Moreover, we surveyed for potential confounders including differences in diet, co-morbidities, and associated medications and there were no significant differences between CTCL patients and age-matched healthy controls. In the future, stricter control of potential confounders including hygiene practices and environmental exposures such as ultraviolet radiation may improve study validity.
This work further supports increasing evidence that the host microbiome is closely associated with CTCL pathogenesis and treatment response. Future studies include exploring changes to the gut microbiome with IR therapy, and studying whether altering the skin microbiota pre-radiation may contribute to increased local control of individual lesions and enhanced abscopal responses. Elucidating the timeline on which microbial shifts occur will also be essential to fully understanding this relationship. Finally, larger studies with longer follow-up will increase the robustness of our results and allow us to explore whether microbial shifts are sustained over time. In demonstrating decreased pathogenic, and increased protective microbial species with IR, this work also contributes to increasing evidence that the skin microbiome mirrors CTCL disease severity. Furthermore, while IR is known to work through its direct apoptotic effects, our work suggests that IR may also help restore the skin microbiome through the renewal of healthy commensals, and reduction of pathogenic microbes that may directly or indirectly drive antigenic stimulation of malignant T-cells. In the future, better understanding of the relationship between the skin microbiome and IR may allow for microbially-optimized IR treatment strategies that maximize tumor clearance and systemic anti-tumor immunity.
Data availability statement
The data presented in the study are deposited in the NCBI short read archive, accession number PRJNA1100592.
Ethics statement
The studies involving humans were approved by Northwestern University Institutional Review Board (STU00209226) University of Chicago Institutional Review Board (IRB22-0595). The studies were conducted in accordance with the local legislation and institutional requirements. The participants provided their written informed consent to participate in this study.
Author contributions
LC: Data curation, Visualization, Writing – original draft, Writing – review & editing. YP: Writing – original draft, Writing – review & editing. MH: Data curation, Writing – review & editing. GR-K: Data curation, Investigation, Writing – review & editing. WN: Data curation, Writing – original draft, Writing – review & editing. SG: Formal analysis, Methodology, Resources, Software, Writing – review & editing. PS: Conceptualization, Investigation, Writing – review & editing. HL: Data curation, Formal analysis, Methodology, Writing – review & editing. BM: Data curation, Writing – review & editing. YH: Data curation, Writing – review & editing. JG: Investigation, Supervision, Writing – original draft, Writing – review & editing. RW: Formal analysis, Supervision, Writing – review & editing. MB: Formal analysis, Methodology, Resources, Software, Writing – review & editing. XZ: Conceptualization, Data curation, Funding acquisition, Investigation, Project administration, Resources, Supervision, Writing – original draft, Writing – review & editing.
Funding
The author(s) declare financial support was received for the research, authorship, and/or publication of this article. Supported by a Cutaneous Lymphoma Foundation Catalyst Research Grant, American Cancer Society Institutional Research Grant, Lymphoma Research Foundation Clinical Career Development Award, Gilead Research Scholars Program Award in Hematologic Malignancies, and an institutional grant from the Northwestern University Clinical and Translational Sciences Institute (NUCATS) and the National Institutes of Health (NIH) (GRANT KL2TR001424).
Acknowledgments
The authors would like to thank the patients who contributed to this study.
Conflict of interest
The authors declare that the research was conducted in the absence of any commercial or financial relationships that could be construed as a potential conflict of interest.
Generative AI statement
The author(s) declare that no Generative AI was used in the creation of this manuscript.
Publisher’s note
All claims expressed in this article are solely those of the authors and do not necessarily represent those of their affiliated organizations, or those of the publisher, the editors and the reviewers. Any product that may be evaluated in this article, or claim that may be made by its manufacturer, is not guaranteed or endorsed by the publisher.
Supplementary material
The Supplementary Material for this article can be found online at: https://www.frontiersin.org/articles/10.3389/fimmu.2024.1520214/full#supplementary-material
References
1. Dulmage BO, Kong BY, Holzem K, Guitart J. What is new in CTCL—Pathogenesis, diagnosis, and treatments. Curr Dermatol Rep. (2018) 7:91–8. doi: 10.1007/s13671-018-0214-0
2. Willerslev-Olsen A, Krejsgaard T, Lindahl LM, Bonefeld CM, Wasik MA, Koralov SB, et al. Bacterial toxins fuel disease progression in cutaneous T-cell lymphoma. Toxins (Basel). (2013) 5:1402–21. doi: 10.3390/toxins5081402
3. Blümel E, Munir Ahmad S, Nastasi C, Willerslev-Olsen A, Gluud M, Fredholm S, et al. Staphylococcus aureus alpha-toxin inhibits CD8(+) T cell-mediated killing of cancer cells in cutaneous T-cell lymphoma. Oncoimmunology. (2020) 9:1751561. doi: 10.1080/2162402X.2020.1751561
4. Blümel E, Willerslev-Olsen A, Gluud M, Lindahl LM, Fredholm S, Nastasi C, et al. Staphylococcal alpha-toxin tilts the balance between Malignant and non-malignant CD4(+) T cells in cutaneous T-cell lymphoma. Oncoimmunology. (2019) 8:e1641387. doi: 10.1080/2162402X.2019.1641387
5. Hooper MJ, LeWitt TM, Pang Y, Veon FL, Chlipala GE, Feferman L, et al. Gut dysbiosis in cutaneous T-cell lymphoma is characterized by shifts in relative abundances of specific bacterial taxa and decreased diversity in more advanced disease. J Eur Acad Dermatol Venereol. (2022) 36:1552–63. doi: 10.1111/jdv.18125
6. Hooper MJ, LeWitt TM, Veon FL, Pang Y, Chlipala GE, Feferman L, et al. Nasal dysbiosis in cutaneous T-cell lymphoma is characterized by shifts in relative abundances of non-staphylococcus bacteria. JID Innov. (2022) 2:100132. doi: 10.1016/j.xjidi.2022.100132
7. Zhang Y, Seminario-Vidal L, Cohen L, Hussaini M, Yao J, Rutenberg D, et al. Alterations in the skin microbiota are associated with symptom severity in mycosis fungoides. Front Cell Infect Microbiol. (2022) 12:850509. doi: 10.3389/fcimb.2022.850509
8. Lindahl LM, Willerslev-Olsen A, Gjerdrum LMR, Nielsen PR, Blümel E, Rittig AH, et al. Antibiotics inhibit tumor and disease activity in cutaneous T-cell lymphoma. Blood. (2019) 134:1072–83. doi: 10.1182/blood.2018888107
9. Tandberg DJ, Craciunescu O, Kelsey CR. Radiation therapy for cutaneous T-cell lymphomas. Dermatologic Clinics. (2015) 33:703–13. doi: 10.1016/j.det.2015.05.006
10. Hooper MJ, Enriquez GL, Veon FL, LeWitt TM, Sweeney D, Green SJ, et al. Narrowband ultraviolet B response in cutaneous T-cell lymphoma is characterized by increased bacterial diversity and reduced Staphylococcus aureus and Staphylococcus lugdunensis. Front Immunol. (2022) 13:1022093. doi: 10.3389/fimmu.2022.1022093
11. Nguyen WQ, Chrisman LP, Enriquez GL, Hooper MJ, Griffin TL, Ahmad M, et al. Gut microbiota analyses of cutaneous T-cell lymphoma patients undergoing narrowband ultraviolet B therapy reveal alterations associated with disease treatment. Front Immunol. (2024) 14. doi: 10.3389/fimmu.2023.1280205
12. Patra V, Wagner K, Arulampalam V, Wolf P. Skin microbiome modulates the effect of ultraviolet radiation on cellular response and immune function. iScience. (2019) 15:211–22. doi: 10.1016/j.isci.2019.04.026
13. Trautinger F. Phototherapy of cutaneous T-cell lymphomas. Photochem Photobiol Sci. (2018) 17:1904–12. doi: 10.1039/c8pp00170g
14. Richardson BN, Lin J, Buchwald ZS, Bai J. Skin microbiome and treatment-related skin toxicities in patients with cancer: A mini-review. Front Oncol. (2022) 12. doi: 10.3389/fonc.2022.924849
15. Ramadan M, Hetta HF, Saleh MM, Ali ME, Ahmed AA, Salah M. Alterations in skin microbiome mediated by radiotherapy and their potential roles in the prognosis of radiotherapy-induced dermatitis: a pilot study. Sci Rep. (2021) 11:5179. doi: 10.1038/s41598-021-84529-7
16. Huang B, An L, Su W, Yan T, Zhang H, Yu D-J. Exploring the alterations and function of skin microbiome mediated by ionizing radiation injury. Front Cell Infection Microbiol. (2022) 12. doi: 10.3389/fcimb.2022.1029592
17. Naqib A, Poggi S, Wang W, Hyde M, Kunstman K, Green SJ. Making and sequencing heavily multiplexed, high-throughput 16S ribosomal RNA gene amplicon libraries using a flexible, two-stage PCR protocol. Methods Mol Biol. (2018) 1783:149–69. doi: 10.1007/978-1-4939-7834-2_7
18. Walters W, Hyde ER, Berg-Lyons D, Ackermann G, Humphrey G, Parada A, et al. Improved bacterial 16S rRNA gene (V4 and V4-5) and fungal internal transcribed spacer marker gene primers for microbial community surveys. mSystems. (2016) 1. doi: 10.1128/msystems.00009-15
19. Martin M. Cutadapt removes adapter sequences from high-throughput sequencing reads. EMBnetjournal. (2011) 17:3.
20. Callahan BJ, McMurdie PJ, Rosen MJ, Han AW, Johnson AJA, Holmes SP. DADA2: High-resolution sample inference from Illumina amplicon data. Nat Methods. (2016) 13:581–3. doi: 10.1038/nmeth.3869
21. Quast C, Pruesse E, Yilmaz P, Gerken J, Schweer T, Yarza P, et al. The SILVA ribosomal RNA gene database project: improved data processing and web-based tools. Nucleic Acids Res. (2013) 41:D590–6. doi: 10.1093/nar/gks1219
22. Murali A, Bhargava A, Wright ES. IDTAXA: a novel approach for accurate taxonomic classification of microbiome sequences. Microbiome. (2018) 6:140. doi: 10.1186/s40168-018-0521-5
23. Davis NM, Proctor DM, Holmes SP, Relman DA, Callahan BJ. Simple statistical identification and removal of contaminant sequences in marker-gene and metagenomics data. Microbiome. (2018) 6:226. doi: 10.1186/s40168-018-0605-2
24. Ahle CM, Stødkilde K, Afshar M, Poehlein A, Ogilvie LA, Söderquist B, et al. Staphylococcus saccharolyticus: an overlooked human skin colonizer. Microorganisms. (2020) 8:1105. doi: 10.3390/microorganisms8081105
25. Ahle C, Stødkilde-Jørgensen K, Poehlein A, Streit W, Hüpeden J, Brüggemann H. Comparison of three amplicon sequencing approaches to determine staphylococcal populations on human skin. BMC Microbiol. (2021) 21. doi: 10.1186/s12866-021-02284-1
26. McMurdie PJ, Holmes S. phyloseq: an R package for reproducible interactive analysis and graphics of microbiome census data. PloS One. (2013) 8:e61217. doi: 10.1371/journal.pone.0061217
28. Oksanen J, Blanchet FG, Kindt R, Legendre P, Minchin P, O’Hara B, et al. Vegan: community ecology package. R Package Version 22-1. (2015) 2:1–2.
29. Love MI, Huber W, Anders S. Moderated estimation of fold change and dispersion for RNA-seq data with DESeq2. Genome Biol. (2014) 15:550. doi: 10.1186/s13059-014-0550-8
30. Roberts JS, Atanasova KR, Lee J, Diamond G, Deguzman J, Hee Choi C, et al. Opportunistic pathogen porphyromonas gingivalis modulates danger signal ATP-mediated antibacterial NOX2 pathways in primary epithelial cells. Front Cell Infect Microbiol. (2017) 7:291. doi: 10.3389/fcimb.2017.00291
31. Rzewuska M, Kwiecień E, Chrobak-Chmiel D, Kizerwetter-Świda M, Stefańska I, Gieryńska M. Pathogenicity and virulence of trueperella pyogenes: A review. Int J Mol Sci. (2019) 20:2737. doi: 10.3390/ijms20112737
32. van der Krieken DA, Rikken G, Ederveen THA, Jansen PAM, Rodijk-Olthuis D, Meesters LD, et al. Gram-positive anaerobic cocci guard skin homeostasis by regulating host-defense mechanisms. iScience. (2023) 26:106483. doi: 10.1016/j.isci.2023.106483
33. Kierzkowska M, Markowska K, Majewska A. Knowledge, attitude and practice regarding staphylococcus pettenkoferi. Infect Dis Rep. (2022) 14:112–20. doi: 10.3390/idr14010015
34. Chowdhary M, Song A, Zaorsky NG, Shi W. Total skin electron beam therapy in mycosis fungoides—a shift towards lower dose? Chin Clin Oncol. (2018) 8:9. doi: 10.21037/cco.2018.09.02
35. Smythe P, Wilkinson HN. The skin microbiome: current landscape and future opportunities. Int J Mol Sci. (2023) 24:3950. doi: 10.3390/ijms24043950
36. Łyko M, Jankowska-Konsur A. The skin microbiome in cutaneous T-cell lymphomas (CTCL)-A narrative review. Pathogens. (2022) 11:935. doi: 10.3390/pathogens11080935
37. Salava A, Deptula P, Lyyski A, Laine P, Paulin L, Väkevä L, et al. Skin microbiome in cutaneous T-cell lymphoma by 16S and whole-genome shotgun sequencing. J Invest Dermatol. (2020) 140:2304–8.e7. doi: 10.1016/j.jid.2020.03.951
38. Morgan EA, Henrich TJ, Jarell AD, Shieh WJ, Zaki SR, Marty FM, et al. Infectious granulomatous dermatitis associated with Rothia mucilaginosa bacteremia: A case report. Am J Dermatopathol. (2010) 32:175–9. doi: 10.1097/DAD.0b013e3181b1c5ad
39. Bystritsky RJ. Cellulitis. Infect Dis Clin North Am. (2021) 35:49–60. doi: 10.1016/j.idc.2020.10.002
40. Ji C, Guo W, Amir H. Experience of diagnosis and treatment of hard-to-heal wounds infected with Acinetobacter baumannii: a case study. J Wound Care. (2024) 33:278–85. doi: 10.12968/jowc.2024.33.4.278
41. Burns RL, Lowe L. Xanthomonas maltophilia infection presenting as erythematous nodules. J Am Acad Dermatol. (1997) 37:836–8. doi: 10.1016/S0190-9622(97)80006-0
42. Jain S, Zain J, O’Connor O. Novel therapeutic agents for cutaneous T-Cell lymphoma. J Hematol Oncol. (2012) 5:24. doi: 10.1186/1756-8722-5-24
43. Krejsgaard T, Willerslev-Olsen A, Lindahl LM, Bonefeld CM, Koralov SB, Geisler C, et al. Staphylococcal enterotoxins stimulate lymphoma-associated immune dysregulation. Blood. (2014) 124:761–70. doi: 10.1182/blood-2014-01-551184
44. Choi M, Lee Y, Lee NK, Bae CH, Park DC, Paik HD, et al. Immunomodulatory effects by bifidobacterium longum KACC 91563 in mouse splenocytes and macrophages. J Microbiol Biotechnol. (2019) 29:1739–44. doi: 10.4014/jmb.1812.12002
45. Kim S, Han SY, Lee J, Kim NR, Lee BR, Kim H, et al. Bifidobacterium longum and galactooligosaccharide improve skin barrier dysfunction and atopic dermatitis-like skin. Allergy Asthma Immunol Res. (2022) 14:549–64. doi: 10.4168/aair.2022.14.5.549
Keywords: cutaneous T-cell lymphoma, microbiome, radiation, radiotherapy, total skin electron beam therapy, skin cancer, lymphoma
Citation: Chrisman LP, Pang Y, Hooper MJ, Rajeev-Kumar G, Nguyen WQ, Green SJ, Seed PC, Liang H, Mittal BB, Hasan Y, Guitart J, Weichselbaum RR, Burns MB and Zhou XA (2024) Ionizing radiation improves skin bacterial dysbiosis in cutaneous T-cell lymphoma. Front. Immunol. 15:1520214. doi: 10.3389/fimmu.2024.1520214
Received: 30 October 2024; Accepted: 06 December 2024;
Published: 24 December 2024.
Edited by:
Somya Aggarwal, Washington University in St. Louis, United StatesReviewed by:
Facundo Fiocca Vernengo, Charité Medical University of Berlin, GermanyManasi Balachandran, University of Tennessee Medical Center, United States
Copyright © 2024 Chrisman, Pang, Hooper, Rajeev-Kumar, Nguyen, Green, Seed, Liang, Mittal, Hasan, Guitart, Weichselbaum, Burns and Zhou. This is an open-access article distributed under the terms of the Creative Commons Attribution License (CC BY). The use, distribution or reproduction in other forums is permitted, provided the original author(s) and the copyright owner(s) are credited and that the original publication in this journal is cited, in accordance with accepted academic practice. No use, distribution or reproduction is permitted which does not comply with these terms.
*Correspondence: Xiaolong A. Zhou, YWxhbi56aG91QG5vcnRod2VzdGVybi5lZHU=
†These authors have contributed equally to this work and share senior authorship