- 1Department of Medicine, Aab Cardiovascular Research Institute, University of Rochester School of Medicine and Dentistry, Rochester, NY, United States
- 2Department of Physical Medicine and Rehabilitation, University of Rochester School of Medicine and Dentistry, Rochester, NY, United States
- 3Department of Translational Biomedical Science, University of Rochester School of Medicine and Dentistry, Rochester, NY, United States
- 4Department of Biomedical Genetics, University of Rochester School of Medicine and Dentistry, Rochester, NY, United States
- 5Department of Kinesiology, University of Texas at San Antonio, San Antonio, TX, United States
- 6Department of Molecular Microbiology and Immunology, University of Texas at San Antonio, San Antonio, TX, United States
Introduction: The severity of spinal cord injury (SCI) is closely tied to pulmonary function, especially in cases of higher SCI levels. Despite this connection, the underlying pathological mechanisms in the lungs post-SCI are not well understood. Previous research has established a connection between disrupted sympathetic regulation and splenocyte apoptosis in high thoracic SCI, leading to pulmonary dysfunction. The aim of this study was to investigate whether mice with low-level SCI exhibit increased susceptibility to acute lung injury by eliciting systemic inflammatory responses that operate independently of the sympathetic nervous system.
Methods: Here, we employed T9 contusion SCI and exposed mice to aerosolized lipopolysaccharide (LPS) to simulate lung inflammation associated with acute respiratory distress syndrome (ARDS). Twenty-four hours post-LPS exposure, lung tissues and bronchoalveolar lavage (BAL) fluid were analyzed.
Results: LPS markedly induced proinflammatory gene expression (SAA3, IRG1, NLRP3, IL-1beta, MCP-1) and cytokine release (IL-1beta, IL-6, MCP-1) in SCI mice compared to controls, indicating an exaggerated inflammatory response. Infiltration of Ly6G/C positive neutrophils and macrophages was significantly higher in SCI mice lungs post-LPS exposure. Interestingly, spleen size and weight did not differ between control and SCI mice, suggesting that T9 SCI alone does not cause spleen atrophy. Notably, bone-marrow-derived macrophages (BMDMs) from SCI mice exhibited hyper-responsiveness to LPS.
Discussions: This study demonstrated an increase in lung inflammation and immune responses subsequent to low-level T9 SCI, underscoring the widespread influence of systemic inflammation post-SCI, especially pronounced in specific organs like the lungs.
Highlights
● Spinal cord injury leads to increased lung permeability following LPS inhalation.
● Spinal cord injury amplifies cytokine release after exposure to LPS.
● Spinal cord injury affects bone marrow-derived macrophages, showing heightened glycolytic activity and inflammatory conditions.
Introduction
Individuals with spinal cord injury (SCI) face an elevated risk of respiratory complications, with acute lung injury (ALI) and acute respiratory distress syndrome (ARDS) emerging as prominent contributors to morbidity and mortality in this population (1, 2). Notably, pneumonia is a primary contributor to premature mortality in SCI patients, even when considering factors such as immobility, aspiration, and ventilator utilization (3). Importantly, it serves as an independent risk factor contributing to unfavorable neurological and functional long-term outcomes (3).
The underlying reasons for the increased susceptibility to respiratory challenges in SCI patients are multifaceted. First, high-level SCI results in changes in central nervous system (CNS)- immune system interactions (4, 5). “Higher SCI levels” refers to injuries occurring above the T6 level, which disrupt brainstem control over spinal sympathetic outflow. These injuries often lead to more severe neurological deficits and autonomic dysfunction. In contrast, “low-level SCI” refers to injuries occurring below the T6 level, typically resulting in less severe disruption of sympathetic function (6–8). Specifically, higher SCI disrupting the hypothalamic-pituitary-adrenal axis that regulates sympathetic nervous system (SNS) activity causes a hyperadrenergic state (9). Increased catecholamines due to stress-related noradrenergic overactivation decrease blood flow to the abdominal organs, especially the spleen, causing splenic atrophy. Furthermore, high-level SCI stimulates the production and secretion from the adrenal gland of glucocorticoids (GCs) that promote apoptosis of immune cells, particularly lymphocytes. As a result, the incidence of infections is higher in the SCI population, particularly those with severe, high-level SCI, than in the able-bodied population (10–13). Second, increased local as well as systemic inflammation serves as a catalyst for ALI and ARDS. The initial phase of SCI, occurring within a few hours, is characterized by the release of myelin debris, ATP, Ca2+, intracellular proteins, and mitochondrial DNA from damaged cells. These triggers induce inflammatory responses from local microglia, astrocytes, and circulating immune cells (14). The subsequent phase involves cytokines and activated immune cells that prolong inflammation within SCI lesions, exacerbating tissue damage and causing systemic inflammation (15, 16).
Prior investigations in mice have demonstrated that a high thoracic (T3) injury disrupts sympathetic regulation of lymphoid organs, leading to splenocyte apoptosis (4, 17), and impairs lung immunity (17) during the acute phase of spinal cord injury (SCI). However, the effects of T9 (lower level) SCI on lung immunity, in particular during the sub-acute and chronic phases post-SCI and in the absence of a hyperactivated sympathetic nervous system are less well understood (18–20). While respiratory complications, including pneumonia, are prevalent during acute care, particularly among ventilator-dependent patients, respiratory diseases manifest in 30% of SCI patients throughout their lifetime, irrespective of SCI lesion type (21), but a formal accounting of the incidence of these respiratory diseases in individuals with SCI is currently unavailable. This study addresses this gap by establishing a robust animal model to investigate the impact of acute lung injury (ALI) on lung inflammation post-T9 SCI. Understanding the intricate mechanisms involved in SCI-induced respiratory complications is critical for advancing therapeutic strategies and improving acute and chronic outcomes for individuals with spinal cord injuries (22).
Materials and methods
Reagents
Medium: Dulbecco’s modified eagle medium (MT-10-013-CV), Fetal bovine serum (10437-028), Sodium pyruvate (11360070), and Streptomycin/penicillin (15140-122) were from Thermo Fisher Scientific (MA, USA). Chemical: ABC-HRP conjugate (PK-6100), Citrate Buffer pH 6.0, 10x (21545). Cell Lysis Buffer (10X) (9803s) was from Cell Signaling Technology (MA, USA). DAPI Fluoromount-G (0100-20) was from SouthernBiotech (AL, USA). Lipopolysaccharides from Escherichia coli (L3129), Heparin (H3149-25KU), Protease inhibitor cocktail (P8340), and EDTA solution (BP24831), Ketamine (K0001) was from SMH Pharmacy (NY, USA). Normal goat serum (S-1000) were from Vector Laboratories (CA, USA). RTL lysis buffer (79216) was from Qiagen (Hilden, Germany). Saline (2F7123) was from Cardinal Health (OH, Ireland). Xylazine (005469) was from Bimeda (IL, USA). Assay kit: Bicinchoninic acid (BCA) kit (BCA-1) was from Pierce (IL, USA), iScript™ cDNA Synthesis Kit (1708891) and iQ™ SYBR® Green Supermix (1708882) were from Bio-Rad Laboratories (CA, USA). MCP-1 (RUO 432704), TNFα (RUO 430904), IL-1β (RUO 432604) and IL-6 (RUO 431304) ELISA kits were from BioLegend (CA, USA). Antibody: Alexa Fluor 488 Goat Anti-rabbit IgG(H+L) highly cross-adsorbed (A-11034) was from Molecular Probes (OR, USA). Ly6G&6C antibody (550291) from BD Biosciences (New Jersey, USA).
Animals
Female C57BL/6N mice were purchased from (Jackson Laboratory, 000664) aged 12 weeks were used in the experiments. (Victor Chang Cardiac Research Institute). All mice were housed in a specific pathogen-free facility and kept in a temperature-controlled room set to a light and dark cycle of 12 hours each. The mice had ad libitum access to standard mouse chow and water. All of the experiments were approved by the University Committee on Animal Use For Research (UCAR) at the University of Rochester and followed National Institutes of Health guidelines for experimental procedures on mice.
Mouse spinal cord contusion injury
Mice aged 12 weeks were anesthetized with a combination of intraperitoneal ketamine (100 mg/kg) and xylazine (10 mg/kg). If animals responded to painful stimuli (e.g., toe pinch), the anesthetic dose was immediately boosted with a ketamine booster injection (0.5 times the initial dose of ketamine). Signs of anesthesia were monitored frequently during and after the procedure. A laminectomy was performed at the thoracic 9 (T9) level, exposing the dura mater. The spinal cord underwent moderate bilateral contusion using a force-defined injury device (65 kDyn, Infinite Horizon Impactor 400, Precision Systems and Instrumentation). The impact force and displacement were recorded for quality control (Supplementary Figure 1). The injury site was closed in layers, encompassing muscle and skin. A heating pad maintained body temperature at 37°C during surgery and the initial recovery period. Postoperative pain relief was provided through the administration of Buprenorphine SR (0.5-1 mg/kg, subcutaneously 1 hour prior to surgery surgery) and the topical application of xylocaine ointment. To further minimize discomfort, hemostatic gel foam was used to cover the exposed spinal cord, and the muscles and skin were closed in layers. The animal was then returned to a heated cage, and DietGel Recovery was provided to support recovery. Postoperative animals were housed individually for the first 24 hours to optimize care and reduce stress. Additionally, bladders were manually expressed twice daily until reflexive urination returned. Animals were closely monitored daily for signs of pain, such as excessive porphyrin production, lethargy, weight loss, or failure to eat, drink, or groom.
LPS inhalation in vivo
Ten days post-SCI, mice were exposed to aerosolized lipopolysaccharide (LPS). Age-matched female mice with SCI and control mice were subjected to LPS inhalation. The mice were randomly positioned within stainless steel compartments located in a polycarbonate rectangular chamber, connected to a nebulizer on one side and a vacuum system on the other. The LPS exposure dosage and duration adhered to established parameters from prior research (23). A solution of LPS (10mg/10mL saline) was aerosolized into the chamber for 30 minutes, while control mice received aerosolized saline solution. Following complete aerosolization, mice were removed from the chamber and returned to the room. We carefully monitored the mice’s behavior at 2, 6, 12, and 24 hours after exposure. Importantly, whole-body inhalation of LPS did not induce any pain or distress in the mice, as confirmed by our behavioral assessments at these time points.
Bone marrow progenitor cell isolation and bone marrow-derived macrophage differentiation
BMDMs preparation was performed as previously described (24). L929 conditioned media which contains the macrophage growth factor M-CSF, was prepared by culturing L929 cells (ATCC) in complete DMEM (Thermo Fisher Scientific, MT10013CV) supplemented with 10% FBS, and 1% penicillin and streptomycin for 10 days at 37°C, 5% CO2. The L929 conditioned media, was collected, filtered (Vacuum Filter/Storage Bottle System, Corning, 431153), and stored at −80 °C until required. For isolation of BMDMs, tibias and femurs were removed from both male and female mice and flushed with media using a 26-gauge needle. Bone marrow was collected at 500 x g for 2 min at 4°C, resuspended with complete DMEM medium and filtered through a 70-μm cell strainer (VWR international, 10199-657). Bone marrow progenitor cells were cultured in 100 mm dishes for 6-7 days in 70% complete DMEM medium and 30% L929-conditioned medium. Fresh medium (5 mL) was added on day 3. BMDMs were collected by scraping in cold PBS containing EDTA (1 mM). After centrifugation, BMDMs were seeded into 12-well plates at a density of 1.6 × 105 cells/well in DMEM and incubated overnight before use.
LPS stimulation in vitro
To stimulate BMDMs, cultures were rinsed twice with serum and antibiotic-free DMEM medium. The medium was replaced with fresh with serum and antibiotic-free DMEM medium. Cells were stimulated with or without LPS (100 ng/mL) for 3 hours.
Collection of BAL fluid and lung tissue
Mice were anesthetized with a ketamine/xylazine intraperitoneal injection before euthanasia via cervical dislocation. The upper part of the trachea was cannulated and lavaged with 1 mL followed by 0.5 mL of PBS supplemented with 1 mM EDTA. Bronchoalveolar lavage (BAL) fluid underwent centrifugation at 500 x g for 5 min at 4°C. The resulting cell pellets from BAL fluid were lysed, and the protein content of the resulting cell lysates was measured using a BCA kit. Cytokines and chemokines were analyzed in BAL fluids. The superior lobe was rapidly frozen in liquid nitrogen for quantifying gene expression and homogenized in RTL lysis buffer.
Collection of lung tissue for immunofluorescent staining
Mice were euthanized via intraperitoneal injection of ketamine (130 mg/kg) and xylazine (8.8mg/kg). The lungs were perfused free of blood by gentle infusion of 10 ml PBS containing 1 mM EDTA through the right ventricle. Lungs were inflated with 2mL, PBS-equilibrated 4% formaldehyde (VWR International, PI28908). Lung tissues were removed, drop fixed in 4% formaldehyde, embedded in paraffin, cut into 5 μm sections, and mounted onto slides. Sections were deparaffinized before use. Sections were washed 3 times in PBS followed by antigen retrieval for 20 minutes with steam using 1X Citrate buffer (Millipore, 21545), pH=6.0. Sections were blocked in 10% normal goat serum (Vector Laboratories, S-1000) in PBS for 1 hour at room temperature followed by overnight incubation at 4°C with 1:500 Ly6G&6C antibody (BD Biosciences, 550291) in 2% normal goat serum in PBS overnight. After three washes with PBS, fluorescence-conjugated secondary antibodies (Molecular Probes, A-11034 or A-11081) 1:1000 were incubated for 1 hour at room temperature and followed by three washes with PBS. Nuclei were stained with DAPI-fluoromount-G (Southern Biotech, 0100-20). Fluorescent images were captured using a confocal microscope (Olympus BX51, Software: SPOT Imaging software advanced). Ly6G&6C and DAPI positive cells were quantified by using NIH ImageJ software.
Cytokine assay
BAL fluid samples were cleared by centrifugation at 16k×g for 5 min and stored at −20°C. MCP-1, IL-6, TNFα and IL-1β levels were measured by ELISA kits according to the manufacturer’s instructions. Plasma samples were analyzed by Eve Technologies using the Mouse Cytokine Proinflammatory Focused 10-Plex Discovery Assay® Array (MDF10), which measures the following cytokines: GM-CSF, IFNγ, IL-1β, IL-2, IL-4, IL-6, IL-10, IL-12, MCP-1, and TNF-α.
RNA extraction and real-time PCR
The superior lobe of the lung tissues was used for RNA extraction and Real-time PCR. Complementary DNA was generated from 0.1 μg (cell) or 0.5 μg (tissue) RNA through reverse transcription. Amplification reactions included a target-specific portion of the reverse transcription product and 1 μM forward and reverse primers in SYBR Green qPCR master mix. Fluorescence was analyzed using a CFX connect real-time PCR system. Gene expression was normalized with β-actin and an independent reference sample using the delta delta CT method. Specific transcript amplification was confirmed by melting curve analysis at the end of each PCR experiment. The primer sequences are provided in Table 1.
Statistics
Comparisons between SCI and control groups after LPS exposure were analyzed by two-way ANOVA followed by post hoc T tests using Bonferroni correction for multiple comparisons. In vitro experiments were repeated as three independent procedures, with duplicate or triplicate wells averaged prior to statistical analysis. All data were presented as mean ± SEM. GraphPad Prism 10.0 was used for statistical analysis. P values were indicated as follow: * < 0.05, ** < 0.01, *** < 0.001, **** < 0.0001.
Results
The extent of pulmonary function impairment varies based on the severity of spinal cord injury (SCI), with more pronounced dysfunction observed at higher injury levels. Recent research has demonstrated that a high thoracic (T3) injury disrupts crucial sympathetic regulation of lymphoid organs, leading to splenocyte apoptosis (4, 17). In the case of T9 SCI, there is no hyperactivation of the sympathetic nervous system, but the injury triggers systemic inflammatory responses (22). Limited information is available regarding the impact of low SCI on immune defense mechanisms in the lungs. In this investigation, we conducted T9 contusion SCI using the Infinite Horizon Impactor (25). Ten days post-SCI, mice were exposed to aerosolized lipopolysaccharide (LPS), a bacterial endotoxin known to initiate a pro-inflammatory response in mouse lungs. This LPS model mimics a natural route of entry into the host respiratory tract and replicates aspects of the inflammatory cascades associated with lung inflammation and acute respiratory distress syndrome (ARDS) in humans (23). Therefore, it serves as a valuable model for studying the effects of SCI that may amplify these cascades and intensify the disease process. SCI and control mice were subjected to a 30-minute exposure to LPS within stainless steel compartments placed inside a polycarbonate rectangular chamber, while control mice received aerosolized saline solution (23).
At 24 hours post-exposure to LPS, lung tissues and bronchoalveolar lavage (BAL) fluid were collected. Initially, we assessed lung injury and the expression of proinflammatory cytokine genes in lung tissues. SAA3 (Serum Amyloid A3) is an acute-phase protein that plays a key role in the inflammatory response (26). IRG1 (Immunoresponsive gene 1) is involved in macrophage activation and inflammation (27). NLRP3 (NOD-like receptor family pyrin domain containing 3) is a critical component of the inflammasome, which activates pro-inflammatory cytokines such as IL-1β (28–30). IL-1β (Interleukin-1 beta) is a potent pro-inflammatory cytokine that plays a central role in initiating and propagating the inflammatory response (28). MCP-1 (Monocyte Chemoattractant Protein-1) is a chemokine that recruits monocytes to sites of inflammation (31). TNF-α (Tumor Necrosis Factor-alpha) is a major cytokine involved in systemic inflammation and immune regulation (32). Following exposure to saline, there was no difference in proinflammatory cytokine gene expression between control and SCI mice. LPS significantly increased the expression levels of SAA3, IRG1, NLRP3, IL-1β, MCP-1, and TNFα mRNA in lung tissues from all animals. However, these elevations were significantly greater in lung tissues from SCI mice (with the exception of TNFα, Figures 1A–F). One possible explanation for the lack of difference in TNF-α expression between SCI and non-SCI animals is that TNF-α is a central mediator of inflammation and is typically produced as an early response (3-6 hours) to LPS stimuli. Since the lung tissues were collected 24 hours after LPS exposure, the fold changes in TNF-α expression were relatively modest (only 1.5–3 times that of the control). These small changes in expression may be difficult to distinguish, especially in the context of SCI, which could make it harder to detect a significant difference in TNF-α gene expression between the groups.
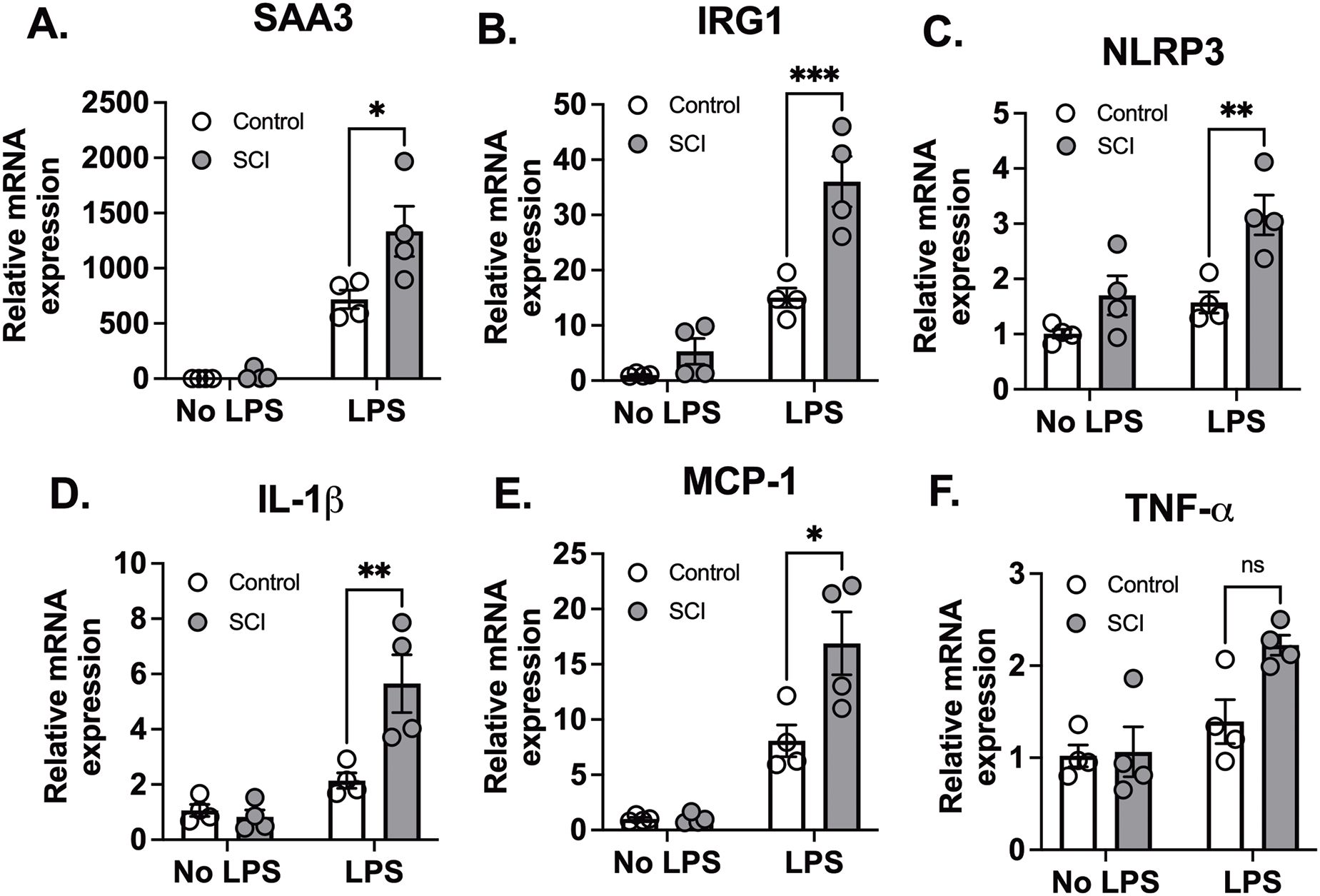
Figure 1. T9 SCI exaggerated lung inflammation after LPS exposure. T9 SCI exaggerated lung inflammation after LPS exposure. C57BL/6N mice underwent T9 SCI surgery. Ten days after SCI, mice were exposed to LPS (10mg/10mL saline) inhalation. Lung tissues were harvested at 24 hr after LPS exposure for gene expression of (A) SAA3, (B) IRG1, (C) NLRP3, (D) IL-1β, (E) MCP-1, and (F) TNF-α in the lung. (n=4 from each group). Data were expressed as mean ± SEM. *P<0.05, **P<0.01, ***P<0.001 among groups ;ns: not significant.
Consistently, SCI alone did not elicit cytokine release when compared to control mice. LPS exposure also led to a markedly greater increase in IL-1β, IL-6, and MCP-1 release in BAL fluid in SCI mice compared to control mice (Figures 2A–D). These findings suggest that SCI alone did not provoke prolonged inflammation in the local lung tissue. Instead, SCI amplifies lung inflammation following exposure to LPS.
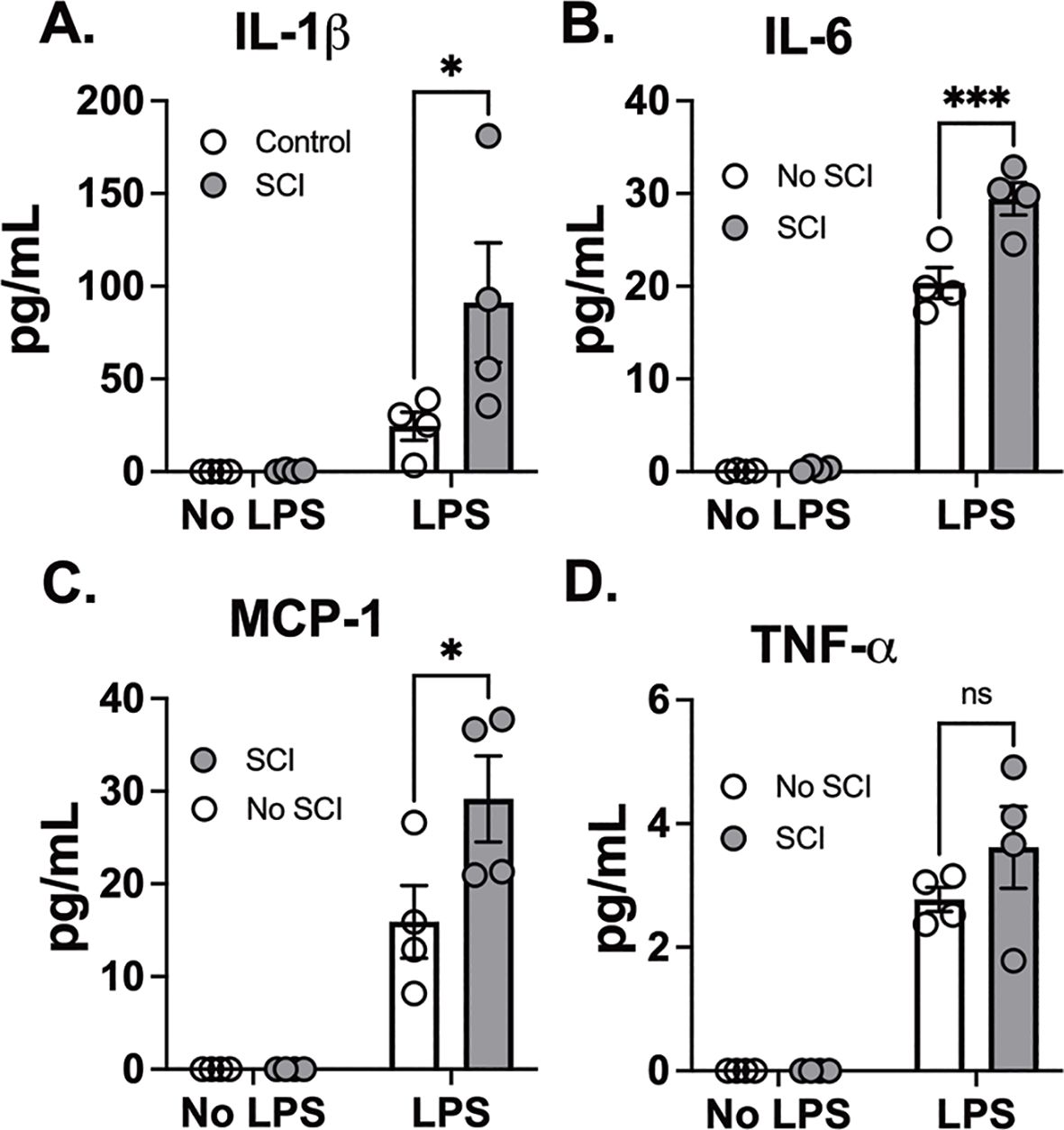
Figure 2. T9 SCI enhanced cytokine production after LPS exposure. C57BL/6N mice underwent T9 SCI surgery. Ten days after SCI, mice were exposed to LPS (10mg/10mL saline) inhalation. Bronchoalveolar lavage (BAL) fluids were harvested at 24 hr after LPS exposure for protein concentration in BAL fluid. Gene expression of (A) IL-1β, (B) IL-6, (C) MCP-1, and (D) TNF-α in the lung. (n=4 from each group). Data were expressed as mean ± SEM. *P<0.05, ***P<0.001 among groups ;ns: not significant.
Inflammatory processes triggered by injury release various mediators such as cytokines, chemokines, and reactive oxygen species. These signaling molecules possess the capacity to influence the structural integrity of both the endothelial and epithelial barriers, thereby increasing lung permeability. Consequently, this phenomenon results in the effusion of fluids into the interstitial spaces and alveoli of the lung. Measuring lung permeability provides a quantitative measure of edema formation, which is a key pathological feature of acute lung injury (33, 34). Ten days post-spinal cord injury (SCI), there was a two-fold increase in protein concentration in the BAL fluid, suggesting increased barrier permeability, although the difference was not statistically significant. Following exposure to LPS, protein levels in the BAL fluid markedly increased in SCI mice compared to control mice (Figure 3A).
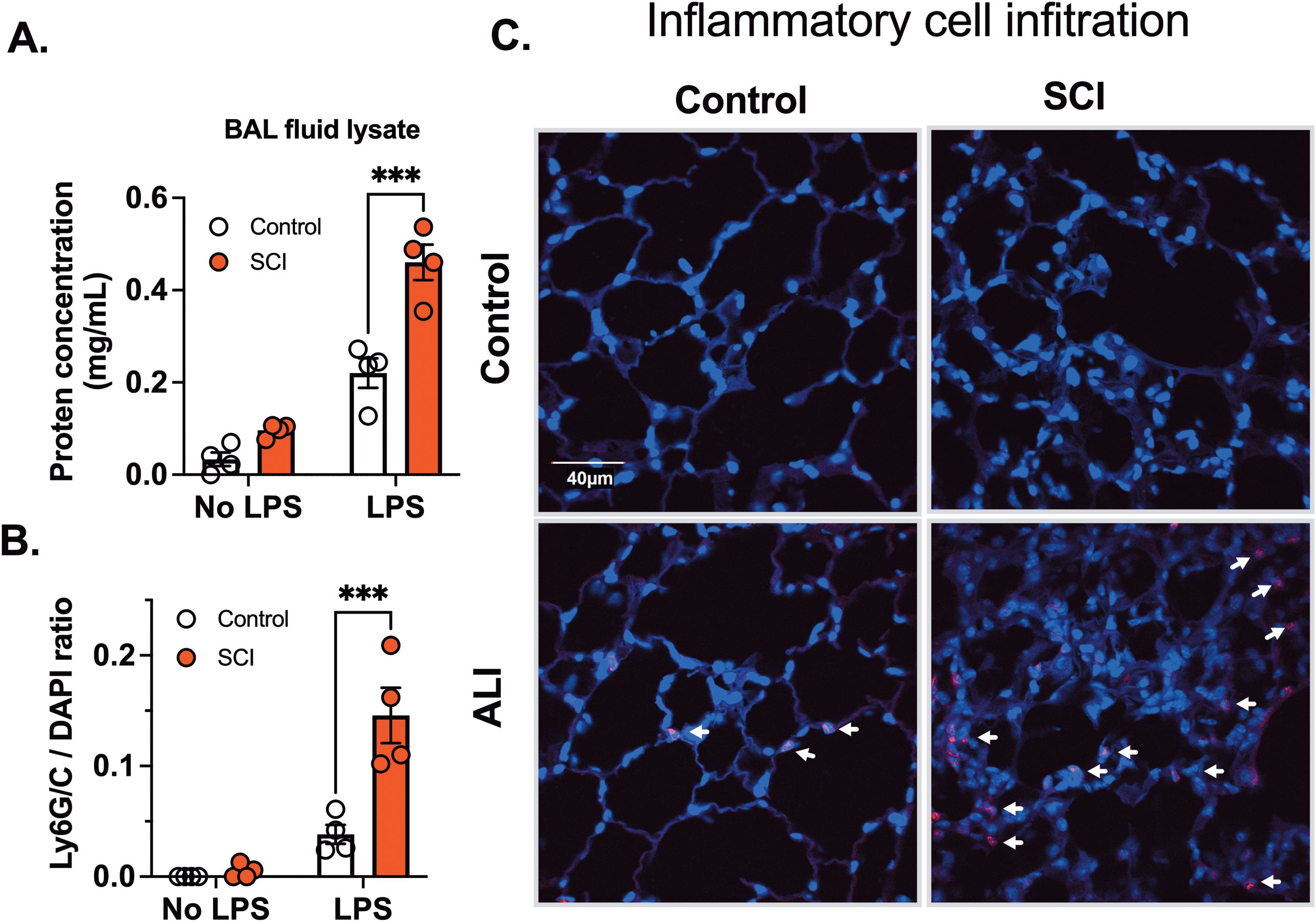
Figure 3. T9 SCI exaggerated inflammatory cell infiltration after LPS exposure. C57BL/6N mice underwent T9 SCI surgery. Ten days after SCI, mice were exposed to LPS (10mg/10mL saline) inhalation. BAL fluid and lung tissues were harvested at 24 hr after LPS exposure for (A) protein concentration in BAL fluid cell lysates. (B) Quantification results of Ly6G/C vs DAPI positive cells (C) Immunofluorescence of representative lung sections for Ly6G/C (Red) vs DAPI (Blue) (scale bar=40 µm). (n=4 from each group). Data were expressed as mean ± SEM. ***P<0.001 among groups.
Inflammatory cell activation and increased permeability form a positive feedback loop. Infiltrated cells release many mediators, that alter permeability, perpetuating inflammation and damaging endothelial barriers, correlating with the severity of lung injury. Inflammatory cell infiltration serves as a diagnostic marker for acute lung injury. Next, we investigated inflammatory cell infiltration using Ly6G/C staining, which marks neutrophils (Ly6G) and macrophages (Ly6C). No infiltration of Ly6G or Ly6C positive cells was observed in SCI mice following saline exposure. However, LPS inhalation led to a significant increase in Ly6G/C positive cells in lung tissue, indicating infiltration of neutrophils and macrophages. In accordance with cytokine findings, there was a significant elevation in Ly6G/C positive cells in lung tissue from SCI mice compared to control mice (Figures 3B, C, arrows point to red cells). These results demonstrate that SCI enhances inflammatory cell infiltration and cytokine secretion in the lung after LPS exposure.
To assess systemic inflammation, we measured 10 different cytokines in plasma 24 hours after exposure to saline or LPS. We observed a significant main effect of SCI on MCP-1 levels (Figure 4A). However, SCI did not further increase MCP-1 levels following LPS exposure compared to LPS alone (Figure 4A). Similarly, there were no significant differences between LPS and SCI+LPS for IL-6 and TNF-α levels (Figures 4B, C). GM-CSF, IFNγ, IL-1β, IL-2, IL-4, IL-10, and IL-12 were undetectable in all plasma samples. These findings align with our lung permeability data, where SCI also showed a main effect on protein concentration (Figure 3A). Since LPS inhalation directly induces lung inflammation, SCI had minimal impact on the systemic response following LPS exposure.
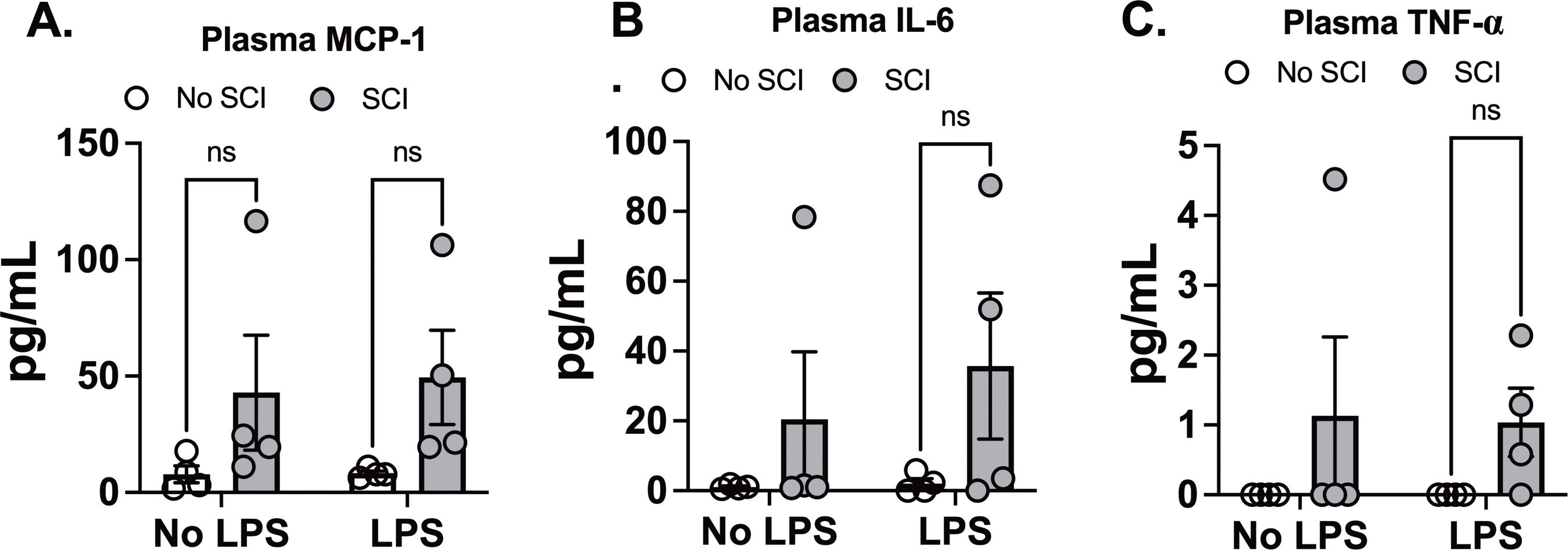
Figure 4. Effect of SCI and LPS exposure on plasma cytokine Levels. C57BL/6N mice underwent T9 SCI surgery. Ten days after SCI, mice were exposed to LPS (10mg/10mL saline) inhalation. Plasma samples were harvested at 24 hr after LPS exposure for cytokine assays. (A) MCP-1, (B) IL-6, and (C) TNF-α. (n=4 from each group). Data were expressed as mean ± SEM. ns: not significant.
Several studies have indicated that a high-level SCI, as opposed to a low-level SCI, leads to splenic atrophy and immune function suppression in mice, primarily due to exaggerated sympathetic reflexes (35–37). To explore whether T9 SCI reduced spleen weights 10 days post-injury, we measured spleen size 24 hours after exposure to saline and LPS. However, we did not observe any significant differences in spleen weight or length between control and SCI mice (Figures 5A–C). Furthermore, LPS exposure did not alter spleen weight or length compared to the saline groups (Figures 5A–C). These findings suggest that T9 SCI alone is insufficient to induce splenic atrophy even in combination with acute lung injury.
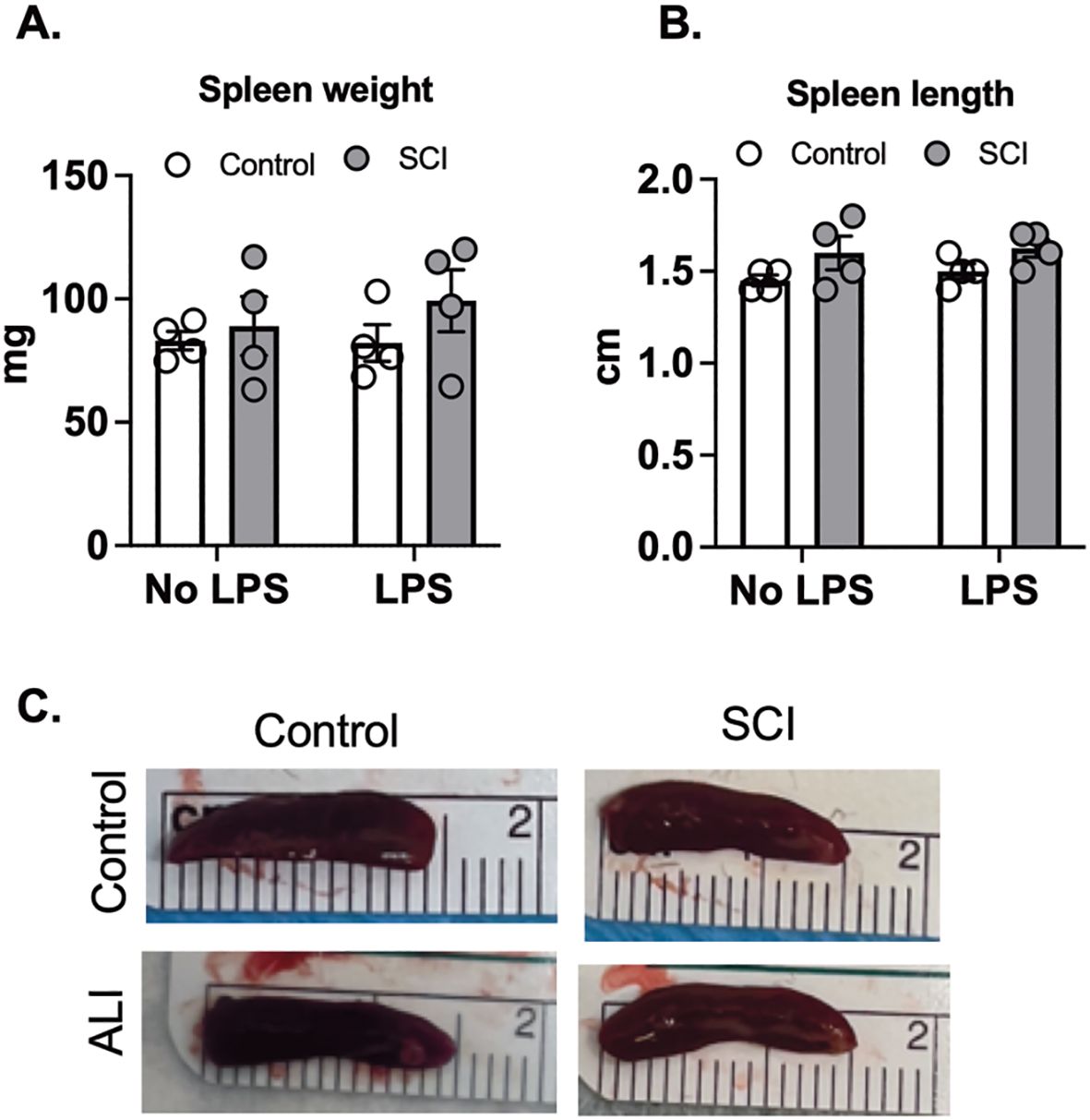
Figure 5. T9 SCI had no effect on spleen size after LPS exposure. C57BL/6N mice underwent T9 SCI surgery. Ten days after SCI, mice were exposed to LPS (10mg/10mL saline) inhalation. Spleens were harvested at 24 hr after LPS exposure. (A) Spleen weight (B) Spleen length was measured. (C) Representative spleen images for length (cm). (n=4 from each group). Data were expressed as mean ± SEM.
While previous studies emphasize mature leukocyte dysfunction as a hallmark of SCI-induced immune impairment, emerging evidence highlights the impact of SCI on the development and mobilization of immune cell precursors within the bone marrow. We collected bone marrow cells from mice 10 days post-SCI and from control mice, subsequently differentiating them into macrophages. In assessing the influence of SCI on these bone marrow-derived macrophages (BMDMs), we examined the expression of both glycolytic and inflammatory marker genes. Our findings indicate that BMDMs from SCI mice exhibit increased glycolytic activity and heightened inflammatory conditions (Figure 6A). After subjecting these macrophages to LPS for 3 hours, BMDMs from SCI mice demonstrated a significantly increased response to LPS, as measured by mRNA levels of NLRP3 and IRG1 (Figures 6B, C). These data show that SCI may alter the maturation of bone marrow derived macrophage precursors thereby contributing to a state of hyper-responsiveness in the lung.
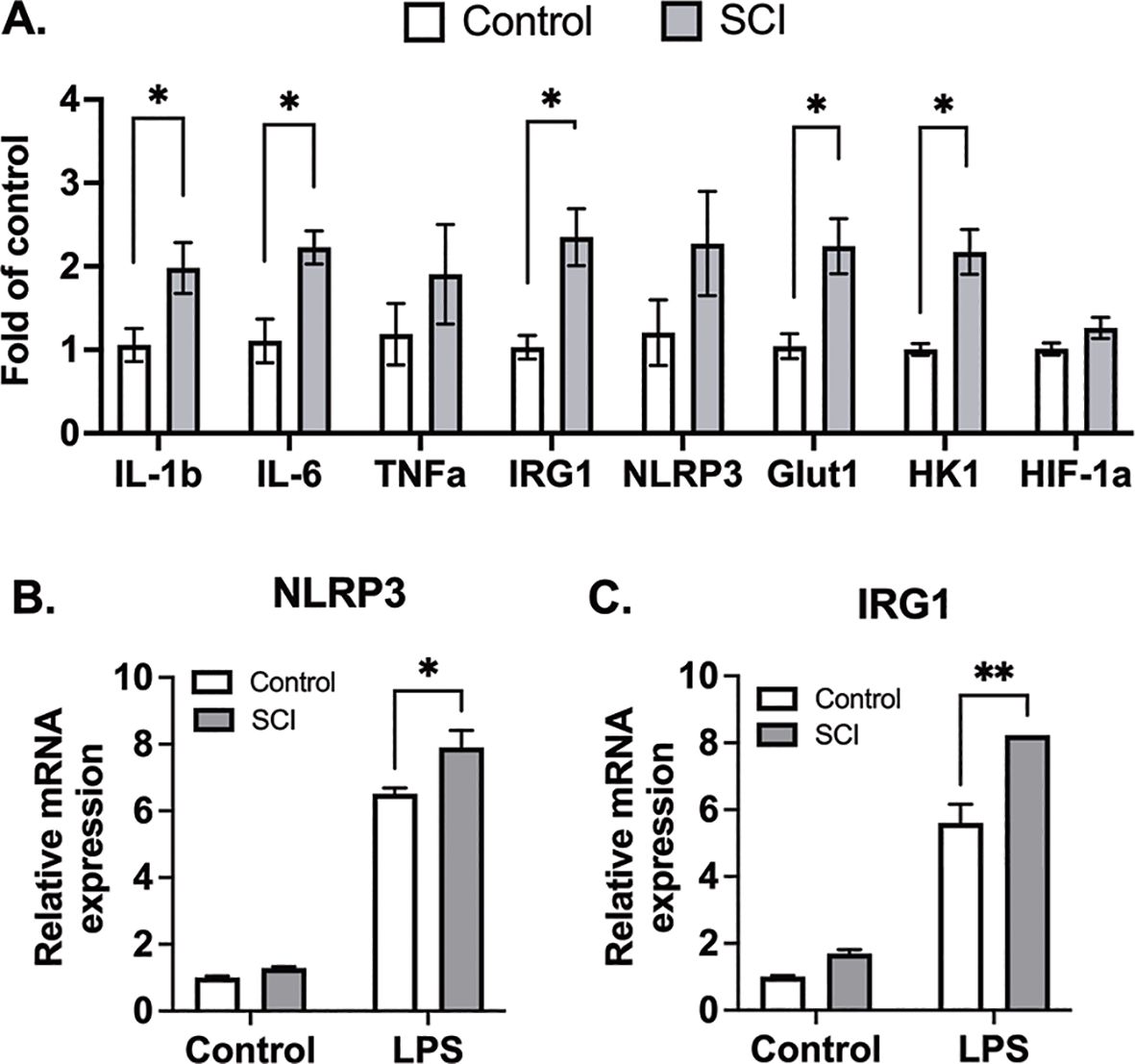
Figure 6. BMDMs from SCI mice are more glycolytic and responsive to inflammatory stimuli. C57BL/6N mice underwent T9 SCI surgery. Ten days after SCI, BMDMs were harvested and differentiated from control and SCI mice. (A) Basal gene expression of BMDMs. (B) NLRP3 and (C) IRG1 gene expression after LPS stimulation for 3 hr (n=4 experiments). Data were expressed as mean ± SEM. *P<0.05, **P<0.01 among groups.
Discussion
In this study, our aim was to elucidate the impact of SCI, specifically at the T9 level, on pulmonary function and immune responses, particularly in the context of lung inflammation induced by aerosolized LPS. Our results indicate that ten days post-SCI recovery, mice exposed to LPS exhibited a marked increase in proinflammatory cytokine gene expression in the lung tissues compared to control mice. We observed a significant increase in cytokine release in BAL fluid from SCI mice compared to control mice following LPS exposure. Additionally, there was a significant increase, both at baseline and in response to LPS, in bone marrow-derived macrophages harvested from SCI mice, indicating that the acute increase in systemic inflammation is maintained in these macrophages even after the systemic inflammation throughout the body resolves. These findings collectively indicate that SCI amplifies lung inflammation in response to LPS, emphasizing the role of SCI in exacerbating inflammatory cascades associated with lung inflammation, pneumonia, and acute respiratory distress syndrome.
The LPS exposure mouse model has been used to study mechanisms of human lung diseases and for the discovery of new drug targets for ALI (38, 39). The LPS inhalation model offers a more natural and physiologically relevant route of entry for LPS into the respiratory tract. Unlike intratracheal or intranasal delivery, which can administer a bolus of an excessive dose of LPS within a few seconds, inhalation allows for a gradual and more controlled dispersion of LPS into the lungs. This better mimics real-world exposure scenarios where pathogens, such as bacteria or their components, enter the lungs via the airways over time. A sudden bolus of LPS, as seen with intratracheal delivery, can overwhelm the lung’s natural defense systems, leading to a less accurate model of pulmonary inflammation and immune response (40). In contrast, inhalation ensures that LPS is more evenly distributed throughout the lung tissue, including both the upper and lower regions of the respiratory tract. This is particularly important in mimicking the way infections or inflammatory responses occur in human patients, where a pathogen often affects multiple regions of the lung, not just a localized area (40). Furthermore, using the LPS inhalation model in our SCI-ALI mouse model enables us to more accurately replicate the complex dynamics of lung injury and immune response following spinal cord injury. This approach is essential for examining the interaction between SCI and pulmonary dysfunction, providing a unique and valuable preclinical model to explore therapeutic strategies for lung inflammation and injury in the context of SCI.
Furthermore, a significant elevation in protein concentration in BAL fluid from SCI mice following LPS exposure compared to control mice, suggests increased lung permeability. A positive feedback loop involving inflammatory cell activation and increased permeability was evident, with infiltrated cells releasing mediators that perpetuated inflammation and damaged barriers. This phenomenon correlated with the severity of lung injury, as demonstrated by increased inflammatory cell infiltration in SCI mice, marked by Ly6G/C staining. It is important to mention that within the first ten days after spinal cord injury (SCI), a slight rise in protein concentration in the BAL fluid was observed. This highlights the significant finding that there are acute changes in vascular damage and alterations in the lung microenvironment following acute SCI inflammation.
A recent study found that patients who developed infections after SCI exhibited altered immune cell populations and cytokine levels, including increased neutrophils and eosinophils, and decreased lymphocytes and eotaxin-1 levels (41). This immune imbalance was associated with a higher susceptibility to infections during the acute and subacute phases of SCI, which can impact both neurological recovery and overall quality of life. Our findings on MCP-1 align with these observations, as MCP-1 is a key chemokine involved in immune cell recruitment and has been implicated in inflammation and infection susceptibility. In our study, SCI significantly affected MCP-1 levels, which is consistent with the systemic immune dysregulation observed in SCI patients, particularly in relation to infection risk. This connection further reinforces the relevance of our findings and supports the broader clinical significance of our work.
Interestingly, despite previous studies indicating splenic atrophy and immune function suppression in high-level SCI, our investigation into T9 SCI did not reveal significant differences in spleen weight or length between control and SCI mice, either with or without LPS exposure. Previous studies show that LPS treatment triggers splenomegaly and the accumulation of immune cells in the spleen four days later (42). In our experiment, spleens were harvested 24 hours after acute lung injury (ALI). We selected this time point based on our prior discovery that lung permeability, inflammation, and immune cell infiltration reach their peak 24 hours after LPS exposure (23). A recent publication by Brennan et al. highlighted that T3 SCI induces splenic atrophy at 28 days post-injury, an effect that was prevented by microglia depletion (43). The study also revealed significant changes in the splenic B cell and T cell zones following SCI (43). Future studies analyzing Mac2 (macrophage), IBA1 (Microglia), B220 (B cell), and CD3 (T cell) markers in the spleen following T9 vs. T3 SCI injury will provide important insights into the level-dependent immune response after SCI. Despite this, our findings indicate that T9 SCI alone is inadequate to prompt alterations in spleen size even during the acute phase of acute lung injury, ten days post-injury.
Moving beyond the lungs, our study examined the impact of T9 SCI on immune cell precursors within the bone marrow. Analysis of bone marrow-derived macrophages (BMDMs) revealed heightened glycolytic activity and increased inflammatory conditions in BMDMs obtained from SCI mice. Similar outcomes were observed in the context of high-level C3 SCI, indicating hyperinflammatory responses in bone marrow cells by day 3 post-injury (44). In humans, SCI has been shown to stimulate apoptosis and decrease proliferation of bone marrow cells (45, 46). These cumulative findings suggest that SCI may directly affect immune function at the origin of all immune cells, specifically within the bone marrow cells. Expanding the focus beyond nerve injury, prior research has established associations between conditions such as diabetes, a western diet, as well as viral and bacterial infections in mice with elevated baseline inflammation in bone marrow cells (47–49). This implies that sterile or non-sterile inflammation may exert a direct influence on regulating the bone marrow microenvironment. Subsequent research endeavors are crucial to unravel the mechanisms contributing to SCI-induced bone marrow hyperinflammation and determine whether such mechanisms are responsible for acute or chronic immune dysfunction.
In conclusion, T9 SCI causes a significant increase in inflammation stimulated by LPS inhalation 10 days after SCI when there is little systemic inflammation. Our data indicates that this heightened inflammatory memory is likely attributable to the impact on bone marrow cells, particularly those undergoing differentiation into macrophages. Various SCI injury levels distinctly affect pulmonary function, immune responses, and the bone marrow microenvironment. The observed rise in lung inflammation and enhanced reactivity of immune cell precursors post-SCI have significant implications for understanding systemic effects on immune function. These findings pave the way for future investigations and the development of SCI subtype-specific therapeutic interventions in this context.
Data availability statement
The original contributions presented in the study are included in the article/Supplementary Material. Further inquiries can be directed to the corresponding author.
Ethics statement
The animal studies were approved by University of Rochester Medical Center (University Committee on Animal Resources). The studies were conducted in accordance with the local legislation and institutional requirements. Written informed consent was obtained from the owners for the participation of their animals in this study.
Author contributions
BB: Conceptualization, Funding acquisition, Resources, Supervision, Writing – review & editing. AP: Investigation, Methodology, Writing – review & editing. VV: Investigation, Methodology, Writing – review & editing. CP: Investigation, Methodology, Writing – review & editing. CH: Conceptualization, Data curation, Formal analysis, Funding acquisition, Investigation, Methodology, Project administration, Resources, Software, Supervision, Validation, Visualization, Writing – original draft, Writing – review & editing.
Funding
The author(s) declare financial support was received for the research, authorship, and/or publication of this article. This research received financial support from the New York State Department of Health (C34726GG and C39071GG awarded to BB and CH), pilot grants from the University of Rochester of Department of Medicine (awarded to BB), and the Department of Environmental Health Sciences (P30 ES001247 awarded to BB and CH), and Trauma Research and Combat Casualty Care Collaborative (175153 awarded to CH) as well as The University of Texas at San Antonio Startup Funding and The College for Health, Community, and Policy (HCAP) Seed Grant (awarded to CH).
Conflict of interest
The authors declare that the research was conducted in the absence of any commercial or financial relationships that could be construed as a potential conflict of interest.
Publisher’s note
All claims expressed in this article are solely those of the authors and do not necessarily represent those of their affiliated organizations, or those of the publisher, the editors and the reviewers. Any product that may be evaluated in this article, or claim that may be made by its manufacturer, is not guaranteed or endorsed by the publisher.
Supplementary material
The Supplementary Material for this article can be found online at: https://www.frontiersin.org/articles/10.3389/fimmu.2024.1483402/full#supplementary-material
References
1. Veeravagu A, Jiang B, Rincon F, Maltenfort M, Jallo J, Ratliff JK. Acute respiratory distress syndrome and acute lung injury in patients with vertebral column fracture(s) and spinal cord injury: a nationwide inpatient sample study. Spinal Cord. (2013) 51:461–5. doi: 10.1038/sc.2013.16
2. Reines HD, Harris RC. Pulmonary complications of acute spinal cord injuries. Neurosurgery. (1987) 21:193–6. doi: 10.1227/00006123-198708000-00010
3. Soden RJ, Walsh J, Middleton JW, Craven ML, Rutkowski SB, Yeo JD. Causes of death after spinal cord injury. Spinal Cord. (2000) 38:604–10. doi: 10.1038/sj.sc.3101080
4. Pruss H, Tedeschi A, Thiriot A, Lynch L, Loughhead SM, Stutte S, et al. Spinal cord injury-induced immunodeficiency is mediated by a sympathetic-neuroendocrine adrenal reflex. Nat Neurosci. (2017) 20:1549–59. doi: 10.1038/nn.4643
5. Mironets E, Osei-Owusu P, Bracchi-Ricard V, Fischer R, Owens EA, Ricard J, et al. Soluble TNFalpha Signaling within the Spinal Cord Contributes to the Development of Autonomic Dysreflexia and Ensuing Vascular and Immune Dysfunction after Spinal Cord Injury. J Neurosci. (2018) 38:4146–62. doi: 10.1523/JNEUROSCI.2376-17.2018
6. Adegeest CY, van Gent JAN, Stolwijk-Swuste JM, Post MWM, Vandertop WP, Oner FC, et al. Influence of severity and level of injury on the occurrence of complications during the subacute and chronic stage of traumatic spinal cord injury: a systematic review. J Neurosurg Spine. (2022) 36:632–52. doi: 10.3171/2021.7.SPINE21537
7. Weaver LC, Marsh DR, Gris D, Brown A, Dekaban GA. Autonomic dysreflexia after spinal cord injury: central mechanisms and strategies for prevention. Prog Brain Res. (2006) 152:245–63. doi: 10.1016/S0079-6123(05)52016-8
8. Curt A, Nitsche B, Rodic B, Schurch B, Dietz V. Assessment of autonomic dysreflexia in patients with spinal cord injury. J Neurol Neurosurg Psychiatry. (1997) 62:473–7. doi: 10.1136/jnnp.62.5.473
9. Phillips AA, Krassioukov AV. Contemporary cardiovascular concerns after spinal cord injury: mechanisms, maladaptations, and management. J Neurotrauma. (2015) 32:1927–42. doi: 10.1089/neu.2015.3903
10. Squair JW, Tigchelaar S, Moon KM, Liu J, Tetzlaff W, Kwon BK, et al. Integrated systems analysis reveals conserved gene networks underlying response to spinal cord injury. Elife. (2018) 7:1–23. doi: 10.7554/eLife.39188
11. Brommer B, Engel O, Kopp MA, Watzlawick R, Muller S, Pruss H, et al. Spinal cord injury-induced immune deficiency syndrome enhances infection susceptibility dependent on lesion level. Brain. (2016) 139:692–707. doi: 10.1093/brain/awv375
12. Krassioukov A, Claydon VE. The clinical problems in cardiovascular control following spinal cord injury: an overview. Prog Brain Res. (2006) 152:223–9. doi: 10.1016/S0079-6123(05)52014-4
13. Failli V, Kopp MA, Gericke C, Martus P, Klingbeil S, Brommer B, et al. Functional neurological recovery after spinal cord injury is impaired in patients with infections. Brain. (2012) 135:3238–50. doi: 10.1093/brain/aws267
14. Oyinbo CA. Secondary injury mechanisms in traumatic spinal cord injury: a nugget of this multiply cascade. Acta Neurobiol Exp (Wars). (2011) 71:281–99. doi: 10.55782/ane-2011-1848
15. Gal P, Kravcukova P, Mokry M, Kluchova D. Chemokines as possible targets in modulation of the secondary damage after acute spinal cord injury: a review. Cell Mol Neurobiol. (2009) 29:1025–35. doi: 10.1007/s10571-009-9392-4
16. Jiang W, Li M, He F, Yao W, Bian Z, Wang X, et al. Protective effects of asiatic acid against spinal cord injury-induced acute lung injury in rats. Inflammation. (2016) 39:1853–61. doi: 10.1007/s10753-016-0414-3
17. Mifflin KA, Brennan FH, Guan Z, Kigerl KA, Filous AR, Mo X, et al. Spinal cord injury impairs lung immunity in mice. J Immunol. (2022) 209:157–70. doi: 10.4049/jimmunol.2200192
18. Parvin S, Williams CR, Jarrett SA, Garraway SM. Spinal cord injury increases pro-inflammatory cytokine expression in kidney at acute and sub-chronic stages. Inflammation. (2021) 44:2346–61. doi: 10.1007/s10753-021-01507-x
19. Norden DM, Bethea JR, Jiang J. Impaired CD8 T cell antiviral immunity following acute spinal cord injury. J Neuroinflamm. (2018) 15:149. doi: 10.1186/s12974-018-1191-8
20. Sun X, Jones ZB, Chen XM, Zhou L, So KF, Ren Y. Multiple organ dysfunction and systemic inflammation after spinal cord injury: a complex relationship. J Neuroinflamm. (2016) 13:260. doi: 10.1186/s12974-016-0736-y
21. Osterthun R, Post MW, van Asbeck FW, van Leeuwen CM, van Koppenhagen CF. Causes of death following spinal cord injury during inpatient rehabilitation and the first five years after discharge. A Dutch cohort study Spinal Cord. (2014) 52:483–8. doi: 10.1038/sc.2014.28
22. Mortezaee K, Khanlarkhani N, Beyer C, Zendedel A. Inflammasome: Its role in traumatic brain and spinal cord injury. J Cell Physiol. (2018) 233:5160–9. doi: 10.1002/jcp.v233.7
23. Hsu CG, Fazal F, Rahman A, Berk BC, Yan C. Phosphodiesterase 10A is a key mediator of lung inflammation. J Immunol. (2021) 206:3010–20. doi: 10.4049/jimmunol.2001026
24. Ying W, Cheruku PS, Bazer FW, Safe SH, Zhou B. Investigation of macrophage polarization using bone marrow derived macrophages. J Vis Exp. (2013) 76:1–8. doi: 10.3791/50323
25. Elahi A, Emerson J, Rudlong J, Keillor JW, Salois G, Visca A, et al. Deletion or inhibition of astrocytic transglutaminase 2 promotes functional recovery after spinal cord injury. Cells. (2021) 10:1–15. doi: 10.3390/cells10112942
26. Ye RD, Sun L. Emerging functions of serum amyloid A in inflammation. J Leukoc Biol. (2015) 98:923–9. doi: 10.1189/jlb.3VMR0315-080R
27. Lang R, Siddique M. Control of immune cell signaling by the immuno-metabolite itaconate. Front Immunol. (2024) 15:1352165. doi: 10.3389/fimmu.2024.1352165
28. Barnett KC, Li S, Liang K, Ting JP. A 360 degrees view of the inflammasome: Mechanisms of activation, cell death, and diseases. Cell. (2023) 186:2288–312. doi: 10.1016/j.cell.2023.04.025
29. Hsu CG, Chavez CL, Zhang C, Sowden M, Yan C, Berk BC. The lipid peroxidation product 4-hydroxynonenal inhibits NLRP3 inflammasome activation and macrophage pyroptosis. Cell Death Differ. (2022) 29:1790–803. doi: 10.1038/s41418-022-00966-5
30. Hsu CG, Li W, Sowden M, Chavez CL, Berk BC. Pnpt1 mediates NLRP3 inflammasome activation by MAVS and metabolic reprogramming in macrophages. Cell Mol Immunol. (2023) 20:131–42. doi: 10.1038/s41423-022-00962-2
31. Gschwandtner M, Derler R, Midwood KS. More than just attractive: how CCL2 influences myeloid cell behavior beyond chemotaxis. Front Immunol. (2019) 10:2759. doi: 10.3389/fimmu.2019.02759
32. Lund MC, Clausen BH, Brambilla R, Lambertsen KL. The role of tumor necrosis factor following spinal cord injury: A systematic review. Cell Mol Neurobiol. (2023) 43:925–50. doi: 10.1007/s10571-022-01229-0
33. Sartori C, Matthay MA. Alveolar epithelial fluid transport in acute lung injury: new insights. Eur Respir J. (2002) 20:1299–313. doi: 10.1183/09031936.02.00401602
34. Chan MC, Kuok DI, Leung CY, Hui KP, Valkenburg SA, Lau EH, et al. Human mesenchymal stromal cells reduce influenza A H5N1-associated acute lung injury in vitro and in vivo. Proc Natl Acad Sci U S A. (2016) 113:3621–6. doi: 10.1073/pnas.1601911113
35. Zhang Y, Guan Z, Reader B, Shawler T, Mandrekar-Colucci S, Huang K, et al. Autonomic dysreflexia causes chronic immune suppression after spinal cord injury. J Neurosci. (2013) 33:12970–81. doi: 10.1523/JNEUROSCI.1974-13.2013
36. Lucin KM, Sanders VM, Popovich PG. Stress hormones collaborate to induce lymphocyte apoptosis after high level spinal cord injury. J Neurochem. (2009) 110:1409–21. doi: 10.1111/j.1471-4159.2009.06232.x
37. Lucin KM, Sanders VM, Jones TB, Malarkey WB, Popovich PG. Impaired antibody synthesis after spinal cord injury is level dependent and is due to sympathetic nervous system dysregulation. Exp Neurol. (2007) 207:75–84. doi: 10.1016/j.expneurol.2007.05.019
38. Matute-Bello G, Frevert CW, Martin TR. Animal models of acute lung injury. Am J Physiol Lung Cell Mol Physiol. (2008) 295:L379–399. doi: 10.1152/ajplung.00010.2008
39. Slavin SA, Leonard A, Grose V, Fazal F, Rahman A. Autophagy inhibitor 3-methyladenine protects against endothelial cell barrier dysfunction in acute lung injury. Am J Physiol Lung Cell Mol Physiol. (2018) 314:L388–96. doi: 10.1152/ajplung.00555.2016
40. Liu F, Li W, Pauluhn J, Trubel H, Wang C. Lipopolysaccharide-induced acute lung injury in rats: comparative assessment of intratracheal instillation and aerosol inhalation. Toxicology. (2013) 304:158–66. doi: 10.1016/j.tox.2012.12.020
41. Grassner L, Klein B, Garcia-Ovejero D, Mach O, Scheiblhofer S, Weiss R, et al. Systemic immune profile predicts the development of infections in patients with spinal cord injuries. J Neurotrauma. (2022) 39:1678–86. doi: 10.1089/neu.2021.0448
42. Liverani E, Rico MC, Yaratha L, Tsygankov AY, Kilpatrick LE, Kunapuli SP. LPS-induced systemic inflammation is more severe in P2Y12 null mice. J Leukoc Biol. (2014) 95:313–23. doi: 10.1189/jlb.1012518
43. Brennan FH, Swarts EA, Kigerl KA, Mifflin KA, Guan Z, Noble BT, et al. Microglia promote maladaptive plasticity in autonomic circuitry after spinal cord injury in mice. Sci Transl Med. (2024) 16:eadi3259. doi: 10.1126/scitranslmed.adi3259
44. Carpenter RS, Marbourg JM, Brennan FH, Mifflin KA, Hall JCE, Jiang RR, et al. Spinal cord injury causes chronic bone marrow failure. Nat Commun. (2020) 11:3702. doi: 10.1038/s41467-020-17564-z
45. Chernykh ER, Shevela EY, Leplina OY, Tikhonova MA, Ostanin AA, Kulagin AD, et al. Characteristics of bone marrow cells under conditions of impaired innervation in patients with spinal trauma. Bull Exp Biol Med. (2006) 141:117–20. doi: 10.1007/s10517-006-0109-0
46. Iversen PO, Hjeltnes N, Holm B, Flatebo T, Strom-Gundersen I, Ronning W, et al. Depressed immunity and impaired proliferation of hematopoietic progenitor cells in patients with complete spinal cord injury. Blood. (2000) 96:2081–3. doi: 10.1182/blood.V96.6.2081
47. Edgar L, Akbar N, Braithwaite AT, Krausgruber T, Gallart-Ayala H, Bailey J, et al. Hyperglycemia induces trained immunity in macrophages and their precursors and promotes atherosclerosis. Circulation. (2021) 144:961–82. doi: 10.1161/CIRCULATIONAHA.120.046464
48. Christ A, Gunther P, Lauterbach MAR, Duewell P, Biswas D, Pelka K, et al. Western diet triggers NLRP3-Dependent innate immune reprogramming. Cell. (2018) 172:162–75.e114. doi: 10.1016/j.cell.2017.12.013
Keywords: spinal cord injury, inflammation, acute lung injury, macrophage, pulmonary dysfunction
Citation: Berk BC, Pereira A, Vizcarra VS, Pröschel C and Hsu CG (2025) Spinal cord injury enhances lung inflammation and exacerbates immune response following exposure to LPS. Front. Immunol. 15:1483402. doi: 10.3389/fimmu.2024.1483402
Received: 30 August 2024; Accepted: 26 December 2024;
Published: 15 January 2025.
Edited by:
Lynn Xiaoling Qiang, Northwell Health, United StatesReviewed by:
Dhananajay Kumar, Louisiana State University Health Shreveport, United StatesAngélica Coyoy-Salgado, Mexican Social Security Institute, Mexico
Copyright © 2025 Berk, Pereira, Vizcarra, Pröschel and Hsu. This is an open-access article distributed under the terms of the Creative Commons Attribution License (CC BY). The use, distribution or reproduction in other forums is permitted, provided the original author(s) and the copyright owner(s) are credited and that the original publication in this journal is cited, in accordance with accepted academic practice. No use, distribution or reproduction is permitted which does not comply with these terms.
*Correspondence: Chia George Hsu, Q2hpYS5Ic3VAdXRzYS5lZHU=; Bradford C. Berk, QmVya0B1cm1jLnJvY2hlc3Rlci5lZHU=
†ORCID: Bradford C. Berk, orcid.org/0000-0002-2767-4115
Amanda Pereira, orcid.org/0000-0002-2616-966X
Velia Sofia Vizcarra, orcid.org/0000-0002-2349-7502
Christoph Pröschel, orcid.org/0000-0002-4473-2225
Chia George Hsu, orcid.org/0000-0002-1133-4116