- 1Department of Biomedical Sciences, James H Quillen College of Medicine, East Tennessee State University, Johnson City, TN, United States
- 2Department of Surgery, James H. Quillen College of Medicine, East Tennessee State University, Johnson City, TN, United States
- 3James H. Quillen College of Medicine, East Tennessee State University, Johnson City, TN, United States
- 4Program in Neuroscience, College of Arts and Science, Vanderbilt University, Nashville, TN, United States
- 5Center of Excellence in Inflammation, Infectious Disease and Immunity, James H. Quillen College of Medicine, East Tennessee State University, Johnson City, TN, United States
Lactate significantly impacts immune cell function in sepsis and septic shock, transcending its traditional view as just a metabolic byproduct. This review summarizes the role of lactate as a biomarker and its influence on immune cell dynamics, emphasizing its critical role in modulating immune responses during sepsis. Mechanistically, key lactate transporters like MCT1, MCT4, and the receptor GPR81 are crucial in mediating these effects. HIF-1α also plays a significant role in lactate-driven immune modulation. Additionally, lactate affects immune cell function through post-translational modifications such as lactylation, acetylation, and phosphorylation, which alter enzyme activities and protein functions. These interactions between lactate and immune cells are central to understanding sepsis-associated immune dysregulation, offering insights that can guide future research and improve therapeutic strategies to enhance patient outcomes.
1 Introduction
Elevation in lactate levels is observed in a variety of critical illnesses, making lactate a useful biomarker for illness severity and prognosis (1, 2). In 1964, the use of lactate as a prognostic tool was first proposed by Weil et al. based on their pioneering observation that high lactate levels (> 4 mmol/L) correlated significantly with adverse outcomes in patients with shock (3). Since then, substantial clinical studies have been performed to establish the association between lactate levels and severity of sepsis (4, 5). Notably, the Third International Consensus Definitions for Sepsis and Septic Shock (Sepsis-3) has defined serum lactate level exceeding 2 mmol/L is as a clinical criterion in identifying patients with sepsis and septic shock (6). This consensus document has been considered as a milestone for inclusion of lactate in clinical guidelines and highlights lactate’s role in the pathogenesis of sepsis/septic shock.
Lactate has historically been known as a byproduct of glucose metabolism (7). Recent evidence has shown that lactate is an essential signaling molecule and epigenetic modulator, which plays a crucial role in the biological and pathological functions of different cells (8). The glycolytic pathway is central to this process, converting glucose to pyruvate within the cytosol of cells. Depending on the conditions, pyruvate either fuels the tricarboxylic acid (TCA) cycle for energy via oxidative phosphorylation (OXPHOS) or is converted to lactate by the enzyme lactate dehydrogenase A (LDHA) in the cytosol (9–11). With enough oxygen, pyruvate enters the TCA cycle, leading to the production of carbon dioxide and high-energy carriers for adenosine triphosphate (ATP) synthesis (12). In anaerobic conditions, cells convert pyruvate into lactate using LDHA to maintain ATP production albeit less efficiently (11, 13). Traditionally, we attribute the increased lactate levels to tissue hypoperfusion in patients with sepsis/septic shock (14). However, as our knowledge of pathogenesis of sepsis advances, it has become clear that other processes, not directly related to tissue oxygenation, may increase lactate production in sepsis/septic shock, such as activation of immune cells, impaired lactate clearance due to multiple organ injuries, and mitochondrial defects (15–18).
It is now recognized that sepsis is associated with a profound immunosuppression, which is a predisposing risk factor of nosocomial infection and mortality (19). Previous studies have demonstrated that lactate is a potent immunosuppressant in tumor microenvironments, thereby favoring tumor cell growth (20). Similarly, emerging evidence shows that lactate directly modulates the functions of a variety of immune cell, which contributes to immune paralysis in sepsis (21, 22). Despite the difficulty in targeting lactate production due to its complexity, targeting lactate receptor G protein-couple receptor 81 (GPR81) and the lactate transporter (MCT1) is suggested to restore immune responses in in vitro studies (21, 23).
In this present review, we focus on the molecular mechanisms by which lactate regulates immune responses during sepsis. First, we summarize the clinical recognition of lactate’s role in sepsis/septic shock patients. We then discuss the molecular and cellular mechanisms by which lactate determines the fate and behavior of immune cells in sepsis/septic shock. Last, we highlight the therapeutic potential of targeting lactate metabolism and lactate-associated signaling in treating sepsis/septic shock.
2 Recognition of lactate in sepsis/septic shock
2.1 Early observations
As early as 1843, Johann Joseph Scherer, a German physician-chemist, observed the presence of lactic acid in seven case reports of young women who died peripartum (24). These patients were diagnosed with perimetritis and with secondary peritonitis, hemorrhagic shock, or cerebral hemorrhage (24). Scherer hypothesized that the production of lactic acid was enhanced in such severe diseases. Scherer’s pioneering case reports are considered to be the foundational documentation of lactic acid as an indicator of septic and hemorrhagic shock, thereby paving the way for future exploration of lactic acid’s diagnostic and prognostic potential in various conditions.
During the 1960s -1980s, high lactate levels in patients with circulatory failure and shock were routinely observed in clinical practice (3, 4, 25–27). It was found that blood lactate levels indicate the severity of shock and offer a crucial prognostic index, effectively predicting outcomes even before the onset of severe hypotension (25). Also, these studies suggested that lactic acid is a major contributor to the metabolic acidosis observed in early shock. Lactate from venous blood in the right atrium, superior vena cava, or pulmonary artery are nearly identical to arterial levels, as shown by high correlation in studies (28). Huckabee suggested that a more precise evaluation of oxygen debt is achieved by measuring “excess lactate,” which refers to an imbalanced rise in lactate levels relative to pyruvate (29, 30). However, Weil et al. concluded that lactate levels alone may be a simpler and more sensitive prognostic indicator of the severity of shock (4). The study on 56 shock patients revealed an 89% mortality rate with lactate levels of 4 mmol/L or higher (3). Moreover, during fluid resuscitation of 17 patients with noncardiogenic shock, it was observed that the lactate concentration of 9 patients decreased by more than 5% within the first 60 minutes (31), suggesting that serial lactate tests during shock proved more reliable for prognosis. Although these early studies were not precise in terms of severe sepsis/septic shock, they did hold true for many of the subsequent studies (32, 33).
2.2 Recognition in sepsis
In the late 1980s, Cohen and Woods suggested that elevated lactate levels could result from inadequate oxygen supply (type A hyperlactatemia) or from factors unrelated to tissue hypoxia (type B hyperlactatemia) (34). Although seemingly simple, this rigid classification can be challenging to apply in complex clinical scenarios, particularly in the hyperlactatemia associated with sepsis, where it is categorized by some as type A and by others as type B (35). Indeed, this classification belie the complexity and breadth of the detailed kinetics involved in the production and utilization of lactate by tissues (35). Peripheral shunting (36) and heightened adrenergic stimulation (37) can also cause hyperlactatemia, but their prevalence and clinical significance in sepsis patients remain unclear. Despite this, lactate is a valuable marker for assessing tissue hypoxia and disease severity, importantly, independent on blood pressure (38). Research has found that blood lactate levels are more effective than oxygen-related metrics in predicting septic shock outcomes (39), with sequential blood lactate measurements being able to anticipate subsequent multiple organ failure (17). At that time, the significance of blood lactate concentrations equal to or exceeding 4 mmol/L in the context of early goal-directed therapy was acknowledged by clinical researchers (40, 41).
2.3 Inclusion in clinical guidelines
Lactate has appeared as a marker of hypoperfusion in the definition of severe sepsis and septic shock proposed in 1992 (42). In the first edition of the Surviving Sepsis Campaign (SSC) guidelines, lactate emerged as a measure of severity and symptomatic assessment of therapeutic endpoints (43). Guidelines suggest measuring serum lactate within 6 hours for suspected severe sepsis or septic shock patients. Lactate levels over 4 mmol/L indicate the need for early resuscitation therapy. Moreover, improved morbidity and mortality in severe sepsis and septic shock are linked to lactate clearance, aligning with the focus of SSC on treating tissue hypoperfusion early in resuscitation. Studies have shown that lactate clearance <10% has good specificity and sensitivity as an assessment to predict morbidity and mortality during hospitalization (15). Additionally, an analysis of the relevant database concluded that the guideline’s emphasis on measuring lactate provides tangible clinical benefits to patients (44). Subsequent studies have validated the idea that lactate can guide sepsis treatment (5).
2.4 Risk stratification
Accumulating evidence suggests the feasibility of applying lactate levels to sepsis risk stratification. For example, a retrospective multi-center study suggested that clinicians can utilize blood lactate concentrations greater than 0.75 mmol/L as an indicator to identify patients at an elevated risk of mortality (45). Patients in the emergency department with suspected infection and moderate lactate levels face a moderate to high mortality risk, even in the absence of hypotension (46). This suggests that lactate levels have substantial prognostic value in critical illness, including sepsis. Of note, it is reported that modifying treatments based on surrogate physiological targets from invasive catheter measurements is not essential for reducing mortality (47). This aligns with findings that serial blood lactate monitoring is equally effective as catheter-based measurements (5, 48). Moreover, research indicates that lactate clearance is associated with reduced mortality in critically ill patients, offering optimal prognostic utility for clinical application (1).
2.5 Integration into diagnosis and treatment algorithms
The pivotal role of lactate is reinforced in the Sepsis-3 definition of septic shock, which is distinguished from sepsis by the need for vasopressors to sustain a mean arterial pressure of 65 mm Hg or higher and a serum lactate level exceeding 2 mmol/L without hypovolemia (6). Lactate levels are also recommended for screening undifferentiated adult patients suspected of having sepsis, even when it’s not yet confirmed (49). Moreover, Gattinoni et al. (50) noted that understanding why lactate levels rise can lead to better treatment strategies, especially in deciding how aggressively to administer fluids to individuals. It was suggested that even if lactate levels do not fully normalize, values close to normal can signify effective resuscitation (51).
2.6 Ongoing research
In addition to being recognized as an important biomarker, lactate is also involved in the host immune responses by serving as a vital energy source for immune cells and other tissues in shock (52, 53). Recently, we and others have reported that lactate is a potent signaling molecule in mediating immune cell dysfunction and cardiovascular injuries in sepsis (21, 23, 54, 55). In addition, it has been reported that lactate regulates histone acetylation through inhibiting histone deacetylases, leading to altered gene expression (56). This observation highlights the role of lactate as an epigenetic modulator. In agreement, a recent study by Zhang and colleagues discovered that lactate induces a novel post-translational modification, named lactylation in which a lactyl group is added to lysine residues in histones (57). Lactate-induced histone lactylation differs not only in mechanisms from lactate-induced histone acetylation but also in the specific genes affected (57, 58). It is noteworthy that we and others have reported that lactate can promote the lactylation of non-histone proteins, such as high mobility group box 1 (HMGB1) and Snail1, in sepsis and other disease states (21, 55). This review examines the most recent advances in the mechanisms by which lactate regulates immune cell responses in sepsis/septic shock.
3 Sources of lactate in sepsis/septic shock
In sepsis and septic shock, lactate levels increase due to multiple factors (as shown in Figure 1). However, considering the potential overlap among individual causes, we lean towards a simplified classification based on the mechanism of lactate elevation: increased lactate production and impaired catabolism.
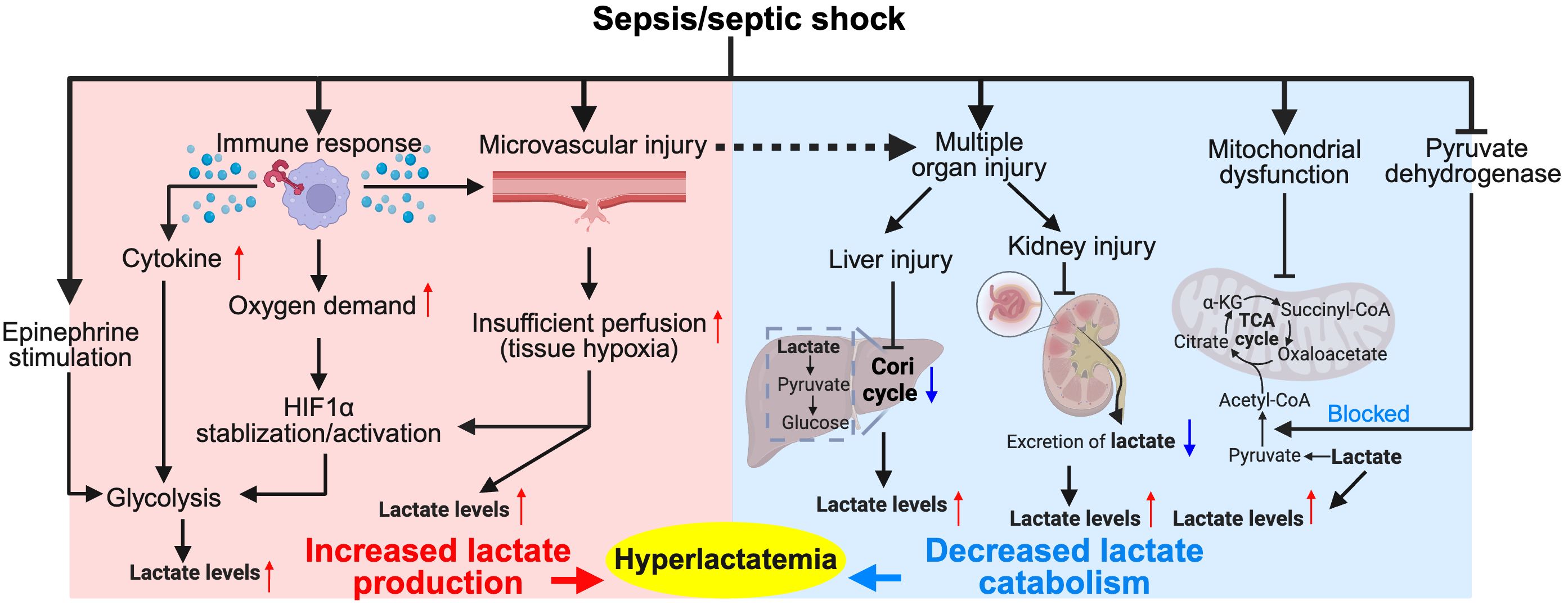
Figure 1. Major pathophysiological mechanisms of hyperlactatemia in sepsis/septic shock. The diagram illustrates the complex interplay between the immune response, tissue hypoxia, and metabolic alterations during sepsis and septic shock. Activation of immune cells leads to increased cytokine production, which along with epinephrine stimulation, enhances glycolysis and stabilizes hypoxia-inducible factor 1-alpha (HIF1α), resulting in increased lactate production. Tissue hypoxia due to microvascular injury further exacerbates lactate production. Concurrently, the Cori cycle in the liver and kidneys converts lactate back to glucose, but this process is often impaired in sepsis due to organ dysfunction, leading to decreased lactate catabolism and contributing to hyperlactatemia. This condition is further complicated by mitochondrial dysfunction, which impairs the conversion of pyruvate into the tricarboxylic acid (TCA) cycle intermediates, exacerbating lactate accumulation. Hyperlactatemia serves as both a marker of metabolic distress and a target for therapeutic intervention in sepsis management. Red colored arrows indicate activated events, while blue colored arrows indicate suppressed events, following sepsis/septic shock.
3.1 Increased lactate production
The human body constantly produces lactic acid (59), with levels spiking under increased cellular oxygen demand and/or reduced oxygen supply. In contrast to increased oxygen demand, hypoxia not only directly leads to lactate production, but also inhibits the degradation of Hypoxia-inducible Factor 1-alpha (HIF1α) and promotes its transcriptional activation (60). HIF-1α plays a multifaced role in regulating glycolysis and lactate production. It enhances glycolysis by inducing the transcription of glycolytic enzymes and membrane transporters, thereby increasing glucose flux (61, 62). Simultaneously, the expression of LDHA, a critical enzyme for lactate production, is heightened upon HIF-1α activation, leading to elevated lactate levels (63). During the hyperinflammatory phase of sepsis, increased oxygen consumption by activated immune cells leads to tissue hypoxia, which, in turn, stabilizes the transcription factor HIF-1α and consequently increases lactate production (64–66). For example, changes in glycolytic metabolism, induced by Toll-like receptors (TLRs), are key to activating dendritic cells, with anaerobic ATP production proving beneficial in low-oxygen conditions typical of infection/inflammation (67). This is consistent with a previous report that HIF-1α boosts lipopolysaccharide (LPS)-induced glycolysis in dendritic cells (68). Therefore, increased oxygen demands during inflammatory responses underscores a critical adaptation of activated immune cells to HIF-1α-dependent elevation of lactate production.
In the early phase, sepsis is characterized by a pronounced surge of the pro-inflammatory cytokines (69). Numerous studies have indicated that pro-inflammatory cytokines, especially interleukin (IL)-1β, are critical mediators in aerobic glycolysis and lactate production (70–73). Palsson-McDermott et al. demonstrated that TLR4-mediated tetramerization of pyruvate Kinase M2 (PKM2) promotes the transcription of IL-1β, leading to enhanced lactate production in LPS-activated macrophages (74). In addition, other pro-inflammatory cytokines, including IL-2, IL-3, IL-7, interferon-γ (IFN-γ) and tumor necrosis factor-α (TNF-α), also enforce the glucose metabolism and lactate production (71–73). These observations are in line with a previous study showing that lactate production in sepsis may be attributed more to inflammation rather than serving solely as a marker of tissue hypoxia (75).
It is noteworthy that enhanced lactate production in response to infection is a ubiquitous phenomenon and can occur in nearly all cells during sepsis. Early T cell activation (minutes to hours) increases aerobic glycolysis and diverts pyruvate to lactate production in a T cell receptor (TCR)-dependent mechanism (76). Neutrophils exhibit high glycolytic activity with limited mitochondrial respiration (77, 78). It is reported that human neutrophils can secrete lactic acid via a monocarboxylate transporter (79). In a murine model of acute inflammation, it is further illustrated that nicotinamide adenine dinucleotide phosphate (NADPH) oxidase (NOX)/reactive oxygen species (ROS)-mediated HIF-1α activation is required for lactate production in activated neutrophils (80). While activated immune cells are recognized as a primary source of lactate production during sepsis, the condition also induces upregulation of glycolysis and lactate production in numerous other cells and tissues. Endothelial cells (ECs) constitute the inner cellular lining of the blood vessels (81). Emerging evidence indicates that activated ECs rely heavily on glycolysis rather than on OXPHOS for ATP production during immune responses due to the low mitochondrial content, which consequently enhance lactate accumulation (82–85).
3.2 Impaired catabolism of lactate
If a large amount of lactic acid accumulates in the body, lactic acidosis will ensue (86). In response, efficient mechanisms are required for its clearance. The homeostasis of lactate is primarily maintained through its catabolism, which involves the conversion of lactate into pyruvate through the lactate dehydrogenase B (LDHB) enzyme (87). Pyruvate then enters the TCA cycle in mitochondria for further oxidation and energy production through pyruvate dehydrogenase (PDH), contributing to irreversible lactate removal (88). In severe sepsis, mitochondrial dysfunction (16, 89) and pyruvate dehydrogenase dysregulation (90–93) decrease OXPHOS, which interferes with the TCA cycle. This, in turn, accelerates lactate accumulation in sepsis. Intriguingly, emerging evidence from both clinical and pre-clinical studies indicates the activity of PDH is decreased in sepsis (90, 94, 95). It is reported that PDH activity and quantity are significantly lower in the peripheral blood mononuclear cells of patients with sepsis than the control group (90). Further analysis showed that the level of PDH activity is lower in sepsis non-survivors when compared to survivors (90). Importantly, an inverse association between baseline lactate levels and PDH activity in these patients, suggesting that PDH dysregulation contributes to enhanced lactate levels in sepsis (90). In a rat model of sepsis, induced by intraperitoneal inoculation of Escherichia coli and Bacteroides fragilis, active form of PDH is decreased by 70% in skeletal muscle (94). A recent study also reports that decreased PDH activity in endothelial cells leads to lactate production and endothelial injuries in LPS-induced sepsis (95). Mechanistically, sepsis stimulates the activation of pyruvate dehydrogenase kinase (PDHK), which negatively regulates PDH activation via inhibitory phosphorylation (96).
In addition, excess lactate can be resolved via the Cori cycle or lactic acid cycle (97). Circulating lactate shuttles to the liver, where it is reutilized by hepatocytes through gluconeogenesis to form glucose again (97). Also, it can be oxidized and removed by different tissues or secreted into the urine via the kidneys. However, we must acknowledge that the phenomenon of hepatic and renal dysfunction is not uncommon in severe sepsis and septic shock, which could potentially contribute to hyperlactatemia in sepsis. This notion is supported by the observation that higher lactate levels correlate with higher Sequential Organ Failure Assessment (SOFA) and quick SOFA (qSOFA) scores (98, 99). Other conditions, such as peripheral shunting (36) and heightened adrenergic stimulation (37) can also cause hyperlactatemia, but their prevalence and clinical significance in sepsis patients remain unclear.
4 Lactate regulates immune cell function in sepsis
In sepsis, lactate plays a pivotal role in regulating immune cell functions and metabolic processes (Figures 2–4). This regulation occurs through multiple mechanisms. Lactate interacts with immune cells via specific receptors and transport mechanisms. Primarily, lactate transport across cell membranes depends on monocarboxylate transporters (MCTs), particularly MCT1 and MCT4 (21, 100). MCT belongs to the solute carrier 16 (SLC16) family (101). MCT1, encoded by the SLC16A1 gene, primarily facilitates the uptake of lactate into cells, including liver cells for gluconeogenesis (102–104). MCT4, found in glycolytically active cells, predominantly manages the export of lactate, crucial for maintaining high rates of glycolysis, and its expression is upregulated via the myeloid differentiation primary response 88 (MYD88)/nuclear factor kappa-light-chain-enhancer of activated B cells (NF-kB) pathway (105, 106). Moreover, sodium-conjugated lactate can be transported by SLC5A8 and SLC5A12 (107). Indeed, the unique effects on CD8+ and CD4+ T cells are determined by the distinct expression of MCT1 and SCL5A12, respectively (108).
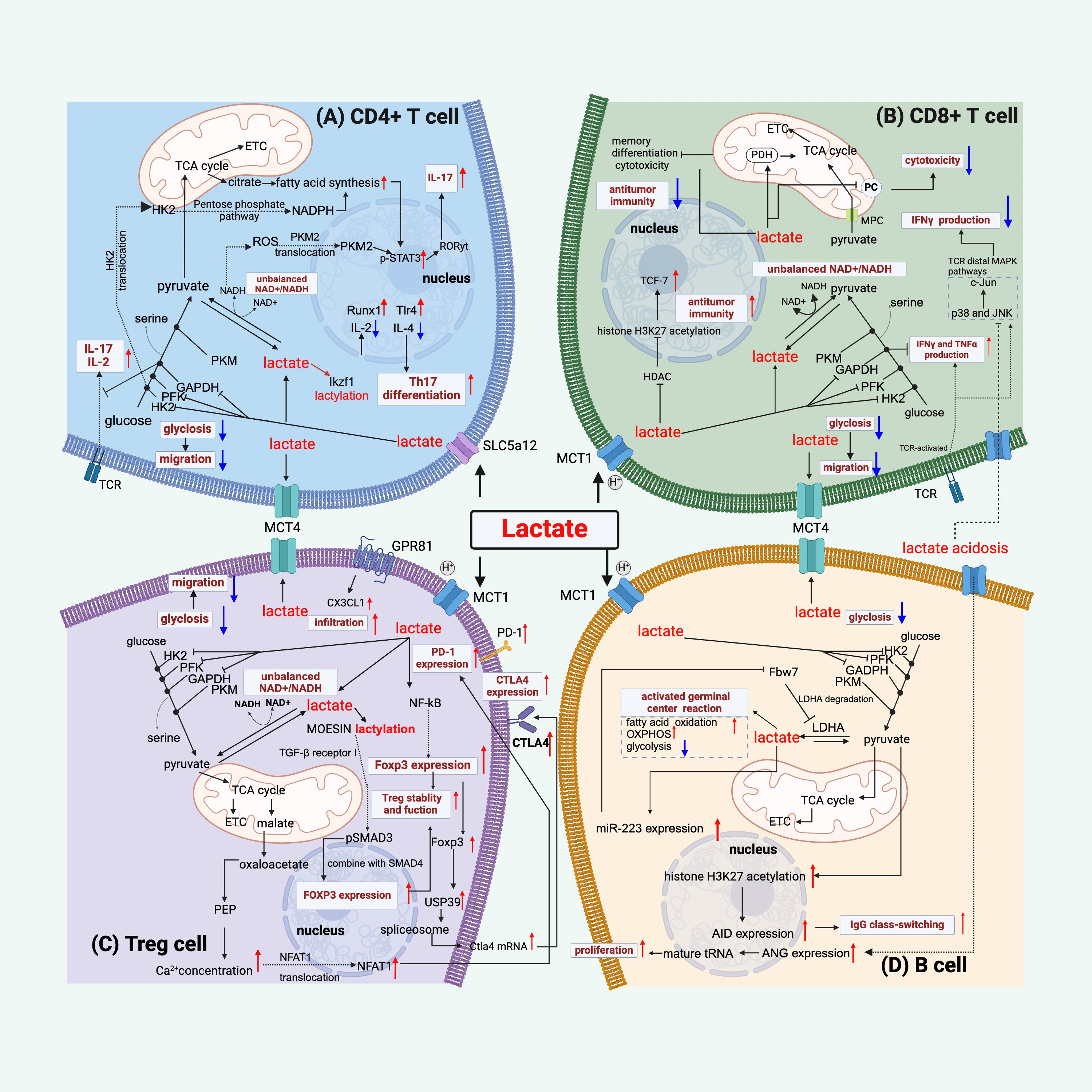
Figure 2. Lactate regulates lymphocyte function. (A) Lactate regulates CD4+ T cell function by the following mechanisms: inhibiting glycolysis and thus migration, disrupting the balance between NAD+ and NADH, mediating an increase in STAT3 phosphorylation by affecting PKM2 nuclear translocation and facilitating fatty acid synthesis, thereby increasing IL-17 production, and regulating gene expression by lactylation of Ikzf1, which facilitates differentiation to Th17 cells. (B) Lactate modulates CD8+ T cells by mechanisms such as, inhibiting glycolysis and thus migration, disrupting the balance between NAD+ and NADH, affecting pyruvate metabolism and thus reducing cytotoxicity, inhibiting histone deacetylase (HDAC) and thus promoting acetylation of histone H3K27 and thus increasing anti-tumor immunity, and its inhibition of the glycolytic enzyme GAPDH contributes to the production of IFN-γ, but appears to inhibit TCR-mediated IFN-γ production. (C) For Treg cells, in addition to limiting glycolysis, lactate binding to GPR81 enhances their infiltrative capacity, it improves PD1 expression by increasing the NAFT1 nuclear translocation mechanism, and it mediates high CTLA4 expression by up-regulating Foxp3, and lactate also promotes the lactylation of MOESIN to improve Treg function. (D) Lactate acts on B cells by the following mechanisms: promotion of germinal center function, inhibition of glycolysis, promotion of proliferation through increased ANG expression or positive feedback through miR-223-mediated lactate production, and lactate mediates the acetylation of histone H3K27 and thus promotes IgG class switching. Red colored arrows indicate activated events, while blue colored arrows indicate suppressed events, by lactate during sepsis/septic shock.
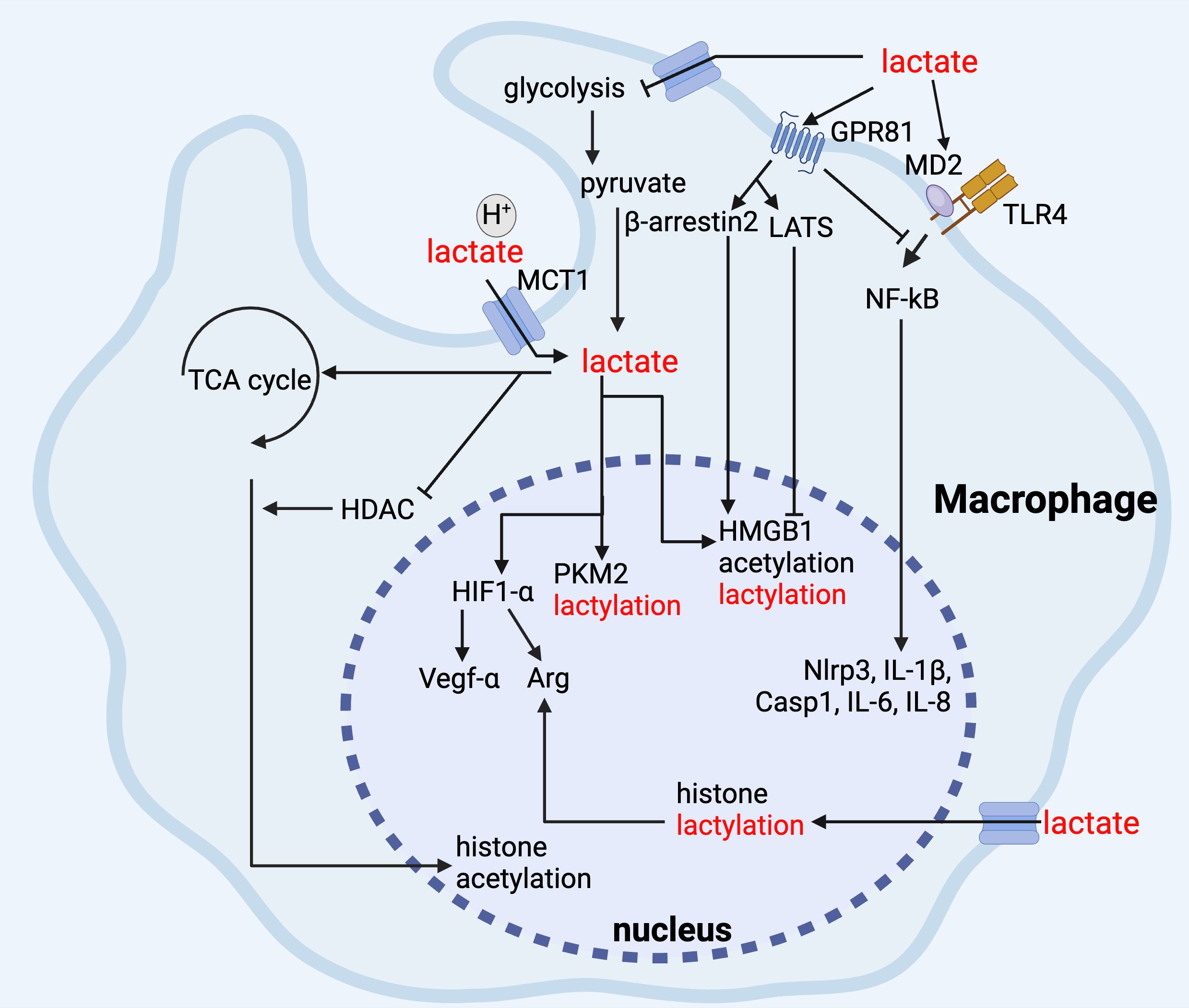
Figure 3. Lactate regulates macrophage function. In macrophages, lactate inhibits glycolysis, and its binding to GPR81 inhibits TLR-mediated pro-inflammatory responses via NF-kB, and it promotes Arg-1 and Vegf-α gene expression via up-regulation of HIF-1α, and the elevation of Arg-1 promotes histone lactylation, and lactate maintains its anti-inflammatory function via inhibition of HDAC and promotion of histone acetylation via TCA cycling. In addition, lactate can maintain anti-inflammatory function by inhibiting HDAC and promoting TCA cycle to promote histone acetylation, whereas for non-histone proteins, such as HMGB1, lactate can mediate lactylation and acetylation through β-arrestin2 promotion of p300/CBP and LATS/YAP-mediated inhibition of SIRT1.
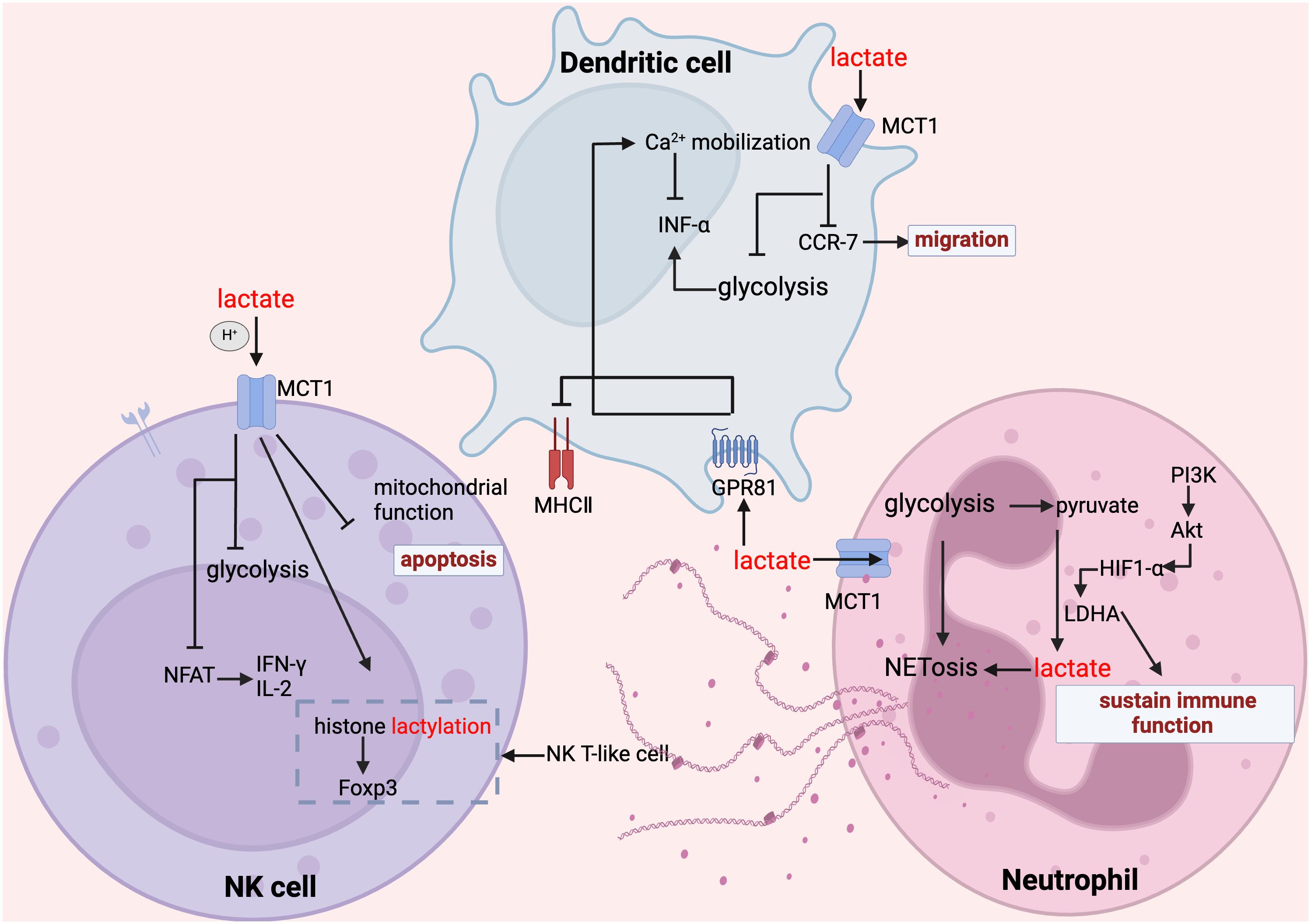
Figure 4. Lactate regulates NK cell, dendritic cell and neutrophil function. In NK cells, lactate inhibits glycolysis, suppresses mitochondrial function, inhibits the expression of NFAT and NKp46, promotes apoptosis and attenuates their ability to secrete inflammatory factors; whereas, for NK-T cells, the increase in histone lactylation promotes the expression of Foxp3. In dendritic cells, lactate inhibited glycolysis, inhibited CCR-7 and thus migration, lactate inhibited MHC II function through GPR81 signaling, and in addition GPR81-mediated Ca2+ mobilization inhibited IFN-α secretion. In mesangial cells, lactate maintains their immune function and promotes their NET formation.
The main receptor for lactate is the GPR81, also known as hydroxycarboxylic acid receptor 1 (HCAR1), which plays a significant role in mediating lactate’s effects on energy and lipid metabolism, neuron protection, and inflammation (105, 109–118). In critical illnesses, such as sepsis and cancer, where immune dysregulation is prevalent, understanding the lactate mediated signaling is essential (20, 98, 119–121). Accumulating clinical and pre-clinical evidence has suggested that lactate is a potent modulator of immune responses by influencing both the activity and the metabolic regulation of immune cells (122). A prospective cohort study in septic shock patients found that changes in lymphocytic mitochondrial metabolism correlate with post-resuscitation arterial lactate levels but not with hypoperfusion status (123). Additionally, lactate regulates immune cell function through post-translational modifications such as lactylation, acetylation and phosphorylation, alter the activities of enzymes and the functions of various proteins (21, 23, 124). In sepsis-associated lung injury, histone lactylation-regulated methyltransferase-like 3 (METTL3) promotes ferroptosis (125). This complex interplay highlights the importance of lactate in immune regulation during sepsis, underscoring its potential as a target for therapeutic intervention.
4.1 Lymphocytes
The impact of sepsis and septic shock on T and B lymphocytes has been reported previously (126–129). Davie et al. (126) found that lower immunoglobulin G1 (IgG1), IgM, IgA in B cells and disrupted T cell ratios at Intensive Care Unit (ICU) admission signaled poor outcomes, while sepsis brings fluctuating immunoglobulin levels and worsening B/T cell changes. In addition, septic shock further depletes B cell IgM and alters T cell markers, with post-mortem findings of reduced lymphoid structure and T cell function, revealing profound immune suppression (126). However, understanding the origins and progression of lymphopenia remains a significant knowledge gap in the immunology of pre-sepsis and sepsis (130). It is intriguing that a growing corpus of evidence underscores the significance of lactate in this context.
T cells encompass various subsets, and distinct T cell subsets have different metabolic characteristics. Our discussion focuses on effector T cells, specifically CD4+ and CD8+ T cells, along with regulatory T (Treg) cells. In sepsis, all effector T cells except Treg cells decline (127), coupled with studies suggesting T lymphocyte exhaustion as a central aspect of the septic immunosuppressive effects (128, 129).
At the cellular level, lactate impacts the function and quantity of effector T cells. It inhibits glycolysis and restricts effector T cell proliferation through redox changes and reductive stress, independent of acidity (131–133). Recent research has shown that lactic acid undermines T cell function by weakening the T cell redox system through a reduction in oxidant and antioxidant molecule production (134). Additionally, lactic acid will accumulate and inhibit the differentiation of T cells in vitro (132). However, this effect appears to be pH-dependent, as normalizing the pH levels restores T cell function (131).
In sepsis, elevated expression of programmed cell death protein-1 (PD-1) or its ligand PD-L1 impairs T cell function, increasing mortality (135, 136). Targeting these molecules has shown promise in mitigating sepsis-induced immunosuppression by improving T cell efficacy (137, 138). Cellular signaling pathway studies revealed that lactate’s role extends to promoting PD-1 expression via nuclear factor of activated T cells1 (NFAT1) translocation by GPR81 signaling (139), then inducing effector T cell apoptosis and impairing cytotoxicity (140, 141). Elevated PD-1 and higher PD-L1 on antigen-presenting cells mark immune suppression beyond T cells, making lactate/lactate-associated signaling a valuable target. Recent research supports that lactate can induce a polarization of the effector phenotype of CD4+ T cells, which can lead to more IL-17 and IL-2 production (108, 142), and of CD8+ T cells, resulting in increased IFN-γ production (142). This increase is dependent on the activation of CD3/T cell receptor (TCR) signaling.
At the metabolic level, lactate can serve as an alternative carbon source for T cells, supporting its metabolism, and fostering polarization and activation (143, 144). Interestingly, lactate stimulates the mitochondrial electron transport chain without being metabolized, which boosts naive T cell proliferation and then their effector capabilities (145). In addition, lactate can induce metabolic reprogramming of T cells by enhancing fatty acid synthesis. This is mediated by nuclear PKM2/signal transducer and activator of transcription 3 (STAT3) signaling (146). High lactate can also mediate pyruvate shunt by affecting pyruvate carboxylase activity in CD8+ T cells, which in turn contributes to their decreased cytotoxicity (147). Moreover, lactate is reported to promote memory phenotype differentiation in CD8+ T cells by interfering with mitochondrial pyruvate metabolism (148) and supports TCA cycle anaplerosis in effector T cells (143). Interestingly, HIF-1α-induced mitochondrial metabolic reprogramming may be responsible for persistent infection-associated T cell exhaustion (149). However, the significance of lactate in relation to this mechanism requires thorough evaluation. Notably, immune cells undergo metabolic changes during both the hyperinflammatory and immunotolerant stages of sepsis, highlighting the significance of lactate in these processes.
At the epigenetic level, lactate-induced lactylation plays a crucial role in CD4+ T cell differentiation. Specifically, high lactylation on the transcription factor Ikzf1 enhances T helper type 17 (Th17) cell differentiation by directly influencing the expression of Th17-related genes, such as Runx1, Tlr4, IL-2, and IL-4 (150). Lactate can suppress histone deacetylase activity which results in increased acetylation of Tcf7 (56) then can enhance the function of anti-tumor immune (151) and stemness of CD8+ T cells (152). Understanding these epigenetic mechanisms could provide insights into new therapeutic strategies for modulating immune responses in sepsis.
Regulatory T (Treg) cells play a vital role in the pathogenesis of critical illness, including sepsis and cancer, through their immunosuppressive functions (153, 154). Treg cells persistently increase during the late phase of sepsis (155), which contributes to the impaired immune responses in sepsis. A key aspect of their immunosuppressive role is that Treg cells can thrive in high lactate conditions without losing proliferative or functional capacity (156, 157). Mechanistic studies revealed that lactate mitigates the harmful effects of high glucose on Treg cells (158). In addition, lactate is reported to promote the transformation of naive T cells into Treg cells by activating NF-kB signaling and upregulates Foxp3, which supports the expansion of Treg cell populations and suppresses T cell pathogenicity (159, 160). In tumor microenvironment, lactate bolsters Treg cell stability and function by promoting MOESIN lactylation (161). A recent study has shown that lactate treatment enhances MOESIN lactylation, which boosts its interaction with TGF-β receptor I, and lactate also activates downstream TGF-β signaling through SMAD3 phosphorylation, leading to enhanced differentiation of Treg cells (161). I believe that this partly explains the mechanisms of combining anti-PD-1 with reduced lactate production by LDH inhibitor is more effective than anti-PD-1 alone (161). Since cancers and sepsis share similar immunosuppressive profiles, suppressed lactate production, which reduces Treg cell induction, is noteworthy. Cytotoxic T lymphocyte antigen 4 (CTLA-4) is a crucial immune checkpoint receptor that inhibits the activation and proliferation of T cell in sepsis (162, 163). Pre-clinical studies demonstrated that treatment with anti-CTLA-4 antibody maintains effector T cell function and improves sepsis survival outcomes (162, 164). Notably, lactate enhances the expression of CTLA-4 in tumor-infiltrating Treg cells via altering RNA splicing (165). It is intriguing to investigate whether lactate modulates CTLA-4 expression in Treg cells, potentially impairing immune responses in sepsis.
Sepsis is associated with significant B cells depletion due to apoptosis (166). An observational study revealed that the depletion of memory B cell populations played a role in sepsis-induced immunosuppression (167). B cells not only contribute to antibody production, but crucially enhance cytokine responses and bacterial clearance via communications with other immune cells, such as macrophages (168–171). Therefore, the decline in B cell numbers and impaired B cell function are considered as a prognostic biomarker for sepsis deterioration (171–174). Particularly, innate response activator (IRA) B cells are key in regulating inflammatory responses and managing sepsis outcomes, with their dysfunction linked to increased mortality, marking them and their produced IL-23 as potential targets for therapy (175, 176). Also, in sepsis, macrophage inflammation is intensified and lipolysis in adipose tissue is hindered due to the age-associated accumulation of B cells (170). However, compared to T cells, our understanding of the regulatory effects of lactate on B cells is relatively limited. B cells generate antibodies through germinal center (GC) and extrafollicular reactions (177). A recent study indicates that LDHA knockout in B cells hinders GC formation and antibody responses (178). And high lactate ensuring pyruvate for H3K27 acetylation, crucial for IgG class-switching (179).
Beyond direct evidence, the sophisticated mechanisms of lactate metabolism in lymphoma research may provide new insights for sepsis research. Increased levels of LDH are correlated with a higher mortality rate in B cell lymphoma patients (180, 181). Serum LDH is a key marker for aggressive non-Hodgkin lymphoma and is one of the factors listed in the International Prognostic index (182, 183). Moreover, lactic acid promotes B cell proliferation (184, 185), either by cleavage of mature tRNA at the anticodon loop via enhanced angiogenin (ANG) expression (186), or by inducing miR-223 expression to target Fbw7 (187). Additionally, blocking MCT exposes the therapeutic potential for virus-induced lymphomas (188).
4.2 Macrophages
Macrophages are widely distributed and present in almost all tissues and organs performing various functions, primarily maintaining internal environmental balance and resisting the invasion of pathogens (189). The unique characteristic of macrophages is their ability to polarize into different phenotypes, such as the M1-like macrophages and M2-like macrophages, in different microenvironments (190). M1 macrophages exhibit a pro-inflammatory phenotype, which can release various inflammatory cytokines and promote the inflammatory response. In contrast, M2 macrophages display an anti-inflammatory phenotype capable of producing an anti-inflammatory response and repairing damaged tissues (191). In sepsis-induced immunosuppression, macrophages exhibit altered cytokine secretion with decreased levels of TNF-α, IL-1β, and IL-12, and increased levels of TGF-β, IL-10, and macrophage migration inhibitory factor (MIF) (192). In addition, antigen presentation is reduced in the immunosuppressive stage of sepsis, as evidenced by lower human leukocyte antigen-DR isotype (HLA-DR) expression and decreased antigen uptake (192).
Substantial evidence has demonstrated that lactate exerts several inhibitory effects on pro-inflammatory (M1) macrophages, including hindering their migration, glycolysis, inflammasome assembly, and chemokine and cytokine secretion (122). Activation of TLRs stimulates the production of pro-inflammation cytokines and induces the polarization of macrophages towards M1 phenotype. Notably, we and others have shown that lactic acid reduces LPS-induced production of pro-inflammatory cytokines in macrophages (193) and promotes macrophage polarization into M2 macrophages (194). Indeed, it is found that lactic acid promotes the transcription of genes associated with M2 macrophage polarization, a process reliant on MCT function, HIF-1α activation, and induction of inducible cyclic adenosine monophosphate (cAMP) early repressor (ICER) (195–198). Our recent study also revealed that lactic acid suppresses NF-κB p65 nuclear translocation, a typical inflammatory signal (23, 194), via GPR81 signaling in macrophages (23, 80, 199). Additionally, lactate disrupts the assembly of TLR-4-mediated NLR family pyrin domain containing 3 (NLRP3) inflammasome and IL-1β secretion in a GPR81-dependent mechanism in macrophages (113). Given that lactate preferably promotes M2 macrophage polarization and inhibits M1 polarization, it is hypothesized that in sepsis, elevated lactate levels could worsen the immunosuppressive state by driving macrophages toward an anti-inflammatory M2 phenotype. This shift may further impair pathogen clearance and weaken pro-inflammatory responses, exacerbating sepsis-induced immunosuppression. Further investigation into targeting lactate metabolism in macrophages could offer therapeutic potential in sepsis.
Lactate can also regulate the function of macrophages by serving as a critical mediator in the metabolic-epigenetic link (57, 200, 201). Previous studies indicate that lactate is a primary carbon source for histone acetylation, significantly influencing epigenetic modifications (202–204). Shi and colleagues recently discovered that lactate fuels histone H3K27 acetylation, enabling the expression of immunosuppressive genes like Nr4a1, thus transcriptionally repressing macrophage pro-inflammatory functions (204). This histone acetylation leads to LPS tolerance and results in long-term immunosuppression. In addition, lactate-induced lactylation of histone H3 lysine 18 residue (H3K18la) is found to increase the production of inflammatory cytokines such as IL-2, IL-5, IL-6, IL-8, IL-10, IL-17, IFN-α, and arginine (Arg) in patients, thereby accelerating the development of an anti-inflammatory response of macrophages in sepsis (205). As lactyl and acetyl groups both stem from glucose metabolism and share regulatory enzymes, there might be a dynamic equilibrium between histone lactylation and acetylation (206). However, the exact nature of this equilibrium is not fully understood. Of note, H3K18la levels correlate with SOFA scores, ICU stay time, and lactate levels, suggesting that H3K18la is a potential biomarker for the diagnosis and prognosis of septic shock (205). However, additional clinically relevant data are still required to substantiate this. Histone lactylation also aligns with inflammatory markers in sepsis, as confirmed by other studies (205, 207).
Lactate-induced non-histone lactylation modifications also have inevitable impacts on macrophage functions in sepsis. High mobility group box 1 (HMGB1) is a non-histone DNA binding protein, which can be released into extracellular environment and as a late mediator of endotoxin lethality in sepsis (208–210). As a DAMP, extracellular HMGB1 is tightly associated with several types of cell death in sepsis, including apoptosis, autophagy, pyroptosis, and ferroptosis, which can deeply influence macrophage function (209, 211–217). Our recent study demonstrated a novel role of lactate in promoting HMGB1 lactylation and acetylation within macrophages, leading to consequent release of HMGB1 via exosome secretion in polymicrobial sepsis (21). It is also reported that the lactylation of PKM2 critically hampers glycolysis and shifts macrophages towards a repair-oriented phenotype (218). This change is marked by an increased expression of Arg-1, which supports wound healing, thus highlighting PKM2 as a pivotal regulator of macrophage metabolic adaptations (218). The role of lactate in promoting phosphorylation is also important. We have demonstrated that lactate reduces TNF-α and IL-6 levels in LPS-stimulated macrophages by inhibiting NF-κB and yes-associated protein (YAP) activation (23). Mechanistically, lactate triggers the activation of AMPK and LATS1 in a GPR81-dependent manner, leading to YAP phosphorylation and its subsequent degradation (23).
While the previous discussion highlights lactate’s inhibitory effects on macrophage activation, it is also important to recognize that lactate paradoxically exerts pro-inflammatory effects. It is reported that lactic acid triggers the production of IL-23 in peripheral blood mononuclear cells (PMBCs) in the presence of LPS stimulation, which may subsequently stimulate lymphocyte activation (219). Similarly, Samuvel et al. found that lactate activates myeloid differentiation factor 2 (MD2), a co-receptor for TLR4, which intensifies the TLR4-mediated pro-inflammatory response and increases NF-κB pathway-dependent gene transcription in human macrophages (220). Mechanistic study further revealed that the lactate-enhanced TLR4 signaling activation is mediated by lactate transporter MCT (220). Furthermore, studies emphasize that lactate can fuel a specialized glycolytic process in macrophages, reliant on the enzyme PFKFB2, which, when activated by efferocytosis, continuously supports further efferocytosis (221). Therefore, further refined experiments are necessary to clarify this discrepancy.
4.3 NK cells
Natural killer (NK) cells are effector lymphocytes of the innate immune system. They are uniquely primed for rapid and non-specific innate immune response against infections, without the need of antigen-presenting cells or prior exposure to pathogens (222, 223). As the primary innate lymphocyte population, NK cells are pivotal in orchestrating early responses to bacterial infections. Although they bolster the antimicrobial functions of myeloid cells, especially macrophages, by producing interferon-γ (IFN-γ) (222), a large number of animal experiments and human-related studies have confirmed the deleterious effects of overwhelming activation of NK cells in acute sepsis (224–227). It is noteworthy that sepsis rapidly induces phenotypic alterations and extensive cellular apoptosis in various types of immune cells, including NK cells, leading to profound immune paralysis (228–232). Single-cell RNA sequencing revealed downregulation of cytotoxic genes in NK cells among late-stage sepsis patients, possibly tied to recurring severe infections (233). Jensen et al. discovered that a reduction in NK cells correlates with a worse sepsis prognosis (234), which is consistent with a previous study by Giamarellos-Bourboulis et al. showing that severe Gram-negative sepsis patients with increased NK cells survived longer that those patients with relatively lower NK cells (235).
Accumulating evidence indicates that high levels of lactate contribute to impaired function and decreased numbers of NK cells in both inflammatory diseases (236) and cancers (237–241). Using a murine cytomegalovirus (MCMV) model of infection, Dodard et al. found that lactate, independent of acidification, preferentially induces cellular apoptosis of tissue resident NK (trNK) cells when compared with conventional NK (cNK) cells in the liver (236). In addition, mechanistic studies revealed that mitochondrial fitness is impaired in trNK cells in comparison to cNK cells, which intensifies the cytotoxicity of lactate to trNK cells (236). In agreement, lactate-induced apoptosis of NK cells via enhancing mitochondrial stress is observed in colorectal liver metastasis (CRLM) tumors (237). Moreover, lactate is reported to decrease nuclear factor of activated T cells (NFAT), impairing NFAT-dependent IL-2, which is necessary for NK cell function (238, 242, 243). This deters NK cell activation and reduces IFN-γ, hindering immune surveillance in cancers (238). Downregulation of MCT4, a lactate transporter, restore the function of NK cells and the expression of cytokines (239). Lactate damages NK cell cytolytic function (240) via the SIX1/LDHA axis (241). Notably, this study found that in the lactate-rich malignant pleural fluid, forkhead box P3 (Foxp3)+ NKT-like cells showed increased histone lactylation at the Foxp3 promoter site, reduced by the lactate transporter inhibitor 7ACC2 (244). The above studies of lactate-NK cell associations have primarily concentrated on cancers, it may not be directly applicable to sepsis. However, given the versatile nature of protein and lactate localization within cells, these mechanisms should not be disregarded.
4.4 Dendritic cells
As a pivotal antigen-presenting cell (245), dendritic cells (DC) rely on elevated glycolysis for activation, leading to substantial lactic acid production (246). They express high levels of GPR81, enabling lactate to bind and subsequently suppress MHC-II expression (247) and impacting their maturation and differentiation (248–250). Consistent with this, dendritic cells increasingly show immunological tolerance towards pathogens in sepsis with elevated levels of lactate (251). Lactate downregulates C-C chemokine type 7 (CCR-7) (252), a migration molecule, and CD11c, a DC marker (253). Furthermore, lactate can hinder DC functionality by promoting Ca2+ mobilization to regulate IFN-α expression and reducing the levels of cAMP, IL-6, IL-12, and type I IFN in a GPR81-dependent mechanism (254). It is reported that DCs are a major source of IL-10 in infectious disease (255). IL-10 expression defines an immunosuppressive DC population (256–259). DCs exposed to high lactate environment express more IL-10 (260–263), suggesting that lactate induces an immunosuppressive phenotype of DCs. Interestingly, genetic depletion of lactate receptor GPR81 not only suppresses IL-10 production in DCs but also boosts the production of pro-inflammatory cytokines (IL-6, IL-1β and IL-12) in DCs, which protects against experimental colitis (264).
Beyond GPR81-dependent mechanisms, lactate influences DC function by altering antigen presentation and cross-priming of CD8+ T cells (265). Consistent with this, dysfunctional dendritic cells reduce T cell activation, emphasizing its role in sepsis-associated immune paralysis (251). In addition, lactate inhibits the polarization of monocytes into DCs (250, 266). Moreover, it has been shown that lactate reprograms the metabolism of DCs, resulting in reduced glycolysis and increased fatty acid oxidation (FAO) (193, 267). These may be important reasons for the decrease in the number of DCs in sepsis with elevated lactate levels. DC function in sepsis patients is closely linked to their specific microenvironment (268). It has been shown that preventing apoptosis in dendritic cells during sepsis have the potential to improve survival (269). With the advancement of DC-related sepsis clinical trials, exploring lactate could be worthwhile. These multifaceted roles of lactate underscore its significant impact on DCs in sepsis.
4.5 Neutrophils
Neutrophils are one of most important components of cellular innate immunity (270) and they make up the majority of bone marrow-derived white blood cells (271). They are the first cells to reach sites of infection and provide initial support before adaptive immune responses activate (272). Neutrophil dysregulation in sepsis at the early stage involves not only an increase in immature/nonfunctional neutrophils in the blood but also exacerbates sepsis pathology through free radical oxygen production (273). These functions are dependent on glycolysis (78, 274). The most notable characteristic of neutrophils is their phagocytic function, for which lactate can supply the required ATP. It is possible lactate may also enhance this function via PI3K/Akt signaling (275). Also, treatment with both endogenous and exogenous lactate enhances the ability of neutrophils to form neutrophil extracellular traps (NETs) (276). Wen et al. reviewed the possible mechanisms of NET contribution to sepsis and noted that similar extracellular traps may exist for macrophages, dendritic cells, mast cells, eosinophils, and basophils (277). Also, lactate buildup in sepsis can reduce neutrophil apoptosis by modulating the MCT1/PD-L1 pathway (278).
In terms of cell migration, lactate promotes expression of CXCL1 and CXCL2, which are neutrophil mobilizers, and increases bone marrow vascular permeability by GRP81 signaling to help neutrophil migration (80). Chowdhury et al. revealed different mechanisms of how lactate promotes neutrophil migration and worsen vascular injury by AKT/HIF-1α/LDHA signaling (270).
Some clinical research indicates that L-lactate level in sputum was positively correlated with neutrophil count (279–281), making lactate a potential biomarker of lung inflammation. Furthermore, the relationship between neutrophil count and concentration of plasma lactate, neutrophil-to-lymphocyte ratio, and the concentration of plasma lactate can predict the outcomes in patients with sepsis (282–284).
5 The promise of targeting lactate metabolism and lactate-associated signaling
Currently, the lack of effective treatments leaves a critical gap in the management of sepsis/septic shock. As discussed above, it has become clear that lactate is not merely a byproduct of anerobic metabolism but is also extensively involved in modulating immune responses in sepsis. This insight paves the way for new therapeutic avenues by targeting lactate metabolism and lactate-associated signaling pathway in sepsis.
Direct inhibition of lactate production has been evaluated in clinical trials, especially in the field of cancer. This approach specifically targets metabolic pathways involved in lactate synthesis, with a primary focus on LDH, a key enzyme in this process. Gossypol (AT-101), a potent LDHA inhibitor, has been evaluated in treating various types of cancers in Phase I, Phase II and single arm and randomized trials (285, 286). Within these trials, a modest benefit was observed both in monotherapy and in combination with chemoradiation therapies (285, 287–290). In addition, CHK-336, a first-in-class orally active LDHA inhibitor, has been assessed for its tolerability, safety, and pharmacokinetic (PK) profile in healthy volunteers (Phase I, NCT05367661, Chinook Therapeutics Inc.). However, no clinical trials have been conducted to evaluate the safety, tolerance, and efficacy of LDHA inhibitors in sepsis/septic shock. Given that lactate is ubiquitously produced by nearly all cells, suppressing LDHA could lead to unpredictable and potentially adverse effects in sepsis. In contrast to the direct suppression of lactate production, enhancing lactate clearance, via hemofiltration and renal replacement therapy, offers a more balanced approach to manage elevated lactate levels, particularly in conditions like sepsis where systemic metabolic demands are heightened (291–294).
This review highlights several classic signaling pathways targeted by lactate that have long been the focus of basic and clinical research, leading to the development of potential therapies such as HMGB1 inhibitors, MIF inhibitors, MAPK inhibitors, NF-κB inhibitors, Sirtuin-1 activators, and antioxidants. Among these targets are also membrane receptors, such as the TLR4 antagonist TAK-242 (295), GPR81 agonist 3,5-DHBA and antagonist 3-OBA (21, 23, 54), and MCT antagonist (100). And drugs targeting NETs with therapeutic potential for sepsis, such as small polyanions, are also being evaluated in sepsis-related clinical trials (Phase II, NCT06548854, Grand Medical Pty Ltd). Additionally, the observation that lactate elevates the expression of PD-1 and CTLA-4 in Treg cells may provide insights into whether lactate should be measured in clinical trials of immune checkpoint inhibitors for sepsis. However, the potential benefits of targeting these lactate-associated pathways could be compromised by the systemic nature of sepsis/septic shock.
It is noteworthy that cellular therapies using mesenchymal stem cells (MSCs) have been initiated in clinical trials for sepsis (296, 297). It is worth considering whether cell transfer of genetic modified immune cells (targeting MCTs, GPR81 or lactate-associated signaling) could overcome lactate-induced immunosuppression (298–300). We and others have reported that MCT-mediated lactate uptake and lactate/GPR81-dependent signaling are potentially involved in regulating immune cell responses in murine polymicrobial sepsis (21, 23, 80). Additionally, preclinical studies have demonstrated that the lactate/GPR81 axis plays a significant role in promoting cancer cachexia. This finding is further supported by clinical observations in lung cancer patients, where elevated serum lactate levels correlate with disease progression (301). Persistent inflammation, immunosuppression, and catabolism syndrome (PICS) can develop in patients who survive initial sepsis (302). Further research is needed to determine if lactate drives PICS similarly to cancer cachexia and if this can be confirmed in the context of sepsis/septic shock.
6 Conclusion
Lactate, initially deemed a metabolic waste, has emerged as a key player in regulating immune cells and inflammation. The role of lactate has been particularly scrutinized in the context of sepsis, where it serves as a crucial biomarker for monitoring patient status. Despite extensive research, the specific effects of lactic acid on different immune cells, such as B lymphocytes, remain unclear, highlighting a gap in our understanding of its broader impacts on the immune system. However, lactate-guided resuscitation leaves much to be desired. Understanding lactate as part of a complex metabolic network, closely linked to various immune cell responses in sepsis, shifts the focus away from viewing it solely as a therapeutic target. The mechanisms through which lactic acid influences receptors like GPR81 and GPR132 are also not thoroughly understood, highlighting a need for further investigation to pinpoint its precise roles and regulatory mechanisms in immune function. In sepsis, the relationship of lactate with immunosuppression is of significant interest, with definitive mechanisms yet to be established. Better understanding the influence of this molecule on immune cell function in sepsis could greatly enhance the effectiveness of immune-related clinical trials for this condition.
Author contributions
TZ: Software, Writing – original draft, Writing – review & editing. LC: Writing – original draft. GK: Writing – review & editing. ES: Writing – review & editing. XW: Writing – review & editing. TH: Writing – review & editing. DW: Writing – review & editing. CL: Writing – review & editing. MF: Writing – review & editing. KY: Conceptualization, Supervision, Writing – original draft, Writing – review & editing.
Funding
The author(s) declare that financial support was received for the research, authorship, and/or publication of this article. This work was supported, in part, by NIH grants AI174020 (CL), HL071837 (CL), HL153270 (CL), GM083016 (CL and DW), GM119197 (DW), and C06RR0306551 (ETSU), Shock Society Research Investigator Fellowship (MF), and Quillen Research Enhancement Award (KY).
Conflict of interest
The authors declare that the research was conducted in the absence of any commercial or financial relationships that could be construed as a potential conflict of interest.
The author(s) declared that they were an editorial board member of Frontiers, at the time of submission. This had no impact on the peer review process and the final decision.
Publisher’s note
All claims expressed in this article are solely those of the authors and do not necessarily represent those of their affiliated organizations, or those of the publisher, the editors and the reviewers. Any product that may be evaluated in this article, or claim that may be made by its manufacturer, is not guaranteed or endorsed by the publisher.
References
1. Zhang Z, Xu X. Lactate clearance is a useful biomarker for the prediction of all-cause mortality in critically ill patients: a systematic review and meta-analysis*. Crit Care Med. (2014) 42:2118–25. doi: 10.1097/CCM.0000000000000405
2. Andersen LW, Mackenhauer J, Roberts JC, Berg KM, Cocchi MN, Donnino MW. Etiology and therapeutic approach to elevated lactate levels. Mayo Clinic Proc. (2013) 88:1127–40. doi: 10.1016/j.mayocp.2013.06.012
3. Broder G, Weil MH. EXCESS LACTATE: AN INDEX OF REVERSIBILITY OF SHOCK IN HUMAN PATIENTS. Sci (New York NY). (1964) 143:1457–9. doi: 10.1126/science.143.3613.1457
4. Weil MH, Afifi AA. Experimental and clinical studies on lactate and pyruvate as indicators of the severity of acute circulatory failure (shock). Circulation. (1970) 41:989–1001. doi: 10.1161/01.cir.41.6.989
5. Jones AE, Shapiro NI, Trzeciak S, Arnold RC, Claremont HA, Kline JA. Lactate clearance vs central venous oxygen saturation as goals of early sepsis therapy: a randomized clinical trial. Jama. (2010) 303:739–46. doi: 10.1001/jama.2010.158
6. Singer M, Deutschman CS, Seymour CW, Shankar-Hari M, Annane D, Bauer M, et al. The third international consensus definitions for sepsis and septic shock (Sepsis-3). Jama. (2016) 315:801–10. doi: ajama.2016.0287
7. Vander Heiden MG, Cantley LC, Thompson CB. Understanding the Warburg effect: the metabolic requirements of cell proliferation. Sci (New York NY). (2009) 324:1029–33. doi: 10.1126/science.1160809
8. Rabinowitz JD, Enerback S. Lactate: the ugly duckling of energy metabolism. Nat Metab. (2020) 2:566–71. doi: 10.1038/s42255-020-0243-4
9. Fantin VR, St-Pierre J, Leder P. Attenuation of LDH-A expression uncovers a link between glycolysis, mitochondrial physiology, and tumor maintenance. Cancer Cell. (2006) 9:425–34. doi: 10.1016/j.ccr.2006.04.023
10. Prebble JN. The discovery of oxidative phosphorylation: a conceptual off-shoot from the study of glycolysis. Stud history philosophy Biol Biomed Sci. (2010) 41:253–62. doi: 10.1016/j.shpsc.2010.07.014
11. Glancy B, Kane DA, Kavazis AN, Goodwin ML, Willis WT, Gladden LB. Mitochondrial lactate metabolism: history and implications for exercise and disease. J Physiol. (2021) 599:863–88. doi: 10.1113/JP278930
12. Gray LR, Tompkins SC, Taylor EB. Regulation of pyruvate metabolism and human disease. Cell Mol Life Sci. (2014) 71:2577–604. doi: 10.1007/s00018-013-1539-2
13. Al Tameemi W, Dale TP, Al-Jumaily RMK, Forsyth NR. Hypoxia-modified cancer cell metabolism. Front Cell Dev Biol. (2019) 7:4. doi: 10.3389/fcell.2019.00004
14. Cohen RD, Woods HF, Krebs HA. Clinical and biochemical aspects of lactic acidosis. New Jersey: Blackwell Scientific Publications Oxford (1976).
15. Nguyen HB, Rivers EP, Knoblich BP, Jacobsen G, Muzzin A, Ressler JA, et al. Early lactate clearance is associated with improved outcome in severe sepsis and septic shock. Crit Care Med. (2004) 32:1637–42. doi: 10.1097/01.CCM.0000132904.35713.A7
16. Brealey D, Brand M, Hargreaves I, Heales S, Land J, Smolenski R, et al. Association between mitochondrial dysfunction and severity and outcome of septic shock. Lancet (London England). (2002) 360:219–23. doi: 10.1016/S0140-6736(02)09459-X
17. Bakker J, Gris P, Coffernils M, Kahn RJ, Vincent JL. Serial blood lactate levels can predict the development of multiple organ failure following septic shock. Am J surgery. (1996) 171:221–6. doi: 10.1016/S0002-9610(97)89552-9
18. Ye L, Jiang Y, Zhang M. Crosstalk between glucose metabolism, lactate production and immune response modulation. Cytokine Growth factor Rev. (2022) 68:81–92. doi: 10.1016/j.cytogfr.2022.11.001
19. Faix JD. Biomarkers of sepsis. Crit Rev Clin Lab Sci. (2013) 50:23–36. doi: 10.3109/10408363.2013.764490
20. Wang ZH, Peng WB, Zhang P, Yang XP, Zhou Q. Lactate in the tumour microenvironment: From immune modulation to therapy. EBioMedicine. (2021) 73:103627. doi: 10.1016/j.ebiom.2021.103627
21. Yang K, Fan M, Wang X, Xu J, Wang Y, Tu F, et al. Lactate promotes macrophage HMGB1 lactylation, acetylation, and exosomal release in polymicrobial sepsis. Cell Death Differ. (2022) 29:133–46. doi: 10.1038/s41418-021-00841-9
22. Nolt B, Tu F, Wang X, Ha T, Winter R, Williams DL, et al. Lactate and immunosuppression in sepsis. Shock (Augusta Ga). (2018) 49:120–5. doi: 10.1097/SHK.0000000000000958
23. Yang K, Xu J, Fan M, Tu F, Wang X, Ha T, et al. Lactate suppresses macrophage pro-inflammatory response to LPS stimulation by inhibition of YAP and NF-κB activation via GPR81-mediated signaling. Front Immunol. (2020) 11:587913. doi: 10.3389/fimmu.2020.587913
24. Kompanje EJ, Jansen TC, van der Hoven B, Bakker J. The first demonstration of lactic acid in human blood in shock by Johann Joseph Scherer (1814-1869) in January 1843. Intensive Care Med. (2007) 33:1967–71. doi: 10.1007/s00134-007-0788-7
25. Peretz DI, Scott HM, Duff J, Dossetor JB, MacLean LD, McGregor M. The significance of lacticacidemia in the shock syndrome. Ann New York Acad Sci. (1965) 119:1133–41. doi: 10.1111/j.1749-6632.1965.tb47467.x
26. Ballinger WF, Vollenweider H, Pierucci L, Templeton JY. The accumulation and removal of excess lactate in arterial blood during hypothermia and biventricular bypass. Surgery. (1962) 51(6):738–45.
27. Peretz DI, McGregor M, Dossetor JB. LACTICACIDOSIS: A CLINICALLY SIGNIFICANT ASPECT OF SHOCK. Can Med Assoc J. (1964) 90:673–5.
28. Weil MH, Michaels S, Rackow EC. Comparison of blood lactate concentrations in central venous, pulmonary artery, and arterial blood. Crit Care Med. (1987) 15:489–90. doi: 10.1097/00003246-198705000-00006
29. Huckabee WE. Relationships of pyruvate and lactate during anaerobic metabolism. II. Exercise and formation of O-debt. J Clin Invest. (1958) 37:255–63. doi: 10.1172/JCI103604
30. Huckabee WE. Relationships of pyruvate and lactate during anaerobic metabolism. I. Effects of infusion of pyruvate or glucose and of hyperventilation. J Clin Invest. (1958) 37:244–54. doi: 10.1172/JCI103603
31. Vincent JL, Dufaye P, Berré J, Leeman M, Degaute JP, Kahn RJ. Serial lactate determinations during circulatory shock. Crit Care Med. (1983) 11:449–51. doi: 10.1097/00003246-198306000-00012
32. Vincent JL, Sakr Y, Sprung CL, Ranieri VM, Reinhart K, Gerlach H, et al. Sepsis in European intensive care units: results of the SOAP study. Crit Care Med. (2006) 34:344–53. doi: 10.1097/01.CCM.0000194725.48928.3A
33. Trzeciak S, Dellinger RP, Chansky ME, Arnold RC, Schorr C, Milcarek B, et al. Serum lactate as a predictor of mortality in patients with infection. Intensive Care Med. (2007) 33:970–7. doi: 10.1007/s00134-007-0563-9
34. Cohen RD, Woods HF. Lactic acidosis revisited. Diabetes. (1983) 32:181–91. doi: 10.2337/diab.32.2.181
35. Gutierrez G, Wulf ME. Lactic acidosis in sepsis: a commentary. Intensive Care Med. (1996) 22:6–16. doi: 10.1007/BF01728325
36. Ince C, Mik EG. Microcirculatory and mitochondrial hypoxia in sepsis, shock, and resuscitation. J Appl Physiol (Bethesda Md: 1985). (2016) 120:226–35. doi: 10.1152/japplphysiol.00298.2015
37. James JH, Luchette FA, McCarter FD, Fischer JE. Lactate is an unreliable indicator of tissue hypoxia in injury or sepsis. Lancet (London England). (1999) 354:505–8. doi: 10.1016/S0140-6736(98)91132-1
38. Bernardin G, Pradier C, Tiger F, Deloffre P, Mattei M. Blood pressure and arterial lactate level are early indicators of short-term survival in human septic shock. Intensive Care Med. (1996) 22:17–25. doi: 10.1007/BF01728326
39. Bakker J, Coffernils M, Leon M, Gris P, Vincent JL. Blood lactate levels are superior to oxygen-derived variables in predicting outcome in human septic shock. Chest. (1991) 99:956–62. doi: 10.1378/chest.99.4.956
40. Haupt MT. Goal-oriented hemodynamic therapy. New Engl J Med. (1996) 334:799. doi: 10.1056/NEJM199603213341213
41. Rivers E, Nguyen B, Havstad S, Ressler J, Muzzin A, Knoblich B, et al. Early goal-directed therapy in the treatment of severe sepsis and septic shock. New Engl J Med. (2001) 345:1368–77. doi: 10.1056/NEJMoa010307
42. Bone RC, Balk RA, Cerra FB, Dellinger RP, Fein AM, Knaus WA, et al. Definitions for sepsis and organ failure and guidelines for the use of innovative therapies in sepsis. The ACCP/SCCM Consensus Conference Committee. American College of Chest Physicians/Society of Critical Care Medicine. Chest. (1992) 101:1644–55. doi: 10.1378/chest.101.6.1644
43. Dellinger RP, Carlet JM, Masur H, Gerlach H, Calandra T, Cohen J, et al. Surviving Sepsis Campaign guidelines for management of severe sepsis and septic shock. Crit Care Med. (2004) 32:858–73. doi: 10.1097/01.CCM.0000117317.18092.E4
44. Casserly B, Phillips GS, Schorr C, Dellinger RP, Townsend SR, Osborn TM, et al. Lactate measurements in sepsis-induced tissue hypoperfusion: results from the Surviving Sepsis Campaign database. Crit Care Med. (2015) 43:567–73. doi: 10.1097/CCM.0000000000000742
45. Nichol AD, Egi M, Pettila V, Bellomo R, French C, Hart G, et al. Relative hyperlactatemia and hospital mortality in critically ill patients: a retrospective multi-centre study. Crit Care (London England). (2010) 14:R25. doi: 10.1186/cc8888
46. Puskarich MA, Illich BM, Jones AE. Prognosis of emergency department patients with suspected infection and intermediate lactate levels: a systematic review. J Crit Care. (2014) 29:334–9. doi: 10.1016/j.jcrc.2013.12.017
47. Rowan KM, Angus DC, Bailey M, Barnato AE, Bellomo R, Canter RR, et al. Early, goal-directed therapy for septic shock - A patient-level meta-analysis. New Engl J Med. (2017) 376:2223–34. doi: 10.1056/NEJMoa1701380
48. Lilly CM. The ProCESS trial–a new era of sepsis management. New Engl J Med. (2014) 370:1750–1. doi: 10.1056/NEJMe1402564
49. Evans L, Rhodes A, Alhazzani W, Antonelli M, Coopersmith CM, French C, et al. Surviving sepsis campaign: international guidelines for management of sepsis and septic shock 2021. Intensive Care Med. (2021) 47:1181–247. doi: 10.1007/s00134-021-06506-y
50. Gattinoni L, Vasques F, Camporota L, Meessen J, Romitti F, Pasticci I, et al. Understanding lactatemia in human sepsis. Potential impact for early management. Am J Respir Crit Care Med. (2019) 200:582–9. doi: 10.1164/rccm.201812-2342OC
51. Arnold MJ. Surviving sepsis: updated guidelines from the society of critical care medicine. Am Family physician. (2022) 106:589–90.
52. Zhuang R, Chen J, Cheng HS, Assa C, Jamaiyar A, Pandey AK, et al. Perivascular fibrosis is mediated by a KLF10-IL-9 signaling axis in CD4+ T cells. Circ Res. (2022) 130:1662–81. doi: 10.1161/CIRCRESAHA.121.320420
53. Matejovic M, Radermacher P, Fontaine E. Lactate in shock: a high-octane fuel for the heart? Intensive Care Med. (2007) 33:406–8. doi: 10.1007/s00134-006-0524-8
54. Yang K, Fan M, Wang X, Xu J, Wang Y, Gill PS, et al. Lactate induces vascular permeability via disruption of VE-cadherin in endothelial cells during sepsis. Sci Adv. (2022) 8:eabm8965. doi: 10.1126/sciadv.abm8965
55. Fan M, Yang K, Wang X, Chen L, Gill PS, Ha T, et al. Lactate promotes endothelial-to-mesenchymal transition via Snail1 lactylation after myocardial infarction. Sci Adv. (2023) 9:eadc9465. doi: 10.1126/sciadv.adc9465
56. Feng Q, Liu Z, Yu X, Huang T, Chen J, Wang J, et al. Lactate increases stemness of CD8 + T cells to augment anti-tumor immunity. Nat Commun. (2022) 13:4981. doi: 10.1038/s41467-022-32521-8
57. Zhang D, Tang Z, Huang H, Zhou G, Cui C, Weng Y, et al. Metabolic regulation of gene expression by histone lactylation. Nature. (2019) 574:575–80. doi: 10.1038/s41586-019-1678-1
58. Zong Z, Xie F, Wang S, Wu X, Zhang Z, Yang B, et al. Alanyl-tRNA synthetase, AARS1, is a lactate sensor and lactyltransferase that lactylates p53 and contributes to tumorigenesis. Cell. (2024) 187:2375–92 e33. doi: 10.1016/j.cell.2024.04.002
59. Rogatzki MJ, Ferguson BS, Goodwin ML, Gladden LB. Lactate is always the end product of glycolysis. Front Neurosci. (2015) 9:22. doi: 10.3389/fnins.2015.00022
60. Hayashi Y, Yokota A, Harada H, Huang G. Hypoxia/pseudohypoxia-mediated activation of hypoxia-inducible factor-1α in cancer. Cancer science. (2019) 110:1510–7. doi: 10.1111/cas.13990
61. Semenza GL. Targeting HIF-1 for cancer therapy. Nat Rev Cancer. (2003) 3:721–32. doi: 10.1038/nrc1187
62. Wang T, Liu H, Lian G, Zhang SY, Wang X, Jiang C. HIF1α-induced glycolysis metabolism is essential to the activation of inflammatory macrophages. Mediators inflammation. (2017) 2017:9029327. doi: 10.1155/2017/9029327
63. Ivashkiv LB. The hypoxia-lactate axis tempers inflammation. Nat Rev Immunol. (2020) 20:85–6. doi: 10.1038/s41577-019-0259-8
64. Fitzpatrick SF. Immunometabolism and sepsis: A role for HIF? Front Mol Biosci. (2019) 6:85. doi: 10.3389/fmolb.2019.00085
65. Kim JW, Tchernyshyov I, Semenza GL, Dang CV. HIF-1-mediated expression of pyruvate dehydrogenase kinase: a metabolic switch required for cellular adaptation to hypoxia. Cell Metab. (2006) 3:177–85. doi: 10.1016/j.cmet.2006.02.002
66. Yang L, Xie M, Yang M, Yu Y, Zhu S, Hou W, et al. PKM2 regulates the Warburg effect and promotes HMGB1 release in sepsis. Nat Commun. (2014) 5:4436. doi: 10.1038/ncomms5436
67. Krawczyk CM, Holowka T, Sun J, Blagih J, Amiel E, DeBerardinis RJ, et al. Toll-like receptor-induced changes in glycolytic metabolism regulate dendritic cell activation. Blood. (2010) 115:4742–9. doi: 10.1182/blood-2009-10-249540
68. Jantsch J, Chakravortty D, Turza N, Prechtel AT, Buchholz B, Gerlach RG, et al. Hypoxia and hypoxia-inducible factor-1 alpha modulate lipopolysaccharide-induced dendritic cell activation and function. J Immunol (Baltimore Md: 1950). (2008) 180:4697–705. doi: 10.4049/jimmunol.180.7.4697
69. Shimaoka M, Park EJ. Advances in understanding sepsis. Eur J Anaesthesiol Suppl. (2008) 42:146–53. doi: 10.1017/S0265021507003389
70. Kvacskay P, Yao N, Schnotz JH, Scarpone R, Carvalho RA, Klika KD, et al. Increase of aerobic glycolysis mediated by activated T helper cells drives synovial fibroblasts towards an inflammatory phenotype: new targets for therapy? Arthritis Res Ther. (2021) 23:56. doi: 10.1186/s13075-021-02437-7
71. Bauer DE, Harris MH, Plas DR, Lum JJ, Hammerman PS, Rathmell JC, et al. Cytokine stimulation of aerobic glycolysis in hematopoietic cells exceeds proliferative demand. FASEB J. (2004) 18:1303–5. doi: 10.1096/fj.03-1001fje
72. Holt V, Moren B, Fryklund C, Colbert RA, Stenkula KG. Acute cytokine treatment stimulates glucose uptake and glycolysis in human keratinocytes. Cytokine. (2023) 161:156057. doi: 10.1016/j.cyto.2022.156057
73. Wang F, Zhang S, Jeon R, Vuckovic I, Jiang X, Lerman A, et al. Interferon gamma induces reversible metabolic reprogramming of M1 macrophages to sustain cell viability and pro-inflammatory activity. EBioMedicine. (2018) 30:303–16. doi: 10.1016/j.ebiom.2018.02.009
74. Palsson-McDermott EM, Curtis AM, Goel G, Lauterbach MAR, Sheedy FJ, Gleeson LE, et al. Pyruvate kinase M2 regulates hif-1alpha activity and IL-1beta induction and is a critical determinant of the warburg effect in LPS-activated macrophages. Cell Metab. (2015) 21:347. doi: 10.1016/j.cmet.2015.01.017
75. Haji-Michael PG, Ladriere L, Sener A, Vincent JL, Malaisse WJ. Leukocyte glycolysis and lactate output in animal sepsis and ex vivo human blood. Metabolism. (1999) 48:779–85. doi: 10.1016/s0026-0495(99)90179-8
76. Menk AV, Scharping NE, Moreci RS, Zeng X, Guy C, Salvatore S, et al. Early TCR signaling induces rapid aerobic glycolysis enabling distinct acute T cell effector functions. Cell Rep. (2018) 22:1509–21. doi: 10.1016/j.celrep.2018.01.040
77. Oren R, Farnham AE, Saito K, Milofsky E, Karnovsky ML. Metabolic patterns in three types of phagocytizing cells. J Cell Biol. (1963) 17:487–501. doi: 10.1083/jcb.17.3.487
78. Maianski NA, Geissler J, Srinivasula SM, Alnemri ES, Roos D, Kuijpers TW. Functional characterization of mitochondria in neutrophils: a role restricted to apoptosis. Cell Death Differ. (2004) 11:143–53. doi: 10.1038/sj.cdd.4401320
79. Simchowitz L, Textor JA. Lactic acid secretion by human neutrophils. Evidence for an H+ + lactate- cotransport system. J Gen Physiol. (1992) 100:341–67. doi: 10.1085/jgp.100.2.341
80. Khatib-Massalha E, Bhattacharya S, Massalha H, Biram A, Golan K, Kollet O, et al. Lactate released by inflammatory bone marrow neutrophils induces their mobilization via endothelial GPR81 signaling. Nat Commun. (2020) 11:3547. doi: 10.1038/s41467-020-17402-2
81. Trimm E, Red-Horse K. Vascular endothelial cell development and diversity. Nat Rev Cardiol. (2023) 20:197–210. doi: 10.1038/s41569-022-00770-1
82. De Bock K, Georgiadou M, Schoors S, Kuchnio A, Wong BW, Cantelmo AR, et al. Role of PFKFB3-driven glycolysis in vessel sprouting. Cell. (2013) 154:651–63. doi: 10.1016/j.cell.2013.06.037
83. Oldendorf WH, Cornford ME, Brown WJ. The large apparent work capability of the blood-brain barrier: a study of the mitochondrial content of capillary endothelial cells in brain and other tissues of the rat. Ann Neurol. (1977) 1:409–17. doi: 10.1002/ana.410010502
84. Barth E, Stammler G, Speiser B, Schaper J. Ultrastructural quantitation of mitochondria and myofilaments in cardiac muscle from 10 different animal species including man. J Mol Cell Cardiol. (1992) 24:669–81. doi: 10.1016/0022-2828(92)93381-S
85. Zhang H, Wang Y, Qu M, Li W, Wu D, Cata JP, et al. Neutrophil, neutrophil extracellular traps and endothelial cell dysfunction in sepsis. Clin Transl Med. (2023) 13:e1170. doi: 10.1002/ctm2.1170
86. Bennis Y, Bodeau S, Batteux B, Gras-Champel V, Masmoudi K, Maizel J, et al. A study of associations between plasma metformin concentration, lactic acidosis, and mortality in an emergency hospitalization context. Crit Care Med. (2020) 48:e1194–e202. doi: 10.1097/CCM.0000000000004589
87. Lee TY. Lactate: a multifunctional signaling molecule. Yeungnam Univ J Med. (2021) 38:183–93. doi: 10.12701/yujm.2020.00892
88. Jha MK, Lee IK, Suk K. Metabolic reprogramming by the pyruvate dehydrogenase kinase-lactic acid axis: Linking metabolism and diverse neuropathophysiologies. Neurosci Biobehav Rev. (2016) 68:1–19. doi: 10.1016/j.neubiorev.2016.05.006
89. Garrabou G, Moren C, Lopez S, Tobias E, Cardellach F, Miro O, et al. The effects of sepsis on mitochondria. J Infect Dis. (2012) 205:392–400. doi: 10.1093/infdis/jir764
90. Nuzzo E, Berg KM, Andersen LW, Balkema J, Montissol S, Cocchi MN, et al. Pyruvate dehydrogenase activity is decreased in the peripheral blood mononuclear cells of patients with sepsis. A prospective observational trial. Ann Am Thorac Society. (2015) 12:1662–6. doi: 10.1513/AnnalsATS.201505-267BC
91. Vary TC, Siegel JH, Nakatani T, Sato T, Aoyama H. Effect of sepsis on activity of pyruvate dehydrogenase complex in skeletal muscle and liver. Am J Physiol. (1986) 250:E634–40. doi: 10.1152/ajpendo.1986.250.6.E634
92. Vary TC. Sepsis-induced alterations in pyruvate dehydrogenase complex activity in rat skeletal muscle: effects on plasma lactate. Shock (Augusta Ga). (1996) 6:89–94. doi: 10.1097/00024382-199608000-00002
93. Alamdari N, Constantin-Teodosiu D, Murton AJ, Gardiner SM, Bennett T, Layfield R, et al. Temporal changes in the involvement of pyruvate dehydrogenase complex in muscle lactate accumulation during lipopolysaccharide infusion in rats. J Physiol. (2008) 586:1767–75. doi: 10.1113/jphysiol.2007.149625
94. Vary TC, Hazen SA, Maish G, Cooney RN. TNF binding protein prevents hyperlactatemia and inactivation of PDH complex in skeletal muscle during sepsis. J Surg Res. (1998) 80:44–51. doi: 10.1006/jsre.1998.5324
95. Mao L, Sun M, Chen Z, Zeng Z, Wu J, Chen Z, et al. The pyruvate dehydrogenase complex mitigates LPS-induced endothelial barrier dysfunction by metabolic regulation. Shock (Augusta Ga). (2022) 57:308–17. doi: 10.1097/SHK.0000000000001931
96. Vary TC, Hazen S. Sepsis alters pyruvate dehydrogenase kinase activity in skeletal muscle. Mol Cell Biochem. (1999) 198:113–8. doi: 10.1023/A:1006993910781
97. Soeters PB, Shenkin A, Sobotka L, Soeters MR, de Leeuw PW, Wolfe RR. The anabolic role of the Warburg, Cori-cycle and Crabtree effects in health and disease. Clin Nutr (Edinburgh Scotland). (2021) 40:2988–98. doi: 10.1016/j.clnu.2021.02.012
98. Liu Z, Meng Z, Li Y, Zhao J, Wu S, Gou S, et al. Prognostic accuracy of the serum lactate level, the SOFA score and the qSOFA score for mortality among adults with Sepsis. Scand J Trauma Resusc Emerg Med. (2019) 27:51. doi: 10.1186/s13049-019-0609-3
99. Oh DH, Kim MH, Jeong WY, Kim YC, Kim EJ, Song JE, et al. Risk factors for mortality in patients with low lactate level and septic shock. J Microbiol Immunol Infect. (2019) 52:418–25. doi: 10.1016/j.jmii.2017.08.009
100. Caslin HL, Abebayehu D, Abdul Qayum A, Haque TT, Taruselli MT, Paez PA, et al. Lactic acid inhibits lipopolysaccharide-induced mast cell function by limiting glycolysis and ATP availability. J Immunol (Baltimore Md: 1950). (2019) 203:453–64. doi: 10.4049/jimmunol.1801005
101. Halestrap AP. The monocarboxylate transporter family–Structure and functional characterization. IUBMB Life. (2012) 64:1–9. doi: 10.1002/iub.573
102. Halestrap AP, Meredith D. The SLC16 gene family-from monocarboxylate transporters (MCTs) to aromatic amino acid transporters and beyond. Pflugers Archiv: Eur J Physiol. (2004) 447:619–28. doi: 10.1007/s00424-003-1067-2
103. Alarcón P, Manosalva C, Conejeros I, Carretta MD, Muñoz-Caro T, Silva LMR, et al. d(-) lactic acid-induced adhesion of bovine neutrophils onto endothelial cells is dependent on neutrophils extracellular traps formation and CD11b expression. Front Immunol. (2017) 8:975. doi: 10.3389/fimmu.2017.00975
104. Halestrap AP. The SLC16 gene family - structure, role and regulation in health and disease. Mol aspects Med. (2013) 34:337–49. doi: 10.1016/j.mam.2012.05.003
105. Sun S, Li H, Chen J, Qian Q. Lactic acid: no longer an inert and end-product of glycolysis. Physiol (Bethesda Md). (2017) 32:453–63. doi: 10.1152/physiol.00016.2017
106. Tan Z, Xie N, Banerjee S, Cui H, Fu M, Thannickal VJ, et al. The monocarboxylate transporter 4 is required for glycolytic reprogramming and inflammatory response in macrophages. J Biol Chem. (2015) 290:46–55. doi: 10.1074/jbc.M114.603589
107. Srinivas SR, Gopal E, Zhuang L, Itagaki S, Martin PM, Fei YJ, et al. Cloning and functional identification of slc5a12 as a sodium-coupled low-affinity transporter for monocarboxylates (SMCT2). Biochem J. (2005) 392:655–64. doi: 10.1042/BJ20050927
108. Haas R, Smith J, Rocher-Ros V, Nadkarni S, Montero-Melendez T, D’Acquisto F, et al. Lactate regulates metabolic and pro-inflammatory circuits in control of T cell migration and effector functions. PloS Biol. (2015) 13:e1002202. doi: 10.1371/journal.pbio.1002202
109. Sun Z, Han Y, Song S, Chen T, Han Y, Liu Y. Activation of GPR81 by lactate inhibits oscillatory shear stress-induced endothelial inflammation by activating the expression of KLF2. IUBMB Life. (2019) 71:2010–9. doi: 10.1002/iub.2151
110. Wu G, Dai Y, Yan Y, Zheng X, Zhang H, Li H, et al. The lactate receptor GPR81 mediates hepatic lipid metabolism and the therapeutic effect of metformin on experimental NAFLDs. Eur J Pharmacol. (2022) 924:174959. doi: 10.1016/j.ejphar.2022.174959
111. Laroche S, Stil A, Germain P, Cherif H, Chemtob S, Bouchard JF. Participation of L-lactate and its receptor HCAR1/GPR81 in neurovisual development. Cells. (2021) 10(7):1640. doi: 10.3390/cells10071640
112. Madaan A, Nadeau-Vallée M, Rivera JC, Obari D, Hou X, Sierra EM, et al. Lactate produced during labor modulates uterine inflammation via GPR81 (HCA(1)). Am J obstetrics gynecology. (2017) 216:60.e1–.e17. doi: 10.1016/j.ajog.2016.09.072
113. Hoque R, Farooq A, Ghani A, Gorelick F, Mehal WZ. Lactate reduces liver and pancreatic injury in Toll-like receptor- and inflammasome-mediated inflammation via GPR81-mediated suppression of innate immunity. Gastroenterology. (2014) 146:1763–74. doi: 10.1053/j.gastro.2014.03.014
114. de Castro Abrantes H, Briquet M, Schmuziger C, Restivo L, Puyal J, Rosenberg N, et al. The lactate receptor HCAR1 modulates neuronal network activity through the activation of G(α) and G(βγ) subunits. J neuroscience: Off J Soc Neurosci. (2019) 39:4422–33. doi: 10.1523/JNEUROSCI.2092-18.2019
115. Ohno Y, Oyama A, Kaneko H, Egawa T, Yokoyama S, Sugiura T, et al. Lactate increases myotube diameter via activation of MEK/ERK pathway in C2C12 cells. Acta physiologica (Oxford England). (2018) 223:e13042. doi: 10.1111/apha.13042
116. Roland CL, Arumugam T, Deng D, Liu SH, Philip B, Gomez S, et al. Cell surface lactate receptor GPR81 is crucial for cancer cell survival. Cancer Res. (2014) 74:5301–10. doi: 10.1158/0008-5472.CAN-14-0319
117. Liu C, Kuei C, Zhu J, Yu J, Zhang L, Shih A, et al. 3,5-Dihydroxybenzoic acid, a specific agonist for hydroxycarboxylic acid 1, inhibits lipolysis in adipocytes. J Pharmacol Exp Ther. (2012) 341:794–801. doi: 10.1124/jpet.112.192799
118. Harun-Or-Rashid M, Inman DM. Reduced AMPK activation and increased HCAR activation drive anti-inflammatory response and neuroprotection in glaucoma. J neuroinflammation. (2018) 15:313. doi: 10.1186/s12974-018-1346-7
119. Li Z, Wang Q, Huang X, Yang M, Zhou S, Li Z, et al. Lactate in the tumor microenvironment: A rising star for targeted tumor therapy. Front Nutr. (2023) 10:1113739. doi: 10.3389/fnut.2023.1113739
120. Apostolova P, Pearce EL. Lactic acid and lactate: revisiting the physiological roles in the tumor microenvironment. Trends Immunol. (2022) 43:969–77. doi: 10.1016/j.it.2022.10.005
121. Nalos M, Robergs R. Understanding hyperlactatemia in sepsis: are we there yet? Am J Respir Crit Care Med. (2019) 200:1069–70. doi: 10.1164/rccm.201905-0962LE
122. Caslin HL, Abebayehu D, Pinette JA, Ryan JJ. Lactate is a metabolic mediator that shapes immune cell fate and function. Front Physiol. (2021) 12:688485. doi: 10.3389/fphys.2021.688485
123. Nedel WL, Strogulski NR, Kopczynski A, Rodolphi MS, Montes THM, Júnior JA, et al. Association between hyperlactatemia, perfusional parameters, and lymphocyte mitochondrial dysfunction in septic shock patients. Shock (Augusta Ga). (2022) 57:378–83. doi: 10.1097/SHK.0000000000001868
124. Sun Z, Song Y, Li J, Li Y, Yu Y, Wang X. Potential biomarker for diagnosis and therapy of sepsis: Lactylation. Immunity Inflammation disease. (2023) 11:e1042. doi: 10.1002/iid3.1042
125. Wu D, Spencer CB, Ortoga L, Zhang H, Miao C. Histone lactylation-regulated METTL3 promotes ferroptosis via m6A-modification on ACSL4 in sepsis-associated lung injury. Redox Biol. (2024) 74:103194. doi: 10.1016/j.redox.2024.103194
126. Davies K, McLaren JE. Destabilisation of T cell-dependent humoral immunity in sepsis. Clin Sci (London England: 1979). (2024) 138:65–85. doi: 10.1042/CS20230517
127. Venet F, Chung CS, Kherouf H, Geeraert A, Malcus C, Poitevin F, et al. Increased circulating regulatory T cells (CD4(+)CD25 (+)CD127 (-)) contribute to lymphocyte anergy in septic shock patients. Intensive Care Med. (2009) 35:678–86. doi: 10.1007/s00134-008-1337-8
128. Li Q, Sun M, Zhou Q, Li Y, Xu J, Fan H. Integrated analysis of multi-omics data reveals T cell exhaustion in sepsis. Front Immunol. (2023) 14:1110070. doi: 10.3389/fimmu.2023.1110070
129. Inoue S, Suzuki K, Komori Y, Morishita Y, Suzuki-Utsunomiya K, Hozumi K, et al. Persistent inflammation and T cell exhaustion in severe sepsis in the elderly. Crit Care (London England). (2014) 18:R130. doi: 10.1186/cc13941
130. Rubio I, Osuchowski MF, Shankar-Hari M, Skirecki T, Winkler MS, Lachmann G, et al. Current gaps in sepsis immunology: new opportunities for translational research. Lancet Infect diseases. (2019) 19:e422–e36. doi: 10.1016/S1473-3099(19)30567-5
131. Fischer K, Hoffmann P, Voelkl S, Meidenbauer N, Ammer J, Edinger M, et al. Inhibitory effect of tumor cell-derived lactic acid on human T cells. Blood. (2007) 109:3812–9. doi: 10.1182/blood-2006-07-035972
132. Decking SM, Bruss C, Babl N, Bittner S, Klobuch S, Thomas S, et al. LDHB overexpression can partially overcome T cell inhibition by lactic acid. Int J Mol Sci. (2022) 23(11):5970. doi: 10.3390/ijms23115970
133. Quinn WJ 3rd, Jiao J, TeSlaa T, Stadanlick J, Wang Z, Wang L, et al. Lactate limits T cell proliferation via the NAD(H) redox state. Cell Rep. (2020) 33:108500. doi: 10.1016/j.celrep.2020.108500
134. Rostamian H, Khakpoor-Koosheh M, Jafarzadeh L, Masoumi E, Fallah-Mehrjardi K, Tavassolifar MJ, et al. Restricting tumor lactic acid metabolism using dichloroacetate improves T cell functions. BMC cancer. (2022) 22:39. doi: 10.1186/s12885-021-09151-2
135. Zhang Y, Li J, Lou J, Zhou Y, Bo L, Zhu J, et al. Upregulation of programmed death-1 on T cells and programmed death ligand-1 on monocytes in septic shock patients. Crit Care (London England). (2011) 15:R70. doi: 10.1186/cc10059
136. Ruan WS, Feng MX, Xu J, Xu YG, Song CY, Lin LY, et al. Early activation of myeloid-derived suppressor cells participate in sepsis-induced immune suppression via PD-L1/PD-1 axis. Front Immunol. (2020) 11:1299. doi: 10.3389/fimmu.2020.01299
137. Chen Y, Guo DZ, Zhu CL, Ren SC, Sun CY, Wang Y, et al. The implication of targeting PD-1:PD-L1 pathway in treating sepsis through immunostimulatory and anti-inflammatory pathways. Front Immunol. (2023) 14:1323797. doi: 10.3389/fimmu.2023.1323797
138. Chang KC, Burnham CA, Compton SM, Rasche DP, Mazuski RJ, McDonough JS, et al. Blockade of the negative co-stimulatory molecules PD-1 and CTLA-4 improves survival in primary and secondary fungal sepsis. Crit Care (London England). (2013) 17:R85. doi: 10.1186/cc12711
139. Kumagai S, Koyama S, Itahashi K, Tanegashima T, Lin YT, Togashi Y, et al. Lactic acid promotes PD-1 expression in regulatory T cells in highly glycolytic tumor microenvironments. Cancer Cell. (2022) 40:201–18.e9. doi: 10.1016/j.ccell.2022.01.001
140. Chen Y, Feng Z, Kuang X, Zhao P, Chen B, Fang Q, et al. Increased lactate in AML blasts upregulates TOX expression, leading to exhaustion of CD8(+) cytolytic T cells. Am J Cancer Res. (2021) 11:5726–42.
141. Xu J, Ma X, Yu K, Wang R, Wang S, Liu R, et al. Lactate up-regulates the expression of PD-L1 in kidney and causes immunosuppression in septic Acute Renal Injury. J Microbiol Immunol Infect. (2021) 54:404–10. doi: 10.1016/j.jmii.2019.10.006
142. Wen J, Cheng S, Zhang Y, Wang R, Xu J, Ling Z, et al. Lactate anions participate in T cell cytokine production and function. Sci China Life Sci. (2021) 64:1895–905. doi: 10.1007/s11427-020-1887-7
143. Tu VY, Ayari A, O’Connor RS. Beyond the lactate paradox: how lactate and acidity impact T cell therapies against cancer. Antibodies (Basel Switzerland). (2021) 10(3):25. doi: 10.3390/antib10030025
144. Barbieri L, Veliça P, Gameiro PA, Cunha PP, Foskolou IP, Rullman E, et al. Lactate exposure shapes the metabolic and transcriptomic profile of CD8+ T cells. Front Immunol. (2023) 14:1101433. doi: 10.3389/fimmu.2023.1101433
145. Cai X, Ng CP, Jones O, Fung TS, Ryu KW, Li D, et al. Lactate activates the mitochondrial electron transport chain independently of its metabolism. Mol Cell. (2023) 83:3904–20.e7. doi: 10.1016/j.molcel.2023.09.034
146. Pucino V, Certo M, Bulusu V, Cucchi D, Goldmann K, Pontarini E, et al. Lactate buildup at the site of chronic inflammation promotes disease by inducing CD4(+) T cell metabolic rewiring. Cell Metab. (2019) 30:1055–74.e8. doi: 10.1016/j.cmet.2019.10.004
147. Elia I, Rowe JH, Johnson S, Joshi S, Notarangelo G, Kurmi K, et al. Tumor cells dictate anti-tumor immune responses by altering pyruvate utilization and succinate signaling in CD8(+) T cells. Cell Metab. (2022) 34:1137–50.e6. doi: 10.1016/j.cmet.2022.06.008
148. Wenes M, Jaccard A, Wyss T, Maldonado-Pérez N, Teoh ST, Lepez A, et al. The mitochondrial pyruvate carrier regulates memory T cell differentiation and antitumor function. Cell Metab. (2022) 34:731–46.e9. doi: 10.1016/j.cmet.2022.03.013
149. Wu H, Zhao X, Hochrein SM, Eckstein M, Gubert GF, Knöpper K, et al. Mitochondrial dysfunction promotes the transition of precursor to terminally exhausted T cells through HIF-1α-mediated glycolytic reprogramming. Nat Commun. (2023) 14:6858. doi: 10.1038/s41467-023-42634-3
150. Fan W, Wang X, Zeng S, Li N, Wang G, Li R, et al. Global lactylome reveals lactylation-dependent mechanisms underlying T(H)17 differentiation in experimental autoimmune uveitis. Sci Adv. (2023) 9:eadh4655. doi: 10.1126/sciadv.adh4655
151. Rundqvist H, Veliça P, Barbieri L, Gameiro PA, Bargiela D, Gojkovic M, et al. Cytotoxic T-cells mediate exercise-induced reductions in tumor growth. eLife. (2020) 9:e59996. doi: 10.7554/eLife.59996
152. Hermans D, Gautam S, García-Cañaveras JC, Gromer D, Mitra S, Spolski R, et al. Lactate dehydrogenase inhibition synergizes with IL-21 to promote CD8(+) T cell stemness and antitumor immunity. Proc Natl Acad Sci United States America. (2020) 117:6047–55. doi: 10.1073/pnas.1920413117
153. Gerriets VA, Kishton RJ, Johnson MO, Cohen S, Siska PJ, Nichols AG, et al. Foxp3 and Toll-like receptor signaling balance T(reg) cell anabolic metabolism for suppression. Nat Immunol. (2016) 17:1459–66. doi: 10.1038/ni.3577
154. Weinberg SE, Singer BD, Steinert EM, Martinez CA, Mehta MM, Martínez-Reyes I, et al. Mitochondrial complex III is essential for suppressive function of regulatory T cells. Nature. (2019) 565:495–9. doi: 10.1038/s41586-018-0846-z
155. Monneret G, Debard AL, Venet F, Bohe J, Hequet O, Bienvenu J, et al. Marked elevation of human circulating CD4+CD25+ regulatory T cells in sepsis-induced immunoparalysis. Crit Care Med. (2003) 31:2068–71. doi: 10.1097/01.CCM.0000069345.78884.0F
156. Angelin A, Gil-de-Gómez L, Dahiya S, Jiao J, Guo L, Levine MH, et al. Foxp3 reprograms T cell metabolism to function in low-glucose, high-lactate environments. Cell Metab. (2017) 25:1282–93.e7. doi: 10.1016/j.cmet.2016.12.018
157. Lacroix R, Rozeman EA, Kreutz M, Renner K, Blank CU. Targeting tumor-associated acidity in cancer immunotherapy. Cancer immunology immunotherapy: CII. (2018) 67:1331–48. doi: 10.1007/s00262-018-2195-z
158. Watson MJ, Vignali PDA, Mullett SJ, Overacre-Delgoffe AE, Peralta RM, Grebinoski S, et al. Metabolic support of tumour-infiltrating regulatory T cells by lactic acid. Nature. (2021) 591:645–51. doi: 10.1038/s41586-020-03045-2
159. Comito G, Iscaro A, Bacci M, Morandi A, Ippolito L, Parri M, et al. Lactate modulates CD4(+) T-cell polarization and induces an immunosuppressive environment, which sustains prostate carcinoma progression via TLR8/miR21 axis. Oncogene. (2019) 38:3681–95. doi: 10.1038/s41388-019-0688-7
160. Lopez Krol A, Nehring HP, Krause FF, Wempe A, Raifer H, Nist A, et al. Lactate induces metabolic and epigenetic reprogramming of pro-inflammatory Th17 cells. EMBO Rep. (2022) 23:e54685. doi: 10.15252/embr.202254685
161. Gu J, Zhou J, Chen Q, Xu X, Gao J, Li X, et al. Tumor metabolite lactate promotes tumorigenesis by modulating MOESIN lactylation and enhancing TGF-β signaling in regulatory T cells. Cell Rep. (2022) 39:110986. doi: 10.1016/j.celrep.2022.110986
162. Inoue S, Bo L, Bian J, Unsinger J, Chang K, Hotchkiss RS. Dose-dependent effect of anti-CTLA-4 on survival in sepsis. Shock (Augusta Ga). (2011) 36:38–44. doi: 10.1097/SHK.0b013e3182168cce
163. Mewes C, Buttner B, Hinz J, Alpert A, Popov AF, Ghadimi M, et al. CTLA-4 genetic variants predict survival in patients with sepsis. J Clin Med. (2019) 8(1):70. doi: 10.3390/jcm8010070
164. Paterson CW, Fay KT, Chen CW, Klingensmith NJ, Gutierrez MB, Liang Z, et al. CTLA-4 checkpoint inhibition improves sepsis survival in alcohol-exposed mice. Immunohorizons. (2024) 8:74–88. doi: 10.4049/immunohorizons.2300060
165. Ding R, Yu X, Hu Z, Dong Y, Huang H, Zhang Y, et al. Lactate modulates RNA splicing to promote CTLA-4 expression in tumor-infiltrating regulatory T cells. Immunity. (2024) 57:528–40.e6. doi: 10.1016/j.immuni.2024.01.019
166. van der Poll T, van de Veerdonk FL, Scicluna BP, Netea MG. The immunopathology of sepsis and potential therapeutic targets. Nat Rev Immunol. (2017) 17:407–20. doi: 10.1038/nri.2017.36
167. Shankar-Hari M, Fear D, Lavender P, Mare T, Beale R, Swanson C, et al. Activation-associated accelerated apoptosis of memory B cells in critically ill patients with sepsis. Crit Care Med. (2017) 45:875–82. doi: 10.1097/CCM.0000000000002380
168. Zhang B, Vogelzang A, Miyajima M, Sugiura Y, Wu Y, Chamoto K, et al. B cell-derived GABA elicits IL-10(+) macrophages to limit anti-tumour immunity. Nature. (2021) 599:471–6. doi: 10.1038/s41586-021-04082-1
169. Suchanek O, Ferdinand JR, Tuong ZK, Wijeyesinghe S, Chandra A, Clauder AK, et al. Tissue-resident B cells orchestrate macrophage polarisation and function. Nat Commun. (2023) 14:7081. doi: 10.1038/s41467-023-42625-4
170. Carey A, Nguyen K, Kandikonda P, Kruglov V, Bradley C, Dahlquist KJV, et al. Age-associated accumulation of B cells promotes macrophage inflammation and inhibits lipolysis in adipose tissue during sepsis. Cell Rep. (2024) 43:113967. doi: 10.1016/j.celrep.2024.113967
171. Kelly-Scumpia KM, Scumpia PO, Weinstein JS, Delano MJ, Cuenca AG, Nacionales DC, et al. B cells enhance early innate immune responses during bacterial sepsis. J Exp Med. (2011) 208:1673–82. doi: 10.1084/jem.20101715
172. Guérin E, Orabona M, Raquil MA, Giraudeau B, Bellier R, Gibot S, et al. Circulating immature granulocytes with T-cell killing functions predict sepsis deterioration*. Crit Care Med. (2014) 42:2007–18. doi: 10.1097/CCM.0000000000000344
173. Duan S, Jiao Y, Wang J, Tang D, Xu S, Wang R, et al. Impaired B-cell maturation contributes to reduced B cell numbers and poor prognosis in sepsis. Shock (Augusta Ga). (2020) 54:70–7. doi: 10.1097/SHK.0000000000001478
174. Monserrat J, de Pablo R, Diaz-Martin D, Rodriguez-Zapata M, de la Hera A, Prieto A, et al. Early alterations of B cells in patients with septic shock. Crit Care (London England). (2013) 17:R105. doi: 10.1186/cc12750
175. Weber GF, Chousterman BG, He S, Fenn AM, Nairz M, Anzai A, et al. Interleukin-3 amplifies acute inflammation and is a potential therapeutic target in sepsis. Sci (New York NY). (2015) 347:1260–5. doi: 10.1126/science.aaa4268
176. Rauch PJ, Chudnovskiy A, Robbins CS, Weber GF, Etzrodt M, Hilgendorf I, et al. Innate response activator B cells protect against microbial sepsis. Sci (New York NY). (2012) 335:597–601. doi: 10.1126/science.1215173
177. Elsner RA, Shlomchik MJ. Germinal center and extrafollicular B cell responses in vaccination, immunity, and autoimmunity. Immunity. (2020) 53:1136–50. doi: 10.1016/j.immuni.2020.11.006
178. Sharma R, Smolkin RM, Chowdhury P, Fernandez KC, Kim Y, Cols M, et al. Distinct metabolic requirements regulate B cell activation and germinal center responses. Nat Immunol. (2023) 24:1358–69. doi: 10.1038/s41590-023-01540-y
179. Chi W, Kang N, Sheng L, Liu S, Tao L, Cao X, et al. MCT1-governed pyruvate metabolism is essential for antibody class-switch recombination through H3K27 acetylation. Nat Commun. (2024) 15:163. doi: 10.1038/s41467-023-44540-0
180. Yadav C, Ahmad A, D’Souza B, Agarwal A, Nandini M, Ashok Prabhu K, et al. Serum lactate dehydrogenase in non-hodgkin’s lymphoma: A prognostic indicator. Indian J Clin biochemistry: IJCB. (2016) 31:240–2. doi: 10.1007/s12291-015-0511-3
181. Vaccher E, Tirelli U, Spina M, Talamini R, Errante D, Simonelli C, et al. Age and serum lactate dehydrogenase level are independent prognostic factors in human immunodeficiency virus-related non-Hodgkin’s lymphomas: a single-institute study of 96 patients. J Clin oncology: Off J Am Soc Clin Oncol. (1996) 14:2217–23. doi: 10.1200/JCO.1996.14.8.2217
182. Ruppert AS, Dixon JG, Salles G, Wall A, Cunningham D, Poeschel V, et al. International prognostic indices in diffuse large B-cell lymphoma: a comparison of IPI, R-IPI, and NCCN-IPI. Blood. (2020) 135:2041–8. doi: 10.1182/blood.2019002729
183. William BM, Bongu NR, Bast M, Bociek RG, Bierman PJ, Vose JM, et al. The utility of lactate dehydrogenase in the follow up of patients with diffuse large B-cell lymphoma. Rev Bras hematologia e hemoterapia. (2013) 35:189–91. doi: 10.5581/1516-8484.20130054
184. Lee SC, Marzec M, Liu X, Wehrli S, Kantekure K, Ragunath PN, et al. Decreased lactate concentration and glycolytic enzyme expression reflect inhibition of mTOR signal transduction pathway in B-cell lymphoma. NMR biomedicine. (2013) 26:106–14. doi: 10.1002/nbm.2825
185. Pike SE, Markey SP, Ijames C, Jones KD, Tosato G. The role of lactic acid in autocrine B-cell growth stimulation. Proc Natl Acad Sci United States America. (1991) 88:11081–5. doi: 10.1073/pnas.88.24.11081
186. Mo X, Du S, Chen X, Wang Y, Liu X, Zhang C, et al. Lactate induces production of the tRNA(His) half to promote B-lymphoblastic cell proliferation. Mol therapy: J Am Soc Gene Ther. (2020) 28:2442–57. doi: 10.1016/j.ymthe.2020.09.010
187. Yao S, Guo T, Zhang F, Chen Y, Xu F, Luo D, et al. Fbw7 inhibits the progression of activated B-cell like diffuse large B-cell lymphoma by targeting the positive feedback loop of the LDHA/lactate/miR-223 axis. Front Oncol. (2022) 12:842356. doi: 10.3389/fonc.2022.842356
188. Bonglack EN, Messinger JE, Cable JM, Ch’ng J, Parnell KM, Reinoso-Vizcaino NM, et al. Monocarboxylate transporter antagonism reveals metabolic vulnerabilities of viral-driven lymphomas. Proc Natl Acad Sci United States America. (2021) 118(25):e2022495118. doi: 10.1073/pnas.2022495118
189. Davies LC, Jenkins SJ, Allen JE, Taylor PR. Tissue-resident macrophages. Nat Immunol. (2013) 14:986–95. doi: 10.1038/ni.2705
190. Martinez FO, Gordon S. The M1 and M2 paradigm of macrophage activation: time for reassessment. F1000prime Rep. (2014) 6:13. doi: 10.12703/P
191. Murray PJ, Allen JE, Biswas SK, Fisher EA, Gilroy DW, Goerdt S, et al. Macrophage activation and polarization: nomenclature and experimental guidelines. Immunity. (2014) 41:14–20. doi: 10.1016/j.immuni.2014.06.008
192. Nedeva C. Inflammation and cell death of the innate and adaptive immune system during sepsis. Biomolecules. (2021) 11(7):1011. doi: 10.3390/biom11071011
193. Errea A, Cayet D, Marchetti P, Tang C, Kluza J, Offermanns S, et al. Lactate inhibits the pro-inflammatory response and metabolic reprogramming in murine macrophages in a GPR81-independent manner. PloS One. (2016) 11:e0163694. doi: 10.1371/journal.pone.0163694
194. Zhou HC, Yu WW, Yan XY, Liang XQ, Ma XF, Long JP, et al. Lactate-driven macrophage polarization in the inflammatory microenvironment alleviates intestinal inflammation. Front Immunol. (2022) 13:1013686. doi: 10.3389/fimmu.2022.1013686
195. Colegio OR, Chu NQ, Szabo AL, Chu T, Rhebergen AM, Jairam V, et al. Functional polarization of tumour-associated macrophages by tumour-derived lactic acid. Nature. (2014) 513:559–63. doi: 10.1038/nature13490
196. Bohn T, Rapp S, Luther N, Klein M, Bruehl TJ, Kojima N, et al. Tumor immunoevasion via acidosis-dependent induction of regulatory tumor-associated macrophages. Nat Immunol. (2018) 19:1319–29. doi: 10.1038/s41590-018-0226-8
197. Liu N, Luo J, Kuang D, Xu S, Duan Y, Xia Y, et al. Lactate inhibits ATP6V0d2 expression in tumor-associated macrophages to promote HIF-2α-mediated tumor progression. J Clin Invest. (2019) 129:631–46. doi: 10.1172/JCI123027
198. Zhang L, Li S. Lactic acid promotes macrophage polarization through MCT-HIF1α signaling in gastric cancer. Exp Cell Res. (2020) 388:111846. doi: 10.1016/j.yexcr.2020.111846
199. Yan Y, Li X, Yang Q, Zhang H, Hettinga K, Li H, et al. Dietary d-lactate intake facilitates inflammatory resolution by modulating M1 macrophage polarization. Mol Nutr Food Res. (2022) 66:e2200196. doi: 10.1002/mnfr.202200196
200. Noe JT, Rendon BE, Geller AE, Conroy LR, Morrissey SM, Young LEA, et al. Lactate supports a metabolic-epigenetic link in macrophage polarization. Sci Adv. (2021) 7:eabi8602. doi: 10.1126/sciadv.abi8602
201. Yu J, Chai P, Xie M, Ge S, Ruan J, Fan X, et al. Histone lactylation drives oncogenesis by facilitating m(6)A reader protein YTHDF2 expression in ocular melanoma. Genome Biol. (2021) 22:85. doi: 10.1186/s13059-021-02308-z
202. An YJ, Jo S, Kim JM, Kim HS, Kim HY, Jeon SM, et al. Lactate as a major epigenetic carbon source for histone acetylation via nuclear LDH metabolism. Exp Mol Med. (2023) 55:2238–47. doi: 10.1038/s12276-023-01095-w
203. Latham T, Mackay L, Sproul D, Karim M, Culley J, Harrison DJ, et al. Lactate, a product of glycolytic metabolism, inhibits histone deacetylase activity and promotes changes in gene expression. Nucleic Acids Res. (2012) 40:4794–803. doi: 10.1093/nar/gks066
204. Shi W, Cassmann TJ, Bhagwate AV, Hitosugi T, Ip WKE. Lactic acid induces transcriptional repression of macrophage inflammatory response via histone acetylation. Cell Rep. (2024) 43:113746. doi: 10.1016/j.celrep.2024.113746
205. Chu X, Di C, Chang P, Li L, Feng Z, Xiao S, et al. Lactylated histone H3K18 as a potential biomarker for the diagnosis and predicting the severity of septic shock. Front Immunol. (2021) 12:786666. doi: 10.3389/fimmu.2021.786666
206. Dai X, Lv X, Thompson EW, Ostrikov KK. Histone lactylation: epigenetic mark of glycolytic switch. Trends Genet. (2022) 38:124–7. doi: 10.1016/j.tig.2021.09.009
207. Dichtl S, Lindenthal L, Zeitler L, Behnke K, Schlosser D, Strobl B, et al. Lactate and IL6 define separable paths of inflammatory metabolic adaptation. Sci Adv. (2021) 7(26):eabg3505. doi: 10.1126/sciadv.abg3505
208. Wang H, Bloom O, Zhang M, Vishnubhakat JM, Ombrellino M, Che J, et al. HMG-1 as a late mediator of endotoxin lethality in mice. Sci (New York NY). (1999) 285:248–51. doi: 10.1126/science.285.5425.248
209. Deng M, Tang Y, Li W, Wang X, Zhang R, Zhang X, et al. The endotoxin delivery protein HMGB1 mediates caspase-11-dependent lethality in sepsis. Immunity. (2018) 49:740–53.e7. doi: 10.1016/j.immuni.2018.08.016
210. Li J, Zhu CS, He L, Qiang X, Chen W, Wang H. A two-decade journey in identifying high mobility group box 1 (HMGB1) and procathepsin L (pCTS-L) as potential therapeutic targets for sepsis. Expert Opin Ther Targets. (2023) 27:575–91. doi: 10.1080/14728222.2023.2239495
211. Liu D, Huang SY, Sun JH, Zhang HC, Cai QL, Gao C, et al. Sepsis-induced immunosuppression: mechanisms, diagnosis and current treatment options. Military Med Res. (2022) 9:56. doi: 10.1186/s40779-022-00422-y
212. Liebold I, Al Jawazneh A, Casar C, Lanzloth C, Leyk S, Hamley M, et al. Apoptotic cell identity induces distinct functional responses to IL-4 in efferocytic macrophages. Sci (New York NY). (2024) 384:eabo7027. doi: 10.1126/science.abo7027
213. Man SM, Karki R, Kanneganti TD. Molecular mechanisms and functions of pyroptosis, inflammatory caspases and inflammasomes in infectious diseases. Immunol Rev. (2017) 277:61–75. doi: 10.1111/imr.12534
214. Kang R, Zeng L, Zhu S, Xie Y, Liu J, Wen Q, et al. Lipid peroxidation drives gasdermin D-mediated pyroptosis in lethal polymicrobial sepsis. Cell Host Microbe. (2018) 24:97–108.e4. doi: 10.1016/j.chom.2018.05.009
215. Nakahira K, Haspel JA, Rathinam VA, Lee SJ, Dolinay T, Lam HC, et al. Autophagy proteins regulate innate immune responses by inhibiting the release of mitochondrial DNA mediated by the NALP3 inflammasome. Nat Immunol. (2011) 12:222–30. doi: 10.1038/ni.1980
216. Hampton T. Autophagy genes linked to sepsis survival in mice. Jama. (2019) 322:1244–5. doi: 10.1001/jama.2019.14766
217. Tang D, Kang R, Livesey KM, Cheh CW, Farkas A, Loughran P, et al. Endogenous HMGB1 regulates autophagy. J Cell Biol. (2010) 190:881–92. doi: 10.1083/jcb.200911078
218. Wang J, Yang P, Yu T, Gao M, Liu D, Zhang J, et al. Lactylation of PKM2 suppresses inflammatory metabolic adaptation in pro-inflammatory macrophages. Int J Biol Sci. (2022) 18:6210–25. doi: 10.7150/ijbs.75434
219. Witkin SS, Alvi S, Bongiovanni AM, Linhares IM, Ledger WJ. Lactic acid stimulates interleukin-23 production by peripheral blood mononuclear cells exposed to bacterial lipopolysaccharide. FEMS Immunol Med Microbiol. (2011) 61:153–8. doi: 10.1111/j.1574-695X.2010.00757.x
220. Samuvel DJ, Sundararaj KP, Nareika A, Lopes-Virella MF, Huang Y. Lactate boosts TLR4 signaling and NF-kappaB pathway-mediated gene transcription in macrophages via monocarboxylate transporters and MD-2 up-regulation. J Immunol (Baltimore Md: 1950). (2009) 182:2476–84. doi: 10.4049/jimmunol.0802059
221. Schilperoort M, Ngai D, Katerelos M, Power DA, Tabas I. PFKFB2-mediated glycolysis promotes lactate-driven continual efferocytosis by macrophages. Nat Metab. (2023) 5:431–44. doi: 10.1038/s42255-023-00736-8
222. Guo Y, Patil NK, Luan L, Bohannon JK, Sherwood ER. The biology of natural killer cells during sepsis. Immunology. (2018) 153:190–202. doi: 10.1111/imm.12854
223. Wang F, Cui Y, He D, Gong L, Liang H. Natural killer cells in sepsis: Friends or foes? Front Immunol. (2023) 14:1101918. doi: 10.3389/fimmu.2023.1101918
224. Sherwood ER, Lin CY, Tao W, Hartmann CA, Dujon JE, French AJ, et al. Beta 2 microglobulin knockout mice are resistant to lethal intraabdominal sepsis. Am J Respir Crit Care Med. (2003) 167:1641–9. doi: 10.1164/rccm.200208-950OC
225. Guo Y, Luan L, Patil NK, Wang J, Bohannon JK, Rabacal W, et al. IL-15 enables septic shock by maintaining NK cell integrity and function. J Immunol (Baltimore Md: 1950). (2017) 198:1320–33. doi: 10.4049/jimmunol.1601486
226. Andaluz-Ojeda D, Iglesias V, Bobillo F, Almansa R, Rico L, Gandía F, et al. Early natural killer cell counts in blood predict mortality in severe sepsis. Crit Care (London England). (2011) 15:R243. doi: 10.1186/cc10501
227. Goldmann O, Chhatwal GS, Medina E. Contribution of natural killer cells to the pathogenesis of septic shock induced by Streptococcus pyogenes in mice. J Infect Dis. (2005) 191:1280–6. doi: 10.1086/428501
228. Holub M, Kluckova Z, Beneda B, Hobstova J, Huzicka I, Prazak J, et al. Changes in lymphocyte subpopulations and CD3+/DR+ expression in sepsis. Clin Microbiol Infect. (2000) 6:657–60. doi: 10.1046/j.1469-0691.2000.00175.x
229. Chiche L, Forel JM, Thomas G, Farnarier C, Cognet C, Guervilly C, et al. Interferon-gamma production by natural killer cells and cytomegalovirus in critically ill patients. Crit Care Med. (2012) 40:3162–9. doi: 10.1097/CCM.0b013e318260c90e
230. Pastille E, Pohlmann S, Wirsdorfer F, Reib A, Flohe SB. A disturbed interaction with accessory cells upon opportunistic infection with Pseudomonas aeruginosa contributes to an impaired IFN-gamma production of NK cells in the lung during sepsis-induced immunosuppression. Innate Immun. (2015) 21:115–26. doi: 10.1177/1753425913517274
231. Wang L, Zhang G, Sun W, Zhang Y, Tian Y, Yang X, et al. Comprehensive analysis of immune cell landscapes revealed that immune cell ratio eosinophil/B cell memory is predictive of survival in sepsis. Eur J Med Res. (2023) 28:565. doi: 10.1186/s40001-023-01506-8
232. Jiang W, Li X, Wen M, Liu X, Wang K, Wang Q, et al. Increased percentage of PD-L1(+) natural killer cells predicts poor prognosis in sepsis patients: a prospective observational cohort study. Crit Care (London England). (2020) 24:617. doi: 10.1186/s13054-020-03329-z
233. Darden DB, Dong X, Brusko MA, Kelly L, Fenner B, Rincon JC, et al. A novel single cell RNA-seq analysis of non-myeloid circulating cells in late sepsis. Front Immunol. (2021) 12:696536. doi: 10.3389/fimmu.2021.696536
234. Jensen IJ, Winborn CS, Fosdick MG, Shao P, Tremblay MM, Shan Q, et al. Polymicrobial sepsis influences NK-cell-mediated immunity by diminishing NK-cell-intrinsic receptor-mediated effector responses to viral ligands or infections. PloS pathogens. (2018) 14:e1007405. doi: 10.1371/journal.ppat.1007405
235. Giamarellos-Bourboulis EJ, Tsaganos T, Spyridaki E, Mouktaroudi M, Plachouras D, Vaki I, et al. Early changes of CD4-positive lymphocytes and NK cells in patients with severe Gram-negative sepsis. Crit Care (London England). (2006) 10:R166. doi: 10.1186/cc5111
236. Dodard G, Tata A, Erick TK, Jaime D, Miah SMS, Quatrini L, et al. Inflammation-induced lactate leads to rapid loss of hepatic tissue-resident NK cells. Cell Rep. (2020) 32:107855. doi: 10.1016/j.celrep.2020.107855
237. Harmon C, Robinson MW, Hand F, Almuaili D, Mentor K, Houlihan DD, et al. Lactate-mediated acidification of tumor microenvironment induces apoptosis of liver-resident NK cells in colorectal liver metastasis. Cancer Immunol Res. (2019) 7:335–46. doi: 10.1158/2326-6066.CIR-18-0481
238. Brand A, Singer K, Koehl GE, Kolitzus M, Schoenhammer G, Thiel A, et al. LDHA-associated lactic acid production blunts tumor immunosurveillance by T and NK cells. Cell Metab. (2016) 24:657–71. doi: 10.1016/j.cmet.2016.08.011
239. Long Y, Gao Z, Hu X, Xiang F, Wu Z, Zhang J, et al. Downregulation of MCT4 for lactate exchange promotes the cytotoxicity of NK cells in breast carcinoma. Cancer Med. (2018) 7:4690–700. doi: 10.1002/cam4.1713
240. Husain Z, Huang Y, Seth P, Sukhatme VP. Tumor-derived lactate modifies antitumor immune response: effect on myeloid-derived suppressor cells and NK cells. J Immunol (Baltimore Md: 1950). (2013) 191:1486–95. doi: 10.4049/jimmunol.1202702
241. Ge W, Meng L, Cao S, Hou C, Zhu X, Huang D, et al. The SIX1/LDHA axis promotes lactate accumulation and leads to NK cell dysfunction in pancreatic cancer. J Immunol Res. (2023) 2023:6891636. doi: 10.1155/2023/6891636
242. Wang H, Grzywacz B, Sukovich D, McCullar V, Cao Q, Lee AB, et al. The unexpected effect of cyclosporin A on CD56+CD16- and CD56+CD16+ natural killer cell subpopulations. Blood. (2007) 110:1530–9. doi: 10.1182/blood-2006-10-048173
243. Bendickova K, Fric J. Roles of IL-2 in bridging adaptive and innate immunity, and as a tool for cellular immunotherapy. J leukocyte Biol. (2020) 108:427–37. doi: 10.1002/JLB.5MIR0420-055R
244. Wang ZH, Zhang P, Peng WB, Ye LL, Xiang X, Wei XS, et al. Altered phenotypic and metabolic characteristics of FOXP3(+)CD3(+)CD56(+) natural killer T (NKT)-like cells in human Malignant pleural effusion. Oncoimmunology. (2023) 12:2160558. doi: 10.1080/2162402X.2022.2160558
245. Balan S, Arnold-Schrauf C, Abbas A, Couespel N, Savoret J, Imperatore F, et al. Large-scale human dendritic cell differentiation revealing notch-dependent lineage bifurcation and heterogeneity. Cell Rep. (2018) 24:1902–15.e6. doi: 10.1016/j.celrep.2018.07.033
246. Marin E, Bouchet-Delbos L, Renoult O, Louvet C, Nerriere-Daguin V, Managh AJ, et al. Human tolerogenic dendritic cells regulate immune responses through lactate synthesis. Cell Metab. (2019) 30:1075–90.e8. doi: 10.1016/j.cmet.2019.11.011
247. Brown TP, Bhattacharjee P, Ramachandran S, Sivaprakasam S, Ristic B, Sikder MOF, et al. The lactate receptor GPR81 promotes breast cancer growth via a paracrine mechanism involving antigen-presenting cells in the tumor microenvironment. Oncogene. (2020) 39:3292–304. doi: 10.1038/s41388-020-1216-5
248. Chen DS, Mellman I. Oncology meets immunology: the cancer-immunity cycle. Immunity. (2013) 39:1–10. doi: 10.1016/j.immuni.2013.07.012
249. Palucka K, Banchereau J. Cancer immunotherapy via dendritic cells. Nat Rev Cancer. (2012) 12:265–77. doi: 10.1038/nrc3258
250. Gottfried E, Kunz-Schughart LA, Ebner S, Mueller-Klieser W, Hoves S, Andreesen R, et al. Tumor-derived lactic acid modulates dendritic cell activation and antigen expression. Blood. (2006) 107:2013–21. doi: 10.1182/blood-2005-05-1795
251. Fan X, Liu Z, Jin H, Yan J, Liang HP. Alterations of dendritic cells in sepsis: featured role in immunoparalysis. BioMed Res Int. (2015) 2015:903720. doi: 10.1155/2015/903720
252. Goetze K, Walenta S, Ksiazkiewicz M, Kunz-Schughart LA, Mueller-Klieser W. Lactate enhances motility of tumor cells and inhibits monocyte migration and cytokine release. Int J Oncol. (2011) 39:453–63. doi: 10.3892/ijo
253. Sangsuwan R, Thuamsang B, Pacifici N, Allen R, Han H, Miakicheva S, et al. Lactate exposure promotes immunosuppressive phenotypes in innate immune cells. Cell Mol bioengineering. (2020) 13:541–57. doi: 10.1007/s12195-020-00652-x
254. Raychaudhuri D, Bhattacharya R, Sinha BP, Liu CSC, Ghosh AR, Rahaman O, et al. Lactate induces pro-tumor reprogramming in intratumoral plasmacytoid dendritic cells. Front Immunol. (2019) 10:1878. doi: 10.3389/fimmu.2019.01878
255. Ng CT, Oldstone MB. Infected CD8alpha- dendritic cells are the predominant source of IL-10 during establishment of persistent viral infection. Proc Natl Acad Sci United States America. (2012) 109:14116–21. doi: 10.1073/pnas.1211910109
256. Bhattacharyya S, Sen P, Wallet M, Long B, Baldwin AS Jr., Tisch R. Immunoregulation of dendritic cells by IL-10 is mediated through suppression of the PI3K/Akt pathway and of IkappaB kinase activity. Blood. (2004) 104:1100–9. doi: 10.1182/blood-2003-12-4302
257. De Smedt T, Van Mechelen M, De Becker G, Urbain J, Leo O, Moser M. Effect of interleukin-10 on dendritic cell maturation and function. Eur J Immunol. (1997) 27:1229–35. doi: 10.1002/eji.1830270526
258. Kryczanowsky F, Raker V, Graulich E, Domogalla MP, Steinbrink K. IL-10-modulated human dendritic cells for clinical use: identification of a stable and migratory subset with improved tolerogenic activity. J Immunol (Baltimore Md: 1950). (2016) 197:3607–17. doi: 10.4049/jimmunol.1501769
259. Chang J, Kunkel SL, Chang CH. Negative regulation of MyD88-dependent signaling by IL-10 in dendritic cells. Proc Natl Acad Sci United States America. (2009) 106:18327–32. doi: 10.1073/pnas.0905815106
260. Monti M, Vescovi R, Consoli F, Farina D, Moratto D, Berruti A, et al. Plasmacytoid dendritic cell impairment in metastatic melanoma by lactic acidosis. Cancers. (2020) 12(8):2085. doi: 10.3390/cancers12082085
261. Nasi A, Fekete T, Krishnamurthy A, Snowden S, Rajnavölgyi E, Catrina AI, et al. Dendritic cell reprogramming by endogenously produced lactic acid. J Immunol (Baltimore Md: 1950). (2013) 191:3090–9. doi: 10.4049/jimmunol.1300772
262. Kiran D, Basaraba RJ. Lactate metabolism and signaling in tuberculosis and cancer: A comparative review. Front Cell infection Microbiol. (2021) 11:624607. doi: 10.3389/fcimb.2021.624607
263. Certo M, Tsai CH, Pucino V, Ho PC, Mauro C. Lactate modulation of immune responses in inflammatory versus tumour microenvironments. Nat Rev Immunol. (2021) 21:151–61. doi: 10.1038/s41577-020-0406-2
264. Ranganathan P, Shanmugam A, Swafford D, Suryawanshi A, Bhattacharjee P, Hussein MS, et al. GPR81, a cell-surface receptor for lactate, regulates intestinal homeostasis and protects mice from experimental colitis. J Immunol (Baltimore Md: 1950). (2018) 200:1781–9. doi: 10.4049/jimmunol.1700604
265. Dietl K, Renner K, Dettmer K, Timischl B, Eberhart K, Dorn C, et al. Lactic acid and acidification inhibit TNF secretion and glycolysis of human monocytes. J Immunol (Baltimore Md: 1950). (2010) 184:1200–9. doi: 10.4049/jimmunol.0902584
266. Puig-Kröger A, Pello OM, Muñiz-Pello O, Selgas R, Criado G, Bajo MA, et al. Peritoneal dialysis solutions inhibit the differentiation and maturation of human monocyte-derived dendritic cells: effect of lactate and glucose-degradation products. J leukocyte Biol. (2003) 73:482–92. doi: 10.1189/jlb.0902451
267. Ratter JM, Rooijackers HMM, Hooiveld GJ, Hijmans AGM, de Galan BE, Tack CJ, et al. In vitro and in vivo Effects of Lactate on Metabolism and Cytokine Production of Human Primary PBMCs and Monocytes. Front Immunol. (2018) 9:2564. doi: 10.3389/fimmu.2018.02564
268. Faivre V, Lukaszewicz AC, Alves A, Charron D, Payen D, Haziot A. Accelerated in vitro differentiation of blood monocytes into dendritic cells in human sepsis. Clin Exp Immunol. (2007) 147:426–39. doi: 10.1111/j.1365-2249.2006.03287.x
269. Zheng LY, Duan Y, He PY, Wu MY, Wei ST, Du XH, et al. Dysregulated dendritic cells in sepsis: functional impairment and regulated cell death. Cell Mol Biol letters. (2024) 29:81. doi: 10.1186/s11658-024-00602-9
270. Chowdhury CS, Wareham E, Xu J, Kumar S, Kofron M, Lakshmikanthan S, et al. Rap1b-loss increases neutrophil lactate dehydrogenase activity to enhance neutrophil migration and acute inflammation in vivo. Front Immunol. (2022) 13:1061544. doi: 10.3389/fimmu.2022.1061544
271. Borregaard N. Neutrophils, from marrow to microbes. Immunity. (2010) 33:657–70. doi: 10.1016/j.immuni.2010.11.011
272. Liew PX, Kubes P. The neutrophil’s role during health and disease. Physiol Rev. (2019) 99:1223–48. doi: 10.1152/physrev.00012.2018
273. Hotchkiss RS, Monneret G, Payen D. Sepsis-induced immunosuppression: from cellular dysfunctions to immunotherapy. Nat Rev Immunol. (2013) 13:862–74. doi: 10.1038/nri3552
274. Quiroga J, Alarcón P, Manosalva C, Taubert A, Hermosilla C, Hidalgo MA, et al. Glycolysis and mitochondrial function regulate the radical oxygen species production induced by platelet-activating factor in bovine polymorphonuclear leukocytes. Veterinary Immunol immunopathology. (2020) 226:110074. doi: 10.1016/j.vetimm.2020.110074
275. Pan T, Sun S, Chen Y, Tian R, Chen E, Tan R, et al. Immune effects of PI3K/Akt/HIF-1α-regulated glycolysis in polymorphonuclear neutrophils during sepsis. Crit Care (London England). (2022) 26:29. doi: 10.1186/s13054-022-03893-6
276. Awasthi D, Nagarkoti S, Sadaf S, Chandra T, Kumar S, Dikshit M. Glycolysis dependent lactate formation in neutrophils: A metabolic link between NOX-dependent and independent NETosis. Biochim Biophys Acta Mol basis disease. (2019) 1865:165542. doi: 10.1016/j.bbadis.2019.165542
277. Wen X, Xie B, Yuan S, Zhang J. The “Self-sacrifice” of immuneCells in sepsis. Front Immunol. (2022) 13:833479. doi: 10.3389/fimmu.2022.833479
278. Fei M, Zhang H, Meng F, An G, Tang J, Tong J, et al. Enhanced lactate accumulation upregulates PD-L1 expression to delay neutrophil apoptosis in sepsis. View. (2024) 5(1):20230053. doi: 10.1002/VIW.20230053
279. Kolpen M, Dalby Sørensen C, Faurholt-Jepsen D, Hertz FB, Jensen P, Bestle MH. Endotracheal lactate reflects lower respiratory tract infections and inflammation in intubated patients. APMIS: Acta pathologica microbiologica immunologica Scandinavica. (2022) 130:507–14. doi: 10.1111/apm.13224
280. Fredman G, Kolpen M, Hertz FB, Petersen PT, Jensen AV, Baunbaek-Egelund G, et al. The inflamed sputum in lower respiratory tract infection: l-lactate levels are correlated to neutrophil accumulation. APMIS: Acta pathologica microbiologica immunologica Scandinavica. (2019) 127:72–9. doi: 10.1111/apm.12913
281. Nielsen BU, Kolpen M, Jensen P, Katzenstein T, Pressler T, Ritz C, et al. Neutrophil count in sputum is associated with increased sputum glucose and sputum L-lactate in cystic fibrosis. PloS One. (2020) 15:e0238524. doi: 10.1371/journal.pone.0238524
282. Huang JL, Zhou XX, Luo P, Lu XY, Liang LH, Lan GB, et al. Neutrophil-to-lymphocyte ratio and lactate dehydrogenase for early diagnosis of AIDS patients with Talaromyces marneffei infection. Ann palliative Med. (2022) 11:588–97. doi: 10.21037/apm
283. Karon BS, Tolan NV, Wockenfus AM, Block DR, Baumann NA, Bryant SC, et al. Evaluation of lactate, white blood cell count, neutrophil count, procalcitonin and immature granulocyte count as biomarkers for sepsis in emergency department patients. Clin Biochem. (2017) 50:956–8. doi: 10.1016/j.clinbiochem.2017.05.014
284. Liu Y, Zheng J, Zhang D, Jing L. Neutrophil-lymphocyte ratio and plasma lactate predict 28-day mortality in patients with sepsis. J Clin Lab Anal. (2019) 33:e22942. doi: 10.1002/jcla.22942
285. Renner O, Mayer M, Leischner C, Burkard M, Berger A, Lauer UM, et al. Systematic review of gossypol/AT-101 in cancer clinical trials. Pharm (Basel). (2022) 15(2):144. doi: 10.3390/ph15020144
286. Doherty JR, Cleveland JL. Targeting lactate metabolism for cancer therapeutics. J Clin Invest. (2013) 123:3685–92. doi: 10.1172/JCI69741
287. Stein MN, Goodin S, Gounder M, Gibbon D, Moss R, Portal D, et al. A phase I study of AT-101, a BH3 mimetic, in combination with paclitaxel and carboplatin in solid tumors. Invest New Drugs. (2020) 38:855–65. doi: 10.1007/s10637-019-00807-2
288. Ailawadhi S, Parrondo RD, Dutta N, Han B, Ciccio G, Cherukuri Y, et al. AT-101 enhances the antitumor activity of lenalidomide in patients with multiple myeloma. Cancers. (2023) 15(2):477. doi: 10.3390/cancers15020477
289. Fiveash JB, Ye X, Peerboom DM, Mikkelsen T, Chowdhary S, Rosenfeld M, et al. Clinical trials of R-(-)-gossypol (AT-101) in newly diagnosed and recurrent glioblastoma: NABTT 0602 and NABTT 0702. PloS One. (2024) 19:e0291128. doi: 10.1371/journal.pone.0291128
290. Song S, Chen Q, Li Y, Lei G, Scott A, Huo L, et al. Targeting cancer stem cells with a pan-BCL-2 inhibitor in preclinical and clinical settings in patients with gastroesophageal carcinoma. Gut. (2021) 70:2238–48. doi: 10.1136/gutjnl-2020-321175
291. Wang Q, Liu F, Tao W, Qian K. Timing of renal replacement therapy in patients with sepsis-associated acute kidney injury: A systematic review and meta-analysis. Aust Crit Care. (2024) 37:369–79. doi: 10.1016/j.aucc.2023.06.011
292. Barbar SD, Clere-Jehl R, Bourredjem A, Hernu R, Montini F, Bruyere R, et al. Timing of renal-replacement therapy in patients with acute kidney injury and sepsis. New Engl J Med. (2018) 379:1431–42. doi: 10.1056/NEJMoa1803213
293. Hellman T, Uusalo P, Jarvisalo MJ. Renal replacement techniques in septic shock. Int J Mol Sci. (2021) 22(19):10238. doi: 10.3390/ijms221910238
294. Borthwick EM, Hill CJ, Rabindranath KS, Maxwell AP, McAuley DF, Blackwood B. High-volume haemofiltration for sepsis in adults. Cochrane Database Syst Rev. (2017) 1:CD008075. doi: 10.1002/14651858.CD008075.pub3
295. Rice TW, Wheeler AP, Bernard GR, Vincent JL, Angus DC, Aikawa N, et al. A randomized, double-blind, placebo-controlled trial of TAK-242 for the treatment of severe sepsis. Crit Care Med. (2010) 38:1685–94. doi: 10.1097/CCM.0b013e3181e7c5c9
296. Alp E, Gonen ZB, Gundogan K, Esmaoglu A, Kaynar L, Cetin A, et al. The effect of mesenchymal stromal cells on the mortality of patients with sepsis and septic shock: A promising therapy. Emerg Med Int. (2022) 2022:9222379. doi: 10.1155/2022/9222379
297. Homma K, Bazhanov N, Hashimoto K, Shimizu M, Heathman T, Hao Q, et al. Mesenchymal stem cell-derived exosomes for treatment of sepsis. Front Immunol. (2023) 14:1136964. doi: 10.3389/fimmu.2023.1136964
298. Adu-Berchie K, Brockman JM, Liu Y, To TW, Zhang DKY, Najibi AJ, et al. Adoptive T cell transfer and host antigen-presenting cell recruitment with cryogel scaffolds promotes long-term protection against solid tumors. Nat Commun. (2023) 14:3546. doi: 10.1038/s41467-023-39330-7
299. Aziz M, Ode Y, Zhou M, Ochani M, Holodick NE, Rothstein TL, et al. B-1a cells protect mice from sepsis-induced acute lung injury. Mol Med. (2018) 24:26. doi: 10.1186/s10020-018-0029-2
300. Winkler J, Tittlbach H, Schneider A, Vasova I, Strobel J, Herold S, et al. Adoptive transfer of donor B lymphocytes: a phase 1/2a study for patients after allogeneic stem cell transplantation. Blood Adv. (2024) 8:2373–83. doi: 10.1182/bloodadvances.2023012305
301. Liu X, Li S, Cui Q, Guo B, Ding W, Liu J, et al. Activation of GPR81 by lactate drives tumour-induced cachexia. Nat Metab. (2024) 6:708–23. doi: 10.1038/s42255-024-01011-0
Keywords: sepsis, lactate, lactic acid, lactylation, immune cells, inflammation, immune response, immunosuppression
Citation: Zhang T, Chen L, Kueth G, Shao E, Wang X, Ha T, Williams DL, Li C, Fan M and Yang K (2024) Lactate’s impact on immune cells in sepsis: unraveling the complex interplay. Front. Immunol. 15:1483400. doi: 10.3389/fimmu.2024.1483400
Received: 19 August 2024; Accepted: 05 September 2024;
Published: 20 September 2024.
Edited by:
Guo-Chang Fan, University of Cincinnati, United StatesReviewed by:
Jimin Guo, Beijing University of Chemical Technology, ChinaSongyun Deng, Central South University, China
Wenxin Yu, Genentech Inc., United States
Copyright © 2024 Zhang, Chen, Kueth, Shao, Wang, Ha, Williams, Li, Fan and Yang. This is an open-access article distributed under the terms of the Creative Commons Attribution License (CC BY). The use, distribution or reproduction in other forums is permitted, provided the original author(s) and the copyright owner(s) are credited and that the original publication in this journal is cited, in accordance with accepted academic practice. No use, distribution or reproduction is permitted which does not comply with these terms.
*Correspondence: Kun Yang, yangk1@etsu.edu